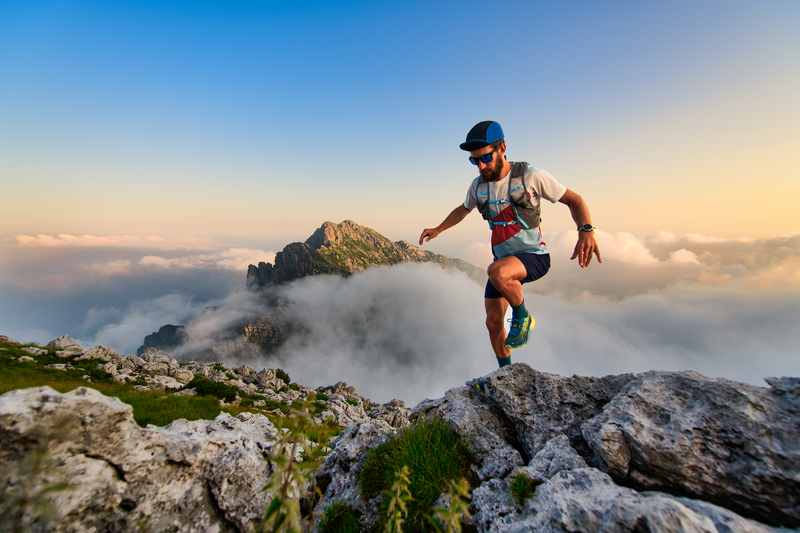
95% of researchers rate our articles as excellent or good
Learn more about the work of our research integrity team to safeguard the quality of each article we publish.
Find out more
ORIGINAL RESEARCH article
Front. Ecol. Evol. , 17 March 2020
Sec. Evolutionary Ecology of Social Behaviour
Volume 8 - 2020 | https://doi.org/10.3389/fevo.2020.00062
This article is part of the Research Topic Microbial Drivers of Sociality – from Multicellularity to Animal Societies View all 18 articles
External immune defense, such as antimicrobial secretions, is not generally viewed as part of the immune system. Nevertheless, it constitutes a first barrier to pathogens and manipulates microbial environments. Hygienic measures ranging from the protection of oneself or conspecifics, the nesting site, to stored food may be more efficient with secreted antimicrobials. However, the relationship between external immune defense and internal immunity, including potential life-history trade-offs, is not well-understood. As hymenopteran venom often contains antimicrobial peptides it could serve as an external immune defense. Assuming that antimicrobial venom is costly its production might be traded-off against the internal immune defense. Here we compared the antimicrobial activity of venom and hemolymph in fourteen different bumblebee species according to their life-history strategies and characteristics, i.e., overwintered queens, workers and young queens and cuckoo queens. We found no direct relation between antimicrobial activity of hemolymph and venom. Across all species, hemolymph mainly showed lysozyme-like activity (LLA) whereas venom mainly showed antimicrobial peptide (AMP) activity. While LLA activity in the hemolymph was similar among species and life-history strategies and characteristics, both factors significantly differed in venom AMP activity. Independent of body size or fat body content, young queens showed the highest venom AMP activity, followed by workers, overwintered queens, and cuckoo queens. Venom as a potential external immune trait seems not directly linked to internal immunity in bumblebees. However, the investment in external defense depends on the species and the life-history strategies and characteristics of an individual, such as social status or condition.
Organisms are constantly exposed to parasites and opportunistic microbial pathogens. Due to this constant microbial selection pressure, organisms have evolved numerous defense mechanisms, most of which are part of their immune system. Physical barriers, such as the skin or the insect cuticle, form a first line of defense. However, once pathogens have overcome this barrier, a complex interaction of humoral and cellular immune reactions exists to minimize the threat (Bulet et al., 1999; Lemaitre and Hoffmann, 2007). These immune responses can be constitutively expressed and/or induced upon recognition of a pathogen or of stress induced by pathogens (Schmid-Hempel, 2011). In insects, part of the constitutive immune effectors are peptides that circulate the hemolymph, for example lysozyme (Schmid-Hempel, 2011). Upon pathogen recognition, antimicrobial peptides (AMP) are induced and expressed in many different tissues (Schmid-Hempel, 2011). Both AMPs and lysozyme can be found in the fat body, in hemocytes (Cotter et al., 2004), in the midgut, in salivary glands (Hamilton et al., 2011), within reproductive organs (Samakovlis et al., 1991; Lung et al., 2001; Otti et al., 2009), and even on the cuticle (Ashida and Brey, 1995).
The maintenance and use of the immune system are costly and underly trade-offs with other life-history traits. Hosts should invest in immune defense mechanisms that reduce the risk of infection efficiently (Zuk and Stoehr, 2002; Moret, 2003). One option to minimize costs is to stop threats at the earliest moment possible by, for example, manipulating the microbial community in the immediate environment with externalized antimicrobial secretions (Otti et al., 2014). External immune defenses can be any heritable trait acting outside an organism, improving protection from pathogens or manipulating microbial composition in favor of the given organism and should therefore be seen as a part of an organisms' immune system (Otti et al., 2014). Similar to internal immune defense, allocation to external immune defense is predicted to depend on ecological characteristics, such as pathogen pressure, and life-history of an animal.
Antimicrobial secretions have been found in many different animal taxa, including insects. Such secretions occur in different glands of hymenopterans, e.g., in metapleural glands of ants (Tragust, 2016), in salivary glands of termites (Bulmer et al., 2009), and in the venom glands of ants (Tragust et al., 2013), bees (Kuhn-Nentwig, 2003), and wasps (Turillazzi et al., 2006). Both ants and honeybees have been shown to distribute antimicrobial secretions from their venom on their cuticle and nests to manipulate microbial communities in their environment. In this case, the venom serves as an external immune defense (reviewed in Tragust, 2016).
The connection between external and internal immune defenses and the role played by ecology and individual condition are not well-understood. Ecological and physiological aspects are known to influence internal immune defenses (Schmid-Hempel, 2005, 2011; Siva-Jothy et al., 2005; Adamo, 2009) and life-history theory implies that immune defense traits have costs and are traded-off against other fitness components (Sheldon and Verhulst, 1996; Moret and Schmid-Hempel, 2000). The same should apply to internal vs. external immune defense, also because the investment in immunity is selected to be optimal (Westra et al., 2015; Boots and Best, 2018). Baracchi and Turillazzi (2010) found venom components to vary with social status in honeybees, indicating that individuals with different life-history strategies or characteristics (i.e., different castes, eusocial, and parasitic life) might invest differently into external immune defenses. For example, during independent colony founding, queens of eusocial Hymenoptera need to care for the brood and keep potential pathogens at bay before the emergence of the first workers. This might require a larger investment into external immune defenses in comparison to a later life stage when queens are sheltered within the nest, cared for and groomed by workers. Then they may hardly ever have the need to use external immune defenses like venom, especially in comparison to a worker engaged in hygienic or defensive tasks in and around the nest.
Additionally, immune defenses may depend on the physiological condition of an individual (Schmid-Hempel, 2003; Cotter et al., 2019). Since resources are stored in the fat body, insects rely on their fat content to effectively use their immune defenses (Dolezal et al., 2019). The fat body, the production site of hemolymph proteins and several AMPs (Ferrandon et al., 2007; Dolezal et al., 2019), highlighting the importance of this organ as an important part of the immune system. Individuals with higher fat content relative to their body mass should be able to invest more in the production of antimicrobial peptides and have higher expression of immune defenses (Cotter et al., 2019).
At high population density, animals will generally experience a greater risk of pathogen exposure than at low density (Hochberg, 1991; Stow et al., 2007; Turnbull et al., 2011). Insects are known to be selected for higher immunity at higher densities, i.e., the expression of immune defenses is density-dependent. For example, several insect species show higher degrees of melanization of the cuticle, i.e., the insect skin is denser and thicker under high rearing densities than when raised alone (Reeson et al., 1998; Wilson et al., 2002). In social species, group size is obviously related to the density and the proximity of individuals within the nest (Naug and Camazine, 2002) and high relatedness among them (Baer and Schmid-Hempel, 1999) should make social insects highly vulnerable to pathogens. However, in social insects collectively performed immune defenses have evolved, such as allogrooming or the distribution of antimicrobial secretions among nestmates, that have been shown to effectively reduce pathogen pressure (Otti et al., 2014; Cremer et al., 2018). In commercial bumblebees, artificial group size manipulation led to higher innate immune gene expression in groups of workers relative to individually kept workers (Richter et al., 2012). Group size might, therefore, affect internal and external immune defenses.
