- 1Department of Biology, Institute of Zoology, Functional Ecology, University Hamburg, Hamburg, Germany
- 2School of Biological and Environmental Sciences, Liverpool John Moores University, Liverpool, United Kingdom
- 3School of Biology and Ecology, University of Maine, Orono, ME, United States
Mammalian heterotherms, species that employ short or long periods of torpor, are found in many different climatic regions. Although the underlying physiological mechanisms of heterothermy in species from lower latitudes (i.e., the tropics and southern hemisphere) appear analogous to those of temperate and arctic heterotherms, the ultimate triggers and resulting patterns of energy expenditure and body temperature are often noticeably different. Phenotypic flexibility in the patterns of thermoregulation in non-Holarctic species can be extensive (depending on body condition, environmental parameters and species competition) and the factors responsible for inducing heterothermy are more variable in non-Holarctic species. As well as being a regular adaptation to seasonality, heterothermy can also be employed as a response to unpredictability in environmental parameters and as a response to emergency situations. Non-Holarctic heterotherms also challenge the notion that regular inter-bout arousals during hibernation are obligatory and suggest all that is necessary to maintain proper functioning during hibernation is an occasional passive return to—or maintenance of—a relatively high body temperature. The study of non-Holarctic heterotherms has led to the conclusion that heterothermy must be defined on the basis of mechanistic, physiological parameters, and not solely by body temperature; yet we are still limited in our abilities to record such mechanistic parameters in the field. It is now believed that homeothermy in mammals evolved in hot climates via an ancestral heterothermic state. Similar to extant warm-climate heterotherms, early mammals could have relied mainly on passive body temperature regulation with a capacity for short- to longer-term up-regulation of metabolism when needed. Hibernation, as seen in temperate and arctic species may then be a derived state of this ancestral heterothermy, and the study of torpor in warm climates can provide potential models for the energetics of early mammals.
Introduction
Torpor in heterothermic endotherms is a controlled, reversible depression of metabolic rate, and active thermoregulation, well below the usual daily cycle (sensu Geiser and Ruf, 1995). Over the last two to three decades, it has become apparent that torpid states in endotherms are employed in a wide range of ecological and physiological settings and under contrasting conditions (Cossins and Barnes, 1996; Geiser and Brigham, 2012; Boyles et al., 2013; Levesque et al., 2016; Nowack et al., 2017). This challenges the “traditional” view of torpor as essentially an adaptation to mismatches between energy supply and demand during cold seasonal northern hemisphere winters (Geiser, 2004b; Heldmaier et al., 2004; McKechnie and Mzilikazi, 2011). Understanding the different underlying causes and initiators of heterothermy is essential if we are to understand how homeothermy evolved (Grigg and Beard, 2000; Lovegrove, 2012). Torpor use in temperate and arctic species is traditionally differentiated into “daily torpor” and “long-term hibernation.” Daily torpor consists of hypometabolic phases of less than 24 h, with regular, euthermic behavior during the usual active phase, whereas hibernation consists of a series of hypometabolic phases (multiday torpor bouts) over several months that are interspersed with regular euthermic arousals. In “classic” hibernators periodic, or inter-bout, arousals occur about once every 1 or 2 weeks and are presumably needed to sustain mammalian life during long-term torpor. Importantly, however, arousals are energetically expensive phases of active rewarming during hibernation (Carey et al., 2003; Murray et al., 2003) and are associated with increased production of reactive oxygen species and cellular damage (Carey et al., 2000; Brown and Staples, 2011; Nowack et al., 2019). Energy savings during hibernation are more pronounced than during daily torpor, but in contrast to daily torpor, hibernation usually requires preparation (e.g., accumulation of fat stores, modifications of the reproductive and digestive system Barnes et al., 1986; Hume et al., 2002; Sheriff et al., 2013 (but see Liu and Karasov (2011) for an example of a subtropical bat species without pre-fattening) and reliably favorable conditions to allow for a quick recovery when animals terminate hibernation with a reduced body condition. Despite the differentiation between daily heterotherms and hibernators, the extent to which species use torpor can vary substantially as we will detail below.
A Global Perspective of Torpor in Mammals: A Continuum of Physiological Responses
Extant non-Holarctic heterotherms, often living in warm, tropical or subtropical climates, are also capable of conserving energy by reducing metabolism. Although temperate and arctic heterotherms usually employ torpor in a seasonal manner to escape unfavorable winter conditions, the responses reported to date for non-Holarctic heterotherms fall on a continuum (Boyles et al., 2013; Levesque et al., 2016). One extreme is represented by largely heterothermic species that have a highly labile body temperature (Tb; i.e., large daily fluctuations of Tb without actively depressed metabolic rate) and employ torpor (large daily amplitudes in Tb and depressed metabolic rate) at any time of the year, with longer bouts during the hibernation season (Grigg and Beard, 2000; Turbill et al., 2003; Lovegrove and Génin, 2008; Levesque and Lovegrove, 2014; Lovegrove et al., 2014a,b; Dausmann et al., submitted). At the opposite end of the continuum are species that are physiologically able to exhibit torpor but do so only rarely (and usually in form of short bouts of torpor) in emergencies when immediate survival is at risk (Nowack et al., 2010; Dausmann et al., 2012). An intermediate form between heterothermy on a very frequent basis throughout the year or only rare torpor use under extreme circumstances, would be the “classical,” seasonal use of torpor. In this case, Tb is kept rather constant outside of the hibernation season and animals show a regular adjustment of energy balance to seasonal unfavorable conditions (such as low temperature, high rainfall, low food availability, see Table 1).

Table 1. Non-Holarctic heterotherms, including species distribution, patterns of heterothermy and potential factors inducing torpor where known.
The number and phylogenetic diversity of non-Holarctic species with documented torpor use is steadily increasing, with the number doubling in some taxonomic orders since 2011 (Table 1, Figures 1A,B) (McKechnie and Mzilikazi, 2011). Also, thanks to technological advancements, more physiological data can be obtained in the field, enabling functional insights into heterothermic responses of free-ranging animals with their full physiological potential (Chmura et al., 2018). The purpose of our review is to summarize what is known about torpor use in mammals living outside the Holarctic, including what is traditionally termed sub-tropics and tropics, and the more temperate zones of the southern hemisphere (Figure 1A). Therefore, we have synthesized information about the occurrence, the length, minimum Tb and metabolic rate during torpor, as well as the ultimate triggers of torpor use (season, weather, etc.) in heterothermic species outside of the north temperate and arctic zones. We used data from the comprehensive review by Ruf and Geiser (2015) with the addition of more recent descriptions of torpor use in non-Holarctic heterotherms (Table 1, Figures 1A,B). We focus on the proximate factors influencing torpor use, as well as the physiological similarities and differences between mammals using torpor in diverse habitats. Following on previous syntheses (Grigg, 2004; Grigg et al., 2004; Lovegrove, 2012), we further discuss how knowledge of these proximate, and the ultimate causes of torpor use in extant tropical heterotherms can provide insight into the ancestral mammal condition.
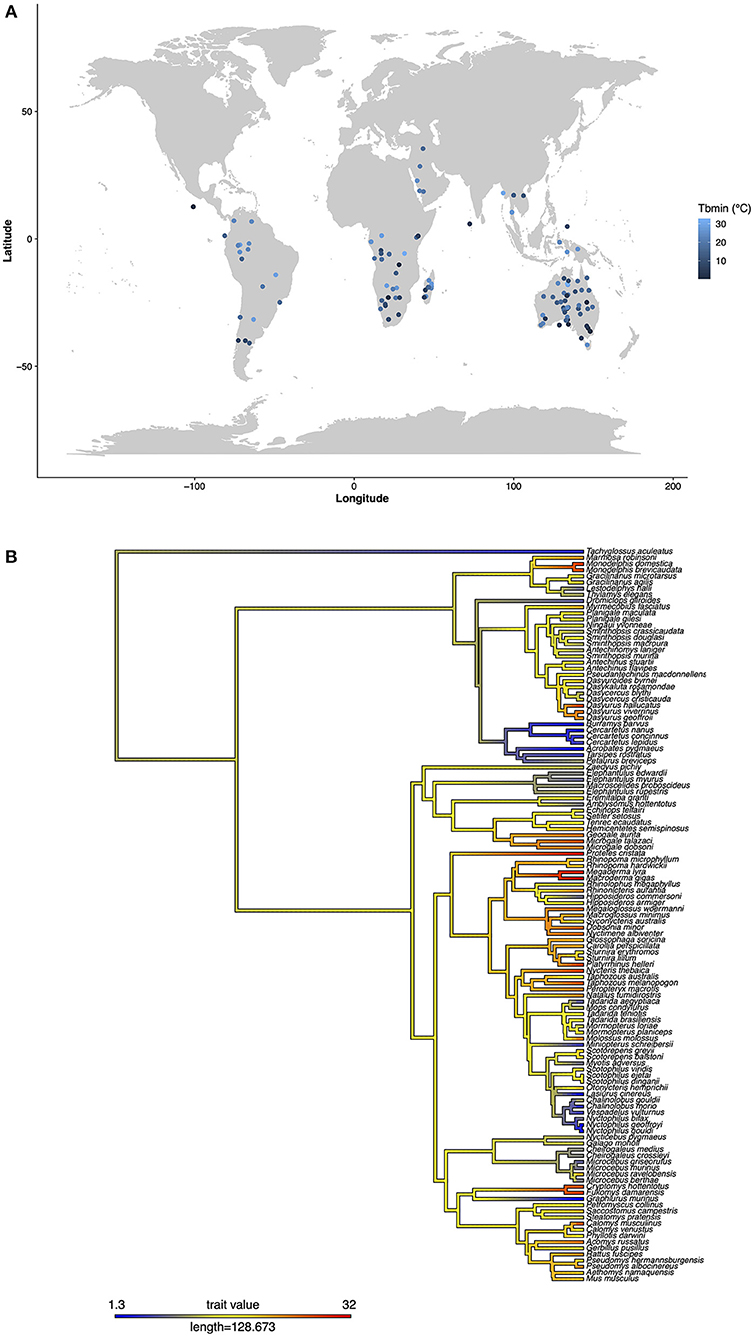
Figure 1. (A) The minimum torpid body temperature (Tbmin) and phylogenetic relationship of non-Holarctic heterotherms, and (B) their geographical distribution. The phylogeny, including estimated ancestral states for Tbmin was plotted using “contMap” in the R library “phytools” (Revell, 2012) and the latest mammalian phylogeny by Upham et al. (2019). Geographical locations were taken as the latitude and longitude at the middle of the species range provided by the PanTHERIA dataset (Jones et al., 2009).
Do Non-Holarctic Heterotherms Fall Into the Daily-Heterotherm—Hibernator Dichotomy?
Daily torpor and hibernation patterns in non-Holarctic species are similar to those observed in temperate/arctic animals, albeit with some distinctive differences. As far as it is known, the physiological basis seems analogous: metabolism (and other physiological variables, such as heart rate, respiration rate, etc.) is actively depressed to a fraction of euthermic levels, usually during the daily resting phase or at the end of the activity phase, and Tb adjusts accordingly (Carey et al., 2003; Geiser, 2004a; Heldmaier et al., 2004). Additionally to active depression of metabolic rate, the abandoning of the differential between Tb and ambient temperature contributes substantially to energy savings during torpor bouts, especially in small species. Usually this results in a drop in Tb, the degree of which depends on ambient temperature (and the degree of daily fluctuations thereof), the insulative capacity of the resting site (see below), and the duration of the torpor bout (Hallam and Mzilikazi, 2011; Kobbe et al., 2011).
Although we can still differentiate daily heterotherms and hibernators in the non-Holarctic dataset, the distinction becomes a bit more blurry. Notably, it is more common for non-Holarctic hibernators to also use short bouts of torpor that typically last less than 24 h but during which metabolic rate is lowered to levels comparable to those during hibernation phases, e.g., seen in Nyctophilus spp. (Geiser and Brigham, 2000; Geiser and Stawski, 2011). To differentiate these from daily torpor use by daily heterotherms, we will refer to those by using the term “short bouts”. Furthermore, many non-Holarctic hibernators do not only use short bouts or hibernation, but often also show an intermediate length of torpor, i.e., “prolonged torpor” lasting up to several days with regular activity (several hours to many days) between torpor bouts (Kobbe et al., 2011; Dausmann, 2014).
Heterothermy as the Norm, Homeothermy as the Exception
Although Holarctic heterotherms usually maintain a high and stable Tb during part of the year, examples from the southern hemisphere show us that this is not the case for all heterotherms. At the extreme end of thermolabililty in heterotherms are the eutherian Tenrecidae, a family of mammals found on Madagascar and the surrounding islands, which have been isolated from the mainstream of mammalian evolution for about 30–56 Myr (Crompton et al., 1978; Everson et al., 2016). They are members of the superorder Afrotheria, a group of mammals whose extant members live predominantly in Africa or are of African origin (Poux et al., 2005). The ecology, behavior and thermoregulatory physiology of these “basoendothermic” mammals have been proposed to be similar to those of the early mammalian endotherms (Lovegrove and Génin, 2008; Levesque and Lovegrove, 2014). All members of this group investigated to date exhibit a generally low basal metabolic rate and highly labile Tb, often closely tracking environmental temperature (Table 1). Even so, the lesser hedgehog tenrec, Echinops telfairi (and likely other species of tenrec as well), has functional brown adipose tissue, enabling non-shivering thermogenesis (Oelkrug et al., 2013). In addition to low basal metabolic rates, many species of tenrecs also enter short daily bouts of torpor and long-term hibernation (Table 1). Some species (E. telfairi, Setifer setosus) seem to maintain euthermy (higher and less variable Tb in the resting-phase) only during reproduction (gestation and/or lactation) (Poppitt et al., 1994; Wein, 2010; Levesque and Lovegrove, 2014; Lovegrove et al., 2014b) presumably to enhance embryonic development and milk production. For males, the occasional rise of Tb above 30°C might be sufficient to allow sperm production (Fietz et al., 2004).
