- 1Department of Biological and Environmental Sciences, University of Gothenburg, Gothenburg, Sweden
- 2Gothenburg Global Biodiversity Centre, Gothenburg, Sweden
- 3CREAF, Cerdanyola del Vallès, Spain
- 4CSIC, Cerdanyola del Vallès, Spain
- 5Department of Genetics, Evolution, and Environment, Centre for Biodiversity and Environment Research, University College London, London, United Kingdom
Urbanization is a major driver of local biodiversity losses, but the traits that determine whether species are able to tolerate urban environments remain poorly understood. Theory suggests that a larger brain should provide higher tolerance to urbanization by enhancing behavioral flexibility to cope with novel challenges. However, assembling empirical evidence for a link between brain size and tolerance to urbanization has proven to be difficult, perhaps because the effect of the brain interacts with life history to influence persistence in urban environments. Here, we provide a global-scale assessment of the role of brain size on urban tolerance, combining quantitative estimations of urban tolerance with detailed information on brain size, life history and ecology for 629 avian species across 27 cities. Our analysis confirms the expected positive association between brain size and urban tolerance, but shows that the relationship is more complex than previously shown. While a large relative brain size generally increases urban tolerance, species with small brains can still attain high success in urban environments if they spread the risk of reproduction across multiple events (i.e., have a low brood value). These alternative strategies, although uncommon in natural conditions, seem to be favored in urban environments, fundamentally restructuring the composition of urban communities. Thus, our results support the notion that brain size mediates tolerance to urbanization, but also shows that there are alternative ways of exploiting urban environments. Our findings reconcile previous conflicting results regarding the effect of brain size on urban tolerance, and provide the basis for improved predictions of the responses of organisms to increasing urbanization over the coming decades.
Introduction
Cities are home to almost 4 billion people and over the coming decades their populations and geographic footprint will continue to swell (Seto et al., 2011, 2012). Urbanization represents one of the most extreme forms of environmental change for biodiversity (Sala, 2000; McKinney, 2006; Newbold et al., 2015) and for the majority of organisms, entails severe fitness costs, with declines in population abundances or local extinctions (Grimm et al., 2008). However, for species capable of exploiting these novel environments, cities provide a potential cornucopia of opportunities, allowing increases in abundance far beyond those found in natural habitats (Sol et al., 2014). These urban tolerant species constitute the slim fraction of biodiversity with which most people have frequent contact and thus have a potentially disproportionate impact on the health and well-being of human societies. Although a number of factors have been identified to predict urban tolerance (Sol et al., 2014), the features that allow these animals to thrive in urban environments remain insufficiently understood.
Among the different explanations for why some animals are able to exploit urban environments, one that has recently received greater attention is the cognitive buffer hypothesis (Allman et al., 1993; Sol, 2009). According to this hypothesis, a large brain should enhance persistence in novel environments by facilitating the construction of behavioral responses to new challenges, an idea supported by growing evidence (Sol et al., 2005, 2008; Sayol et al., 2016b; Fristoe et al., 2017). Behavioral responses have been found to be particularly important in coping with the challenges of urban environments, being instrumental in facilitating the exploitation of new resources, avoidance of human disturbances, and improving communication in noisy conditions (reviewed in Sol et al., 2013). However, whether large brain size predicts success in urban environments remains controversial (Table 1), with early support for this hypothesis (Møller, 2009; Maklakov et al., 2011, 2013), challenged by later studies (Evans et al., 2011; Sol et al., 2014; Dale et al., 2015; Møller and Erritzøe, 2015), only to be re-affirmed by more recent analysis (Callaghan et al., 2019).
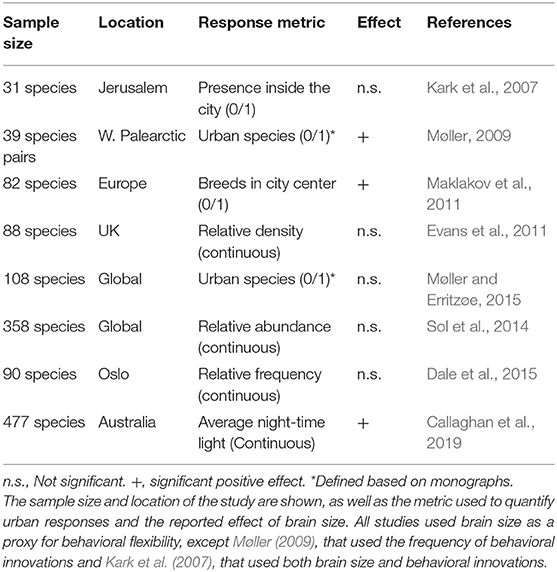
Table 1. Summary of studies that have investigated the association between behavioral flexibility and urban tolerance in birds, in chronological order.
One explanation for why the effect of brain size has been challenging to resolve, is that a large brain size is only one of a number of traits that may influence urban tolerance (Sol et al., 2014). For example, a broader niche is expected to facilitate persistence in urban environments by increasing the likelihood of finding appropriate resources (Evans et al., 2011; Sol et al., 2014; Ducatez et al., 2018; Callaghan et al., 2019) while migratory behavior can promote the colonization of urban areas (Evans et al., 2012). Because brain size correlates with these traits, failure to properly account for such additional drivers may mask the effect of brain size on urban tolerance. For example, if a large brain affects tolerance to urbanization by facilitating broader niches (Ducatez et al., 2015; Sol et al., 2016), including a measure of niche generalism in the model can block the effect of the brain on urban tolerance. Given that migratory species tend to have smaller relative brains than resident species (see Sayol et al., 2016b, and references therein), including migration in the model may also reduce the effect of the brain on urban tolerance. An additional, less appreciated issue, is that brain size may interact with species life history in potentially complex ways to determine urban tolerance. For instance, previous work has shown that avian species that distribute their reproductive effort across a higher number of events—and hence give less value to any single event—are more likely to establish themselves in novel environments, including urban settlements (Sol et al., 2012, 2014; Maspons et al., 2019). By having a life history that prioritizes future over present reproduction, these species can spread the risk of reproductive failure over several breeding attempts and, if conditions become unfavorable, may skip reproduction entirely, thus saving energy for future reproduction. However, a low brood value can be achieved through a longer lifespan, but also by reproducing several times in a same breeding season. For these latter species—which have high reproductive efforts with a low brood value—investing in a large brain and enhanced behavioral flexibility may bring more costs than benefits (Maspons et al., 2019). However, their life history can still buffer individuals against the risks associated with an urban life. Because it is likely that there is no single strategy to become an urban dweller, life history and brain size should be studied together in order to understand how they interact to influence urban tolerance.