In this study, we compared the internal immune defense with external immune defense traits in different bumblebee species (Hymenoptera, Apidae, Bombus spp.), by looking at antibacterial activity of hemolymph (internal) and venom (external) immunity. Bumblebees are very important as they provide pollinator services not only for our crops (Velthuis and van Doorn, 2006) but also in the wild, thereby maintaining plant diversity (Goulson et al., 2008). In Central and Northern Europe, bumblebees have an annual colony cycle with one generation per year, except for some short-lived species that can have two colony cycles, e.g., Pyrobombus pratorum (Sladen, 1912). Eusocial bumblebee colonies have a single reproductive queen (henceforth overwintered queens), non-reproductive female workers and, at the end of a colony cycle in autumn, produce new reproductive females (henceforth young queens) and males. While males die after mating, the then fertilized young queens enter hibernation and found a new colony the following spring (Sladen, 1912). The appearance of sexual and non-sexual individuals performing different tasks can be related to different behaviors and morphologies within life-history strategies and characteristics (O'Donnell et al., 2000). Colony survival, colony growth and production of sexuals depend on the reproductive success of the founding queen and the effort of her workers (Oster and Wilson, 1978). This dependency on one reproductive individual requires special protection of the queens, as they are responsible for the fitness of the whole colony (Cremer et al., 2007).
Before and during the colony founding stage, queens should invest more into their immune defenses to overcome infections. Whereas, after the first workers emerge, those can take care of their queen's health. Some bumblebee species of the subgenus Psithyrus or cuckoo bumblebees have evolved brood parasitism (further referred to as cuckoo queens). Cuckoo queens have an annual life cycle as well. They generally emerge later from hibernation than their host species and then readily search for young host colonies. When cuckoo queens find a host colony, they kill the host queen and enslave the workers to care for their brood (Fisher, 1988). Cuckoo queens produce only reproductive offspring of which again only the females overwinter. Cuckoo queens have a thicker sting and enlarged venom glands (Richards, 1927; Fisher and Sampson, 1992) to improve the probability of a successful colony take over. Such a difference in the life-history strategy of cuckoo queens compared to eusocial queens might have implications for their investment in external and internal immune defense. We expect that cuckoo queens are less well-cared for by the enslaved workers of their host colony in comparison to their own queens. Workers do not benefit from an increase in the cuckoo queen's fitness. Therefore, cuckoo queens need to force workers to provide brood care. Due to the defensive role of venom toward enemies as well as pathogens, cuckoo queens may invest more into external immune defenses in comparison to host queens to compensate for the poor care behaviors. Similarly, constitutively expressed internal immune defenses should be stronger in cuckoo queens.
Here, we compared the internal and external immune defenses of different common bumblebee species in Germany by comparing lysozyme-like activity (LLA) and antimicrobial (AMP) activity of the hemolymph to the venom gland contents. We characterized the variation in immune defenses within and across life-history strategies and characteristics, i.e., cuckoo queens, overwintered queens, young queens, and workers. We predict that different life-history strategies and characteristics should lead to different immune defense strategies in hemolymph and venom. Since reproductive success is based on the success of the reproductive females, we assume overwintered queens and young queens show stronger immune defenses than workers. We also expect young queens to show stronger immune defenses than overwintered queens, as they should have more resources than queens just emerging from hibernation. Predicting the extent of external immune defense for cuckoo bumblebees is less obvious. On the one hand, they might show stronger immune defenses than overwintered queens, because they cannot rely on workers taking care of them. On the other hand, they might show weaker immune defenses, because workers are already protecting the nest and the cuckoo queen only has to fend for herself. Additionally, the strength of immune defense should be linked to individual condition, i.e., size and lipid content stored in the fat body. We assume that individuals from species with large colonies show stronger internal, but weaker external immune defense, than individuals from species with small colonies. This assumption is based on the idea that in large colonies (>150 individuals) the individual contribution to the protection of the group might be smaller than in small colonies (<120 individuals). Finally, we test whether internal and external immune defenses are traded-off against each other to optimize allocation costs.
From April to September 2016 we collected a total of 342 individuals from 14 different bumblebee species (Table S1) in the region of Bayreuth (Upper Franconia, Bavaria, Germany). From nine eusocial bumblebee species we collected 78 overwintered queens between April and June, 59 young queens between July and September and 164 workers between April and September. In addition, we collected 41 cuckoo queens from five social parasite species of eusocial bumblebees between April and June (Table S1). The collection of samples was conducted with the permission of the government of Upper Franconia (Obere Naturschutzbehörde Oberfranken, Permit reference number 55.1-8646-1-7-24). All individuals were identified to the species-level using the identification key published by Gokcezade et al. (2010).
To minimize stress on collected individuals we processed all bumblebees within a maximum of 3 h after field collection. This immediate processing should also give a relatively accurate snapshot of the state of individual immunity and general individual physiology in the field. Once in the laboratory, feces samples were taken (Otti and Schmid-Hempel, 2008) (see Characterization of individual condition) and individuals were immobilized by chilling them on ice for 30 min. Then we collected hemolymph and venom. Hemolymph was collected by inserting a sterilized glass capillary pulled to a fine point (inside diameter 0.58 mm, GB100F-10, Science Products GmbH, Hofheim, Germany) between the sternites of the third and fourth abdominal segment. From the same individual we then collected venom, by first removing the venom gland from the abdomen by pulling the stinger. We used a sterilized glass capillary to remove the venom from the gland. All capillaries were calibrated beforehand to measure the total amount of venom. Hemolymph and venom samples were divided into two parts. One part was used for a lysozyme assay while the other part was used for a zone of inhibition assay to measure antimicrobial peptide activity.
To compare internal and external immune defense across species and female group (i.e., cuckoo, overwintered, and young queens and workers) we investigated a constitutive and an induced immune trait in all individuals. For this we characterized lysozyme-like activity (LLA) and antimicrobial peptide (AMP) activity of the hemolymph and venom, using a lysozyme assay (Otti et al., 2009) and a zone of inhibition assay (Haine et al., 2008), respectively. LLA is often constitutively expressed in insects and has a relatively broad range activity against bacteria (Nayduch and Joyner, 2013). AMP activity is normally induced (Broderick et al., 2009), but still present at rather low levels in non-challenged individuals. However, in many species AMPs are constitutively expressed in venom and antimicrobial secretions (Otti et al., 2014). AMPs are more specific in their activity against bacteria and are among the most potent antimicrobial agents in nature (Broderick et al., 2009).
We prepared 24-well culture plate lids (Nunclon, D7039, Sigma-Aldrich) with 10 ml of bacterial agar [500 mg agar, 50 mg streptomycin sulfate, 1 ml Triton-X, 50 ml dH2O, 250 mg lyophilized Micrococcus lysodeikticus (ATCC No. 4698, M0508, Sigma-Aldrich)]. In a flask the mixture was heated to ~100°C until all components had fully dissolved. Streptomycin sulfate (S6501, Sigma-Aldrich; 0.1 mg/mL) was added to prevent microbial contamination of the plates. We punched 24 equidistant holes (1.5 mm in diameter) into each agar plate using sterile pipette tips. We randomly distributed hemolymph and venom samples of different individuals across plates. From each individual we introduced 1 μl of hemolymph into a hole. For the venom, due to body size differences we could consistently harvest 0.5 μl of venom from queens and 0.25 μl from workers to transfer into the holes. LLA plates were incubated for 48 h at 30°C in an LTE® Raven incubator (Greenfield, Oldham, United Kingdom).
