- Department of Entomology and Plant Pathology, North Carolina State University, Raleigh, NC, United States
Lower termites, as well as their sister group, the subsocial wood-feeding cockroach Cryptocercus, rely on flagellated eukaryotic symbionts in the hindgut to cooperatively digest their wood diet. In Cryptocercus these flagellates undergo encystment cycles tightly coordinated with the molting cycle of their host, yet the resultant cysts play no demonstrated role in their transmission to neonates; the trophozoite stage of the flagellates is passed directly from parents to offspring via hindgut fluids (proctodeal trophallaxis). This pattern suggests that encystment is a vestige from a gregarious cockroach ancestor, when the flagellates had a functional, two-stage life cycle and the cysts were horizontally transmitted among hosts via coprophagy. The strong integration between flagellate encystment cycles and host developmental physiology in Cryptocercus indicates that the relationship of the flagellates with their proposed gregarious cockroach ancestor was not commensal, but parasitic, with flagellates likely obtaining benefits by taking advantage of host gut metabolites and ingested plant debris. When vertical transmission evolved the parasites were ‘captured,’ and their fitness became inescapably embedded in the fitness of their host. The vertical transmission of gut flagellates and the origin of host subsociality via proctodeal trophallaxis can be considered two sides of the same coin. From the host point of view proctodeal trophallaxis marks the origination of parental care by provisioning neonates with nourishment, metabolites and beneficial microbiota. From the flagellate point of view, proctodeal trophallaxis was a shift from horizontal to vertical transmission, pushing them from the parasitic to the mutualistic end of the symbiotic spectrum, arguably making this host behavioral change the most critical juncture in the evolutionary trajectory of the termite lineage.
“… given the complexity of host-microbe interactions, humility and nuance are probably wise stances when surveying the topic.”
–Casadevall and Pirofski, 2019
Introduction
Some of the best studied insect gut communities are those in termites (Robinson et al., 2010), yet as in most symbiotic systems (Huitzil et al., 2018), mechanisms explaining the role of microbiota in the social evolution of the host are poorly understood. The historic literature is dominated by the role of the flagellated protists in the termite gut and their influence on termite social behavior. These protists die prior to the molt of their termite hosts (reviewed by Nalepa, 2017) and must be regained by feeding on the hindgut fluids of a donor nestmate, a behavior called proctodeal trophallaxis (McMahan, 1969). This mandatory interdependence between hosts led to the proposal that termites originated as feeding communities bound by the necessity of exchanging flagellates and only later evolved social care of the brood (Cleveland, 1926; Cleveland et al., 1934; Lin and Michener, 1972; Wilson, 1975). The bulk of evidence, however, indicates that protozoan death at host molt in termites did not precede eusociality. It was a downstream effect of the initial stages of the eusocial condition (alloparental care) and associated with the physiology of developmental arrest and caste control in the hosts (Nalepa, 1994, 2017). Nonetheless, the death of protists at host molt was an evolutionary tipping point, in that beyond that threshold, intrinsic processes in the system drove accelerating change (Nalepa, 2015).
Here I review evidence that a pivotal change in the host-flagellate relationship occurred very early in their shared evolutionary history. Specifically, I examine how the shift from horizontal transmission of protists in a distant gregarious ancestor (as typified by extant gregarious cockroaches), to vertical transmission in the immediate subsocial ancestor of termites (as typified by the wood-feeding cockroach Cryptocercus, sister group to termites) set the stage not only for the evolution of eusociality in descendants, but also for their ecological domination. A caveat is that reliance on conjectured historical associations makes the illumination of processes that led to current patterns challenging.
The term symbiosis used here is the currently accepted one: a symbiont lives in or on a living host, implying neither physiological dependence nor benefit or harm between the organisms involved; it is an interaction between species. Further categorization of symbiosis relates to the cost or benefits to each partner. In commensalism the smaller partner benefits from the relationship, with no fitness effect on the host. There are fitness gains for both partners in mutualism, and a parasite benefits by exploiting but not directly killing its host. The term symbiosis is not synonymous with mutualism (Thompson, 1994; Corliss, 2002; Goater et al., 2014; Tipton et al., 2019).