Outside of the hibernation season (during the austral winter), torpor in E. telfairi and S. setosus seems to be independent of external stimuli and is employed frequently not in response to seasonality or a scarcity of food or water, but throughout the active season (see Figure 2; Lovegrove and Génin, 2008; Wein, 2010; Levesque et al., 2013, 2014; Dausmann et al., submitted). Interestingly, the thermolability shown by many tenrec species is mirrored by another basoendotherm (dubbed “protoendotherm” by Grigg et al., 2004), the short-beaked echidna, Tachyglossus aculeatus (Order Monotremata). Echidnas have a wide distribution in Australia and Tasmania, from cold-temperate to desert habitats, and also occur in tropical areas. They exhibit daily fluctuations in Tb (up to 5°C) and use torpor flexibly (Kuchel, 2003; Grigg et al., 2004; Nicol and Andersen, 2006). Depending on their habitat they increase torpor use during the cold period and either show long-term hibernation (e.g., Tasmania or Australian Alps: Augee and Ealy, 1968; Grigg et al., 1989; Nicol and Andersen, 2002) or prolonged torpor lasting for a few days (Brice et al., 2002; Kuchel, 2003; Western Australian Wheatbelt: Nowack et al., 2016).
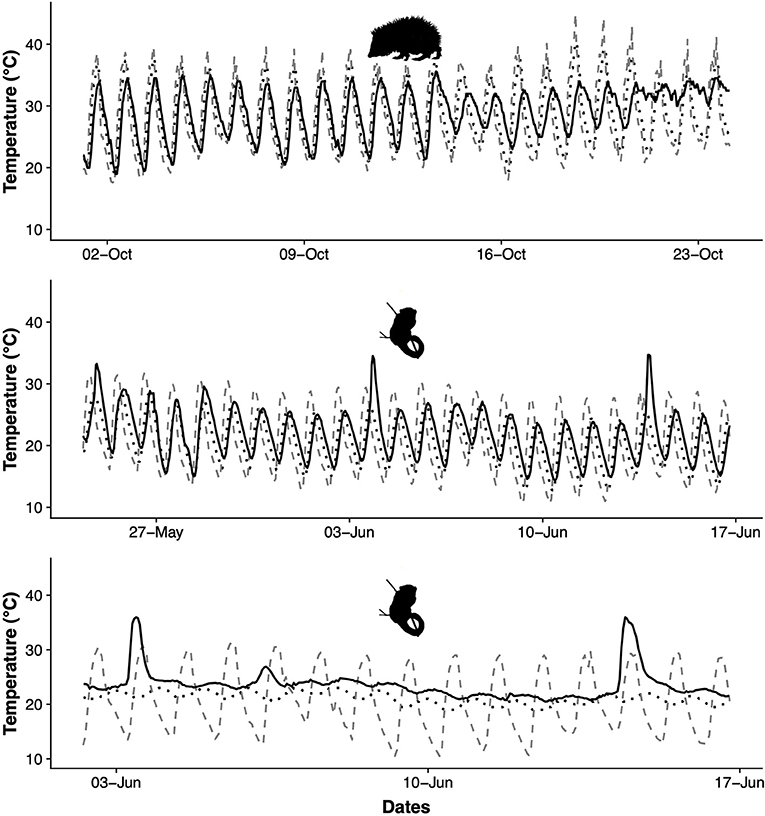
Figure 2. Top panel: Body temperature (black), ambient temperature (dashed gray) and temperature from a nearby nest site (dotted black) of a free ranging greater hedgehog tenrec (Setifer setosus) during the Austral winter (dry season). Redrawn from Lovegrove et al. (2014a) and Levesque et al. (2014). Middle and lower panel: Body temperature (black), ambient temperature (dashed gray) and tree-hole temperature (dotted black) of a free ranging fat-tailed dwarf lemur (Cheirogaleus medius) during the Austral winter (dry season) in a poorly insulated (middle) or moderately well insulated (lower panel) tree-hole.
Although this form of continuous heterothermy, either via torpor or highly variable Tb, has to date predominantly been found in spiny, terrestrial insectivores such as tenrecs and echidnas, it may possibly exist in other groups. For example, another tropical species that uses torpor on a regular day-to-day basis is the Angolan free-tailed bat, Tadarida aegyptiaca. This species uses daily torpor throughout the year and continuously maintains its Tb close to ambient temperatures when at rest (Vivier and van der Merwe, 2007). Given the large phylogenetic range covered by these three groups and the small number of studies investigating thermophysiology in the field, it is likely that there are other species of mammals with similar levels of thermolability that will be revealed with further study.
Seasonal Heterothermic Responses
Strictly seasonal use of torpor seems to be less common in lower latitude heterotherms than in their northern counterparts (Table 1). Furthermore, although most species show regular, seasonal use of daily or prolonged torpor, the use of hibernation, in the classical sense of animals disappearing for months at a time, is not as widespread in non-Holarctic species as it is in temperate/arctic mammalian lineages (Heldmaier et al., 2004; McKechnie and Mzilikazi, 2011; Ruf and Geiser, 2015). In contrast to those tenrec species highlighted above that use some form of heterothermic response during most of the year, other species, such as the tailless tenrec, Tenrec ecaudatus, are generally believed to only hibernate seasonally (Nicoll, 1986). Although based on more recent studies, it is highly likely that they can also use short bouts of torpor outside of the regular hibernation period (Lovegrove et al., 2014b; Treat et al., 2018).
Interestingly, the most strictly seasonal hibernators in the tropics are concentrated in one family of small, nocturnal Malagasy lemurs, the Cheirogaleidae (Table 1). It is likely that all species of this family (i.e., the genera Allocebus, Cheirogaleus, Microcebus, Mirza) are heterotherms, with the exception of members of one genus (Phaner, which has a very specific feeding regime that does not change seasonally) and most species of this family studied to date become torpid during the austral winter under free-ranging conditions (Dausmann, 2008, 2014). Thus, heterothermy in lemurs is a seasonal response, decreasing energy and water demands drastically thereby facilitating survival during the harsh (cold and dry) conditions of the Malagasy winter (Schmid and Speakman, 2000; Schmid et al., 2000). During the dry season in winter, temperatures decline across all habitats of Madagascar, although the extent varies. All dwarf lemurs (Cheirogaleus) are obligate hibernators in their natural environments (Petter, 1978; Hladik et al., 1980; Dausmann, 2008, 2014), from the eastern rainforests to the western dry forests. They hibernate either in tree hollows or buried underground between 3.5 and 8 months, depending on the seasonality of their habitats (Blanco et al., 2013; Dausmann, 2013; Dausmann and Blanco, 2016). As applies to most hibernators, this strategy requires preparation as well as sufficiently favorable conditions for recovery when hibernation is terminated, and reproduction needs to commence promptly. Thus, it can only be expressed in fairly predictable habitats. More flexible responses are shown by the mouse lemurs (Microcebus- over 20 species at present count). Most likely, all species are heterotherms and every mouse lemur species studied up to date has shown some form of seasonal torpor (Table 1). Some of these species have also shown months-long hibernation, however, in each case, only some individuals in a population did so, and individuals alternated between occasional torpor, short bouts of torpor, prolonged torpor and months-long hibernation both within and between winter seasons (Schmid and Ganzhorn, 2009; Kobbe et al., 2011). Allocebus and Mirza species have been reported or presumed to exhibit daily torpor during winter, albeit at different and flexible frequencies, however data have yet to be recorded (Dausmann and Warnecke, 2016; Table 1).
Most lower latitude hibernators, such as many bat species (see Table 1), short-beaked echidnas, Tachyglossus aculeatus (Grigg et al., 1989), and eastern pygmy-possums, Cercartetus nanus (Turner et al., 2012), are not strictly seasonal in their use of torpor and though they will use hibernation in winter, they also undergo shorter bouts of torpor during the rest of the year (see Opportunistic Torpor below). However, like the lemurs mentioned above, there are some species, which show a strict seasonal use of torpor (either daily or prolonged torpor) such as the Southern African hedgehog, Atelerix frontalis (Hallam and Mzilikazi, 2011), the African lesser bushbaby, Galago moholi (Nowack et al., 2010, 2013b), pygmy slow loris, Nycticebus pygmaeus (Streicher, 2004; Ruf et al., 2015), the Damaraland mole-rat, Fukomys damarensis (Streicher, 2010), and the Mountain pygmy possum, Burramys parvus (Geiser and Broome, 1991). However, it has to be noted that sufficient data on year round torpor use are lacking for most species with most measurements restricted to the winter when torpor is expected (Levesque et al., 2016).
Opportunistic Torpor
Similar to the tenrecs and echidnas mentioned above, a number seasonal heterotherms also employ torpor independent of season, if the conditions are sufficiently challenging (Table 1). Opportunistic torpor enables these species to respond promptly to unpredictable environmental changes, such as prolonged droughts or cold spells, as well as to an unusual shortage of food. For example, the eastern and rock elephant shrews, Elephantulus myurus and E. rupestris, routinely show short daily bouts of torpor during the winter, but also use daily torpor opportunistically throughout the year, presumably triggered by low temperatures and high air humidity (Mzilikazi and Lovegrove, 2004; Oelkrug et al., 2012). Furthermore, echidnas and marsupial antechinus (Antechinus spp.) have recently been reported to intensify torpor use in response to the threats of and the reduced food availability after fires (Stawski et al., 2015; Nowack et al., 2016). Other species, such as sugar gliders, Petaurus breviceps, or golden spiny mice, Acomys russatus, have been observed to use or intensify torpor in response to storms or floods (Nowack et al., 2015; Barak et al., 2019). Similarly, many non-Holarctic bat species exhibit prolonged torpor during winter (up to several days; reviewed by Geiser and Stawski, 2011) while also using opportunistic short bouts of torpor in other seasons. For example, the Northern long eared bat, Nyctophilus bifax, uses prolonged torpor (up to 5.4 days) during winter, but short bouts of torpor in response to cold weather conditions during summer (Stawski et al., 2009). Another, only recently studied, example is the Malagasy bat, Macronycteris commersoni. This species roosts in hot caves (≥32°C year-round) and displays a whole spectrum of different torpor responses during summer and winter (Reher et al., 2018). In summer, individuals may remain euthermic or enter torpor bouts lasting up to 6 days (sometimes coinciding with cyclones), while in winter their responses range from short torpor bouts, over prolonged torpor to hibernation with single bouts lasting up to 16 days; the triggers for the duration of torpor use remain unknown (Reher et al., unpublished data).
Other species also show opportunistic torpor independent of season, but in response to ambient conditions or food and water supply (Table 1). Amongst those are the pouched mouse, Saccostomus campestris, which entered torpor in the laboratory over a wide range of ambient temperatures and independent on photoperiod (Lovegrove and Raman, 1998; Mzilikazi and Lovegrove, 2002), the pichi, Zaedyus pichiy, which has been reported to use short bouts of torpor in spring after the hibernation season (Superina and Boily, 2007), the monito del monte, Dromiciops gliroides that entered torpor in the laboratory when kept under long photoperiod and relatively warm temperatures (20°C) (Bozinovic et al., 2004; Nespolo et al., 2010), and the striped faced dunnart, Sminthopsis macroura (Geiser and Baudinette, 1987; Song et al., 1997). In contrast, opportunistic use of heterothermy has been observed in only a handful of Holarctic species. The edible dormouse, Glis glis, for example, has been shown to re-enter hibernation already in July and to remain torpid until the following year (up to 11 months in total) in non-mast years when reproduction is skipped (Bieber and Ruf, 2009; Hoelzl et al., 2015).
Rare Use of Torpor
Not all heterotherms use torpor on a regular basis. A few species are known to be physiologically capable of employing torpor but do so only under adverse conditions and instead remain homeothermic whenever possible. A well-studied example is the African lesser bushbaby, Galago moholi. This species was long thought to be strictly homeothermic, despite cool environmental temperatures and a lack of food present in its habitat during winter. However, Nowack et al. (2010) detected sporadic daily torpor in a small fraction of the population. Since even these few (primarily juvenile and subadult) individuals became torpid only on single occasions, this suggests an unusual or specific trigger and is clearly not a regular seasonal response (Nowack et al., 2010, 2013b). Instead, behavioral and nutritional strategies are used by G. moholi to facilitate survival in winter, including larger sleeping groups, better insulated sleeping places, and changes in diet (Nowack et al., 2013c). In G. moholi, heterothermic phases are always shorter than 24 h and individuals have unusual difficulties rewarming to euthermic levels on cold days. The fact that G. moholi possess brown adipose tissue and the ability to use non-shivering thermogenesis suggests that individuals only enter torpor when their internal energy stores are depleted and that they have to rely on exogenous heat to return to active Tb (Nowack et al., 2013a).