To measure AMP activity, we followed the zone of inhibition assay protocol developed by Haine et al. (2008). For this the gram-positive bacteria Arthrobacter globiformis (ATCC no. 8010) was cultivated from a glycerol stock, stored at −80°C, on LB-agar (10 g tryptone, 5 g yeast, 10 g NaCl, 15 g agar, 1 l H2O) and incubated at 30°C for 24 h in an LTE® Raven incubator (Greenfield, Oldham, United Kingdom). After incubation, one single colony was picked and transferred into 5ml sterile LB broth (10 g tryptone, 5 g yeast, 10 g NaCl, 1 l H2O) to prepare a liquid culture, which was incubated at 30°C for 24 h in a shaking incubator (LTE® Raven incubator, Greenfield, Oldham, United Kingdom) at 150 rpm. 0.1 ml of the liquid culture was transferred into 50 ml of 1% sterile agar at 44°C. From this, assay plates were prepared by pouring 5ml of bacteria-containing agar into a 90mm petri dish. Nine equidistant holes (1.5 mm in diameter) were punched in the agar using sterile pipette tips. One hole was left empty to serve as a sterile control. We randomly distributed hemolymph and venom samples of different individuals across plates. As for LLA we put 1 μl hemolymph from all individuals and 0.5 μl of venom from queens and 0.25 μl from workers into the holes. ZIA plates were incubated for 24 h at 30°C.
After incubation, each plate was photographed using a Gel iX Imager (INTAS Science Imaging Instruments GmbH, Göttingen, Germany) with matching software INTAS GDS (INTAS Science Imaging Instruments GmbH, Göttingen, Germany). For each inhibition zone on a given plate the diameter was measured twice using ImageJ (version 1.50i; Schneider et al., 2012). The zone area from the lysozyme assay was then converted to units of lysozyme using a standard curve (Figure S9 and Supplementary Information) to make LLA comparable to other studies. For AMP activity the zone areas in mm2 were used as a dependent variable.
In addition, we extracted mean colony sizes and size ranges of the sampled bumblebee species from the literature (Table S1) to investigate a potential link between immune function and sociality or group size as was shown for other bee species by Stow et al. (2007).
As we expect individual condition to influence the investment in immune defenses, be it internal or external, we checked for infections in the feces (Otti and Schmid-Hempel, 2008), measured body size and fat body content of all sampled individuals. Immediately after bringing individuals into the laboratory, feces were screened for different parasites (e.g., Nosema sp., trypanosome parasites) under a light microscope. To our astonishment no visible infection with trypanosomes (Crithidia bombi, C. expoeki or Lotmaria sp.) could be detected, because for example Crithidia bombi often shows quite high prevalence during the summer months (Popp et al., 2012). Approximately 1.5% (5 out of 342; 1 worker, 4 young queens) of the individuals showed spores of microsporidia (Nosema sp.). Roughly 23% (77 out of 342) of the individuals had phoretic mites (14% in cuckoo queens (6 out of 41), 34% in overwintered queens (27 out 78), 27% in young queens (16 out of 59) and 17% in workers (28 out of 164). All individuals were assessed for internal and external immune defense.
After dissection, the wings from each bumblebee were removed and the length of the radial cell of the right forewing was measured to the nearest 0.001 mm using ImageJ. The length of the radial cell of the right forewing is a surrogate for body size because this measure is independent of body mass (Medler, 1962; Owen, 1988, 1989). Next, the fat body content of individuals was measured using the lipid extraction protocol developed by Bazazi et al. (2016). First, each bumblebee was placed in a single 15 ml reaction tube and dried at 70°C for 5 days in an UFE 600 compartment drier (memmert, Schwabach, Germany). Then, dry bumblebees were weighed to the nearest 0.01 mg using an OHAUS Explorer balance (OHAUS Europe GmbH, Greifensee, Switzerland). After measuring initial dry body weight, 5 ml of chloroform was added to each reaction tube. To wash out all lipids the chloroform was replaced three times every 24 h. After 72 h the chloroform was removed, and bumblebees were dried for 5 days and weighed to the nearest 0.01 mg to get a measure for dry weight after fat body extraction. The fat body content of each individual was calculated from the difference between initial dry weight and dry weight after fat body extraction. Finally, we calculated the proportion of fat body as a function of initial dry body weight, i.e., fat content relative to dry body weight, as an estimate of individual condition.
The data was analyzed using the statistical platform R version 3.6.1 (R Core Team, 2019). First, with a principal component analysis we investigated if the four female groups formed clusters (see Supplementary Information). The female groups clustered into three groups. Workers were well-separated from overwintered and cuckoo queens and overlapped to some extent with young queens (Figure S2). The young queens also overlapped with the overwintered and cuckoo queens. Finally, the overwintered and cuckoo queens formed one group in the PCA (Figure S2). Due to the clustering of the different eusocial female groups and the extremely different life-history strategy of cuckoo queens, we decided to investigate significant differences in the measured traits between the four female groups. Because parasitic species contained only one female group and eusocial species three, we analyzed the effects of female group and species separately. Therefore, in the first set of models, we used the fixed factors female group and immune defense, i.e., internal vs. external, and their interaction term with species as a random effect. In the second set of models, we used species and immune defense and their interaction term as fixed effects with female group as a random effect. Using the R packages car (Fox and Weisberg, 2011), nlme (Pinheiro et al., 2019) and multcomp (Hothorn et al., 2008), we analyzed the presence-absence of internal and external expression of an immune defense separately for LLA and AMP activity. We fitted linear mixed-effects models (LME) with a binary response variable for the presence or absence of LLA and AMP activity, respectively. Because body size significantly differed between female groups (LME: X2 = 167.09, df = 3, p < 0.001, all pairwise Tukey comparisons: p < 0.05) and species (LME: X2 = 429.48, df = 13, p < 0.001) (Figure S8), we fitted radial cell length as a covariate to control for body size. To account for this procedure in the statistical analysis of immune activity, we represent both immune measures relative to radial cell length on the y-axis in our figures. To analyze the effect of condition on the probability to show an immune defense, we fitted body fat content relative to dry body weight as a covariate. As fixed factors, we first fitted female group and immune defense, i.e., internal vs. external, and their interaction term and in a second series of models, species and immune defense. As random effects, we fitted individual nested within female group nested within species to account for the hierarchical nature of the data and variation between species in the models with the female group as a fixed factor. In the models with species as a fixed factor, we nested individual within female group nested within species as a random effect.
For the analysis of internal and external immune expression, we first removed 26 individuals from nine different species, because they expressed neither LLA nor AMP activity, neither internally nor externally (cuckoo queen: 1 individual, overwintered queen: 11, young queen: 2, worker: 12). Using the R packages glmmTMB (Brooks et al., 2017), DHARMa (Hartig, 2019), and multcomp (Hothorn et al., 2008), we then fitted linear mixed-effects models (LME) with LLA and AMP as response variables to investigate effects of body size, condition, internal vs. external defense, female group and species. The package glmmTMB allowed us to account for zero inflation in the expression of LLA and AMP activity in the LMEs. As fixed factors we first fitted female group and immune defense, i.e., internal vs. external, and their interaction term and in a second series of models we fitted species and immune defense as fixed factors. We again fitted radial cell length as a covariate to control for body size effects. To analyze the effect of condition on immune defense we fitted body fat content relative to dry body weight as a covariate. As random effects we fitted individual nested within female group nested within species to account for the hierarchical nature of the data and for variation between species in the models with the female group as a fixed factor. In the models with species as a fixed factor we nested individual within female group and species as a random effect. If female group or species showed a significant effect, we ran multiple comparisons to identify differences among immune defense mechanisms adjusting p-values according to Westfall (Bretz et al., 2010).