The Hindgut Microbiome
The hindgut microbiome in Cryptocercus and lower termites is a diverse assemblage of bacteria, archaea and viruses, as well as two groups of protists from the “Excavata,” a deep branching supergroup of Eukarya. Most protists are parabasalids, in the phylum Parabasalia, and oxymonads, in the Class Oxymonadea, Phylum Preaxostyla. Members of these two groups are of unclear phylogenetic origin and unique to the termite lineage (Brune, 2011; Ohkuma and Brune, 2011). The bacterial members of the hindgut community are affiliated with more than 15 phyla (Hongoh et al., 2005; Ohkuma, 2008; Dietrich et al., 2014), and many of those in Cryptocercus/lower termites are endo- or ectosymbionts, associated with the surface, cytoplasm or nucleus of the flagellates (Brune and Stingl, 2005; Noda et al., 2006, 2009, 2018; Ikeda-Ohtsubo and Brune, 2009; Sato et al., 2014; Mikaelyan et al., 2017b; Hongoh and Ohkuma, 2018).
Although the physiology of host-microbial interactions in the lineage is far from clear, it is unchallenged dogma (Bignell, 2011) that neither Cryptocercus nor termites can exist without their microbial partners (Cleveland et al., 1934; Breznak and Brune, 1994). The hosts, however, are not completely dependent on flagellates for lignocellulose digestion (Slaytor, 1992). All studied cockroach and termite species have endogenous cellulase genes (Genta et al., 2003; Lo et al., 2003b, 2011; Watanabe and Tokuda, 2010; Tokuda et al., 2014) suggesting a widespread ability to utilize cellulose-based materials as food in the clade. Only Cryptocercus and lower termites have a collaborative relationship with flagellates for lignocellulose digestion. The flagellates and their prokaryotic symbionts orchestrate a microbial feeding chain driven by the primary fermentations of carbohydrates to short-chain fatty acids, the major source of energy for the host. Each of the different flagellate populations appears to have a specific role in lignocellulose digestion (Brugerolle and Radek, 2006; Brune, 2011, 2014).
The hindguts of Cryptocercus and lower termites are flagellate dominated communities. These unicellular eukaryotes can reach population sizes of 105 per host individual, representing 60% of total hindgut weight (Bignell, 2011; Brune, 2011). Nonetheless, the literature on host-microbiome interaction in insects in general and termites in particular is based primarily on their bacterial and fungal symbionts (e.g., Aanen et al., 2002; Engel and Moran, 2013; Mikaelyan et al., 2015a, 2017a; Graf, 2016; Diouf et al., 2018; Otani et al., 2019). This is partly because the mutualistic partnership with flagellates appears unique among insects, but also because of methodological difficulties. Analysis of genomic information in protists is more convoluted than in prokaryotes because of their cytological complexity: they have multiple chromosomes and carry large nuclear and extranuclear genomes (Caron et al., 2009). High variability in 18S rDNA copy number and discordance between eukaryotic genetics and morphology makes characterizing their microbiome more challenging (Chabé et al., 2017; Popovic and Parkinson, 2018); technology, however, is advancing quickly (Hongoh, 2010; Carpenter et al., 2013; Altermatt et al., 2015).
The flagellates of Cryptocercus and the lower termites are derived from parabasalid and oxymonadid lineages that were acquired before Cryptocercus and termites split in the late Jurassic (∼140–170 Mya, Lo et al., 2003a; Lo and Eggleton, 2011; Bourguignon et al., 2015); it is likely that Cryptocercus harbors descendants of the original set of symbiotic flagellates that gave rise to their current diversity (Carpenter et al., 2009; Ohkuma et al., 2009). Most are found nowhere else in nature (Honigberg, 1970), however, a few taxa exhibit a much wider occurrence and are present in cockroaches other than Cryptocercus as well as in a variety of metazoan groups (Wenrich, 1935; Nalepa, 1991).
Here the focus is on the flagellated protists in the termite lineage, with the understanding that they are just one link in a vast array of complex metabolic networks distributed among microbial populations (Hongoh, 2011). Attention is further narrowed to the large flagellates known to engulf wood particles extensively studied by L. R. Cleveland; I acknowledge a bias against the small, often bacterivorous protists in using this approach. Although extraordinarily diverse, the large flagellates will here be treated as a group, as they have variable but similar responses to their environment during the molting cycle of their host (see Nalepa, 2017), a central topic here.