Other heterothermic species which have been found to use torpor only rarely are the sugar glider, Petaurus breviceps (Christian and Geiser, 2007) and the feathertail glider, Acrobates pygmaeus (Jones and Geiser, 1992). Torpor use in P. breviceps seems to be triggered by especially cold and rainy days when animals remain in their nests instead of foraging (Körtner and Geiser, 2000; Nowack et al., 2015), enabling them to occur over a wide range of climatic conditions and even in cold temperate areas (snow and freezing conditions overnight). A. pygmaeus commonly exhibit bouts of short torpor but seem to be able to extend torpor use and undergo prolonged bouts when cold stressed (8–12°C; Jones and Geiser, 1992). Further examples of reluctant use of torpor are found in the rodent family. Although most Australian ash-gray mice, Pseudomys albocinereus, remained euthermic over a range of ambient temperatures when studied in the laboratory, one individual became torpid at 20 and 25°C (Barker et al., 2012). Body temperature recordings also suggest that Australian bush rats (Rattus fuscipes), which have a large geographical distribution, including tropical moist forests, can use torpor to compensate for lost feeding opportunities (unpublished data Nowack and Turbill). On the other hand, sandy inland mice (Pseudomys hermannsburgensis), who are also endemic to Australia, responded to food deprivation and low ambient temperature by becoming hypothermic without being able to arouse spontaneously (Tomlinson et al., 2007). More in-depth studies of species with large latitudinal ranges will provide better understanding of the flexibility of these responses.
Heterothermy as a singular response to acute emergency situations has to our knowledge not been reported for Holarctic mammals. This strategy, however, could be conceivable for members of the Sciuridae, which includes the classic hibernators (ground squirrels and chipmunks in the clade Marmotini). Interestingly, tree squirrels (sub-family Sciurinae) including European red squirrels (Sciurus vulgaris), have not been observed to enter torpid states (Dausmann et al., 2013), although it appears likely that closely-related flying squirrels are capable of shallow torpor (Olson et al., 2017). Tropical and sub-tropical sciurids have not been studied and more field studies are clearly needed to elucidate the potential for rare use of torpor in otherwise homeothermic mammals.
Differences in Body Temperature Are Related to Climate
One could assume that the clearest difference between tropical and Holarctic heterotherms is that Tb does not fall to extremely low levels. Indeed, with the exception of higher elevation habitats, ambient temperature rarely falls to life-threating temperatures below 0°C in the tropics even during winter, and thus individuals should not need mechanisms to avoid freezing. The record holders, in terms of low Tb, amongst mammals are the Arctic ground squirrel, Spermophilus parryii, and the European hazel dormice, Muscardinus avellanarius, both regulating a decrease in Tb down to as low as −2.9°C during hibernation without freezing (Barnes, 1989; Pretzlaff and Dausmann, 2012). Nonetheless, heterotherms from non-temperate or arctic areas can sometimes approach this temperature, and the southern African hedgehog, Atelerix frontalis, which is also one of the largest of all subtropical heterotherms (400–800 g), has been observed to hibernate with a Tb as low as 1°C (Hallam and Mzilikazi, 2011). In lemurs and tenrecs, the lowest Tb during hibernation recorded to date is 6.5°C in M. griseorufus (Table 1; Kobbe et al., 2011), but more commonly Tb is higher between 10°C and 30°C. Indeed, lemurs and tenrecs continue to hibernate even at Tbs >30°C (Figure 2). Similarly, eastern pygmy-possums (Cercartetus nanus) become torpid within their thermo-neutral zone and golden spiny mice (Acomys russatus) even use torpor at ambient temperatures as high as 35°C (Song et al., 1997; Grimpo et al., 2013).
To imperically test for differences in minimal torpid Tb and torpid metabolic rates between Holarctic and non-Holarctic species we used the “plgs” function in the R package “caper”[R version 3.6.2 (Orme et al., 2013; R Development Core Team, 2019)] on data from species in Table 1 and Holarctic species from Ruf and Geiser (2015) and controlled for phylogenetic relatedness using the mammal phylogeny by Upham et al. (2019). We tested for differences between torpor Tb using “tbmin~distribution” and in metabolic rate using “torpidMR~mass+distribution.” Holarctic species had significantly lower minimum torpid Tb (p = 0.001), and torpid metabolic rates (p < 0.0001) than non-Holarctic species, despite a high degree of overlap in both values between the two groups (Figure 3). However, in most, if not all, of these examples from non-Holarctic species the lowest Tb measured was bounded at the lower end by the coldest ambient temperatures, it is therefore difficult to predict if torpor patterns in non-Holarctic species would be similar at colder temperatures. The study of tropical heterotherms emphasizes that heterothermy must be defined on the basis of mechanistic, physiological parameters, such as significantly decreased metabolic rate (or heart rate), and not solely by Tb (Dausmann et al., 2004; Kobbe et al., 2011; McKechnie and Mzilikazi, 2011; Canale et al., 2012). However, our analysis has shown that we only have metabolic rate data from about half of the studied species (52% Holarctic and 57% of Non-Holarctic). Furthermore, the metabolic rate data in Table 1 do not necessarily represent minimum torpid metabolic rate for a species as they might have been taken in one study while longer/deeper torpor bouts have been recorded in another study in which only Tb has been measured (as in the case of Acomys russatus) or because metabolic rate data have been obtained under a (field-)laboratory setup while Tb has been obtained from free-ranging animals; in fact only eight studies that report torpor metabolic rates of non-Holoractic species have been measuring metabolic rates of animals in the field.
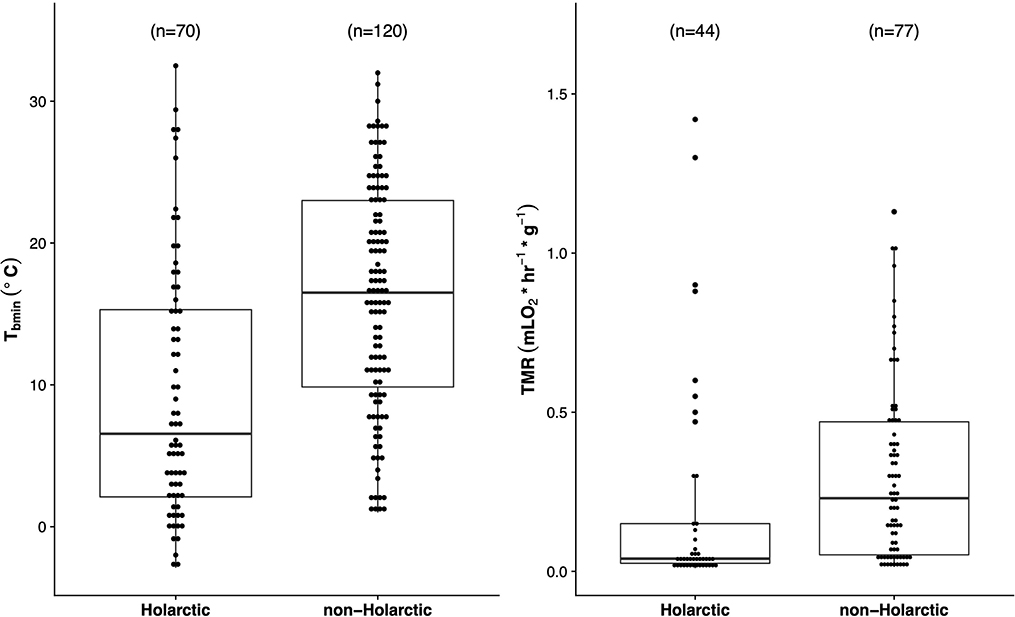
Figure 3. Boxplots (representing the minimum, maximum, median, first quartile and third quartile) of minimal torpid body temperatures (Tb;°C) and minimal torpid metabolic rate Tbmin for the non-Holarctic species with additional Holarctic species described by Ruf and Geiser (2015). Values for both were, on average, significantly higher in non-Holarctic species (see text).
The low risk of freezing in most tropical heterotherms has consequences for several aspects of their physiology including fat metabolism. In the fat-tailed dwarf lemur, C. medius, the main fuel during hibernation is monounsaturated oleic acid, which is synthesized preferentially from dietary carbohydrates obtained before food supply diminishes (sugary fruits; Fietz et al., 2003). This fat is stored in large quantities in the tails as triglycerides, resulting in an almost doubling of body mass (Fietz and Dausmann, 2007). Temperate hibernators, on the other hand, profit from a high content of polyunsaturated fatty acids (PUFAs) during hibernation. A diet rich in n-6 PUFAs has been shown to lead to longer and deeper torpor bouts (Geiser and Kenagy, 1987; Giroud et al., 2013) and thus higher energy savings during winter as they increase the activity of the sarcoplasmic reticulum Ca2+ ATPase (SERCA) and thus counteract the suppressed enzymatic activity and allow for greater fluidity of cell membranes at low temperature. Interestingly, n-3 PUFAs have a so far unexplained negative effect on torpor use (Giroud et al., 2013, 2018) and a diet rich in n-3 PUFAs has been shown to not only significantly reduce torpor use in temperate zone heterotherms but also in the tropical daily heterotherm, Microcebus murinus (Vuarin et al., 2016). However, the amount of n-6 and n-3 PUFAs in the diet is unlikely to be critical for tropical heterotherms as they usually do not hibernate at Tbs close to freezing (Goldman, 1975; Frank, 1991; Vuarin et al., 2016). They may thus avoid autoxidation and the production of toxic fatty acids peroxides, which are by-products of the metabolism of essential fatty acids.
Different Triggers of Torpor Use
Although torpor use in temperate and arctic regions is usually triggered by photoperiod, low temperatures, and limited food ability, the data summarized in Table 1 clearly shows the variety of factors that are triggering torpor in non-Holarctic species. As mentioned above, even during winter months, cold does not seem to be a necessity for tropical and subtropical hibernators and torpor bouts are often caused by seasonal low water availability instead of low food availability. Interestingly, mouse-tailed bats (Rhinopoma) are even considered incapable of entering torpor during cold periods (Kulzer, 1965). Levin et al. (2015) found that two species of mouse-tailed bats (Rhinopoma cystops, Rhinopoma microphyllum) hibernated in a geothermally heated cave in winter with a stable ambient temperature of 19–23.8°C although colder caves were available. Both species showed the lowest torpor metabolic rate at ~20°C and aroused at ambient temperature below 16°C (Levin et al., 2015). Thus, the availability of warm caves has allowed them to expand their subtropical distribution range from semi-arid and warm regions in Asia and Africa into southern Israel, the northern edge of their distribution (Levin et al., 2008, 2015). Similarly, the lesser long-eared bat (Nyctophilus geoffroyi) uses short bouts of torpor in tropical northern Australia in winter where ambient temperatures do not drop below 16.5°C (Geiser et al., 2011). Opportunistic torpor is often triggered by unpredictability in environmental parameters, such as unseasonal food or water shortages (e.g., droughts or fires), conditions that are less frequently encountered on an unpredictable basis in temperate and arctic regions.
The Advantages of Passive Arousals
Torpor at relatively high ambient temperatures still offers energetic savings, but without some of the major disadvantages. Many vital bodily functions can continue to operate at the comparatively high torpid Tb of non-Holarctic heterotherms and warming to euthermic levels is relatively inexpensive because animals can mostly rely on energy-saving, passive rewarming (Dausmann et al., 2009). An Australian desert bat, the inland free-tailed bat Ozimops petersi (former Mormopterus species 3; Lumsden, 2019), for example, can arouse from torpor without an obvious active thermogenic support (Bondarenco et al., 2013). Moreover, individuals can start being active at relatively low Tb, and then activity itself can be used as a means of heat production. Ortmann et al. (1997) first observed the so-called two-step arousal in a species of mouse lemur (M. murinus). Individuals rewarming from torpor initially use passive means via exogenous heat sources to raise their Tb to about 27°C before employing endogenous heat production, which keeps metabolic rate (and energy expenditure) during the rewarming process low. Since then, this mechanism has been verified in many other tropical heterotherms that closely synchronize their arousal times with the onset of rising environmental temperature which they may supplement by basking (Lovegrove et al., 1999; Schmid, 2000; Geiser et al., 2002; Mzilikazi et al., 2002; Geiser and Drury, 2003; Dausmann et al., 2005; Lovegrove and Génin, 2008; Warnecke et al., 2008; Kobbe et al., 2011; McKechnie and Mzilikazi, 2011; Nowack et al., 2013b; Dausmann et al., submitted). Although mostly observed in species inhabiting relatively warm habitats, a recent study found that Djungarian hamsters (Phodopus sungorus), originally inhabiting Asian steppes, actively seek the heat of a basking lamp when rewarming from torpor under laboratory conditions (Geiser et al., 2016). This finding suggests that the use of passive rewarming might also be common in Holarctic heterotherms.
Perhaps one of the most surprising discoveries from warm climate hibernators is that periodic arousals, thought to be essential to proper functioning during hibernation, are not necessarily needed (Dausmann et al., 2005; Lovegrove et al., 2014b). Depending on the nature of the hibernaculum (insulative capacities) and ambient temperature, the Tb of tropical species can fluctuate widely during hibernation (daily fluctuations of Tb can exceed 25°C per day). Under these conditions, when Tb occasionally rises passively above about 30°C, the expensive arousals are abandoned in Cheirogaleus (Figure 2). In contrast, individuals hibernating in better insulated sites (large trees or underground), which have a lower but more stable Tb, show regular periodic arousals, just like their temperate or arctic counterparts (Dausmann et al., 2004, 2005). Similarly, the common tenrec (Tenrec ecaudatus) forgoes arousal completely while hibernating in an underground burrow where Tbs remain above 25°C for the winter (Lovegrove et al., 2014b). Although the ultimate factors necessitating periodic arousals in hibernators remain enigmatic (proposed explanations include reduction of oxidative stress and sleep debt, production of gene products, activation of the immune system, limitation of neurophysiological damage; Carey et al., 2003), it seems clear that the capacity to attain a high Tb passively determines whether or not arousals are required (Dausmann, 2014). For example, arctic ground squirrels, S. parryii, consistently sleep during arousals (Daan et al., 1991). Their electroencephalography shows the decrease in slow wave activity as would be expected when sleep debt had increased during the preceding phase of torpor. Thus, it has been postulated that the need for sleep slowly accumulates during torpor and that returning to euthermy is periodically required to sleep (Daan et al., 1991; Trachsel et al., 1991). Indeed, C. medius display aspects of sleep (rapid eye movement sleep) during the phases of passively heated warmer Tb during hibernation (in contrast to phases of low Tb), possibly preventing the accumulation of sleep debt, and ensuring brain function and memory consolidation in individuals with highly fluctuating Tb (Krystal et al., 2013). To date, the only other mammal to not exhibit periodic arousals besides hibernating lemurs and tenrecs (Dausmann et al., 2004; Lovegrove et al., 2014b) are black bears, Ursus americanus, hibernating at Tb of >30°C (Tøien et al., 2011). The opportunity to abandon periodic arousals not only saves energy, but also limits the damaging high level of oxidative stress resulting from the increased production of reactive oxygen species during active rewarming (Carey et al., 2003; Giroud et al., 2009).