To investigate associations between traits we accounted for phylogenetic relatedness of the different bumblebee species by running phylogenetic comparative analysis with phylogenetic generalized least-squares (PGLS) using the R packages Rphylip (Felsenstein, 2005; Revell and Chamberlain, 2014) and caper (Orme et al., 2018). First, we constructed a phylogenetic tree using four DNA sequences from the literature with the software Geneious (see Supplementary Information, Table S2). Second, we analyzed the strength of the antimicrobial activity of individuals in relation to colony size in the eusocial species. For this we calculated the mean external and internal LLA and AMP activity, i.e., mean units of lysozyme per μl and zone area in mm2, respectively, for each female group and species. We used PGLS to relate each immune defense mechanism to mean colony size of the sampled bumblebee species (extracted from literature; Table S1).
Third, we investigated a general link in the expression of internal and external immune defense with condition we additionally tested for associations between external LLA and AMP activity, between internal LLA and external AMP, as well as all between four immune defense traits and fat content relative to dry body weight fitting PGLS.
Total fat content was significantly positively correlated with initial dry body weight (PGLS: estimate = 0.071, SE = 0.013, R2 = 0.72, t = 5.620, p < 0.0001) and body size (PGLS: estimate = 0.041, SE = 0.006, R2 = 0.79, t = 6.749, p < 0.0001). However, body condition (fat content relative to dry body weight) did not correlate with body size (PGLS: estimate = −0.042, SE = 0.046, R2 = 0.07, t = −0.915, p = 0.38). Initial dry body weight was significantly positively correlated with body size (PGLS: estimate = 0.547, SE = 0.030, R2 = 0.96, t = 18.017, p < 0.0001).
Overall, the three different types of queens and workers significantly affected the proportion of individuals showing internal or external LLA (LME: X2 = 13.304, df = 3, p < 0.01) (Figure 1A), but not AMP activity (LME: X2 = 2.760, df = 3, p = 0.43) (Figure 1B). Young queens and workers showed the highest proportions of LLA expression and young queens had a significantly higher proportion of individuals expressing LLA than overwintered queens (Tukey comparison: p < 0.01).
Figure 1. Proportion of individuals showing constitutive expression of (A) LLA and (B) AMP activity for both internal (hemolymph) and external (venom) immune defense for each female group. Positive tests for internal immune defense are shown in light gray and for external immune defense in dark gray. Bars give mean proportions with error bars representing one standard error and black circles give the proportion for each species in a female group.
Overall, we found that species significantly varied in the proportion of individuals expressing LLA (LME: X2 = 31.740, df = 13, p < 0.01) (Figures 2A,B) and AMP activity (LME: X2 = 59.715, df = 13, p < 0.001) (Figures 2C,D). Two species comparisons showed significantly different proportions of individuals expressing LLA (B. pratorum vs. B. lucorum and B. pratorum vs. B. sylvarum: Tukey comparisons: p < 0.05; all other Tukey comparisons p > 0.05). For LLA the proportion of individuals expressing internal or external immunity depended on the species (LME: X2 = 27.961, df = 13, p < 0.01), whereas for AMP activity it did not matter (LME: X2 = 14.566, df = 13, p = 0.34).
Figure 2. Proportion of individuals showing constitutive expression of (A) internal and (B) external LLA and (C) internal and (D) external AMP activity for each species. Positive tests for parasitic species are shown in light gray and for eusocial species in dark gray. Bars give mean proportions with error bars representing one standard error.
Significantly fewer individuals expressed external (40 ± 24%) than internal LLA (67 ± 8%, mean ± sd) (LME: X2 = 63.545, df = 1, p < 0.001) (Figure 1A), whereas significantly more individuals expressed external (65 ± 12%) than internal AMP activity (39 ± 5%) (LME: X2 = 76.623, df = 1, p < 0.001) (Figure 1B). Further, the probability of showing internal or external LLA depended on the female group (LME: X2 = 31.340, df = 3, p < 0.001). Cuckoo and overwintered queens showed a bigger difference in the proportion of external vs. internal expression of LLA (25 ± 19 vs. 73 ± 18% and 19 ± 15 vs. 67 ± 28%, respectively) than young queens and workers (73 ± 20 vs. 57 ± 33% and 45 ± 19 vs. 73 ± 9%, respectively) (Figure 1A). Interestingly, only young queens showed a higher proportion of external than internal LLA (Figure 1A). The proportion of individuals expressing external or internal AMP activity did not depend on the female group (LME: X2 = 3.590, df = 3, p = 0.31). For AMP activity, young queens and workers showed a bigger difference between external and internal immune defense expression (82 ± 16 vs. 41 ± 24% and 67 ± 20 vs. 44 ± 12%, respectively) than cuckoo and overwintered queens (54 ± 34 vs. 31 ± 27% and 60 ± 32 vs. 39 ± 38%, respectively) (Figure 1B). Approximately 8% (26 out of 342) of the sampled bumblebees showed no measurable LLA and AMP activity in both internal and external immune defense.
Neither body size (LME: LLA: X2 = 2.254, df = 1, p = 0.13; AMP activity: X2 = 0.07, df = 1, p = 0.79) nor fat content relative to dry body weight (LME: LLA: X2 = 1.242, df = 1, p = 0.27; AMP activity: X2 = 1.527, df = 1, p = 0.22) affected the probability of constitutively expressing an immune defense.
Overall, the different types of queens and workers significantly affected LLA (LME: X2 = 14.160, df = 3, p < 0.01) (Figure 3A) and AMP activity (LME: X2 = 108.886, df = 3, p < 0.001) (Figure 3B). Young queens showed the highest LLA followed by workers overwintered queens and cuckoo queens (Tukey comparison: young vs. overwintered queens: p < 0.05 and young queens vs. workers: p < 0.01 (Figure 3B). All female types differed from each other in their expression of AMP activity (cuckoo vs. overwintered queens: Tukey comparison: p < 0.05, all other pairwise Tukey comparisons: p < 0.001).
Figure 3. Mean constitutive expression of (A) LLA as units of lysozyme per μl and (B) AMP activity as zone area in mm2 relative to body size (immune activity divided by radial cell length in mm) for both internal (hemolymph) and external (venom) immune defense for each female group. Internal immune defense is given as filled light gray circles and external immune defense as dark gray circles. Error bars represent one standard error and open light gray circles show immune activity for each species in a female group.
Overall, LLA showed a similar pattern across species (LME: X2 = 5.585, df = 13, p = 0.96) (Figure 4A), whereas AMP activity significantly varied between species (LME: X2 = 52.194, df = 13, p < 0.001) (Figure 4B and Table S5).
Figure 4. Mean constitutive expression of (A) LLA as units of lysozyme per μl and (B) AMP activity as zone area in mm2 relative to body size (immune activity divided by radial cell length in mm) for both internal (hemolymph) and external (venom) immune defense for each female group and species.
External LLA was significantly lower than internal LLA (LME: X2 = 16.470, df = 1, p < 0.001) (Figure 3A), whereas external AMP activity was significantly higher than internal AMP activity (LME: X2 = 344.375, df = 1, p < 0.001) (Figure 3B). Further, the strength of external and internal AMP activity depended on the female group (LME: X2 = 8.409, df = 3, p < 0.05).
Young queens expressed roughly 10 times higher and workers eight times higher external than internal AMP activity, whereas cuckoo and overwintered queens showed only five and four times higher external than internal AMP activity, respectively (Figure 3B). Young queens showed the highest external LLA (194.14 ± 93.60 mm2 relative body size) followed by workers (130.21 ± 71.30 mm2 relative body size), overwintered queens (30.73 ± 22.20 mm2 relative body size) with cuckoo queens having the lowest external LLA (17.50 ± 12.60 mm2 relative body size) (Figure 3A). However, internal LLA followed a similar pattern, indicating that the difference between external and internal LLA is not related to female group (LME: X2 = 1.536, df = 3, p = 0.67) (Figure 3A).
Neither body size (LME: LLA: X2 = 1.028, df = 1, p = 0.31; AMP activity: X2 = 0.196, df = 1, p = 0.66) nor fat content relative to dry body weight (LME: LLA: X2 = 0.001, df = 1, p = 0.98; AMP activity: X2 = 1.731, df = 1, p = 0.19) (Figure S9) affected the expression of an immune defense.