Flagellates
Flagellates are predominantly free-swimming organisms (Raven, 2000; Bogitsh et al., 2018), and require water for the trophic stage of their life cycle. Most cannot tolerate the viscid, moist rather than wet environments in which bacteria thrive (Bradbury, 1987). The oxymonad and parabasalid flagellates are furthermore amitochondriate and typically inhabit anoxic or hypoxic environments, either free-living in rich organic matter in the sediments of water bodies, or as symbionts in anaerobic sites within their animal hosts (Brugerolle and Müller, 2000; Treitli et al., 2018). Flagellates that live in hindguts originally derive from free-living anaerobic protozoa (Wenrich, 1935; Fenchel, 1987), an easy transition as hindguts satisfy both their major habitat requirements: the hindgut environment is both liquid and anoxic, with steep gradients at the oxic-anoxic interface (Brune, 1998; Brune and Friedrich, 2000).
If desiccation and oxygen levels are not a problem, the active, trophic phase of animal associated flagellates are able to travel through the outside environment to a new host (Foissner, 2006). Because protists are so fragile in the trophic stage of their life cycle, however, it is reasonable to assume that dispersal occurs primarily via cysts in terrestrial environments (Corliss and Esser, 1974; Foissner, 2007; Parfrey, 2015). Encystment is a complex, highly sophisticated, gene-regulated differentiating process in which the mobile trophozoite transforms into a dormant, resistant life stage. It typically involves drastic cytoplasmic dehydration, metabolic inactivation, autophagic activity, formation of a cyst wall, gene-silencing, and DNA repair (Gutiérrez et al., 1998, 2001; Schaap and Schilde, 2018). The ‘biological goal’ of encystation is differentiation into a form that can survive in unfavorable conditions. While encystment of free-living protists allows them to survive harsh ecological circumstances, cysts of animal associated protists are first and foremost a transmission strategy (Bradbury, 1987; Vickerman, 2000; Corliss, 2002; Foissner, 2006; Lauwaet et al., 2007).
Transmission
The mechanism by which symbionts are transmitted is important because it influences the extent to which symbiont fitness interests are aligned with those of the host. There are two basic strategies: horizontal transmission, which occurs across positions in space and is assumed to be the basal condition, and vertical, which takes place across generations in time, from parent to offspring (Baquero, 2017). It is vertical transmission that favors mutualistic relationships, because it is the mechanism by which a lineage of symbionts becomes consistently associated with a host, allowing the relationship to become obligatorily codependent. Once ‘captured’ via vertical transmission, selection on the symbionts becomes inescapably embedded in selection on the host (Ewald, 1987; Alizon et al., 2009; Sachs et al., 2011; Mushegian and Ebert, 2016; Fisher et al., 2017; Brown and Akçay, 2019).
The evolution of transmission mode of hindgut flagellates in the termite ancestor was closely associated with changes in host social structure and feeding behaviors (Nalepa et al., 2001). Horizontal transmission of microbes is typified by extant gregarious cockroaches, where hatchlings are inoculated primarily via coprophagy, the ingestion of feces produced by conspecifics which contain protozoan cysts, bacterial cells and spores (Hoyte, 1961b; Cruden and Markovetz, 1984; Hackstein and Stumm, 1994). Vertical transmission is exemplified by Cryptocercus, a subsocial cockroach whose neonates obtain their gut symbionts by feeding directly on the gut fluids of parents (proctodeal trophallaxis) (Seelinger and Seelinger, 1983; Nalepa, 1984; Park et al., 2002). These two taxa bracket the horizontal versus vertical transmission dichotomy in cockroaches. The key question, then, is how was the transition from horizontal to vertical made, and what were the consequences for the flagellate-host relationship?
Horizontal Transmission – Lophomonas Model
In termite ancestors, the step prior to subsociality as exemplified by Cryptocercus was likely a gregarious lifestyle, with groups of kin and non-kin hosts of various developmental stages living together in aggregations with a relatively fluid membership. Gregarious behavior is the most basic social adaptation for microbial transmission, and is exhibited to some degree by a number of unrelated detritivores (Nalepa et al., 2001). The ancestral host-flagellate relationship in the termite clade may have been similar to the one extant gregarious cockroaches have with Lophomonas, a flagellate found sporadically in several species of synanthropic cockroaches and closely related to the Trichonymphida found in the gut of Cryptocercus and lower termites (Kudo, 1926b; Gile and Slamovits, 2012). Lophomonas is typically found in 8–14% of examined hosts, but can reach levels of 48% (see Martinez-Girón et al., 2017). As in other protists found in non-Cryptocercus cockroaches (e.g., Lucas, 1927; Kidder, 1937; Hoyte, 1961a), Lophomonas is not known to engulf wood particles and is consistently described as a commensal (Kudo, 1926a, b; Gile and Slamovits, 2012; Martinez-Girón and Van Woerden, 2013; Martinez-Girón et al., 2017); there is, however, no empirical evidence to support that categorization (discussed further below).