Inter- and Intraspecific Phenotypic Flexibility of Torpor Use in the Tropics
Most interestingly, the distinction between the use of short torpor bouts and hibernation is less clear-cut in non-Holarctic heterotherms (Mzilikazi and Lovegrove, 2004; Cory Toussaint et al., 2010; Kobbe et al., 2011; Canale et al., 2012). Whereas, most Holarctic species usually either hibernate or use daily torpor, warmer climate species often show a mixed use of shorter and longer bouts. In the tropics, there is more variation between closely related species at the same site, between populations of the same species at different sites, between individuals within a population, and even in individuals between years (Dausmann, 2014). As outlined above, some species can switch between hibernation (several months), prolonged torpor over several days, becoming torpid for a few hours sporadically or daily (opportunistic) use of short torpor bouts, or remaining homeothermic. As the best examples of this phenotypic flexibility come from southern Madagascar, the driest and least predictable habitat of the island, we suggest that this flexibility may enable tropical species to inhabit not only seasonal, but also unpredictable habitats. It enables species to respond to the context of specific environmental parameters and their own body condition. For example, in the lemur Microcebus griseorufus and the bat Macronycteris commersoni all of these responses have been observed in different individuals in the same population at the same site (Kobbe and Dausmann, 2009; Kobbe et al., 2011; Reher et al., 2018). Similarly, western rock elephant shrews, E. rupestris, are highly heterothermic in South Africa, whereas the closely-related cape rock elephant shrews, E. edwardii, at the same site remain mostly homeothermic, although the species is capable of short to prolonged bouts of torpor (McKechnie and Mzilikazi, 2011; Boyles et al., 2012). Hottentot golden moles, Amblysomus hottentotus longiceps, and southern African hedgehogs, A. frontalis, seem to be capable of diverse thermoregulatory responses at the same site, and under the same conditions in captivity (Scantlebury et al., 2008; Hallam and Mzilikazi, 2011). Despite hibernation being a more fixed response, there is also variation in thermoregulatory patterns during hibernation, such as in dwarf lemurs and tenrecs, where the insulative properties of the hibernacula determine the occurrence and extent of daily Tb fluctuations and the occurrence of periodic arousals.
Furthermore, the duration of hibernation can be flexible between different populations of the same species, depending on the duration of the period of scarceness in the particular habitat. For example, C. medius hibernates up to 8 months in the western dry forests of Madagascar, but only 3.5 months in the south-western littoral forests, where the dry season is much shorter (Lahann and Dausmann, 2011). Differences in thermoregulatory patterns might also emerge as a result of competition with other, closely related sympatric species. Only half as many individual M. griseorufus entered hibernation in a habitat where the larger M. murinus is present in the littoral forest in the south of Madagascar, compared to where M. griseorufus occurs alone the spiny forest of the south-west (Kobbe et al., unpublished data). As hibernation is thought to be the more advantageous strategy in Microcebus species (higher energy savings; predation avoidance), M. griseorufus could be outcompeted for access to food before the hibernation period by the larger M. murinus, limiting pre-hibernation fattening. If true, then M. griseorufus may have to resort to short or prolonged torpor episodes to cope with the Malagasy winter in areas where it occurs together with M. murinus.
Tropical Origins of Heterothermy, and Endothermy, in Mammals
It now seems increasingly likely that endothermy in mammals evolved from the ancestral ectothermic condition via a heterothermic state (Grigg et al., 2004; Geiser and Stawski, 2011; Lovegrove, 2017). All extant mammals are capable of metabolic heat production (Lovegrove, 2012), but the degree to which they regulate their Tb, both in terms of absolute temperature and level of variability, varies considerably (Boyles et al., 2013; Clarke and O'Connor, 2014). Mammals evolved under climatic conditions similar to modern day tropics, where ambient temperature is high for most of the year, and the costs of maintaining a comparatively high, stable Tb, especially at a lower level (~32°C like in tenrecs and echidnas) would be relatively low (Levesque et al., 2013). This notion is supported by the fact that early mammalian ancestors had small body masses, were nocturnal, and mostly insectivorous (McNab, 1978; Kemp, 2006; O'Leary et al., 2013), similar to the hedgehog tenrecs. Moreover, it has been postulated that small mammals in particular cannot sustain the high rates of evaporation necessary to maintain a Tb more than 2°C below ambient for more than a few hours (Crompton et al., 1978), because of substantial water loss needed for evaporative cooling. Often, tropical and subtropical heterotherms inhabit dry environments and water savings might even be more essential for survival than energy savings (Schmid and Speakman, 2000; Dausmann, 2014). Water loss, however, could be avoided if Tb is regulated slightly above ambient, and the outward flow of heat is varied by insulation, therefore, a switch to a diurnal activity pattern in some species would have necessitated higher Tbs (Crompton et al., 1978), which in turn were only made possible with the evolution of the scrotum (Lovegrove, 2019). The evolution of endothermy in mammals is an emergent property of the evolution of various characteristics that aid in either heat production (e.g., thermogenesis, UCP1) and heat dissipation (e.g., insulation, external scrotums) and happened to a different degree across the mammalian lineage (Lovegrove, 2012, 2019; Seebacher, 2018; Jastroch and Seebacher, 2020). By studying the added level of variability in many thermoregulatory traits, torpor use in particular, observed in species in the tropics and sub-tropics can help shed further light on how endothermy evolved in mammals.
Although advances in understanding the ecological and physiological underpinnings of tropical heterothermy have been substantial, many questions remain. For example, we need to learn more about how blood parameters (lactate levels, as a proxy for hypoxia in the tissue), immunocompetence and sleeping patterns in tropical hibernators differ compared to hibernators with continuously low Tb, especially if we wish to disentangle hibernation specific and low Tb specific physiological responses, which could help elucidating the evolution of homeothermy.
Author Contributions
JN and KD conceived of the idea and wrote the first version of the manuscript. DL ran the analyses and prepared the figures for the manuscript. All authors contributed to the final version of the manuscript.
Conflict of Interest
The authors declare that the research was conducted in the absence of any commercial or financial relationships that could be construed as a potential conflict of interest.
The reviewer FG, declared a past collaboration with the authors to the handling editor.
Acknowledgments
We want to thank William Foley for his valuable comments on an early draft of the manuscript and Thomas Ruf and Fritz Geiser for providing us with a copy of the data of their 2015 review paper.
References
Anderson, D. M. (2004). Aardwolf adaptations: a review. Trans. R. Soc. S. Afr. 59, 99–104. doi: 10.1080/00359190409519168
Arnold, J. (1976). Growth and bioenergetics of the Chuditch, Dasyurus geoffroii (Phd thesis), University of Western Australia, Perth, WA, Australia.
Atherton, R. G., and Haffenden, A. T. (1982). Observations on the reproduction and growth of the long-tailed pygmy-possum Cercartetus caudatus (Marsupialia: Burramyidae), in captivity. Aust. Mammal. 5, 253–259.
Atsalis, S. (1999). Seasonal fluctuations in body fat and activity levels in a rain-forest species of mouse lemur, Microcebus rufus. Int. J. Primatol. 20, 883–910. doi: 10.1023/A:1020826502103
Audet, D., and Thomas, D. W. (1997). Facultative hypothermia as a thermoregulatory strategy in the phyllostomid bats, Carollia perspicillata and Sturnira lilium. J. Comp. Physiol. B 167, 146–152. doi: 10.1007/s003600050058
Augee, M. L., and Ealy, E. H. M. (1968). Torpor in the echidna, Tachyglossus aculeatus. J. Mammal. 49, 446–d454.
Barak, O., Geiser, F., and Kronfeld-Schor, N. (2019). Flood-induced multiday torpor in golden spiny mice (Acomys russatus). Aust. J. Zool. 66, 401–405. doi: 10.1071/ZO19061
Barker, J. M., Cooper, C. E., Withers, P. C., and Cruz-Neto, A. P. (2012). Thermoregulation by an Australian murine rodent, the ash-grey mouse (Pseudomys albocinereus). Comp. Biochem. Physiol. A Mol. Integr. Physiol. 163, 336–342. doi: 10.1016/j.cbpa.2012.07.011
Barnes, B. M. (1989). Freeze avoidance in a mammal: body temperatures below 0°C in an arctic hibernator. Science 244, 1593–1595. doi: 10.1126/science.2740905
Barnes, B. M., Kretzmann, M., Licht, P., and Zucker, I. (1986). The influence of hibernation on testis growth and spermatogenesis in the golden-mantled ground squirrel, Spermophilus lateralis. Biol. Reprod. 35, 1289–1297. doi: 10.1095/biolreprod35.5.1289
Bartels, W., Law, B. S., and Geiser, F. (1998). Daily torpor and energetics in a tropical mammal, the nothern blossom-bat Macroglossus minimus (Megachiroptera). J. Comp. Physiol. B 168, 233–239. doi: 10.1007/s003600050141
Bartholomew, G. A., Dawson, W. R., and Lasiewski, R. C. (1970). Thermoregulation and heterothermy in some of the smaller flying foxes (Megachiroptera) of New Guinea. Zeitschrift Vergleichende Physiol. 70, 196–209. doi: 10.1007/BF00297716
Baxter, R. M. (1996). Evidence for spontaneous torpor in Crocidura flavescens. Acta Theriol. 41, 327–330. doi: 10.4098/AT.arch.96-31
Bennett, N., Jarvis, J., and Cotteril, F. (1993). Poikilothermic traits and thermoregulation in the Afrotropical social subterranean Mashona mole-rat (Cryptomys hottentotus darlingi) (Rodentia: Bathyergidae). J. Zool. 231, 179–186. doi: 10.1111/j.1469-7998.1993.tb01910.x
Bieber, C., and Ruf, T. (2009). Summer dormancy in edible dormice (Glis glis) without energetic constraints. Naturwissenschaften 96, 165–171. doi: 10.1007/s00114-008-0471-z
Blanco, M., and Rahalinarivo, V. (2010). First direct evidence of hibernation in an eastern dwarf lemur species (Cheirogaleus crossleyi) from the high-altitude forest of Tsinjoarivo, central-eastern Madagascar. Naturwissenschaften 97, 945–950. doi: 10.1007/s00114-010-0707-6
Blanco, M. B., Dausmann, K. H., Ranaivoarisoa, J. F., and Yoder, A. D. (2013). Underground hibernation in a primate. Sci. Rep. 3:1768. doi: 10.1038/srep01768
Bondarenco, A., Körtner, G., and Geiser, F. (2013). Some like it cold: summer torpor by freetail bats in the Australian arid zone. J. Comp. Physiol. B 183, 1113–1122. doi: 10.1007/s00360-013-0779-7
Bondarenco, A., Körtner, G., and Geiser, F. (2016). How to keep cool in a hot desert: torpor in two species of free-ranging bats in summer. Temperature 3, 476–483. doi: 10.1080/23328940.2016.1214334
Boyles, J. G., Smit, B., Sole, C. L., and McKechnie, A. E. (2012). Body temperature patterns in two syntopic elephant shrew species during winter. Comp. Biochem. Physiol. A Mol. Integr. Physiol. 161, 89–94. doi: 10.1016/j.cbpa.2011.09.007
Boyles, J. G., Thompson, A. B., McKechnie, A. E., Malan, E., Humphries, M. M., and Careau, V. (2013). A global heterothermic continuum in mammals. Glob. Ecol. Biogeogr. 22, 1029–1039. doi: 10.1111/geb.12077
Bozinovic, F., and Marquet, P. A. (1991). Energetics and torpor in the Atacama desert-dwelling rodent Phyllotis darwinirupestris. J. Mammal. 72, 734–738. doi: 10.2307/1381835
Bozinovic, F., Muñoz, J. L. P., Naya, D. E., and Cruz-Neto, A. P. (2007). Adjusting energy expenditures to energy supply: food availability regulates torpor use and organ size in the Chilean mouse-opossum Thylamys elegans. J. Comp. Physiol. B 177:393. doi: 10.1007/s00360-006-0137-0
Bozinovic, F., and Rosenmann, M. (1988). Daily torpor in Calomys musculinus, a South American rodent. J. Mammal. 69, 150–152. doi: 10.2307/1381762
Bozinovic, F., Ruiz, G., and Rosenmann, M. (2004). Energetics and torpor of a South American “living fossil”, the microbiotheriid Dromiciops gliroides. J. Comp. Physiol. B 174, 293–297. doi: 10.1007/s00360-004-0414-8
Brice, P. H., Grigg, G. C., Beard, L. A., and Donovan, J. A. (2002). Patterns of activity and inactivity in echidnas (Tachyglossus aculeatus) free-ranging in a hot dry climate: correlates with ambient temperature, time of day and season. Austr. J. Zool. 50, 461–475. doi: 10.1071/ZO01080
Brown, G. C. (1999). Metabolism and thermoregulation of individual and clustered long-fingered bats, Miniopterus schreibersii, and the implications for roosting. S. Afr. J. Zool. 34, 166–172. doi: 10.1080/02541858.1999.11448505
Brown, J. C. L., and Staples, J. F. (2011). Mitochondrial metabolic suppression in fasting and daily torpor: consequences for reactive oxygen species production. Physiol. Biochem. Zool. 84, 467–480. doi: 10.1086/661639
Buffenstein, R. (1984). Energy and water balance during torpor and hydropenia in the pigmy gerbil, Gerbillus pusillus. J. Comp. Physiol. B 154, 535–544. doi: 10.1007/BF02515159
Busse, S., Lutter, D., Heldmaier, G., Jastroch, M., and Meyer, C. W. (2014). Torpor at high ambient temperature in a neotropical didelphid, the grey short-tailed opossum (Monodelphis domestica). Naturwissenschaften 101, 1003–1006. doi: 10.1007/s00114-014-1226-7
Canale, C. I., Levesque, D. L., and Lovegrove, B. G. (2012). “Tropical heterothermy: does the exception prove the rule or force a re-definition? “in Living in a Seasonal World: Thermoregulatory and Metabolic Adaptations, eds T. Ruf, C. Bieber, W. Arnold, and E. Millesi (Berlin; Heidelberg: Springer), 29–40. doi: 10.1007/978-3-642-28678-0_3
Carey, H. V., Andrews, M. T., and Martin, S. L. (2003). Mammalian hibernation: cellular and molecular responses to depressed metabolism and low temperature. Physiol. Rev. 83, 1153–1181. doi: 10.1152/physrev.00008.2003
Carey, H. V., Frank, C. L., and Seifert, J. P. (2000). Hibernation induces oxidative stress and activation of NF-κB in ground squirrel intestine. J. Comp. Physiol. B 170, 551–559. doi: 10.1007/s003600000135
Caviedes-Vidal, E., Codelia, E. C., Roig, V., and Doña, R. (1990). Facultative torpor in the South American rodent Calomys venustus (Rodentia: Cricetidae). J. Mammal. 71, 72–75. doi: 10.2307/1381319
Chmura, H. E., Glass, T. W., and Williams, C. T. (2018). Biologging physiological and ecological responses to climatic variation: new tools for the climate change era. Front. Ecol. Evol. 6:92. doi: 10.3389/fevo.2018.00092
Christian, N., and Geiser, F. (2007). To use or not to use torpor? Activity and body temperature as predictors. Naturwissenschaften 94, 483–487. doi: 10.1007/s00114-007-0215-5
Clarke, A., and O'Connor, M. I. (2014). Diet and body temperature in mammals and birds. Glob. Ecol. Biogeogr. 23, 1000–1008. doi: 10.1111/geb.12185
Coburn, D. K., and Geiser, F. (1998). Seasonal changes in energetics and torpor patterns in the subtropical blossom-bat Syconycteris australis (Megachiroptera). Oecologia 113, 467–473. doi: 10.1007/s004420050399
Collins, B., Wooler, R., and Richardson, K. (1987). Torpor by the honey possum, Tarsipes rostratus (Marsupialia: Tarsipedidae), in response to food shortage and low environmental temperature. Aust. Mammal. 11, 51–57.