Mean colony size was neither correlated with LLA (Pearson's product-moment correlation: external: R2 = −0.33, z = −1.660, p = 0.11; internal: R2 = −0.10, z = −0.51, p = 0.62) nor AMP activity (Pearson's product-moment correlation: external: R2 = −0.08, z = −0.39, p = 0.70; internal: R2 = −0.22, z = −1.086, p = 0.29) (Figure S8).
Internal immune defense is given as filled light gray circles and external immune defense as dark gray circles. Error bars represent one standard error and open light gray circles show individual immune trait expression.
Overall, we found no association between external and internal immune defenses neither for LLA (PGLS: estimate = 0.709, SE = 0.834, R2 = 0.06, t = 0.850, p = 0.41) (Figure S9A) nor for AMP activity (PGLS: estimate = 0.048, SE = 0.031, R2 = 0.16, t = 1.522, p = 0.41) (Table S3, Figure S9B). There was also no link between external AMP activity and internal LLA (PGLS: estimate = 0.002, SE = 0.002, R2 = 0.09, t = 1.091, p = 0.30). Finally, none of the four immune defense measures was related to fat content relative to body weight (PGLS: external LLA: estimate = −0.001, SE = 0.001, R2 = 0.32, t = −1.813, p = 0.11; internal LLA: estimate = 0.001, SE = 0.001, R2 = 0.03, t = 0.494, p = 0.64; external AMP: estimate = −0.027, SE = 0.079, R2 = 0.02, t = −0.345, p = 0.74; internal AMP: estimate = −0.010, SE = 0.012, R2 = 0.09, t = −0.819, p = 0.44) (Table S3, Figure S9).
We could show that the constitutive expression of LLA was more prominent internally than externally and constitutive AMP activity was the main component of a potential external immune defense. The presence of an external immune defense and its degree of expression was highest in young queens and workers, indicating differences in the investment in internal and external immunity among the tested female groups. Also, the difference between internal and external immunity was largest in young queens and workers, indicating a greater importance of external immune defenses in those female groups. While species showed very similar patterns of external and internal LLA and internal AMP activity, external AMP activity differed between species. The drivers of such variation remain unknown, but we speculate that this might be related to the ecology of the species, i.e., variation in pathogen prevalence due to different nest sites (Fussell and Corbet, 1992) or pathogen prevalence in combination with population density and genetics (Whitehorn et al., 2011). We could not find any relationship between internal and external immune defense, which might be due to the fact that we measured constitutive expression of immune defense. Further, fat content relative to dry weight seems not to be related to the constitutive expression of immune defenses and neither does body size. We think that an investigation of induced internal immune defenses might add more information on the connection between external and internal immune defenses, and also the importance of fat reserves.
Cuckoo queens and overwintered queens less often showed constitutive expression of external immunity and its activity was also rather low in comparison to young queens and workers. These findings might be explained by the fact that cuckoo and overwintered queens have spent considerable resources to survive the winter and then in spring need to invest into reproduction, which is traded-off against immune defense (Sheldon and Verhulst, 1996; Råberg et al., 1998; Schwenke et al., 2016). Bumblebee queens lose over 25% of their body mass over hibernation (Brown et al., 2003). In comparison to overwintered queens, young queens and workers might have more resources to spend on external immune defenses as they do either not immediately reproduce or do not reproduce at all. In addition, it may be more important for individual workers to be able to defend the colony against enemies and pathogens than just itself. The reduction in immunity of overwintered queens might partly represent an age effect. Immunity decreases with age in several insect species, including bumblebees (Doums et al., 2002; Whitehorn et al., 2011), honeybees (Laughton et al., 2011), stingless bees (Ravaiano et al., 2018), mosquitoes (Hillyer et al., 2005), and crickets (Adamo et al., 2001). Probably also related to age, in the honeybee Apis mellifera, the composition of venom between queens and workers differs (Baracchi and Turillazzi, 2010). Baracchi and Turillazzi (2010) also found differences in the peptide composition of honeybee venom, depending on the task of an individual in the colony, i.e., queens, nursing, and foraging workers. For example, the venom of honeybee queens contains a smaller number of different AMPs than the venom of workers (Baracchi and Turillazzi, 2010). Whether such differences in the composition of venom might affect the variation in external immune defense across the female groups of the different bumblebee species would need to be investigated further.
Young queens had an almost 10 times higher external AMP activity than overwintered queens. In contrast, honeybees reduce immune gene expression over winter (Steinmann et al., 2015). However, honeybees overwinter in colonies and do not hibernate like bumblebees. Probably in preparation for hibernation young queens invest in external immunity similar to the paper wasp Polistes dominulus where females spread their venom within hibernation sites to protect themselves from bacteria during hibernation (Turillazzi et al., 2006). An increased antibacterial activity of the venom might be useful for a better protection. However, we are not aware of any accounts of bumblebee queens using their venom in such a way.
Workers showed stronger external immune defense than overwintered queens. In addition to the effects mentioned above reducing the immunity of an overwintered queen, it could well be that workers increase external immunity to protect the brood and the nest site and to be prepared for the encounter with pathogens when foraging. Higher workload in workers, however, leads to a reduced encapsulation response (König and Schmid-Hempel, 1995; Doums and Schmid-Hempel, 2000).
Interestingly, cuckoo queens had the lowest external as well as the internal immune defense of all female groups. Cuckoo queens might not have to invest in immunity as much as overwintered queens, who might need to keep their brood protected from microbes and pathogens during colony foundation. The basis for a potentially lower immune investment in the parasitic compared to the eusocial life-history strategy might be investigated further by monitoring the immunity of cuckoo and overwintered queens over time. We expect the investment in the external immune defense of cuckoo queens not to change once a nest has been taken over. Mainly, because the usurped nest should already contain workers that most likely already protect the nest site. However, overwintered queens have to establish a new colony. Similar to ant queens, we expect bumblebee queens to upregulate external immune defenses once a nest site has been identified to provide protection for the first brood (Tragust et al., under review).
Species differed in the presence of potential external immunity but varied mainly in the expression of external AMP activity. The pattern for external and internal LLA expression was similar across species and for the differences between female groups within species. For internal LLA, we expected this because LLA is part of the constitutive immune defense and has a rather broad range. The extent of LLA was comparable to LLA in hemolymph of honeybees, the only other hymenopteran where LLA has been measured so far (Dickel et al., 2018).
External AMP activity expression varied across species. We found most differences in external AMP activity among the eusocial species and between eusocial and parasitic species. Cuckoo species showed almost the same extent of external and internal immune defense. Therefore, the investment in external immune defenses seems more based on life-history strategies and characteristics than on species. Even though species differed overall in their AMP activity, the pattern between the female groups was relatively similar in each species. Young queens and workers had a higher potential external immune defense than cuckoo and overwintered queens. Only in one of the nine eusocial species, i.e., B. lapidarius, all female groups had similar external AMP activity. We take this as an indication that life-history strategy and characteristics have a greater impact on the expression of external immunity than ecological or genetic differences between species.
Neither fat reserves nor body size were correlated to immunity or had any effect on it. The differences between female groups or species do not seem to be explained by these two covariates. Similarly, body size had no effect on encapsulation response in B. terrestris (Schmid-Hempel and Schmid-Hempel, 1998) and melanization through the phenoloxidase pathway in the dung fly Scathophaga stercoraria (Hosken, 2001). One interpretation of this finding would be that organisms can compensate for the extra demand by increasing the intake of resources (Schmid-Hempel, 2003). Higher fat reserves were not associated with higher internal and potential external immune defense across the different life-history strategies and characteristics. However, all species showed a similar proportion of fat content, even across life-history strategies and characteristics. Although somehow surprising this finding might suggest that the costs of constitutively expressed immunity are maintenance costs. Such costs would only be visible if variation in the selection for the maintenance of an immune defense varied between species. Therefore, further studies should experimentally test the condition-dependence of external immunity by manipulating diet and/or by immune challenge similar to Moret and Schmid-Hempel (2000).