Lophomonas has a basic two-stage life cycle (Hoyte, 1961a): an active trophozoite (vegetative, trophic stage) in the gut, and a cyst, the dormant, resistant stage excreted to the outside environment by the host. Cysts of Lophomonas reach new hosts via coprophagy; they then by-pass the gizzard and digestive enzymes of the coprophage before reaching the fluid environment of the gut (midgut, according to Lucas, 1927), where the trophozoite emerges from the cyst and establishes itself anew as part of the gut microbiome. Environmental conditions that trigger encystation of protists are not precisely defined, but may occur in response to deficiency of nutrients, osmotic pressure, temperature changes, low pH, accumulation of waste products, and crowding (Bradbury, 1987; Bogitsh et al., 2018). Cysts of Lophomonas are produced sporadically in both juvenile and adult cockroaches, and can be found throughout the year (Kudo, 1926b). There is no evidence that encystation is coordinated with host reproduction or development; both cysts and trophozoites can be found in the gut during host ecdysis. Trophozoites survive the molting process and the size and shape of cysts does not change (Hoyte, 1961a) (Figure 1).
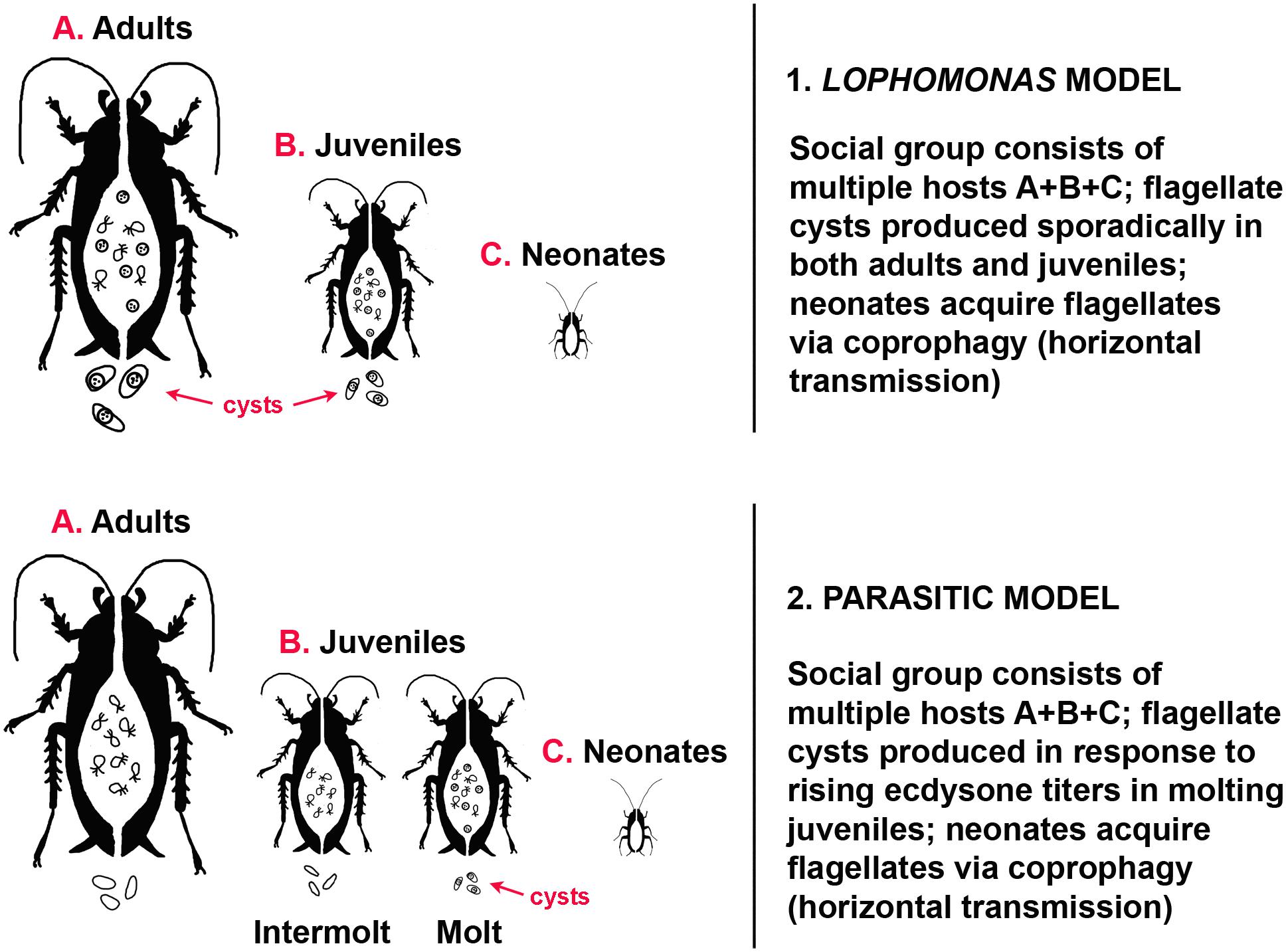
Figure 1. Models of transmission dynamics in gregarious cockroaches. (1) Lophomonas model. Adults (A) do not molt, trophozoites and cysts are present in the gut, cysts are present in the feces. Trophozoites and cysts are present in the gut of molting and intermolt juveniles (B), cysts are present in the feces. Neonates (C) hatch without protists in the gut. (2) Parasitic model. Adults (A) do not molt, trophozoites are present in the gut, no cysts are present in the feces. Trophozoites are present in the gut of intermolt juveniles (B), no cysts in the feces; cysts are present in both the gut and feces of molting juveniles. Neonates (C) hatch without protists in the gut.