Here, we revisit the role of brain size on urban tolerance by combining quantitative estimates of tolerance to urbanization with measurements of brain size, life history, and ecology for 629 avian species. Our approach consists of two stages. In the first stage, we estimate urban tolerance of species by comparing the relative abundance of species in urban vs. surrounding wild habitats, using data from well-characterized communities (Sol et al., 2017). We then conduct phylogenetic-based analyses to explore how relative brain size interacts with life history and ecological traits previously linked to urban tolerance. In the second stage, we conduct a community-level analysis to explore how trait-dependent responses to urbanization alter the structure of urban assemblages. Our global analysis supports the hypothesis that a large brain promotes urban tolerance, but also reveals that the relationship is more complex than generally thought, depending critically on the interaction with species life history.
Materials and Methods
Species Abundance Data
We used a previously compiled dataset on bird abundances in 27 cities for which intensive surveys are available (Sol et al., 2017). We restricted our analysis to studies where abundance was measured in both well-defined urban settlements as well as in the surrounding non-urbanized wild habitats. In total, this dataset contained 1,036 observations of species in different cities (38.4 ± 3.5 SE species per city). Following Sol et al. (2014), we calculated an urban tolerance index for each species as the differences in log-abundances between urban and wild habitats [i.e., log(Urban abundance)-log(Wild abundance)]. A positive urban tolerance index indicates that a species is more common in urban compared to wild habitats while a negative urban tolerance index indicates that a species is more common in wild habitats. We calculated the urban tolerance index for each species × city combination. The raw abundance data for a given city could be recorded as either the number of individuals per unit of survey area or time. This, however, does not affect the calculation of the urban tolerance index, because this metric was computed for each city relative to the matched wild habitat sampled using the same methodology.
Species Traits Data
For the species present in our dataset we collected data on brain size as well as a number of ecological and life-history traits that could affect urban tolerance. Although the trait values of a given species may vary between locations (e.g., urban vs. wild habitats) and cities, information on such intraspecific variation in traits is generally lacking. However, evidence indicated that variation across species was substantially higher than within species, and so we gathered information at the species level. We were able to collate published data on brain volume for 524 of the bird species present in our avian assemblage dataset (See Supplementary Data 2, Supplementary Table 1 to see source for each species). For 95% of these species, brain volume was estimated using the endocast method, which has been shown to give reliable estimates of brain size (Iwaniuk and Nelson, 2002). This method consists of filling an empty skull with lead shot or plastic microspheres, which are then weighed to infer the volume of the skull. For the remaining species (n = 34), brain size was measured as fresh brain weight and converted to volume using the density of fresh brain tissue (1.036 g/ml) (Iwaniuk and Nelson, 2002). We complemented these data with new skull measurements for 105 additional species from 51 families, measured at the Natural History Museum, Tring (UK), following the endocast method. Where possible, the values of measurements for male and female specimens were averaged to obtain an average brain size of each species (specimen measurements are available in Supplementary Data 3). In birds, the ability to construct novel behavioral responses is not related to brain size per se, but the extent to which the brain is either larger or smaller relative to body size (Lefebvre et al., 1997; Overington et al., 2009). We obtained body mass data from the same museum specimens, when available, complemented with estimates from the Handbook of the Birds of the World (Del Hoyo et al., 2018) and the Handbook of avian body masses (Dunning, 2007). We then estimated relative brain size as the residual from a log-log phylogenetic Generalized Least Square regression [using the “phyl.resid” function from R package phytools (Revell, 2012)] of absolute brain size against body mass. This relative brain size measure is strongly correlated with the sizes of pallial brain regions responsible for general-domain cognition, and hence is a good proxy for general behavioral flexibility (Lefebvre and Sol, 2008; Sayol et al., 2016a). As a measure of species life history, we used brood value, which measures the relative value of each reproductive event. To estimate brood value, we first collected information on the number of broods per year and maximum recorded lifespan (years) from various published sources (See Supplementary Table 1). With this information, we were able to obtain the potential total number of broods over the life of an individual, as the product of the maximum lifespan and the number of annual breeding attempts (broods). We then calculated the brood value as the logarithm of 1/total number of breeding attempts. Therefore, species that have fewer reproductive attempts over their lifetime, will have high brood values (i.e., each breeding attempt has a higher value), whereas species with many breeding attempts have low brood values (i.e., each attempt have a relatively smaller value). Although average life expectancy could be a more accurate metric to calculate the average number of breeding attempts per species, we used maximum lifespan instead because this metric has been recorded for a much larger sample of species. As a metric of niche breadth we used an index of habitat generalism based on the co-occurrence of species among different habitat types, which is available for all bird species (Ducatez et al., 2014). Global maps of species distributions (Birdlife International NatureServe, 2012) were used to identify migratory species as those that have some part of the population in different regions during reproductive and non-reproductive seasons. We also used these maps to classify the species in each city as exotic or native. In total, we obtained traits for 629 species, resulting in a final database consisting of 1,036 species per city records across 27 different cities (Figure 1, Supplementary Data 1, 2). When excluding all trait missing values (e.g., to run models with all factors at a time), our dataset contains 436 species and 816 observations.