Even though cuckoo species showed lower immunity than eusocial species, colony size across eusocial species was neither correlated with internal nor with potential external immune defenses. Baracchi et al. (2012) suggested a threshold for a degree of sociality or a sufficient number of individuals in a society to be reached before an efficient collective immunity serves as a mechanism of disease resistance. Bumblebee societies might have already reached such a threshold. It would be interesting to study the colony size continuum in connection to immunity across a larger range of taxa, including ants, wasps, bees, and probably termites.
In conclusion, internal immunity was similar across life-history strategies and characteristics and species while potential external immune defense varied across the life-history strategies, characteristics and species. In general, the venom could serve as a potential external immune defense, but whether or not bumblebees use it, will have to be investigated further. The detection of antimicrobial components in the nest material or hibernation sites, on eggs or brood, or the body surface of bumblebees, as for other insects (e.g., ants, bees, and wasps), could provide evidence for the use of venom as external immune defense. Venom might not be the only component of external immune defense in bumblebees. Therefore, future work will need to incorporate other exocrine glands, such as the mandibular, salivary or Dufour's gland (Ayasse and Jarau, 2014) as these could also contain antimicrobial properties. We found no general link between individual condition and immune defenses. Further studies might find such links by altering either immune defense traits or resource availability.
The datasets generated for this study are available on request to the corresponding author.
GB, HF, and OO conceived the idea and designed the study. GB carried out the study. GB and OO performed the statistical analysis. GB, HF, and OO interpreted the results and wrote the manuscript. All authors read and approved of the final manuscript.
The authors declare that the research was conducted in the absence of any commercial or financial relationships that could be construed as a potential conflict of interest.
We thank Simon Tragust for sharing his knowledge about antimicrobial secretions in ants and helpful statistics advice. Bastian Schauer helped with establishing immunoassay in the lab and we were grateful to Lisa Heuss for comments on earlier drafts of the manuscript and Sara Bellinvia for her ggplot2 skills and Sebastian Steibl for his advice on PCA. The collection of samples was conducted with the permission of the government of Upper Franconia (Obere Naturschutzbehörde Oberfranken, Permit reference number 55.1-8646-1-7-24). This publication was funded by the German Research Foundation (DFG) and the University of Bayreuth in the funding programme Open Access Publishing.
The Supplementary Material for this article can be found online at: https://www.frontiersin.org/articles/10.3389/fevo.2020.00062/full#supplementary-material
Adamo, S. A. (2009). “The impact of physiological state on immune function in insects,” in Insect Infection and Immunity, eds J. Rolff and S. E. Reynolds (Oxford: Oxford University Press), 173–186. doi: 10.1242/jeb.092049
Adamo, S. A., Jensen, M., and Younger, M. (2001). Changes in lifetime immunocompetence in male and female Gryllus texensis (formerly G. integer)*: trade-offs between immunity and reproduction. Anim. Behav. 62, 417–425. doi: 10.1006/anbe.2001.1786
Ashida, M., and Brey, P. T. (1995). Role of the integument in insect defense: pro-phenol oxidase cascade in the cuticular matrix. Proc. Natl. Acad. Sci. U.S.A. 92, 10698–10702. doi: 10.1073/pnas.92.23.10698
Ayasse, M., and Jarau, S. (2014). Chemical ecology of bumble bees. Annu. Rev. Entomol. 59, 299–319. doi: 10.1146/annurev-ento-011613-161949
Baer, B., and Schmid-Hempel, P. (1999). Experimental variation in polyandry affects parasite loads and fitness in a bumble-bee. Nature 397, 151–153. doi: 10.1038/16451
Baracchi, D., Mazza, G., and Turillazzi, S. (2012). From individual to collective immunity: the role of the venom as antimicrobial agent in the Stenogastinae wasp societies. J. Insect Physiol. 58, 188–93. doi: 10.1016/j.jinsphys.2011.11.007
Baracchi, D., and Turillazzi, S. (2010). Differences in venom and cuticular peptides in individuals of Apis mellifera (Hymenoptera: Apidae) determined by MALDI-TOF MS. J. Insect Physiol. 56, 366–375. doi: 10.1016/j.jinsphys.2009.11.013
Bazazi, S., Arganda, S., Moreau, M., Jeanson, R., and Dussutour, A. (2016). Responses to nutritional challenges in ant colonies. Anim. Behav. 111, 235–249. doi: 10.1016/j.anbehav.2015.10.021
Boots, M., and Best, A. (2018). The evolution of constitutive and induced defences to infectious disease. Proc. R. Soc. Lond. B 285:20180658. doi: 10.1098/rspb.2018.0658
Bretz, F., Hothorn, T., Westfal, P., and Westfall, P. H. (2010). Multiple Comparisons Using R. London: Chapman & Hall/CRC.
Broderick, N. A., Welchman, D. P., and Lemaitre, B. (2009). “Recognition and response to microbial infection in Drosophila,” in Insect Infection and Immunity, eds J. Rolff, and S. E. Reynolds (Oxford: Oxford University Press), 13–33. doi: 10.1093/acprof:oso/9780199551354.003.0002
Brooks, M. E., Kristensen, K., van Benthem, K. J., Magnusson, A., Berg, C. W., Nielsen, A., et al. (2017). glmmTMB balances speed and flexibility among packages for zero-inflated generalized linear mixed modeling. R J. 9, 378–400. doi: 10.32614/RJ-2017-066
Brown, M. J. F., Schmid-Hempel, R., and Schmid-Hempel, P. (2003). Strong context-dependent virulence in a host–parasite system: reconciling genetic evidence with theory. J. Anim. Ecol. 72, 994–1002. doi: 10.1046/j.1365-2656.2003.00770.x
Bulet, P., Hetru, C., Dimarcq, J.-L., and Hoffmann, D. (1999). Antimicrobial peptides in insects; structure and function. Dev. Comp. Immunol. 23, 329–344. doi: 10.1016/s0145-305x(99)00015-4
Bulmer, M. S., Bachelet, I., Raman, R., Rosengaus, R. B., and Sasisekharan, R. (2009). Targeting an antimicrobial effector function in insect immunity as a pest control strategy. Proc. Natl. Acad. Sci. U.S.A. 106, 12652–12657. doi: 10.1073/pnas.0904063106
Cotter, S. C., Kruuk, L. E. B., and Wilson, K. (2004). Costs of resistance: genetic correlations and potential trade-offs in an insect immune system. J. Evol. Biol. 17, 421–429. doi: 10.1046/j.1420-9101.2003.00655.x
Cotter, S. C., Reavey, C. E., Tummala, Y., Randall, J. L., Holdbrook, R., Ponton, F., et al. (2019). Diet modulates the relationship between immune gene expression and functional immune responses. Insect Biochem. Mol. Biol. 109, 128–141. doi: 10.1016/j.ibmb.2019.04.009
Cremer, S., Armitage, S. A. O., and Schmid-Hempel, P. (2007). Social Immunity. Curr. Biol. 17, 693–702. doi: 10.1016/j.cub.2007.06.008
Cremer, S., Pull, C. D., and Fürst, M. A. (2018). Social immunity: emergence and evolution of colony-level disease protection. Ann. Rev. Entomol. 63, 105–123. doi: 10.1146/annurev-ento-020117-043110
Dickel, F., Münch, D., Amdam, G. V., Mappes, J., and Freitak, D. (2018). Increased survival of honeybees in the laboratory after simultaneous exposure to low doses of pesticides and bacteria. PLoS ONE 13:e0191256. doi: 10.1371/journal.pone.0191256
Dolezal, T., Krejcova, G., Bajgar, A., Nedbalova, P., and Strasser, P. (2019). Molecular regulations of metabolism during immune response in insects. Insect Biochem. Mol. Biol. 109, 31–42. doi: 10.1016/j.ibmb.2019.04.005
Doums, C., Moret, Y., Benelli, E., and Schmid-Hempel, P. (2002). Senescence of immune defence in Bombus workers. Ecol. Entomol. 27, 138–144. doi: 10.1046/j.1365-2311.2002.00388.x
Doums, C., and Schmid-Hempel, P. (2000). Immunocompetence in workers of a social insect, Bombus terrestris, in relation to foraging activity and parasitic infection. Can. J. Zool. 78, 1060–1066. doi: 10.1139/z00-035
Felsenstein, J. (2005). PHYLIP (Phylogeny Inference Package) Version 3.6. Seattle, WA: Department of Genome Sciences, University of Washington.