Horizontal transmission of Lophomonas is related to gregarious behavior in cockroaches because coprophagy requires some degree of site fidelity and host social contact (Nalepa et al., 2001; Bignell, 2011). Aggregation sites serve as infection banks, concentrations of fecal pellets containing encysted protists together with potential hosts in a limited space. Transmission of the flagellates relies on the excretory and feeding behavior of the cockroaches, and dispersal of the protists in space depends on the movement of the hosts among aggregation sites. It should be noted, however, that airborne cysts of Lophomonas occur and may cause a form of human bronchopulmonary disease (Martinez-Girón and Van Woerden, 2013; Fakhar et al., 2019).
Vertical Transmission – Cryptocercus
In sharing a common ancestor with lower termites, Cryptocercus provides evidence that both vertical transmission of symbionts and metabolic interdependence between host and flagellates were present prior to the evolution of eusociality (Noirot, 1995). Furthermore, the unique life history of Cryptocercus can be assumed to reflect an early evolutionary stage of the termite clade (Klass et al., 2008). Characteristics of Cryptocercus most relevant to the evolution of symbiont transmission are that they are subsocial, living in biparental family groups, and that they are semelparous, i.e., they produce a single clutch of eggs in their lifetime. Field collections in both Asia and the United States are consistent in finding that the basic social structure is a male-female pair, together with a single cohort of offspring (Seelinger and Seelinger, 1983; Nalepa, 1984; Nalepa et al., 1997; Park et al., 2002).
Comparable to the flagellate Lophomonas noted above, the flagellates in Cryptocercus have a basic two-stage life cycle: the vegetative trophozoite, and the resistant cyst. Unlike Lophomonas, however, factors initiating encystment cycles of the flagellates in Cryptocercus are well characterized. Although it was recently noted that data on interactions between host physiology and gut microbiota are lacking (Macke et al., 2017), the work of L. R. Cleveland on the flagellates of Cryptocercus has been consistently overlooked; he clearly established the link between host hormones and the encystment cycles of the gut flagellates in Cryptocercus (reviewed by Nalepa, 2017). Rising titers of host ecdysone associated with juvenile hosts entering their molting cycle is the stimulus initiating encystment cycles in all taxa of cellulolytic hindgut flagellates examined. The timing of these physiological events in the host and symbionts is tightly synchronized, but the signal pathways activated and gene regulation and expression during the process are unexplored (see Jeelani et al., 2012; Gao et al., 2015). Although some of the resultant flagellate cysts are retained in the host hindgut and others are passed in the feces, there is no evidence that these cysts play a role in intergenerational transmission. The social structure of Cryptocercus, combined with the physiology of encystment of these particular protists mandates that flagellate transmission could occur only via proctodeal trophallaxis from parents (Nalepa, 1994). Adults pairs produce a single clutch of eggs in self-excavated galleries in a rotting log, then remain with that single cohort of offspring until parental death. Consequently, older nymphs are not present in galleries when adults reproduce, and cysts are never found in the feces of adults or intermolt juveniles (Cleveland et al.1934). Thus, coprophagy as a mechanism of intergenerational transmission is ruled out; adults do not molt and therefore do not excrete cysts, and older nymphs are absent from the social group. All protozoa in family members originate from the hindgut fluids of founding parents via parental care in the form of anal trophallaxis (Figure 2). After flagellates are established in the gut of neonates at the third instar (Nalepa, 1990), it is possible that cysts are exchanged among siblings via coprophagy (Figure 2). Nonetheless, all flagellates producing those cysts originated from parental hindgut fluids, because all juvenile siblings acquired them as hatchlings via parental trophallaxis.