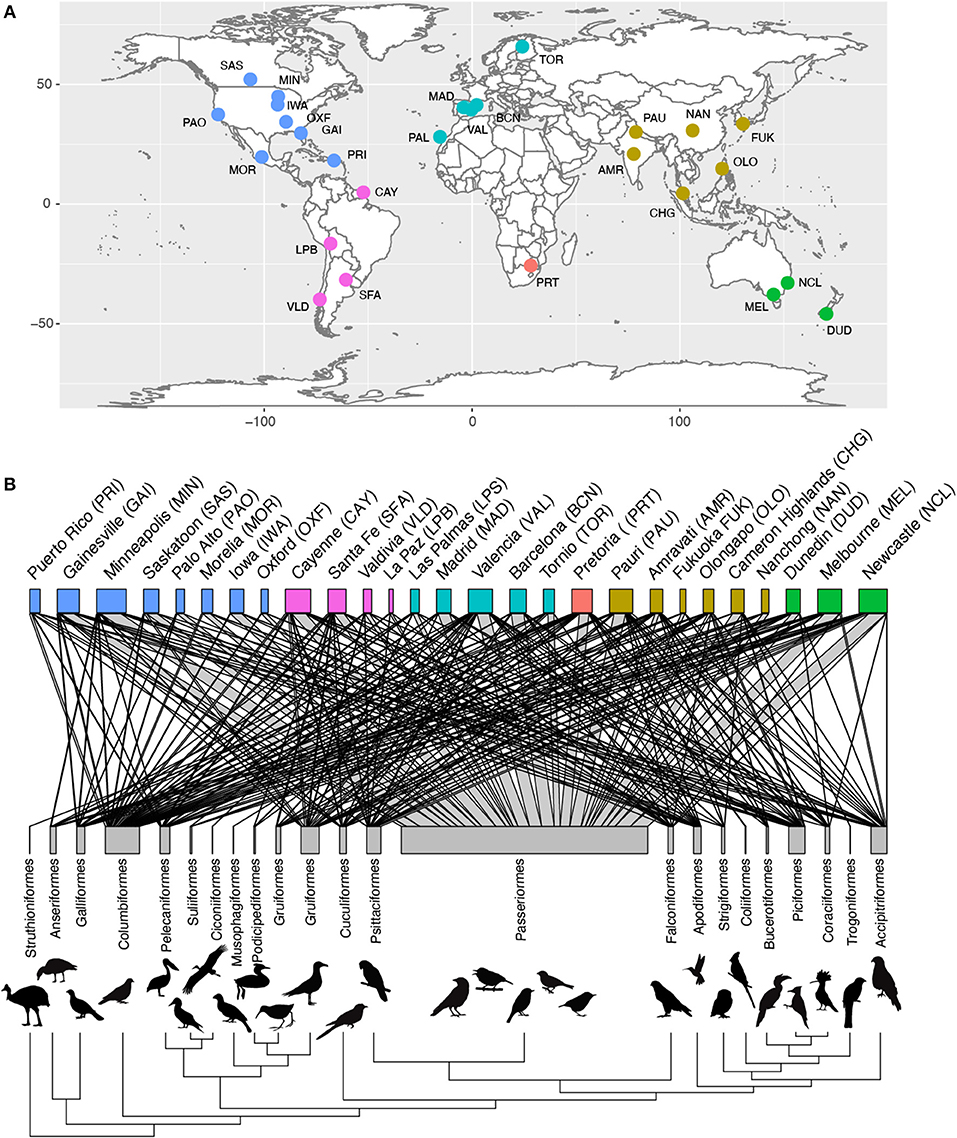
Figure 1. Geographical and taxonomic coverage of the data. Our study includes abundance data from 27 cities from all the continents (A). Abundance and brain size data was available for 629 species from 23 orders of birds including 1,036 unique city × species records. In (B) the distribution across cities of species from each taxonomic order are represented in a network, where the width of the links indicates the number of species from each order occurring in each city and the width of the bars indicates the total number of species in each city (top) and order (bottom). Silhouettes for each order are available at phylopic.org under a public domain license.
Modeling the Predictors of Urban Tolerance
All of our analyses were based on Bayesian Phylogenetic Mixed Models (BPMMs) combined with Markov chain Monte Carlo approximations, as implemented in the MCMCglmm R package v2.20 (Hadfield, 2010). Prior to any analysis, all continuous traits were Z-transformed (Mean centered to 0). We first explored the association between urban tolerance index and brain size by constructing a BPMM with the urban tolerance index as our response variable (Gaussian error distribution) and relative brain size as the unique predictor. To control for phylogenetic effects, we used a maximum clade credibility tree (MCC) from the posterior sample of 10,000 trees with the Ericsson tree backbone (Jetz et al., 2012). We note that using the Hackett tree backbone led to quantitatively almost identical results and so is not reported further. Phylogeny, species and city were included as random factors, and we used an inverse-Wishart prior (V = 1, ν = 0.002) to facilitate model convergence. We then ran additional models including several life-history and ecological variables as predictors of urban tolerance index. First, we ran models including relative brain size with either habitat breadth, migratory behavior and brood value to test for interactions between brain size and each of these traits. We ran three separate models with each variable in turn in order to maximize sample size. Finally, we ran a model including all variables as additive effects along with the significant interactions identified in previous models. Because the establishment success of introduced species—which are abundant in cities—is known to increase with brain size (Sol et al., 2005, 2008; Amiel et al., 2011), in this model, we also included whether the species was native or exotic to ensure that our results were not due to the effect of introduced species. We re-ran this final model after excluding species for which brain size was measured as fresh brain weight to check that the use of different methods did not affect our conclusions. Models were run for 1010,000 iterations, with a burn-in of 10,000 and a thinning interval of 1,000, resulting in a posterior distribution of 1,000 samples. We checked that the autocorrelation of samples was <0.1 and ran each model twice, assessing proper convergence using the Gelman-Rubin statistic, requiring models to have a scaling reduction factor below 1.1 (Gelman and Rubin, 1992). We report the posterior mean and the 95% credible intervals (CIs) for each variable, and assess significance according to pMCMC, which is the proportion of samples in the posterior distribution non-overlapping with zero. Because preliminary results suggested that species with different combinations of brain sizes and brood values have contrasting tolerances to urbanization, we examined how these two traits are related two each other at the species level. With this objective, we ran an additional BPMM with relative brain size as the response variable (Gaussian error distribution) and brood value as a predictor. We used identical prior and sampling options as for models of urban tolerance index, but in this case only phylogenetic structure was included as a random factor (the analysis is done at the species level, with a single value per species).