Cooper, C. E., and Withers, P. C. (2004). Patterns of body temperature variation and torpor in the numbat, Myrmecobius fasciatus (Marsupialia: Myrmecobiidae). J. Therm. Biol. 29, 277–284. doi: 10.1016/j.jtherbio.2004.05.003
Cooper, C. E., and Withers, P. C. (2010). Comparative physiology of Australian quolls (Dasyurus; Marsupialia). J. Comp. Physiol. B 180, 857–868. doi: 10.1007/s00360-010-0452-3
Cooper, C. E., Withers, P. C., and Cruz-Neto, A. P. (2009). Metabolic, ventilatory, and hygric physiology of the gracile mouse opossum (Gracilinanus agilis). Physiol. Biochem. Zool. 82, 153–162. doi: 10.1086/595967
Cory Toussaint, D., Brigham, R. M., and McKechnie, A. E. (2013). Thermoregulation in free-ranging Nycteris thebaica (Nycteridae) during winter: No evidence of torpor. Mammal. Biol. 78, 365–368. doi: 10.1016/j.mambio.2012.10.001
Cory Toussaint, D., McKechnie, A. E., and van der Merwe, M. (2010). Heterothermy in free-ranging male Egyptian Free-tailed bats (Tadarida aegyptiaca) in a subtropical climate. Mamm. Biol. Z. Saugetierkd. 75, 466–470. doi: 10.1016/j.mambio.2009.06.001
Cossins, A. R., and Barnes, B. (1996). Southern discomfort. Nature 382, 582–583. doi: 10.1038/382582a0
Crompton, A. W., Taylor, C. R., and Jagger, J. A. (1978). Evolution of homeothermy in mammals. Nature 272, 333–336. doi: 10.1038/272333a0
Cryan, P. M., and Wolf, B. O. (2003). Sex differences in the thermoregulation and evaporative water loss of a heterothermic bat, Lasiurus cinereus, during its spring migration. J. Exp. Biol. 206:3381. doi: 10.1242/jeb.00574
Daan, S., Barnes, B. M., and Strijkstra, A. M. (1991). Warming up for sleep? Ground squirrels sleep during arousals from hibernation. Neurosci. Lett. 128, 265–268. doi: 10.1016/0304-3940(91)90276-Y
Dausmann, K. H. (2008). “Hypometabolism in primates: Torpor and hibernation,” in Hypometabolism in Animals: Hibernation, Torpor and Cryobiology, eds B. G. Lovegrove and A. E. Mckechnie (Pietermaritzburg: Interpak), 327–336.
Dausmann, K. H. (2013). “Spoilt for choice: selection of hibernacula by Cheirogaleus medius,” in Leaping Ahead: Advances in Prosimian Biology, eds J. Masters, M. Gamba, and F. Génin (New York, NY: Springer New York), 205–214. doi: 10.1007/978-1-4614-4511-1_23
Dausmann, K. H. (2014). Flexible patterns in energy savings: heterothermy in primates. J. Zool. 292, 101–111. doi: 10.1111/jzo.12104
Dausmann, K. H., and Blanco, M. B. (2016). “Possible causes and consequences of different hibernation patterns in Cheirogaleus species: Mitovy fatsy sahala,” in Dwarf and Mouse Lemurs of Madagascar: Biology, Behavior and Conservation Biogeography of the Cheirogaleidae, eds S. M. Lehman, U. Radespiel, and E. Zimmermann (Camebridge: Cambridge University Press), 335–349. doi: 10.1017/CBO9781139871822.018
Dausmann, K. H., Glos, J., Ganzhorn, J. U., and Heldmaier, G. (2004). Physiology: hibernation in a tropical primate. Nature 429, 825–826. doi: 10.1038/429825a
Dausmann, K. H., Glos, J., Ganzhorn, J. U., and Heldmaier, G. (2005). Hibernation in the tropics: lessons from a primate. J. Comp. Physiol. B 175, 147–155. doi: 10.1007/s00360-004-0470-0
Dausmann, K. H., Glos, J., and Heldmaier, G. (2009). Energetics of tropical hibernation. J. Comp. Physiol. B. 175, 147–155. doi: 10.1007/s00360-008-0318-0
Dausmann, K. H., Nowack, J., Kobbe, S., and Mzilikazi, N. (2012). “Afrotropical heterothermy: a continuum of possibilities,” in Living in a Seasonal World: Thermoregulatory and Metabolic Adaptations: 14th Hibernation Symposium, eds T. Ruf, C. Bieber, W. Arnold, and E. Millesi (Berlin; Heidelberg; New York, NY: Springer), 13–27. doi: 10.1007/978-3-642-28678-0_2
Dausmann, K. H., and Warnecke, L. (2016). Primate torpor expression: ghost of the climatic past. Physiology 31, 398–408. doi: 10.1152/physiol.00050.2015
Dausmann, K. H., Wein, J., Turner, J. M., and Glos, J. (2013). Absence of heterothermy in the European red squirrel (Sciurus vulgaris). Mamm. Biol. Z. Saugetierkd. 78, 332–335. doi: 10.1016/j.mambio.2013.01.004
Dawson, T. J., and Wolfers, J. M. (1978). Metabolism, thermoregulation and torpor in shrew sized marsupials of the genus planigale. Comp. Biochem. Physiol. A 59, 305–309. doi: 10.1016/0300-9629(78)90167-6
Eisentraut, M. (1956). Der Winterschlaf mit seinen ökologischen und physiologischen Begleiterscheinungen. Jena: Fischer.
Ellison, G. T. H. (1995). Thermoregulatory responses of cold-acclimated fat mice (Steatomys pratensis). J. Mammal. 76, 240–247. doi: 10.2307/1382332
Everson, K. M., Soarimalala, V., Goodman, S. M., and Olson, L. E. (2016). Multiple loci and complete taxonomic sampling resolve the phylogeny and biogeographic history of tenrecs (Mammalia: Tenrecidae) and reveal higher speciation rates in madagascar's humid forests. Syst. Biol. 65, 890–909. doi: 10.1093/sysbio/syw034
Fielden, L., Waggoner, J., Perrin, M., and Hickmann, G. (1990). Thermoregulation in the Namib Desert golden mole, Eremitalpa granti namibensis (Chrysochloridae). J. Arid Environ. 18, 221–237. doi: 10.1016/S0140-1963(18)30856-5
Fietz, J., and Dausmann, K. H. (2007). “Big is beautiful: fat storage and hibernation as a strategy to cope with marked seasonality in the fat-tailed dwarf lemur (Cheirogaleus medius),” in Lemurs: Ecology and Adaptation, eds L. Gould and M. L. Sauther (Boston, MA: Springer US), 97–110. doi: 10.1007/978-0-387-34586-4_5
Fietz, J., Schlund, W., Dausmann, K. H., Regelmann, M., and Heldmaier, G. (2004). Energetic constraints on sexual activity in the male edible dormouse (Glis glis). Oecologia 138, 202–209. doi: 10.1007/s00442-003-1423-0
Fietz, J., Tataruch, F., Dausmann, K., and Ganzhorn, J. U. (2003). White adipose tissue composition in the free-ranging fat-tailed dwarf lemur (Cheirogaleus medius; Primates), a tropical hibernator. J. Comp. Physiol. B 173, 1–10. doi: 10.1007/s00360-002-0300-1
Fleming, M. (1980). Thermoregulation and torpor in the sugar glider, Petaurus Breviceps (Marsupialia:Petauridae). Aust. J. Zool. 28, 521–534. doi: 10.1071/ZO9800521
Frank, C. L. (1991). Adaptations for hibernation in the depot fats of a ground squirrel (Spermophilus beldingi). Can. J. Zool. 69, 2707–2711. doi: 10.1139/z91-382
Geiser, F. (1986). Thermoregulation and torpor in the kultarr, Antechinomys laniger (Marsupialia: Dasyuridae). J. Comp. Physiol. B 156, 751–757. doi: 10.1007/BF00692755
Geiser, F. (1987). Hibernation and daily torpor in two pygmy possums (Cercartetus spp., Marsupialia). Physiol. Zool. 60, 93–102. doi: 10.1086/physzool.60.1.30158631
Geiser, F. (1988). Daily torpor and thermoregulation in antechinus (Marsupialia): Influence of body mass, season, development, reproduction, and sex. Oecologia 77, 395–399. doi: 10.1007/BF00378050
Geiser, F. (1993). Hibernation in the eastern pygmy-possum, Cercartetus nanus (Marsupialia, Burramyidae). Aust. J. Zool. 41, 67–75. doi: 10.1071/ZO9930067
Geiser, F. (2004a). Metabolic rate and body temperature reduction during hibernation and daily torpor. Annu. Rev. Physiol. 66, 239–274. doi: 10.1146/annurev.physiol.66.032102.115105
Geiser, F. (2004b). The role of torpor in the life of Australian arid zone mammals. Aust. Mammal. 26, 125–134. doi: 10.1071/AM04125
Geiser, F., Augee, M. L., McCarron, H. C. K., and Raison, J. K. (1984). Correlates of torpor in the insectivorous dasyurid marsupial Sminthopsis murina. Aust. Mammal. 7, 185–191.