Ferrandon, D., Imler, J. L., Hetru, C., and Hoffmann, J. A. (2007). The Drosophila systemic immune response: sensing and signalling during bacterial and fungal infections. ?Nat. Rev. Immunol. 7, 862–874. doi: 10.1038/nri2194
Fisher, R. M. (1988). Observations on the behaviours of three European cuckoo bumble bee species (Psithyrus). Ins. Soc. 35:341. doi: 10.1007/BF02225810
Fisher, R. M., and Sampson, B. J. (1992). Morphological specializations of the bumble bee social parasite, Psithyrus ashtoni Cresson (Hymenoptera: Apidae). Can. Entomol. 124, 69–77. doi: 10.4039/Ent12469-1
Fussell, M., and Corbet, S. A. (1992). The nesting places of some British bumble bees. J. Apic. Res. 31, 32–41. doi: 10.1080/00218839.1992.11101258
Gokcezade, J. F., Gereben-Krenn, B.-A., Neumayer, J., and Krenn, H. W. (2010). Feldbestimmungsschlüssel für die Hummeln Österreichs, Deutschlands und der Schweiz. Linzer Biol. Beitr. 42, 5–42. Available online at: https://www.zobodat.at/pdf/LBB_0047_1_0005-0042.pdf
Goulson, D., Lye, G. C., and Darvill, B. (2008). Decline and conservation of bumble bees. Ann. Rev. Entomol. 53, 191–208. doi: 10.1146/annurev.ento.53.103106.093454
Haine, E. R., Pllitt, L., Moret, Y., Siva-Jothy, M. T., and Rolff, J. (2008). Temporal patterns in immune responses to a range of microbial insults (Tenebrio molitor). J. Insect Physiol. 54, 1090–1097. doi: 10.1016/j.jinsphys.2008.04.013
Hamilton, C., Lay, F., and Blumer, M. S. (2011). Subterranean termite prophylactic secretions and external antifungal defences. J. Insect Physiol. 57, 1259–1266. doi: 10.1016/j.jinsphys.2011.05.016
Hartig, F. (2019). DHARMa: Residual Diagnostics for Hierarchical (Multi-Level/Mixed) Regression Models. R package version 0.2.4. Available online at: https://CRAN.R-project.org/package=DHARMa
Hillyer, J. F., Schmidt, S. L., Fuchs, J. F., Boyle, J. P., and Christensen, B. M. (2005). Age-associated mortality in immune challenged mosquitoes (Aedes aegypti) correlates with a decrease in haemocyte numbers. Cell. Microbiol. 7, 39–51. doi: 10.1111/j.1462-5822.2004.00430.x
Hochberg, M. E. (1991). Nonlinear transmission rates and the dynamics of infectious diseases. J. Theor. Biol 153, 301–321. doi: 10.1016/S0022-5193(05)80572-7
Hosken, J. D. (2001). Sex and death: microevolutionary trade-offs between reproductive and immune investment in dung flies. Curr. Biol. 11, R379–R380. doi: 10.1016/s0960-9822(01)00211-1
Hothorn, T., Bretz, F., and Westfall, P. (2008). Simultaneous inference in general parametric models. Biom. J. 50, 346–363. doi: 10.1002/bimj.200810425
König, C., and Schmid-Hempel, P. (1995). Foraging activity and immunocompetence in workers of the bumble bee, Bombus terrestris L. Proc. R. Soc. Lond. B 260, 225–227. doi: 10.1098/rspb.1995.0084
Kuhn-Nentwig, L. (2003). Antimicrobial and cytolytic peptides of venomous arthropods. Cell. Mol. Life Sci. 60, 2651–2668. doi: 10.1007/s00018-003-3106-8
Laughton, A. M., Boots, M., and Siva-Jothy, M. T. (2011). The ontogeny of immunity in the honey bee, Apis mellifera L. following an immune challenge. J. Insect Physiol. 57, 1023–1032. doi: 10.1016/j.jinsphys.2011.04.020
Lemaitre, B., and Hoffmann, J. (2007). The host defense of Drosophila melanogaster. Ann. Rev. Immunol. 25, 697–743. doi: 10.1146/annurev.immunol.25.022106.141615
Lung, O., Kuo, L., and Wolfner, M. F. (2001). Drosophila males transfer antibacterial proteins from their accessory gland and ejaculatory duct to their mates. J. Insect Physiol. 47, 617–622. doi: 10.1016/s0022-1910(00)00151-7
Medler, J. T. (1962). Morphometric studies on bumble bees. Ann. Entomol. Soc. Am. 55, 212–218. doi: 10.1093/aesa/55.2.212
Moret, Y. (2003). Explaining variable costs of the immune response: selection for specific versus non-specific immunity and facultative life history change. Oikos 102, 213–216. doi: 10.1034/j.1600-0706.2003.12496.x
Moret, Y., and Schmid-Hempel, P. (2000). Survival for immunity: the price of immune system activation for bumblebee workers. Science 290, 1166–1167. doi: 10.1126/science.290.5494.1166
Naug, D., and Camazine, S. (2002). The role of colony organization on pathogen transmission in social insects. J. Theor. Biol. 215, 427–439. doi: 10.1006/jtbi.2001.2524
Nayduch, D., and Joyner, C. (2013). Expression of lysozyme in the life history of the house fly (Musca domestica L.). J. Med. Entomol. 50, 847–852. doi: 10.1603/me12167
O'Donnell, S., Reichardt, M., and Foster, R. (2000). Individual and colony factors in bumble bee division of labor (Bombus bifarius nearcticus Handl; Hymenoptera, Apidae). Insect Soc. 47, 164–170. doi: 10.1007/PL00001696
Orme, R., Freckleton, R., Thomas, G., Petzoldt, T., Fritz, S., Isaac, N., et al. (2018). Caper: Comparative Analyses of Phylogenetics and Evolution in R. R package version 1.0.1. Available online at: https://CRAN.R-project.org/package=caper
Oster, G. F., and Wilson, E. O. (1978). Caste and Ecology in the Social Insects. Princeton, NJ: Princeton University Press.