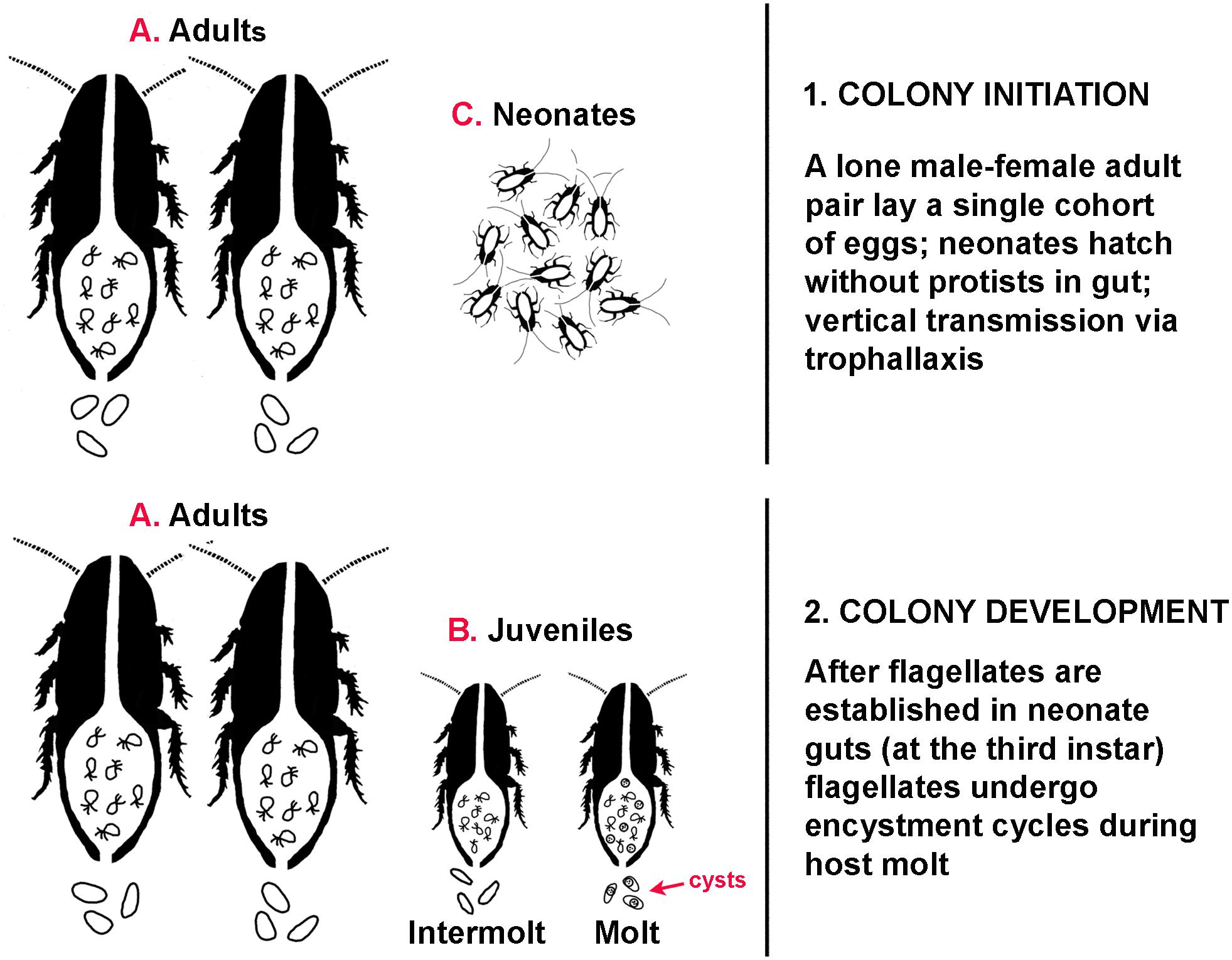
Figure 2. Transmission dynamics during colony ontogeny in the subsocial wood feeding cockroach Cryptocercus. (1) Colony initiation. Adults (A) do not molt, trophozoites are present in the gut, no cysts are present in the feces. Neonates (C) hatch without protists in the gut. (2) Colony development. Trophozoites are present in the gut of adults (A) and juveniles (B); cysts are present in the gut and feces of molting juveniles.