Exploring Brain Size Distributions in Urban and Wild Assemblages
Because brain size and life history were found to interact to predict urban tolerance, we performed additional analysis to further examine how the distribution of brain size changes between wild and urban assemblages and according to different life-history strategies. We calculated the mean urban and wild abundance of each species across all cities globally and classified each species as having either a high or low brood value, defined using the median brood value as the breakpoint (See resulting species averages in Supplementary Data 4). Then, we calculated the weighted mean and weighted standard deviation of brain size for both the global urban and global wild assemblage for each brood value group. To test for statistical differences in brain size between brood value groups we performed two-sample Weighted T-Tests (i.e., Welch tests) for each comparison, without significance-level correction for multiple testing. In addition, we also examined the relative extent to which differences in the weighted-mean brain size of wild and urban bird assemblages are driven by changes in species composition (loss or gain of species from wild habitats to cities) or changes in relative abundance of species that are found both in and outside cities (Supplementary Figure 1). To illustrate the types of species that are favored in urban communities, and how the brain size distribution is altered compared to natural assemblages, we ordered all bird families according to their mean tolerance to urbanization (using the mean urban tolerance index across their species). Then, we separately plotted the brain size distribution of urban exploiters (species from the 10 families with the highest tolerance) and avoiders (species from the 10 families with the lowest tolerance).
Results
We found that relative brain size is positively related to urban tolerance (Posterior mean or ß, with 95% confidence intervals = 0.450 [0.115–0.818], pMCMC = 0.012), when included as a single predictor. In addition, we found that brood value and habitat breadth —but not migratory behavior— were also associated with urban tolerance when added to the previous model (See Supplementary Tables 2–4 for more details). In particular, we found that a lower brood value (ß = −0.382 [−0.595 to −0.112], pMCMC < 0.001) and a broader habitat breadth (ß = 0.29808 [0.106–0.473], pMCMC = 0.002) were associated with increased tolerance to urbanization.
In the model including brood value, the main effect of relative brain size was no longer a significant predictor of urban tolerance. This in part reflects the existence of a weak negative association between brain size and brood value, so that species with relatively large brains were generally associated with low brood values (ß = −0.083 [−0.129 to −0.033], pMCMC < 0.001). However, there was a significant interaction between brood value and relative brain size (ß brain size*brood value = 0.272 [0.028–0.530], pMCMC = 0.028; Supplementary Table 2): Tolerance to urbanization was higher for species with relatively larger brains and low brood values, but the relationship between urban tolerance and brain size changed in species with high brood values (Figure 2). Thus, species with a high brood value (i.e., concentrating most reproductive effort in few events) have lower abundance in urban habitats when they have relatively small brains but have higher abundance when they have relatively larger brains.
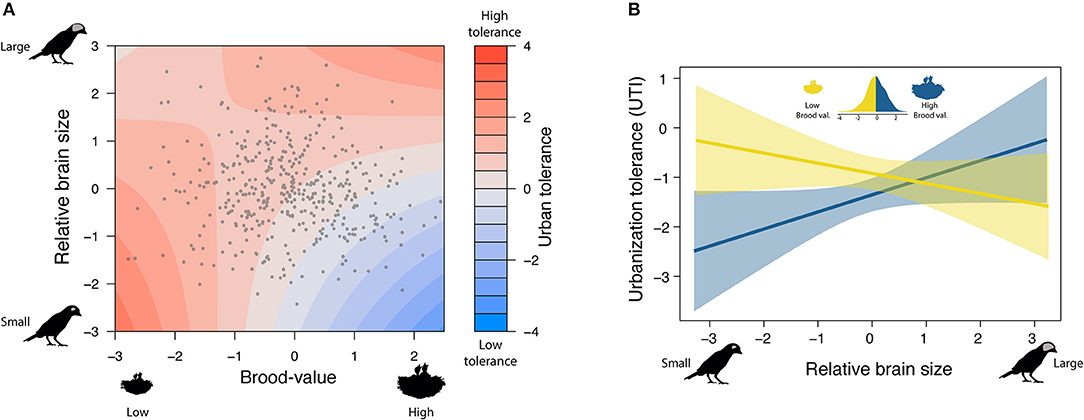
Figure 2. Brain size interacts with brood value to predict urban tolerance. (A) Either species with lower brood values and small brains (bottom-left) or species with high brood values and big brains (up-right) are able to tolerate urban environments. (B) Splitting species into those investing in few breeding attempts (High brood value) or many attempts (Low brood value), helps to visualize the interaction: Brain size increases urban tolerance in species with high brood value but not in species with low brood value.
The interaction between relative brain size and brood value was not due to the frequent presence of exotic species in cities (ß = 0.293 [0.044–0.542], pMCMC =0.024, Table 2, Supplementary Table 5). Similarly, the interaction between brain size and brood value was still significant when including the other ecological predictors (e.g., habitat breadth and migratory behavior) in the model (Table 2, Supplementary Table 5).
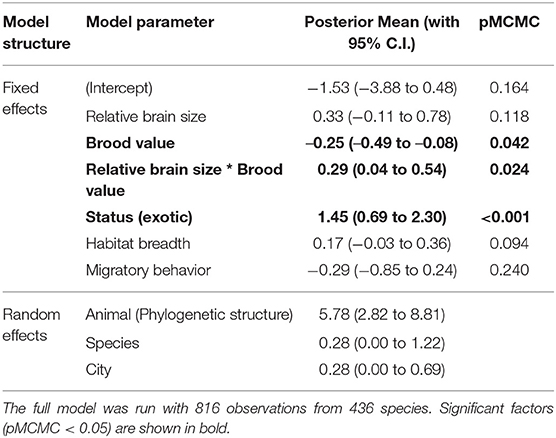
Table 2. The effects of each predictor (Posterior mean with 95% credible interval) on the urban tolerance index, from a Phylogenetic Bayesian mixed model which includes the phylogenetic structure, species identity and city as random effects.