Geiser, F., and Baudinette, R. V. (1987). Seasonality of torpor and thermoregulation in three dasyurid marsupials. J. Comp. Physiol. B 157, 335–344. doi: 10.1007/BF00693360
Geiser, F., and Baudinette, R. V. (1988). Daily torpor and thermoregulation in the small dasyurid marsupials Planigale gilesi and Ningaui yvonneae. Aust. J. Zool. 36, 473–481. doi: 10.1071/ZO9880473
Geiser, F., and Brigham, R. M. (2000). Torpor, thermal biology, and energetics in Australian long-eared bats (Nyctophilus). J. Comp. Physiol. B 170, 153–162. doi: 10.1007/s003600050270
Geiser, F., and Brigham, R. M. (2012). “The other functions of torpor,” in Living in a Seasonal World, eds T. Ruf, C. Bieber, W. Arnold, and E. Millesi (Berlin; Heidelberg: Springer), 109–121. doi: 10.1007/978-3-642-28678-0_10
Geiser, F., and Broome, L. S. (1991). Hibernation in the mountain pygmy possum Burramys parvus (Marsupialia). J. Zool. 223, 593–602. doi: 10.1111/j.1469-7998.1991.tb04390.x
Geiser, F., and Drury, R. L. (2003). Radiant heat affects thermoregulation and energy expenditure during rewarming from torpor. J. Comp. Physiol. B 173, 55–60. doi: 10.1007/s00360-002-0311-y
Geiser, F., and Ferguson, C. (2001). Intraspecific differences in behaviour and physiology: effects of captive breeding on patterns of torpor in feathertail gliders. J. Comp. Physiol. B 171, 569–576. doi: 10.1007/s003600100207
Geiser, F., Gasch, K., Bieber, C., Stalder, G. L., Gerritsmann, H., and Ruf, T. (2016). Basking hamsters reduce resting metabolism, body temperature and energy costs during rewarming from torpor. J. Exp. Biol. 219, 2166–2172. doi: 10.1242/jeb.137828
Geiser, F., Goodship, N., and Pavey, C. R. (2002). Was basking important in the evolution of mammalian endothermy? Naturwissenschaften 89, 412–414. doi: 10.1007/s00114-002-0349-4
Geiser, F., and Kenagy, G. J. (1987). Polyunsaturated lipid diet lengthens torpor and reduces body temperature in a hibernator. Am. J. Physiol. Regul. Integr. Comp. Physiol. 252, R897–R901. doi: 10.1152/ajpregu.1987.252.5.R897
Geiser, F., and Martin, G. M. (2013). Torpor in the Patagonian opossum (Lestodelphys halli): implications for the evolution of daily torpor and hibernation. Naturwissenschaften 100, 975–981. doi: 10.1007/s00114-013-1098-2
Geiser, F., and Masters, P. (1994). Torpor in relation to reproduction in the mulgara, Dasycercus cristicauda (Dasyuridae: Marsupialia). J. Therm Biol. 19, 33–40. doi: 10.1016/0306-4565(94)90007-8
Geiser, F., and Mzilikazi, N. (2011). Does torpor of elephant shrews differ from that of other heterothermic mammals? J. Mammal. 92, 452–459. doi: 10.1644/10-MAMM-A-097.1
Geiser, F., and Pavey, C. R. (2007). Basking and torpor in a rock-dwelling desert marsupial: Survival strategies in a resource-poor environment. J. Comp. Physiol. B 177, 885–892. doi: 10.1007/s00360-007-0186-z
Geiser, F., and Ruf, T. (1995). Hibernation versus daily torpor in mammals and birds: physiological variables and classification of torpor patterns. Physiol. Zool. 68, 935–966. doi: 10.1086/physzool.68.6.30163788
Geiser, F., and Stawski, C. (2011). Hibernation and torpor in tropical and subtropical bats in relation to energetics, extinctions, and the evolution of endothermy. Integr. Comp. Biol. 51, 337–348. doi: 10.1093/icb/icr042
Geiser, F., Stawski, C., Bondarenco, A., and Pavey, C. R. (2011). Torpor and activity in a free-ranging tropical bat: implications for the distribution and conservation of mammals? Naturwissenschaften 98, 447–452. doi: 10.1007/s00114-011-0779-y
Genoud, M., Bonaccorso, F. J., and Anends, A. (1990). Rate of metabolism and temperature regulation in two small tropical insectivorous bats (Peropteryx macrotis and Natalus tumidirostris). Comp. Biochem. Physiol. A 97, 229–234. doi: 10.1016/0300-9629(90)90177-T
Giroud, S., Frare, C., Strijkstra, A., Boerema, A., Arnold, W., and Ruf, T. (2013). Membrane phospholipid fatty acid composition regulates cardiac SERCA activity in a hibernator, the Syrian hamster (Mesocricetus auratus). PLoS ONE 8:e63111. doi: 10.1371/journal.pone.0063111
Giroud, S., Perret, M., Gilbert, C., Zahariev, A., Goudable, J., Maho, Y. L., et al. (2009). Dietary palmitate and linoleate oxidations, oxidative stress, and DNA damage differ according to season in mouse lemurs exposed to a chronic food deprivation. Am. J. Physiol. Regul. Integr. Comp. Physiol. 297, R950–R959. doi: 10.1152/ajpregu.00214.2009
Giroud, S., Stalder, G., Gerritsmann, H., Kübber-Heiss, A., Kwak, J., Arnold, W., et al. (2018). Dietary lipids affect the onset of hibernation in the garden dormouse (Eliomys quercinus): implications for cardiac function. Front. Physiol. 9, 1235. doi: 10.3389/fphys.2018.01235
Goldman, S. (1975). Cold resistance of the brain during hibernation. III. Evidence of a lipid adaptation. Am. J. Physiol. Cell Physiol. 228, 834–838. doi: 10.1016/0003-9861(75)90197-6
Gould, E., and Eisenberg, J. F. (1966). Notes on the biology of the Tenrecidae. J. Mammal. 47, 660–686. doi: 10.2307/1377896
Grant, T. R., and Temple-Smith, P. D. (1987). “Observations on torpor in the small marsupial Dromiciops australis (Marsupialia: Microbiotheriidae),” in Possums and Opossums, ed. M. Archer (Sydney, NSW: Royal Zoological Society), 273–277.
Grigg, G., and Beard, L. (2000). “Hibernation by echidnas in mild climates: hints about the evolution of endothermy?” in Life in the Cold, eds G. Heldmaier and M. Klingenspor (Berlin; Heidelberg: Springer), 5–19. doi: 10.1007/978-3-662-04162-8_1
Grigg, G. C. (2004). “An evolutionary framework for studies of hibernation and short-term torpor,” in Life in the Cold 2004: The Twelfth International Hibernation Symposium (LITC 2004), eds B. M. Barnes and H. V. Carey (Fairbanks, AK: Institute of Arctic Biology, University of Alaska), 131–141.
Grigg, G. C., Beard, L. A., and Augee, M. L. (1989). Hibernation in a monotreme, the echidna (Tachyglossus aculeatus). Comp. Biochem. Physiol. A Physiol. 92, 609–612. doi: 10.1016/0300-9629(89)90375-7
Grigg, G. C., Beard, L. A., and Augee, M. L. (2004). The evolution of endothermy and its diversity in mammals and birds. Physiol. Biochem. Zool. 77, 982–997. doi: 10.1086/425188
Grimpo, K., Legler, K., Heldmaier, G., and Exner, C. (2013). That's hot: golden spiny mice display torpor even at high ambient temperatures. J. Comp. Physiol. B 183, 567–581. doi: 10.1007/s00360-012-0721-4
Hallam, S. L., and Mzilikazi, N. (2011). Heterothermy in the southern African hedgehog, Atelerix frontalis. J. Comp. Physiol. B 181, 437–445. doi: 10.1007/s00360-010-0531-5
Heldmaier, G., Ortmann, S., and Elvert, R. (2004). Natural hypometabolism during hibernation and daily torpor in mammals. Respir. Physiol. Neurobiol. 141, 317–329. doi: 10.1016/j.resp.2004.03.014
Hladik, C. M., Charles-Dominique, P., and Petter, J. J. (1980). “Feeding strategies of five nocturnal prosimians in the dry forest of the west coast of Madagascar,” in Nocturnal Malagasy Primates: Ecology, Physiology, and Behavior, eds P. Charles-Dominique, H. M. Cooper, A. Hladik, C. M. Hladik, E. Pages, G. F. Pariente, A. Petter-Rousseaux, and A. Schilling (New York, NY: Academic Press), 41–73. doi: 10.1016/B978-0-12-169350-3.50007-1
Hoelzl, F., Bieber, C., Cornils, J. S., Gerritsmann, H., Stalder, G. L., Walzer, C., et al. (2015). How to spend the summer? Free-living dormice (Glis glis) can hibernate for 11 months in non-reproductive years. J. Comp. Physiol. B 185, 931–939. doi: 10.1007/s00360-015-0929-1
Hosken, D. J., and Withers, P. C. (1997). Temperature regulation and metabolism of an Australian bat, Chalinolobus gouldii (Chiroptera: Vespertilionidae) when euthermic and torpid. J. Comp. Physiol. B 167, 71–80. doi: 10.1007/s003600050049
Hudson, J. W., and Scott, I. M. (1979). Daily torpor in the laboratory mouse, Mus musculus var. albino. Physiol. Zool. 52, 205–218. doi: 10.1086/physzool.52.2.30152564
Hume, I., Beiglböck, C., Ruf, T., Frey-Roos, F., Bruns, U., and Arnold, W. (2002). Seasonal changes in morphology and function of the gastrointestinal tract of free-living alpine marmots (Marmota marmota). J. Comp. Physiol. B 172, 197–207. doi: 10.1007/s00360-001-0240-1
Hume, T., Geiser, F., Currie, S. E., Körtner, G., and Stawski, C. (2019). Responding to the weather: energy budgeting by a small mammal in the wild. Curr. Zool. 66, 15–20. doi: 10.1093/cz/zoz023
Jacobs, D. S., Kelly, E. J., Mason, M., and Stoffberg, S. (2007). Thermoregulation in two free-ranging subtropical insectivorous bat species: Scotophilus species (Vespertilionidae). Canad. J. Zool. 85, 883–890. doi: 10.1139/Z07-067
Jastroch, M., and Seebacher, F. (2020). Importance of adipocyte browning in the evolution of endothermy. Philos. Trans. R. Soc. Lond. B Biol. Sci. 375:20190134. doi: 10.1098/rstb.2019.0134
Jones, C. J., and Geiser, F. (1992). Prolonged and daily torpor in the feathertail glider, Acrobates pygmaeus (Marsupialia: Acrobatidae). J. Zool. 227, 101–108. doi: 10.1111/j.1469-7998.1992.tb04347.x
Jones, K. E., Bielby, J., Cardillo, M., Fritz, S. A., O'Dell, J., Orme, C. D. L., et al. (2009). PanTHERIA: a species-level database of life history, ecology, and geography of extant and recently extinct mammals. Ecology 90, 2648–2648. doi: 10.1890/08-1494.1
Kelm, D., and Helversen, O. (2007). How to budget metabolic energy: torpor in a small Neotropical mammal. J. Comp. Physiol. B 177, 667–677. doi: 10.1007/s00360-007-0164-5
Kemp, T. S. (2006). The origin of mammalian endothermy: a paradigm for the evolution of complex biological structure. Zool. J. Linnean Soc. 147, 473–488. doi: 10.1111/j.1096-3642.2006.00226.x
Kobbe, S., and Dausmann, K. H. (2009). Hibernation in Malagasy mouse lemurs as a strategy to counter environmental challenge. Naturwissenschaften 96, 1221–1227. doi: 10.1007/s00114-009-0580-3
Kobbe, S., Ganzhorn, J., and Dausmann, K. H. (2011). Extreme individual flexibility of heterothermy in free-ranging Malagasy mouse lemurs (Microcebus griseorufus). J. Comp. Physiol. B 181, 165–173. doi: 10.1007/s00360-010-0507-5
Kobbe, S., Nowack, J., and Dausmann, K. H. (2014). Torpor is not the only option: seasonal variations of the thermoneutral zone in a small primate. J. Comp. Physiol. B 184, 789–797. doi: 10.1007/s00360-014-0834-z
Körtner, G., and Geiser, F. (2000). Torpor and activity patterns in free-ranging sugar gliders Petaurus breviceps (Marsupialia). Oecologia 123, 350–357. doi: 10.1007/s004420051021
Körtner, G., and Geiser, F. (2008). The key to winter survival: Daily torpor in a small arid-zone marsupial. Naturwissenschaften 96:525. doi: 10.1007/s00114-008-0492-7
Körtner, G., Pavey, C. R., and Geiser, F. (2008). Thermal biology, torpor, and activity in free-living mulgaras in arid zone Australia during the winter reproductive season. Physiol. Biochem. Zool. 81, 442–451. doi: 10.1086/589545
Körtner, G., Riek, A., Pavey, C. R., and Geiser, F. (2016). Activity patterns and torpor in two free-ranging carnivorous marsupials in arid Australia in relation to precipitation, reproduction, and ground cover. J. Mammal. 97, 1555–1564. doi: 10.1093/jmammal/gyw113
Krystal, A. D., Schopler, B., Kobbe, S., Williams, C., Rakatondrainibe, H., Yoder, A. D., et al. (2013). The relationship of sleep with temperature and metabolic rate in a hibernating primate. PLoS ONE 8:e69914. doi: 10.1371/journal.pone.0069914
Kuchel, L. (2003). The energetics and patterns of torpor in free-ranging Tachyglossus aculeatus from a warm-temperate climate (Ph.D.) University of Queensland, Brisbane.
Kulzer, E. (1965). Temperaturregulation bei Fledermäusen (Chiroptera) aus verschiedenen Klimazonen. Z. Vgl. Physiol. 50, 1–34. doi: 10.1007/BF00388050
Kulzer, E., Nelson, J. E., McKean, J. L., and Möhres, P. F. (1970). Untersuchungen über die Temperaturregulation australischer Fledermäuse. Zeitschrift Vergleichende Physiol. 69, 426–451. doi: 10.1007/BF00333769
Kulzer, E., and Storf, R. (1980). Sleep-lethargy in the African long-tongued fruit bat Megaloglossus woermanni pagenstecher, 1885. Int. J. Mammal. Biol. 45, 23–29.