The Flaw
Although the Lophomonas to Cryptocercus model may offer a plausible horizontal to vertical transition on the surface, there are flaws in the argument. In Cryptocercus, there is tight integration of the encystment cycles of the flagellates with hormonal cycles in juvenile hosts, despite the cysts having little to do with intergenerational transmission. This suggests that the strong physiological connectivity between host development and the two-stage life cycle of the flagellates is an evolutionary contingency, a trait inherited from a gregarious ancestor (Nalepa et al., 2001). Such clear metabolic integration indicates that the host-flagellate relationship in the ancestor of Cryptocercus was unlikely to be commensal in the classical sense.
Commensals or Parasites?
Although non-pathogenic protozoans are commonly referred to as commensals, this interpretation is problematic because they are physiologically dependent on the host to complete their life cycle and should be considered parasites (Bogitsh et al., 2018). Indeed, the very definition of a parasite indicates an obligate metabolic dependence on the host (Cheng, 1970; Goater et al., 2014); the host has maximum fitness without the parasite, but the parasite without a host has a fitness equal to zero (Combes, 2001). Even so, metabolic dependence on the host is a vague criterion for characterizing the symbiotic relationship of a protist living in the gut of a terrestrial insect, because wherever it may fall on the symbiotic spectrum, it depends on the host gut environment for the trophozoite stage of its life cycle. A flagellate always benefits from the gut environment; consequently, its symbiotic relationship may be better characterized in terms of fitness effects on the host (see Casadevall and Pirofski, 2015). It would be challenging if not impossible, however, to quantify adverse effects of the flagellates on their cockroach partners; in many parasites, negative effects on the fitness of the host are undetectable (Goater et al., 2014); the same can be said of cryptic benefits to the host. There may be a very narrow neutral zone where costs and benefits to the host are in balance and the relationship described as a true commensalism (Figure 3). A range of undetectable effects likely occur on a microscale and are mercurial depending on a number of subtle contextual factors, including those originating from the vast array of other microbial taxa in the gut, and host diet, digestive physiology, developmental stage, and immune responses. Parasitism, then, may be best described as the condition where host cost exceeds benefit, whether or not the cost is detectable. Similarly, mutualism may be thought to occur when the benefit to the host is greater than the cost of hosting the flagellates. Nonetheless, undetectable effects on the host are not amenable to measurement, thus making interactions that fall into the commensalism range (Figure 3) a vague theoretical construct (Zapalski, 2011).
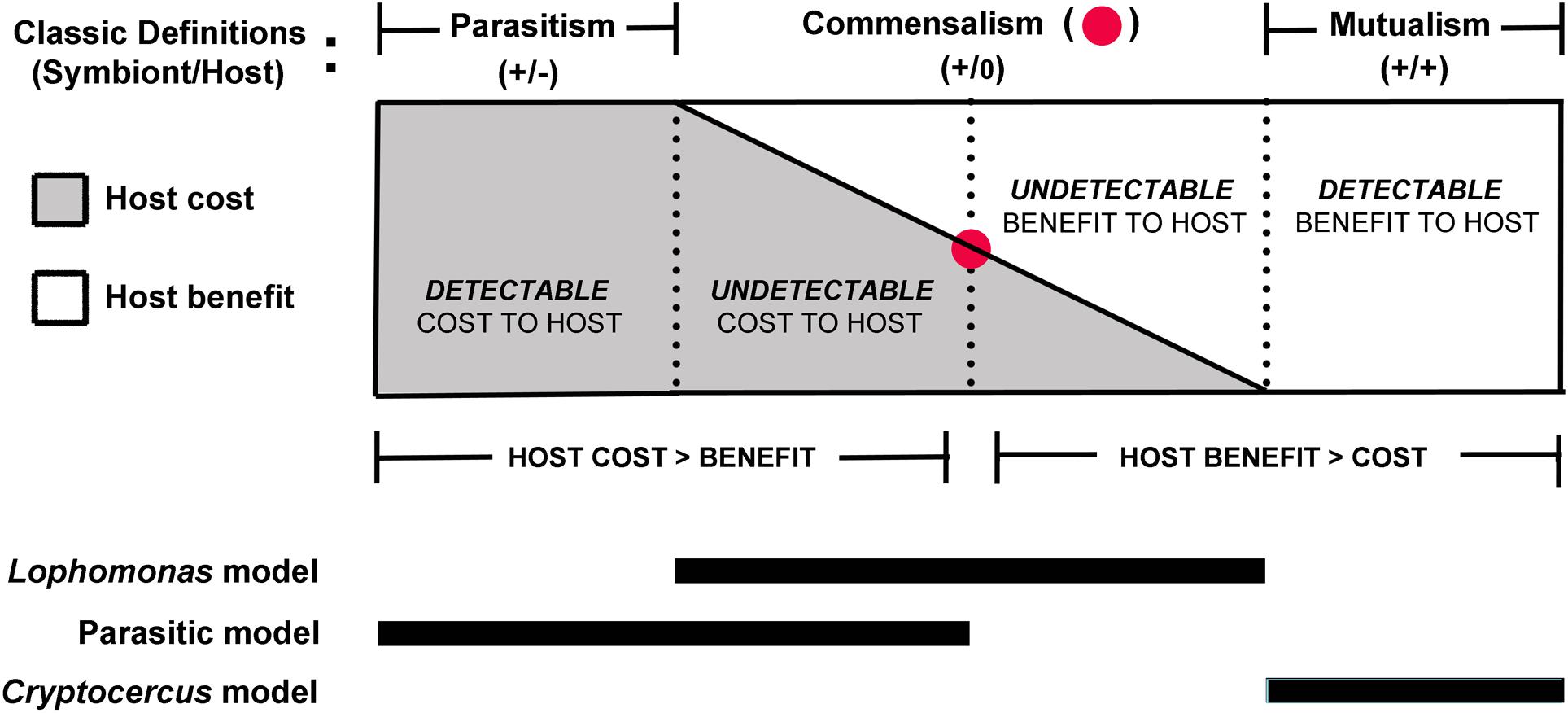