The previous analysis suggests that a species may become an urban dweller with different combinations of brain size and life history. However, what are the consequences for urban communities? We found that while the average brain size (mean weighted by abundance) of wild and urban communities were largely overlapping when all species were considered together (Wild community: −0.07 ± 0.71 SE; Urban community: −0.25 ± 0.85 SE), a clear shift in assemblage structure was evident when species with low and high brood values were examined separately. For wild habitats, the community-weighted average brain size was similar for both low and high brood-value strategies, whereas in urban environments, communities shift toward big-brained species with few breeding attempts over their lifetime and small-brained species with a high number of reproductive events (Figure 3). These shifts were primarily driven by the increase in abundance in cities of species with larger brains and high brood value or small brains and low brood value, but also to a lesser extent by the decrease in abundance or local extinction of species with small brains and high brood values (Supplementary Figure 1).
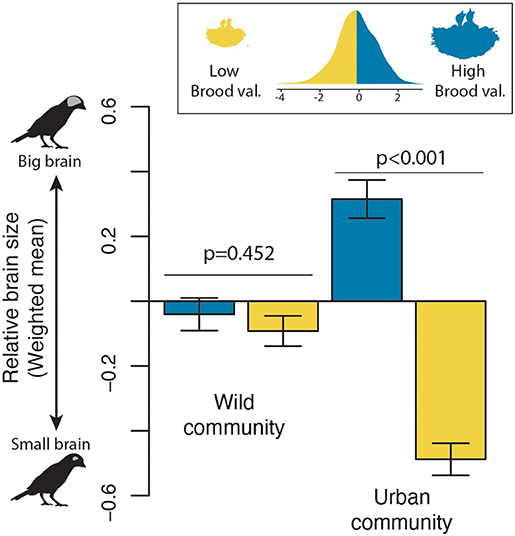
Figure 3. Changes in the relative brain size of wild and urban bird communities. The community-weighted mean relative brain size of wild and urban communities is shown for both high (blue) and low (yellow) brood-value strategies, using the median of the brood value distribution as the breakpoint. P-values indicate the significance level from two-sample Weighted T-Tests (i.e., Welch tests).
Discussion
Using a global dataset combining brain size and urbanization measures across cities, we show that relative brain size is an important predictor of species tolerance to urban habitats. However, our analysis shows that the direction of the effect of brain size is dependent on species life history, resulting in two alternative strategies for thriving in cities. In particular, species that invest in a high number of breeding attempts over their life (i.e., have a low brood value) are more tolerant of urbanization, even when having a small brain size. In contrast, for species that invest in few reproductive events, a brain that is larger than expected by their body size is key to provide urban tolerance. Although relatively uncommon in nature, these trait combinations seem to be favored in urban environments, leading to a striking restructuring of avian assemblages in urban environments. These findings help reconcile previous conflicting results regarding the effect of brain size on urban tolerance and resolve the long-standing conundrum that urban exploiters (i.e., species that thrive in cities) include examples of both small brained (e.g., pigeons and swifts) and large-brained (e.g., crows, gulls, and starlings) species (Figure 4). The existence of multiple strategies to tolerate urban environments may also explain why recent work on mammals (Santini et al., 2019) found that a larger brain size promotes tolerance to urban habitat in some groups (e.g., carnivores and bats) but not in others (e.g., ungulates).
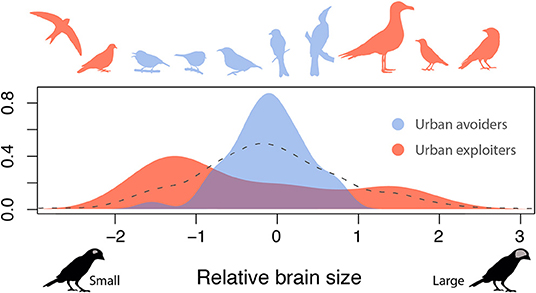
Figure 4. Relative brain size distribution among urban exploiters and avoiders. Shown is the distribution of relative brain size across species from the ten families with the highest (urban exploiters) and ten families with lowest (urban avoiders) urban tolerance (see Supplementary Figure 2 for results with additional families). Silhouettes provide examples of some of the families, ordered by brain size, from left to right: Apodidae, Columbidaee, Zosteropidae, Reguliidae, Coerebidae, Dicruridae, Meliphagidae, Laridae, Passeridae, Corvidae (available at phylopic.org under a public domain license). Gray dashed line shows the distribution of relative brain size for all the species contained in the dataset.
In our study, we found that larger brains are generally correlated (albeit weakly) with low brood values. However, we found that the few species that depart from this relationship are disproportionally represented in cities, revealing two trait combinations to tolerate urban environments: larger brains with high brood values and small brains with low brood values. These two alternative strategies could be seen as a choice between investing either in enhanced behavioral plasticity or in multiple reproductive events. There is indeed increasing evidence, both empirical (Sol et al., 2012, 2014) and theoretical (Maspons et al., 2019) that spreading the reproductive effort across many events can enhance the establishment success of populations in novel environments. Under these conditions, having multiple breeding opportunities can allow individuals to spread the risk through bet-hedging (Stearns, 2000) or to skip reproduction in favor of future events (Forcada et al., 2008), diminishing the costs of reproductive failure. In this case, having a large brain may entail net fitness costs due to the greater energy requirements and time constrains of spreading annual reproduction in several events (Sol et al., 2016).
In contrast, for species with fewer reproductive opportunities (i.e., with a high brood value for each attempt), dealing with urbanization pressures requires having a more plastic behavior, allowing them to respond to altered conditions (Lowry et al., 2013; Sol et al., 2013). There is ample evidence that urban bird populations tend to have greater innovation and problem-solving abilities (Liker and Bokony, 2009; Sol et al., 2011; Audet et al., 2016; Cook et al., 2017; Griffin et al., 2017; Kozlovsky et al., 2017)—traits that are closely linked to a relatively larger brain (Lefebvre et al., 1997; Overington et al., 2009; Benson-Amram et al., 2016)—and that this could potentially help animals to deal with novel challenges (Sol et al., 2007; Sol, 2009). These ideas are consistent with evidence that large-brained species tend to live in more variable environments (Sayol et al., 2016b, 2018; Vincze, 2016; Fristoe et al., 2017), and are more likely to establish when introduced to novel environments (Sol et al., 2005, 2008; Amiel et al., 2011). Although the exact mechanism linking brain size and urban success is not known, increased behavioral plasticity is likely useful in a variety of domains, including feeding innovations (Ducatez et al., 2015), recognizition of novel predation threats (Levey et al., 2009) and choice of the appropriate habitat (Clergeau and Quenot, 2007).