Otti, O., Naylor, R. A., Siva-Jothy, M. T., and Reinhardt, K. (2009). Bacteriolytic activity in the ejaculate of an insect. Am. Nat. 174, 292–295. doi: 10.1086/600099
Otti, O., and Schmid-Hempel, P. (2008). A field experiment on the effect of Nosema bombi in colonies of the bumblebee Bombus terrestris. Ecolo. Entomol. 33, 577–582. doi: 10.1111/j.1365-2311.2008.00998.x
Otti, O., Tragust, S., and Feldhaar, H. (2014). Unifying external and internal immune defences. Trends Ecol. Evol. 29, 625–634. doi: 10.1016/j.tree.2014.09.002
Owen, R. E. (1988). Body size variation and optimal body size of bumble bee queens (Hymenoptera: Apidae). Can. Entomol. 120, 19–27. doi: 10.4039/Ent12019-1
Owen, R. E. (1989). Differential size variation of male and female bumblebees. J. Heredity 80, 39–43. doi: 10.1093/oxfordjournals.jhered.a110786
Pinheiro, J., Bates, D., DebRoy, S., Sarkar, D., and R Core Team. (2019). nlme: Linear and Nonlinear Mixed Effects Models. R package version 3.1-139. Available online at: https://CRAN.R-project.org/package=nlme
Popp, M., Erler, S., and Lattorff, H. M. G. (2012). Seasonal variability of prevalence and occurrence of multiple infections shape the population structure of Crithidia bombi, an intestinal parasite of bumblebees (Bombus spp.). Microbiol. Open 1, 362–372. doi: 10.1002/mbo3.35
R Core Team (2019). R: A Language and Environment for Statistical Computing. Vienna: R Foundation for Statistical Computing. Available online at: https://www.R-project.org/
Råberg, L., Grahn, M., Hasselquist, D., and Svensson, E. (1998). On the adaptive significance of stress-induced immunosuppression. Proc. R. Soc. Lond. 265, 1637–1641. doi: 10.1098/rspb.1998.0482
Ravaiano, S. V., Barbosa, W. F., Campos, L. A., and Martins, G. F. (2018). Variations in circulating hemocytes are affected by age and caste in the stingless bee Melipona quadrifasciata. Sci. Nat. 105:48. doi: 10.1007/s00114-018-1573-x
Reeson, A. F., Wilson, K., Gunn, A., Hails, R. S., and Goulson, D. (1998). Baculovirus resistance in the noctuid Spodoptera exempta is phenotypically plastic and responds to population density. Proc. R. Soc. Lond. 265, 1787–1791. doi: 10.1098/rspb.1998.0503
Revell, L. J., and Chamberlain, S. A. (2014), Rphylip: an R interface for PHYLIP. Methods Ecol Evol. 5, 976–981. doi: 10.1111/2041-210X.12233
Richards, O. W. (1927). The specific characters of the British humblebees (Hymenoptera). Ecol. Entomol. 75, 233–268. doi: 10.1111/j.1365-2311.1927.tb00073.x
Richter, J., Helbing, S., Erler, S., and Lattorff, H. M. G. (2012). Social context-dependent immune gene expression in bumblebees (Bombus terrestris). Behav. Ecol. Sociobiol. 66, 791–796. doi: 10.1007/s00265-012-1327-2
Samakovlis, C., Kylsten, P., Kimbrell, D., Engström, A., and Hultmark, D. (1991). The Andropin gene and its product, a male-specific antibacterial peptide in Drosophila melanogaster. EMBO J. 10, 163–169. doi: 10.1002/j.1460-2075.1991.tb07932.x
Schmid-Hempel, P. (2003). Variation in immune defence as a question of evolutionary ecology. Proc. R. Soc. Lond. B 270, 357–366. doi: 10.1098/rspb.2002.2265
Schmid-Hempel, P. (2005). Evolutionary ecology of insect immune defence. Annu. Rev. Entomol. 50, 529–551. doi: 10.1146/annurev.ento.50.071803.130420
Schmid-Hempel, P. (2011). Evolutionary Parasitology: The Integrated Study of Infections, Immunology, Ecology, and Genetics. Oxford: Oxford University Press.
Schmid-Hempel, R., and Schmid-Hempel, P. (1998). Colony performance and immunocompetence of a social insect, Bombus terrestris, in poor and variable environments. Funct. Ecol. 12, 22–30. doi: 10.1046/j.1365-2435.1998.00153.x
Schneider, C. A., Rasband, W. S., and Eliceiri, K. W. (2012). NIH image to imageJ: 25 years of image analysis. Nat. Methods 9, 671–675. doi: 10.1038/nmeth.2089
Schwenke, R. A., Lazzaro, B. P., and Wolfner, M. F. (2016). Reproduction–immunity trade-offs in insects. Annu. Rev. Entomol. 61, 239–256. doi: 10.1146/annurev-ento-010715-023924
Sheldon, B. C., and Verhulst, S. (1996). Ecological immunology: costly parasite defences and trade-offs in evolutionary ecology. Trends Ecol. Evol. 11, 317–321. doi: 10.1016/0169-5347(96)10039-2
Siva-Jothy, M. T., Moret, Y., and Rolff, J. (2005). Insect immunity: an evolutionary ecology perspective. Adv. Insect Physiol. 32, 1–48. doi: 10.1016/S0065-2806(05)32001-7
Steinmann, N., Corona, M., Neumann, P., and Dainat, B. (2015). Overwintering is associated with reduced expression of immune genes and higher susceptibility to virus infection in honey bees. PLoS ONE 10:e0129956. doi: 10.1371/journal.pone.0129956
Stow, A., Briscoe, D., Gillings, M., Holley, M., Smith, S., Leys, R., et al. (2007). Antimicrobial defences increase with sociality in bees. Biol. Lett. 3, 422–424. doi: 10.1098/rsbl.2007.0178
Tragust, S. (2016). External immune defence in ant societies (Hymenoptera: Formicidae): the role of antimicrobial venom and metapleural gland secretion. Myrmecol. News 23, 119–128. doi: 10.25849/myrmecol.news_023:119
Tragust, S., Mitteregger, B., Barone, V., Konrad, M., Ugelvig, L. V., and Cremer, S. (2013). Ants disinfect fungus-exposed brood by oral uptake and spread of their poison. Curr. Biol. 23, 76–82. doi: 10.1016/j.cub.2012.11.034
Turillazzi, S., Mastrobouni, G., Dani, F. R., Moneti, G., Pieraccini, G., and La Marca, G. (2006). Dominulin A and B: two new antibacterial peptides identified on the cuticle and in the venom of the social paper wasp Polistes dominulus using MALDI-TOF, MALDI-TOF/TOF, and ESI-Ion Trap. J. Am. Soc. Mass Spectrom. 17, 376–383. doi: 10.1016/j.jasms.2005.11.017
Turnbull, C., Hoggard, S., Gillings, M., Palmer, C., Stow, A., Beattie, D., et al. (2011). Antimicrobial strength increases with group size: implications for social evolution. Biol. Lett. 7, 249–252. doi: 10.1098/rsbl.2010.0719
Velthuis, H. H. W., and van Doorn, A. (2006). A century of advances in bumblebee domestication and the economics and environmental aspects off its commercialization for pollination. Apidologie 37, 421–451. doi: 10.1051/apido:2006019
Westra, E. R., van Houte, S., Oyesiku-Blakemore, S., Makin, B., Broniewski, J. M., Best, A., et al. (2015). Parasite exposure drives selective evolution of constitutive versus inducible defense. Curr. Biol. 25, 1043–1049. doi: 10.1016/j.cub.2015.01.065
Whitehorn, P. R., Tinsley, M. C., Brown, M. J. F., Darvill, B., and Goulson, D. (2011). Genetic diversity, parasite prevalence and immunity in wild bumblebees. Proc. R. Soc. Lond. B 278, 1195–1202. doi: 10.1098/rspb.2010.1550
Wilson, K., Thomas, B. M., Blanford, S., Doggett, M., Simpson, S. J., and Moore, S. L. (2002). Coping with crowds: density-dependent disease resistance in desert locusts. Proc. Natl. Acad. Sci. U.S.A. 99, 5471–5475. doi: 10.1073/pnas.082461999
Keywords: immune defense strategies, individual condition, immune traits, antibacterial secretions, density dependence, eusocial insects
Citation: Baeuerle G, Feldhaar H and Otti O (2020) Comparing a Potential External Immune Defense Trait to Internal Immunity in Females of Wild Bumblebees. Front. Ecol. Evol. 8:62. doi: 10.3389/fevo.2020.00062
Received: 01 September 2019; Accepted: 26 February 2020;
Published: 17 March 2020.
Edited by:
Mark A. Elgar, The University of Melbourne, AustraliaReviewed by:
Silvio Erler, Julius Kühn-Institut-Braunschweig, GermanyCopyright © 2020 Baeuerle, Feldhaar and Otti. This is an open-access article distributed under the terms of the Creative Commons Attribution License (CC BY). The use, distribution or reproduction in other forums is permitted, provided the original author(s) and the copyright owner(s) are credited and that the original publication in this journal is cited, in accordance with accepted academic practice. No use, distribution or reproduction is permitted which does not comply with these terms.
*Correspondence: Oliver Otti, b2xpdmVyLm90dGlAdW5pLWJheXJldXRoLmRl
Disclaimer: All claims expressed in this article are solely those of the authors and do not necessarily represent those of their affiliated organizations, or those of the publisher, the editors and the reviewers. Any product that may be evaluated in this article or claim that may be made by its manufacturer is not guaranteed or endorsed by the publisher.
Research integrity at Frontiers
Learn more about the work of our research integrity team to safeguard the quality of each article we publish.