Lahann, P. (2007). Biology of Cheirogaleus major in a littoral rain forest in Southeast Madagascar. Int. J. Primatol. 28, 895–905. doi: 10.1007/s10764-007-9163-3
Lahann, P., and Dausmann, K. (2011). Live fast, die young: flexibility of life history traits in the fat-tailed dwarf lemur (Cheirogaleus medius). Behav. Ecol. Sociobiol. 65, 381–390. doi: 10.1007/s00265-010-1055-4
Levesque, D. L., Lobban, K. D., and Lovegrove, B. G. (2014). Effects of reproductive status and high ambient temperatures on the body temperature of a free-ranging basoendotherm. J. Comp. Physiol. B 184, 1041–1053. doi: 10.1007/s00360-014-0858-4
Levesque, D. L., Lovasoa, O. M. A., Rakotoharimalala, S. N., and Lovegrove, B. G. (2013). High mortality and annual fecundity in a free-ranging basal placental mammal, Setifer setosus (Tenrecidae: Afrosoricida). J. Zool. 291, 205–212. doi: 10.1111/jzo.12063
Levesque, D. L., and Lovegrove, B. G. (2014). Increased homeothermy during reproduction in a basal placental mammal. J. Exp. Biol. 217, 1535–1542. doi: 10.1242/jeb.098848
Levesque, D. L., Nowack, J., and Stawski, C. (2016). Modelling mammalian energetics: the heterothermy problem. Clim. Change Responses 3:7. doi: 10.1186/s40665-016-0022-3
Levin, E., Ar, A., Yom-Tov, Y., and Kronfeld-Schor, N. (2012). “Summer torpor and sexual segregation in the subtropical bat Rhinopoma microphyllum,” in Living in a Seasonal World: Thermoregulatory and Metabolic Adaptations, eds T. Ruf, C. Bieber, W. Arnold, and E. Millesi. (Berlin; Heidelberg: Springer Berlin Heidelberg), 167–174. doi: 10.1007/978-3-642-28678-0_15
Levin, E., Plotnik, B., Amichai, E., Braulke, L. J., Landau, S., Yom-Tov, Y., et al. (2015). Subtropical mouse-tailed bats use geothermally heated caves for winter hibernation. Proc. R. Soc. B Biol. Sci. 282:20142781. doi: 10.1098/rspb.2014.2781
Levin, E., Yom-Tov, Y., Barnea, A., and Huchon, D. (2008). Genetic diversity and phylogeography of the greater mouse-tailed bat Rhinopoma microphyllum (Brünnich, 1782) in the Levant. Acta Chiropterol. 10, 207–212. doi: 10.3161/150811008X414791
Liu, J.-N., and Karasov, W. H. (2011). Hibernation in warm hibernacula by free-ranging formosan leaf-nosed bats, Hipposideros terasensis, in subtropical Taiwan. J. Comp. Physiol. B 181, 125–135. doi: 10.1007/s00360-010-0509-3
Liu, J.-N., and Karasov, W. H. (2012). Metabolism during winter in a subtropical hibernating bat, the Formosan leaf-nosed bat (Hipposideros terasensis). J. Mammal. 93, 220–229. doi: 10.1644/11-MAMM-A-144.1
Lovegrove, B. G. (2012). The evolution of endothermy in cenozoic mammals: a plesiomorphic-apomorphic continuum. Biol. Rev. 87, 128–162. doi: 10.1111/j.1469-185X.2011.00188.x
Lovegrove, B. G. (2017). A phenology of the evolution of endothermy in birds and mammals. Biol. Rev. 92, 1213–1240. doi: 10.1111/brv.12280
Lovegrove, B. G. (2019). Obligatory nocturnalism in triassic archaic mammals: preservation of sperm quality? Physiol. Biochem. Zool. 92, 544–553. doi: 10.1086/705440
Lovegrove, B. G., Canale, C. I., Levesque, D. L., Fluch, G., Reháková-Petru, M., and Ruf, T. (2014a). Are tropical small mammals physiologically vulnerable to arrhenius effects and climate change? Physiol. Biochem. Zool. 87, 30–45. doi: 10.1086/673313
Lovegrove, B. G., and Génin, F. (2008). Torpor and hibernation in a basal placental mammal, the lesser hedgehog tenrec Echinops telfairi. J. Comp. Physiol. B 168, 303–312. doi: 10.1007/s00360-008-0257-9
Lovegrove, B. G., Körtner, G., and Geiser, F. (1999). The energetic cost of arousal from torpor in the marsupial Sminthopsis macroura: benefits of summer ambient temperature cycles. J. Comp. Physiol. B 169, 11–18. doi: 10.1007/s003600050188
Lovegrove, B. G., Lobban, K. D., and Levesque, D. L. (2014b). Mammal survival at the cretaceous–palaeogene boundary: metabolic homeostasis in prolonged tropical hibernation in tenrecs. Proc. R. Soc. B Biol. Sci. 281:20141304. doi: 10.1098/rspb.2014.1304
Lovegrove, B. G., and Raman, J. (1998). Torpor patterns in the pouched mouse (Saccostomus campestris; Rodetia): a model animal for unpredictable environments. J. Comp. Physiol. B 168, 303–312. doi: 10.1007/s003600050150
Lovegrove, B. G., Raman, J., and Perrin, M. R. (2001). Heterothermy in elephant shrews, Elephantulus spp. (Macroscelidea): daily torpor or hibernation? J. Comp. Physiol. B 171, 1–10. doi: 10.1007/s003600000139
Lumsden, L. F. (2019). Ozimops petersi. The IUCN Red List of Threatened Species e.T71534469A147359477. doi: 10.2305/IUCN.UK.2019-2.RLTS.T71534469A147359477.en
Maloney, S. K., Bronner, G. N., and Buffenstein, R. (1999). Thermoregulation in the Angolan free-tailed bat Mops condylurus: a small mammal that uses hot roosts. Physiol. Biochem. Zool. 72, 385–396. doi: 10.1086/316677
Marom, S., Korine, C., Wojciechowski, Michał S., Tracy, Christopher, R., and Pinshow, B. (2006). Energy metabolism and evaporative water loss in the european free-tailed bat and hemprich's long-eared bat (microchiroptera): species sympatric in the Negev Desert. Physiol. Biochem. Zool. 79, 944–956. doi: 10.1086/505999
McKechnie, A. E., and Mzilikazi, N. (2011). Heterothermy in Afrotropical mammals and birds: a review. Integr. Comp. Biol. 51, 349–363. doi: 10.1093/icb/icr035
McNab, B. K. (1978). The evolution of endothermy in the phylogeny of mammals. Am. Nat. 112, 1–21. doi: 10.1086/283249
McNab, B. K., and Bonaccorso, F. J. (2001). The metabolism of New Guinean pteropodid bats. J. Comp. Physiol. B 171, 201–214. doi: 10.1007/s003600000163
Morrison, P., and McNab, B. K. (1962). Daily torpor in a Brazilian murine opossum (marmosa). Comp. Biochem. Physiol. 6, 57–68. doi: 10.1016/0010-406X(62)90043-9
Morton, S. R., and Lee, A. K. (1978). Thermoregulation and metabolism in Planigale maculata (Marsupialia: Dasyuridae). J. Therm. Biol. 3, 117–120. doi: 10.1016/0306-4565(78)90003-7
Muller, J. (1996). Torpor in Sminthopsis douglasi (Honours thesis), LaTrobe University, Melbourne, VIC, Australia.
Murray, M. H., Donald, W. T., and Donald, L. K. (2003). The role of energy availability in mammalian hibernation: a cost-benefit approach. Physiol. Biochem. Zool. 76, 165–179. doi: 10.1086/367950
Mzilikazi, N., and Lovegrove, B. (2002). Reproductive activity influences thermoregulation and torpor in pouched mice, Saccostomus campestris. J. Comp. Physiol. B 172, 7–16. doi: 10.1007/s003600100221
Mzilikazi, N., and Lovegrove, B. G. (2004). Daily torpor in free-ranging rock elephant shrews, Elephantulus myurus: a year-long study. Physiol. Biochem. Zool. 77, 285–296. doi: 10.1086/381470
Mzilikazi, N., Lovegrove, B. G., and Ribble, G. O. (2002). Exogenous passive heating during torpor arousal in free-ranging rock elephant shrews, Elephantulus myurus. Oecologia 133, 307–314. doi: 10.1007/s00442-002-1052-z
Mzilikazi, N., Madikiza, Z., Oelkrug, R., and Baxter, R. M. (2012). “Hibernation in free-ranging African woodland dormice, Graphiurus murinus,” in Living in a Seasonal World, eds T. Ruf, C. Bieber, W. Arnold and E. Millesi (Berlin; Heidelberg: Springer), 41–50. doi: 10.1007/978-3-642-28678-0_4
Nespolo, R. F., Verdugo, C., Cortés, P. A., and Bacigalupe, L. D. (2010). Bioenergetics of torpor in the microbiotherid marsupial, Monito del Monte (Dromiciops gliroides): the role of temperature and food availability. J. Comp. Physiol. B 180, 767–773. doi: 10.1007/s00360-010-0449-y
Nicol, S., and Andersen, N. A. (2002). The timing of hibernation in Tasmanian echidnas: why do they do it when they do? Comp. Biochem. Physiol. Biochem. Mol. Biol. 131, 603–611.
Nicol, S. C., and Andersen, N. A. (2006). Body temperature as an indicator of egg-laying in the echidna, Tachyglossus aculeatus. J. Therm. Biol. 31, 483–490. doi: 10.1016/j.jtherbio.2006.05.001
Nicoll, M. E. (1986). Diel variation in body temperature in Tenrec ecaudatus during seasonal hypothermia. J. Mammal. 67, 759–762. doi: 10.2307/1381143
Nowack, J., Cooper, C. E., and Geiser, F. (2016). Cool echidnas survive the fire. Proc. Biol. Sci 283:20160382. doi: 10.1098/rspb.2016.0382
Nowack, J., Dausmann, K. H., and Mzilikazi, N. (2013a). Nonshivering thermogenesis in the African lesser bushbaby, Galago moholi. J. Exp. Biol. 216, 3811–3817. doi: 10.1242/jeb.089433
Nowack, J., Mzilikazi, N., and Dausmann, K. H. (2010). Torpor on demand: heterothermy in the non-lemur primate Galago moholi. PLoS ONE 5:e10797. doi: 10.1371/journal.pone.0010797
Nowack, J., Mzilikazi, N., and Dausmann, K. H. (2013b). Torpor as an emergency solution in Galago moholi: heterothermy is triggered by different constraints. J. Comp. Physiol. B 183, 547–556. doi: 10.1007/s00360-012-0725-0
Nowack, J., Rojas, A. D., Körtner, G., and Geiser, F. (2015). Snoozing through the storm: torpor use during a natural disaster. Sci. Rep. 5:11243. doi: 10.1038/srep11243
Nowack, J., Stawski, C., and Geiser, F. (2017). More functions of torpor and their roles in a changing world. J. Comp. Physiol. B 187, 889–897. doi: 10.1007/s00360-017-1100-y
Nowack, J., Tarmann, I., Hoelzl, F., Smith, S., Giroud, S., and Ruf, T. (2019). Always a price to pay: hibernation at low temperatures comes with a trade-off between energy savings and telomere damage. Biol. Lett. 15:20190466. doi: 10.1098/rsbl.2019.0466
Nowack, J., Wippich, M., Mzilikazi, N., and Dausmann, K. H. (2013c). Surviving the cold, dry period in Africa: behavioral adjustments as an alternative to heterothermy in Galago moholi. Int. J. Primatol. 34, 49–64. doi: 10.1007/s10764-012-9646-8
Oelkrug, R., Goetze, N., Exner, C., Lee, Y., Ganjam, G. K., Kutschke, M., et al. (2013). Brown fat in a protoendothermic mammal fuels eutherian evolution. Nat. Commun. 4:2140. doi: 10.1038/ncomms3140
Oelkrug, R., Meyer, C., Heldmaier, G., and Mzilikazi, N. (2012). Seasonal changes in thermogenesis of a free-ranging afrotherian small mammal, the western rock elephant shrew (Elephantulus rupestris). J. Comp. Physiol. B 182, 715–727. doi: 10.1007/s00360-012-0647-x
O'Leary, M. A., Bloch, J. I., Flynn, J. J., Gaudin, T. J., Giallombardo, A., Giannini, N. P., et al. (2013). The placental mammal ancestor and the post–K-Pg radiation of placentals. Science 339, 662–667. doi: 10.1126/science.1229237
Olson, M. N., Bowman, J., and Burness, G. (2017). Seasonal energetics and torpor use in North American flying squirrels. J. Therm. Biol. 70, 46–53. doi: 10.1016/j.jtherbio.2017.10.006
O'Mara, M. T., Rikker, S., Wikelski, M., Maat, A. T., Pollock, H. S., and Dechmann, D. K. N. (2017). Heart rate reveals torpor at high body temperatures in lowland tropical free-tailed bats. R. Soc. Open Sci. 4:171359. doi: 10.1098/rsos.171359
Opazo, J. C., Nespolo, R. F., and Bozinovic, F. (1999). Arousal from torpor in the chilean mouse-opposum (Thylamys elegans): does non-shivering thermogenesis play a role? Comp. Biochem. Physiol. A 123, 393–397. doi: 10.1016/S1095-6433(99)00081-1
Orme, D., Freckleton, R., Petzoldt, T., and Fritz, S. (2013). The Caper Package: Comparative Analysis of Phylogenetics and Evolution. R.R package version 5. Available online at: https://cran.r-project.org/web/packages/caper/caper.pdf
Ortmann, S., Heldmaier, G., Schmid, J., and Ganzhorn, J. U. (1997). Spontaneous daily torpor in malagasy mouse lemurs. Naturwissenschaften 84, 28–32. doi: 10.1007/s001140050344
Perrin, M. R., and Ridgard, B. W. (1999). Thermoregulation and patterns of torpor in the spectacled dormouse, Graphiurus ocularis (A. Smith 1829) (Gliridae). Trop. Zool. 12, 253–266. doi: 10.1080/03946975.1999.10539392
Petter, J. (1978). “Ecological and physiological adaptations of five sympatric nocturnal lemurs to seasonal variations in food production,” in Recent Advances in Primatology, eds D. J. Chivers and J. Herbert (New York, NY: Academic Press), 211–223.