Our work reaffirms the importance of brain size in determining species responses to changing environments (Sol et al., 2005; Sayol et al., 2016b; Fristoe et al., 2017), but also highlights the need to consider behavioral flexibility in the context of life history (Sol et al., 2016; Fristoe and Botero, 2019; Maspons et al., 2019). These two factors must therefore be studied together in order to fully understand how organisms respond to current anthropogenic impacts. In this context, an important avenue for further work will be to explore how the interaction between brain size and life history affects the response of organisms to other kinds of anthropogenic threats such as habitat alterations (Shultz et al., 2005) and the potential consequences for global extinction risk (Gonzalez-Voyer et al., 2016; Tobias and Pigot, 2019). In the face of increasing urbanization, our findings can be used to predict those species that will better respond to the coming challenges as well-identify those species of greater sensitivity where conservation efforts should be concentrated.
Data Availability Statement
All datasets generated for this study are included in the article/Supplementary Material.
Author Contributions
FS and DS conceived the study and collected the data on species traits and species abundances, respectively. FS and AP analyzed the data. All authors equally contributed to write the paper and approved the final version.
Funding
This work was possible thanks to a career development bursary to FS from the British Ornithologists' Union, funding from the Ministry of science, innovation and universities—Spanish government (CGL2017-90033-P) to DS and a Royal Society University Fellowship to AP.
Conflict of Interest
The authors declare that the research was conducted in the absence of any commercial or financial relationships that could be construed as a potential conflict of interest.
Acknowledgments
We wish to thank Blandine F. Doligez and Laure Cauchard who invited us to contribute to the Special Issue that contains this work. We are also grateful to Judith White and all the other staff that helped us navigate through the bird skeleton collection of the Natural History Museum at Tring.
Supplementary Material
The Supplementary Material for this article can be found online at: https://www.frontiersin.org/articles/10.3389/fevo.2020.00058/full#supplementary-material
References
Allman, J., McLaughlin, T., and Hakeem, A. (1993). Brain weight and life-span in primate species. Proc. Natl. Acad. Sci. U.S.A. 90, 118–122. doi: 10.1073/pnas.90.1.118
Amiel, J. J., Tingley, R., and Shine, R. (2011). Smart moves: effects of relative brain size on establishment success of invasive amphibians and reptiles. PLoS ONE 6:e18277. doi: 10.1371/journal.pone.0018277
Audet, J.-N., Ducatez, S., and Lefebvre, L. (2016). The town bird and the country bird: problem solving and immunocompetence vary with urbanization. Behav. Ecol. 27, 637–644. doi: 10.1093/beheco/arv201
Benson-Amram, S., Dantzer, B., Stricker, G., Swanson, E. M., and Holekamp, K. E. (2016). Brain size predicts problem-solving ability in mammalian carnivores. Proc. Natl. Acad. Sci. U.S.A. 113, 2532–2537. doi: 10.1073/pnas.1505913113
Birdlife International NatureServe (2012). Bird Species Distribution Maps of the World. Cambridge; Arlington, TX: NatureServe, BirdLife International. Available online at: http://www.birdlife.org/datazone/info/spcdownload (accessed June 6, 2019).
Callaghan, C. T., Major, R. E., Wilshire, J. H., Martin, J. M., Kingsford, R. T., and Cornwell, W. K. (2019). Generalists are the most urban-tolerant of birds: a phylogenetically controlled analysis of ecological and life history traits using a novel continuous measure of bird responses to urbanization. Oikos 128, 845–858. doi: 10.1111/oik.06158
Clergeau, P., and Quenot, F. (2007). Roost selection flexibility of European starlings aids invasion of urban landscape. Landsc. Urban Plan. 80, 56–62. doi: 10.1016/j.landurbplan.2006.06.002
Cook, M. O., Weaver, M. J., Hutton, P., and McGraw, K. J. (2017). The effects of urbanization and human disturbance on problem solving in juvenile house finches (Haemorhous mexicanus). Behav. Ecol. Sociobiol. 71:85. doi: 10.1007/s00265-017-2304-6
Dale, S., Lifjeld, J. T., and Rowe, M. (2015). Commonness and ecology, but not bigger brains, predict urban living in birds. BMC Ecol. 15:12. doi: 10.1186/s12898-015-0044-x
Del Hoyo, J., Elliott, A., Sargatal, J., Christie, D., and de Juana, E. (2018). Handbook of the Birds of the World Alive. Barcelona: Lynx Edicions.
Ducatez, S., Clavel, J., and Lefebvre, L. (2015). Ecological generalism and behavioural innovation in birds: technical intelligence or the simple incorporation of new foods? J. Anim. Ecol. 84, 79–89. doi: 10.1111/1365-2656.12255
Ducatez, S., Sayol, F., Sol, D., and Lefebvre, L. (2018). Are urban vertebrates city specialists, artificial habitat exploiters, or environmental generalists? Integr. Comp. Biol. 58, 929–938. doi: 10.1093/icb/icy101
Ducatez, S., Tingley, R., and Shine, R. (2014). Using species co-occurrence patterns to quantify relative habitat breadth in terrestrial vertebrates. Ecosphere 5, 1–12. doi: 10.1890/ES14-00332.1
Dunning, J. B. Jr. (2007). CRC Handbook of Avian Body Masses. Second Edition, Boca Raton, FL: CRC Press. doi: 10.1201/9781420064452
Evans, K. L., Chamberlain, D. E., Hatchwell, B. J., Gregory, R. D., and Gaston, K. J. (2011). What makes an urban bird? Glob. Change Biol. 17, 32–44. doi: 10.1111/j.1365-2486.2010.02247.x
Evans, K. L., Newton, J., Gaston, K. J., Sharp, S. P., McGowan, A., and Hatchwell, B. J. (2012). Colonisation of urban environments is associated with reduced migratory behaviour, facilitating divergence from ancestral populations. Oikos 121, 634–640. doi: 10.1111/j.1600-0706.2011.19722.x
Forcada, J., Trathan, P. N., and Murphy, E. J. (2008). Life history buffering in Antarctic mammals and birds against changing patterns of climate and environmental variation. Glob. Change Biol. 14, 2473–2488. doi: 10.1111/j.1365-2486.2008.01678.x
Fristoe, T. S., and Botero, C. A. (2019). Alternative ecological strategies lead to avian brain size bimodality in variable habitats. Nat. Commun. 10:3818. doi: 10.1038/s41467-019-11757-x
Fristoe, T. S., Iwaniuk, A. N., and Botero, C. A. (2017). Big brains stabilize populations and facilitate colonization of variable habitats in birds. Nat. Ecol. Evol. 1, 1706–1715. doi: 10.1038/s41559-017-0316-2
Gelman, A., and Rubin, D. B. (1992). Inference from iterative simulation using multiple sequences. Stat. Sci. 7, 457–472. doi: 10.1214/ss/1177011136
Gonzalez-Voyer, A., González-Suárez, M., Vilà, C., and Revilla, E. (2016). Larger brain size indirectly increases vulnerability to extinction in mammals. Evolution 70, 1364–1375. doi: 10.1111/evo.12943
Griffin, A. S., Netto, K., and Peneaux, C. (2017). Neophilia, innovation and learning in an urbanized world: a critical evaluation of mixed findings. Curr. Opin. Behav. Sci. 16, 15–22. doi: 10.1016/j.cobeha.2017.01.004
Grimm, N. B., Faeth, S. H., Golubiewski, N. E., Redman, C. L., Wu, J., Bai, X., et al. (2008). Global change and the ecology of cities. Science 319, 756–760. doi: 10.1126/science.1150195
Hadfield, J. D. (2010). MCMC methods for multi-response generalized linear mixed models: the MCMCglmm R package. J. Stat. Softw. 33, 1–22. doi: 10.18637/jss.v033.i02
Iwaniuk, A. N., and Nelson, J. E. (2002). Can endocranial volume be used as an estimate of brain size in birds? Can. J. Zool. 80, 16–23. doi: 10.1139/z01-204
Jetz, W., Thomas, G. H., Joy, J. B., Hartmann, K., and Mooers, A. O. (2012). The global diversity of birds in space and time. Nature 491, 444–448. doi: 10.1038/nature11631
Kark, S., Iwaniuk, A., Schalimtzek, A., and Banker, E. (2007). Living in the city: can anyone become an “urban exploiter”? J. Biogeogr. 34, 638–651. doi: 10.1111/j.1365-2699.2006.01638.x
Kozlovsky, D. Y., Weissgerber, E. A., and Pravosudov, V. V. (2017). What makes specialized food-caching mountain chickadees successful city slickers? Proc. Roy. Soc. B Biol. Sci. 284:20162613. doi: 10.1098/rspb.2016.2613
Lefebvre, L., and Sol, D. (2008). Brains, lifestyles and cognition: are there general trends? Brain Behav. Evol. 72, 135–144. doi: 10.1159/000151473
Lefebvre, L., Whittle, P., and Lascaris, E. (1997). Feeding innovations and forebrain size in birds. Anim. Behav. 53, 549–560. doi: 10.1006/anbe.1996.0330
Levey, D. J., Londono, G. A., Ungvari-Martin, J., Hiersoux, M. R., Jankowski, J. E., Poulsen, J. R., et al. (2009). Urban mockingbirds quickly learn to identify individual humans. Proc. Natl. Acad. Sci. U.S.A. 106, 8959–8962. doi: 10.1073/pnas.0811422106
Liker, A., and Bokony, V. (2009). Larger groups are more successful in innovative problem solving in house sparrows. Proc. Natl. Acad. Sci. U.S.A. 106, 7893–7898. doi: 10.1073/pnas.0900042106
Lowry, H., Lill, A., and Wong, B. B. M. (2013). Behavioural responses of wildlife to urban environments: behavioural responses to urban environments. Biol. Rev. 88, 537–549. doi: 10.1111/brv.12012
Maklakov, A. A., Immler, S., Gonzalez-Voyer, A., Ronn, J., and Kolm, N. (2011). Brains and the city: big-brained passerine birds succeed in urban environments. Biol. Lett. 7, 730–732. doi: 10.1098/rsbl.2011.0341
Maklakov, A. A., Immler, S., Gonzalez-Voyer, A., Ronn, J., and Kolm, N. (2013). Brains and the city in passerine birds: re-analysis and confirmation of the original result. Biol. Lett. 9:20130859. doi: 10.1098/rsbl.2013.0859
Maspons, J., Molowny-Horas, R., and Sol, D. (2019). Behaviour, life history and persistence in novel environments. Philos. Trans. Roy. Soc. B Biol. Sci. 374:20180056. doi: 10.1098/rstb.2018.0056
McKinney, M. L. (2006). Urbanization as a major cause of biotic homogenization. Biol. Conserv. 127, 247–260. doi: 10.1016/j.biocon.2005.09.005
Møller, A. P. (2009). Successful city dwellers: a comparative study of the ecological characteristics of urban birds in the Western Palearctic. Oecologia 159, 849–858. doi: 10.1007/s00442-008-1259-8
Møller, A. P., and Erritzøe, J. (2015). Brain size and urbanization in birds. Avian Res. 6:8. doi: 10.1186/s40657-015-0017-y