Poppitt, S. D., Speakman, J. R., and Racey, P. A. (1994). Energetics of reproduction in the lesser hedgehog tenrec, Echinops telfairi (Martin). Physiol. Zool. 67, 976–994. doi: 10.1086/physzool.67.4.30163874
Poux, C., Madsen, O., Marquard, E., Vieites, D. R., de Jong, W. W., and Vences, M. (2005). Asynchronous colonization of madagascar by the four endemic clades of primates, tenrecs, carnivores, and rodents as inferred from nuclear genes. Syst. Biol. 54, 719–730. doi: 10.1080/10635150500234534
Pretzlaff, I., and Dausmann, K. H. (2012). “Impact of climatic variation on the hibernation physiology of Muscardinus avellanarius,” in Living in a Seasonal World, eds T. Ruf, C. Bieber, W. Arnold, and E. Millesi (Berlin; Heidelberg: Springer), 85–97. doi: 10.1007/978-3-642-28678-0_8
R Development Core Team (2019). R: A Language and Environment for Statistical Computing. Vienna: R Foundation for Statistical Computing.
Randrianambinina, B., Rakotondravony, D., Radespiel, U., and Zimmermann, E. (2003). Seasonal changes in general activity, body mass and reproduction of two small nocturnal primates: a comparison of the golden brown mouse lemur (Microcebus ravelobensis) in Northwestern Madagascar and the brown mouse lemur (Microcebus rufus) in Eastern Madagascar. Primates 44, 321–331. doi: 10.1007/s10329-003-0046-8
Rasweiler, J. (1973). Care and management of the long-tongued bat, Glossophaga soricina (Chiroptera: Phyllostomatidae), in the laboratory, with observations on estivation induced by food deprivation. J. Mammal. 54, 391–404. doi: 10.2307/1379126
Reher, S., Ehlers, J., Rabarison, H., and Dausmann, K. H. (2018). Short and hyperthermic torpor responses in the Malagasy bat Macronycteris commersoni reveal a broader hypometabolic scope in heterotherms. J. Comp. Physiol. B 188, 1015–1027. doi: 10.1007/s00360-018-1171-4
Revell, L. J. (2012). Phytools: an R package for phylogenetic comparative biology (and other things). Methods Ecol. Evol. 3, 217–223. doi: 10.1111/j.2041-210X.2011.00169.x
Rojas, A. D., Körtner, G., and Geiser, F. (2014). Torpor in free-ranging antechinus: does it increase fitness? Naturwissenschaften 101, 105–114. doi: 10.1007/s00114-013-1136-0
Ruf, T., and Geiser, F. (2015). Daily torpor and hibernation in birds and mammals. Biol. Rev. 90, 891–926. doi: 10.1111/brv.12137
Ruf, T., Streicher, U., Stalder, G. L., Nadler, T., and Walzer, C. (2015). Hibernation in the pygmy slow loris (Nycticebus pygmaeus): multiday torpor in primates is not restricted to Madagascar. Sci. Rep. 5:17392. doi: 10.1038/srep17392
Scantlebury, M., Lovegrove, B., Jackson, C., Bennett, N., and Lutermann, H. (2008). Hibernation and non-shivering thermogenesis in the Hottentot golden mole (Amblysomus hottentottus longiceps). J. Comp. Physiol. B 178, 887–897. doi: 10.1007/s00360-008-0277-5
Schmid, J. (2000). Daily torpor in the gray mouse lemur (Microcebus murinus) in Madagascar: energetic consequences and biological significance. Oecologica 123, 175–183. doi: 10.1007/s004420051003
Schmid, J., and Ganzhorn, J. U. (2009). Optional strategies for reduced metabolism in gray mouse lemurs. Naturwissenschaften 96, 737–741. doi: 10.1007/s00114-009-0523-z
Schmid, J., Ruf, J., and Heldmaier, G. (2000). Metabolism and temperature regulation during daily torpor in the smallest primate, the pygmy mouse lemur (Microcebus myoxinus) in Madagascar. J. Comp. Physiol. B 170, 59–68. doi: 10.1007/s003600050008
Schmid, J., and Speakman, J. R. (2000). Daily energy expenditure of the grey mouse lemur (Microcebus murinus): a small primate that uses torpor. J. Comp. Physiol. B 170, 633–641. doi: 10.1007/s003600000146
Schubert, K. A., Boerema, A. S., Vaanholt, L. M., de Boer, S. F., Strijkstra, A. M., and Daan, S. (2010). Daily torpor in mice: high foraging costs trigger energy-saving hypothermia. Biol. Lett. 6, 132–135. doi: 10.1098/rsbl.2009.0569
Seebacher, F. (2018). The evolution of metabolic regulation in animals. Comp. Biochem. Physiol. B Biochem. Mol. Biol. 224, 195–203. doi: 10.1016/j.cbpb.2017.11.002
Sheriff, M. J., Fridinger, R. W., Tøien, ø., Barnes, B. M., and Buck, C. L. (2013). Metabolic rate and prehibernation fattening in free-living arctic ground squirrels. Physiol. Biochem. Zool. 86, 515–527. doi: 10.1086/673092
Song, X., Körtner, G., and Geiser, F. (1997). Thermal relations of metabolic rate reduction in a hibernating marsupial. Am. J. Physiol. Regul. Integr. Comp. Physiol. 273, R2097–R2104. doi: 10.1152/ajpregu.1997.273.6.R2097
Song, X., Körtner, G., and Geiser, F. (1998). Temperature selection and use of torpor by the marsupial Sminthopsis macroura. Physiol. Behav. 64, 675–682. doi: 10.1016/S0031-9384(98)00136-X
Soriano, P. J., Ruiz, A., and Arends, A. (2002). Physiological responses to ambient temperature manipulation by three species of bats from Andean cloud forests. J. Mammal. 83, 445–457. doi: 10.1644/1545-1542(2002)083<0445:PRTATM>2.0.CO;2
Stawski, C., Körtner, G., Nowack, J., and Geiser, F. (2015). The importance of mammalian torpor for survival in a post-fire landscape. Biol. Lett. 11:20150134. doi: 10.1098/rsbl.2015.0134
Stawski, C., Turbill, C., and Geiser, F. (2009). Hibernation by a free-ranging subtropical bat (Nyctophilus bifax). J. Comp. Physiol. B 179, 433–441. doi: 10.1007/s00360-008-0328-y
Stephenson, P. J., and Racey, P. A. (1993). Reproductive energetics of the Tenrecidae (Mammalia: Insectivora). II. The shrew-tenrecs, Microgale spp. Physiol. Zool. 66, 664–685. doi: 10.1086/physzool.66.5.30163817
Stephenson, P. J., and Racey, P. A. (1994). Seasonal variation in resting metabolic rate and body temperature of streaked tenrecs, Hemicentetes nigriceps and H. semispinosus (Insectivora: Tenrecidae). J. Zool. 232, 285–294. doi: 10.1111/j.1469-7998.1994.tb01573.x
Streicher, S. (2010). The effect of environmental variables on patterns of body temperature in the Damaraland mole-rat, Fukomys damarensis (Ogilby 1838) (MSc). Pretoria: University of Pretoria.
Streicher, U. (2004). Aspects of ecology and conservation of the pygmy loris Nycticebus pygmaeus in Vietnam (Ph.D.). Ludwig-Maximilians-Universität München, Munich.
Superina, M., and Boily, P. (2007). Hibernation and daily torpor in an armadillo, the pichi (Zaedyus pichiy). Comp. Biochem. Physiol. A Mol. Integr. Physiol. 148, 893–898. doi: 10.1016/j.cbpa.2007.09.005
Tøien, Ø., Blake, J., Edgar, D. M., Grahn, D. A., Heller, H. C., and Barnes, B. M. (2011). Hibernation in black bears: independence of metabolic suppression from body temperature. Science 331, 906–909. doi: 10.1126/science.1199435
Tomlinson, S., Withers, P. C., and Cooper, C. (2007). Hypothermia versus torpor in response to cold stress in the native Australian mouse Pseudomys hermannsburgensis and the introduced house mouse Mus musculus. Comp. Biochem. Physiol. A Mol. Integr. Physiol. 148, 645–650. doi: 10.1016/j.cbpa.2007.08.013
Tomlinson, S., Withers, P. C., and Maloney, S. K. (2012). Flexibility in thermoregulatory physiology of two dunnarts, Sminthopsis macroura and Sminthopsis ooldea (Marsupialia; Dasyuridae). J. Exp. Biol. 215, 2236–2246. doi: 10.1242/jeb.065516
Trachsel, L., Edgar, D. M., and Heller, H. C. (1991). Are ground squirrels sleep deprived during hibernation? Am. J. Physiol. 260, R1123–R1129. doi: 10.1152/ajpregu.1991.260.6.R1123
Treat, M. D., Scholer, L., Barrett, B., Khachatryan, A., McKenna, A. J., Reyes, T., et al. (2018). Extreme physiological plasticity in a hibernating basoendothermic mammal, Tenrec ecaudatus. J. Exp. Biol. 221:jeb185900. doi: 10.1242/jeb.185900
Turbill, C. (2006). Thermoregulatory behavior of tree-roosting chocolate wattled bats (Chalinolobus morio) during summer and winter. J. Mammal. 87, 318–323. doi: 10.1644/05-MAMM-A-167R1.1
Turbill, C., and Geiser, F. (2008). Hibernation by tree-roosting bats. J. Comp. Physiol. B 178:597. doi: 10.1007/s00360-007-0249-1
Turbill, C., Körtner, G., and Geiser, F. (2003). Natural use of heterothermy by a small, tree-roosting bat during summer. Physiol. Biochem. Zool. 76, 868–876. doi: 10.1086/378915
Turner, J. M., Körtner, G., Warnecke, L., and Geiser, F. (2012). Summer and winter torpor use by a free-ranging marsupial. Comp. Biochem. Physiol. A Mol. Integr. Physiol. 162, 274–280. doi: 10.1016/j.cbpa.2012.03.017
Upham, N. S., Esselstyn, J. A., and Jetz, W. (2019). Inferring the mammal tree: Species-level sets of phylogenies for questions in ecology, evolution, and conservation. PLoS Biol. 17:e3000494. doi: 10.1371/journal.pbio.3000494
Vivier, L., and van der Merwe, M. (2007). The incidence of torpor in winter and summer in the Angolan free-tailed bat, Mops condylurus (Microchiroptera: Molossidae), in a subtropical environment, Mpumulanga, South Africa. Afr. Zool. 42, 50–58. doi: 10.3377/1562-7020(2007)42[50:TIOTIW]2.0.CO;2
Vuarin, P., Henry, P.-Y., Perret, M., and Pifferi, F. (2016). Dietary supplementation with n-3 polyunsaturated fatty acids reduces torpor use in a tropical daily heterotherm. Physiol. Biochem. Zool. 89, 536–545. doi: 10.1086/688659
Warnecke, L., Turner, J., and Geiser, F. (2008). Torpor and basking in a small arid zone marsupial. Naturwissenschaften 95, 73–78. doi: 10.1007/s00114-007-0293-4
Wein, J. (2010). Effects of ambient temperature on tropical hibernation in the lesser hedgehog tenrec, echinops telfairi. (Ph.D. thesis). University of Hamburg, Hamburg.
Willis, C. K. R., Brigham, R. M., and Geiser, F. (2006). Deep, prolonged torpor by pregnant, free-ranging bats. Naturwissenschaften 93, 80–83. doi: 10.1007/s00114-005-0063-0
Willis, C. K. R., Turbill, C., and Geiser, F. (2005). Torpor and thermal energetics in a tiny Australian vespertilionid, the little forest bat (Vespadelus vulturnus). J. Comp. Physiol. B 175, 479–486. doi: 10.1007/s00360-005-0008-0
Withers, P., Louw, G., and Henschel, J. (1980). Energetics and water relations of Namib desert rodents. S. Afr. J. Zool. 15, 131–137. doi: 10.1080/02541858.1980.11447700
Keywords: hibernation, heterothermy, southern hemisphere, tropics, torpor
Citation: Nowack J, Levesque DL, Reher S and Dausmann KH (2020) Variable Climates Lead to Varying Phenotypes: “Weird” Mammalian Torpor and Lessons From Non-Holarctic Species. Front. Ecol. Evol. 8:60. doi: 10.3389/fevo.2020.00060
Received: 31 October 2019; Accepted: 26 February 2020;
Published: 31 March 2020.
Edited by:
Carlos Alonso Alvarez, National Museum of Natural Sciences (MNCN), SpainReviewed by:
Fritz Geiser, University of New England, AustraliaAndrew McKechnie, University of Pretoria, South Africa
Copyright © 2020 Nowack, Levesque, Reher and Dausmann. This is an open-access article distributed under the terms of the Creative Commons Attribution License (CC BY). The use, distribution or reproduction in other forums is permitted, provided the original author(s) and the copyright owner(s) are credited and that the original publication in this journal is cited, in accordance with accepted academic practice. No use, distribution or reproduction is permitted which does not comply with these terms.
*Correspondence: Julia Nowack, Si5Ob3dhY2smI3gwMDA0MDtsam11LmFjLnVr