Newbold, T., Hudson, L. N., Hill, S. L. L., Contu, S., Lysenko, I., Senior, R. A., et al. (2015). Global effects of land use on local terrestrial biodiversity. Nature 520, 45–50. doi: 10.1038/nature14324
Overington, S. E., Morand-Ferron, J., Boogert, N. J., and Lefebvre, L. (2009). Technical innovations drive the relationship between innovativeness and residual brain size in birds. Anim. Behav. 78, 1001–1010. doi: 10.1016/j.anbehav.2009.06.033
Revell, L. J. (2012). Phytools: an R package for phylogenetic comparative biology (and other things). Methods Ecol. Evol. 3, 217–223. doi: 10.1111/j.2041-210X.2011.00169.x
Sala, O. E. (2000). Global biodiversity scenarios for the year 2100. Science 287, 1770–1774. doi: 10.1126/science.287.5459.1770
Santini, L., González-Suárez, M., Russo, D., Gonzalez-Voyer, A., von Hardenberg, A., and Ancillotto, L. (2019). One strategy does not fit all: determinants of urban adaptation in mammals. Ecol. Lett. 22, 365–376. doi: 10.1111/ele.13199
Sayol, F., Downing, P. A., Iwaniuk, A. N., Maspons, J., and Sol, D. (2018). Predictable evolution towards larger brains in birds colonizing oceanic islands. Nat. Commun. 9:2820. doi: 10.1038/s41467-018-05280-8
Sayol, F., Lefebvre, L., and Sol, D. (2016a). Relative brain size and its relation with the associative pallium in birds. Brain Behav. Evol. 87, 69–77. doi: 10.1159/000444670
Sayol, F., Maspons, J., Lapiedra, O., Iwaniuk, A. N., Székely, T., and Sol, D. (2016b). Environmental variation and the evolution of large brains in birds. Nat. Commun. 7:13971. doi: 10.1038/ncomms13971
Seto, K. C., Fragkias, M., Güneralp, B., and Reilly, M. K. (2011). A meta-analysis of global urban land expansion. PLoS ONE 6:e23777. doi: 10.1371/journal.pone.0023777
Seto, K. C., Guneralp, B., and Hutyra, L. R. (2012). Global forecasts of urban expansion to 2030 and direct impacts on biodiversity and carbon pools. Proc. Natl. Acad. Sci. U.S.A. 109, 16083–16088. doi: 10.1073/pnas.1211658109
Shultz, S., Bradbury, R. B., Evans, K. L., Gregory, R. D., and Blackburn, T. M. (2005). Brain size and resource specialization predict long-term population trends in British birds. Proc. Roy. Soc. B Biol. Sci. 272, 2305–2311. doi: 10.1098/rspb.2005.3250
Sol, D. (2009). Revisiting the cognitive buffer hypothesis for the evolution of large brains. Biol. Lett. 5, 130–133. doi: 10.1098/rsbl.2008.0621
Sol, D., Bacher, S., Reader, S. M., and Lefebvre, L. (2008). Brain size predicts the success of mammal species introduced into novel environments. Am. Nat. 172, S63–S71. doi: 10.1086/588304
Sol, D., Bartomeus, I., González-Lagos, C., and Pavoine, S. (2017). Urbanisation and the loss of phylogenetic diversity in birds. Ecol. Lett. 20, 721–729. doi: 10.1111/ele.12769
Sol, D., Duncan, R. P., Blackburn, T. M., Cassey, P., and Lefebvre, L. (2005). Big brains, enhanced cognition, and response of birds to novel environments. Proc. Natl. Acad. Sci. U.S.A. 102, 5460–5465. doi: 10.1073/pnas.0408145102
Sol, D., González-Lagos, C., Moreira, D., Maspons, J., and Lapiedra, O. (2014). Urbanisation tolerance and the loss of avian diversity. Ecol. Lett. 17, 942–950. doi: 10.1111/ele.12297
Sol, D., Griffin, A. S., Bartomeus, I., and Boyce, H. (2011). Exploring or avoiding novel food resources? The novelty conflict in an invasive bird. PLoS ONE 6:e19535. doi: 10.1371/journal.pone.0019535
Sol, D., Lapiedra, O., and González-Lagos, C. (2013). Behavioural adjustments for a life in the city. Anim. Behav. 85, 1101–1112. doi: 10.1016/j.anbehav.2013.01.023
Sol, D., Maspons, J., Vall-llosera, M., Bartomeus, I., Garcia-Pena, G. E., Pinol, J., et al. (2012). Unraveling the life history of successful invaders. Science 337, 580–583. doi: 10.1126/science.1221523
Sol, D., Sayol, F., Ducatez, S., and Lefebvre, L. (2016). The life-history basis of behavioural innovations. Philos. Trans. Roy. Soc. B Biol. Sci. 371:20150187. doi: 10.1098/rstb.2015.0187
Sol, D., Szekely, T., Liker, A., and Lefebvre, L. (2007). Big-brained birds survive better in nature. Proc. Roy. Soc. B: Biol. Sci. 274, 763–769. doi: 10.1098/rspb.2006.3765
Stearns, S. C. (2000). Life history evolution: successes, limitations, and prospects. Naturwissenschaften 87, 476–486. doi: 10.1007/s001140050763
Tobias, J. A., and Pigot, A. L. (2019). Integrating behaviour and ecology into global biodiversity conservation strategies. Philos. Trans. Roy. Soci. B 374:20190012. doi: 10.1098/rstb.2019.0012
Keywords: urban ecology, anthropogenic changes, avian communities, brain mass, brood value, biodiversity, urban exploiters, urban avoiders
Citation: Sayol F, Sol D and Pigot AL (2020) Brain Size and Life History Interact to Predict Urban Tolerance in Birds. Front. Ecol. Evol. 8:58. doi: 10.3389/fevo.2020.00058
Received: 23 September 2019; Accepted: 26 February 2020;
Published: 25 March 2020.
Edited by:
Blandine Françoise Doligez, Centre National de la Recherche Scientifique (CNRS), FranceReviewed by:
Trevor Fristoe, University of Konstanz, GermanyVeronika Bókony, Hungarian Academy of Sciences (MTA), Hungary
Copyright © 2020 Sayol, Sol and Pigot. This is an open-access article distributed under the terms of the Creative Commons Attribution License (CC BY). The use, distribution or reproduction in other forums is permitted, provided the original author(s) and the copyright owner(s) are credited and that the original publication in this journal is cited, in accordance with accepted academic practice. No use, distribution or reproduction is permitted which does not comply with these terms.
*Correspondence: Ferran Sayol, ferran.sayol@bioenv.gu.se