Figure 3. Spectrum of symbiotic interactions in proposed models of the relationship of cockroaches to their gut flagellates. Parasitism and mutualism bookend an unprovable theoretical interval (commensalism) within the continuum of the symbiotic spectrum (see Zapalski, 2011); undetectable effects do not lend themselves to empirical proof. Because the protists always benefit from the relationship, the most relevant criteria for classification of the interaction are detectable damage or benefit in the host, described by the damage response framework (Casadevall and Pirofski, 2015). Regardless of the degree of detectable or undetectable negative impact on the host in the parasitic model, a change to vertical transmission would have strong potential to push the interaction to the mutualistic end of the spectrum.
The well-being of Lophomonas is known to be directly linked to the well-being of its host (Hoyte, 1961a); it can be eliminated from the gut by starving its cockroach partner, and benefits from a host diet of carbohydrates (Armer, 1944). Its nutritional needs are met by phagocytosing starch grains, fungi, spores, small flagellates and bacteria found in the surrounding fluid environment (Kudo, 1926b), but also may include metabolic products of the host. Lophomonas clearly benefits from the host gut environment, but detectable costs or benefits to its host await further study.
Metabolic Connectivity
A commensal relationship is inconsistent with the physiological level of host–flagellate life cycle integration that must have existed in the gregarious predecessor of Cryptocercus, suggesting that the relationship was on the parasitic end of the symbiotic spectrum. The exquisite coordination of the complex encystment cycles of the flagellates with the molting cycle of their host (see Nalepa, 2017) suggests that parasitic flagellates in the Cryptocercus-termite clade were already present in a distant gregarious ancestor. A two-stage life cycle that is synchronized with host life history is a common feature among extant parasites (Møller et al., 1993; Chávez-Munguía et al., 2007), and steroid hormones in particular seem to stimulate the finely tuned developmental shift to the dormant transmission stage (Lawrence, 1986, 1991; Beckage, 1991). Encystment of Opalina ranarum, for example, is coordinated with the breeding season of its amphibian host via their gonadotropic and sex hormones (Bieniarz, 1950; El Mofty and Smyth, 1964). The orchestration of host and symbiont life cycles in the Cryptocercus-termite clade suggests that the flagellates were highly integrated with host physiology long before their host was dependent on the flagellates.
Why Coordinate Developmental Cycles?
In the gregarious ancestor, parasitic flagellates evolved to respond to a shift in host hormonal titers in anticipation of an appropriate time to initiate physiological changes associated with dispersal as cysts outside the host body; encystment cycles are frequently associated with seasonality of the environment or other kinds of periodicity. Because parasite fitness is determined primarily by transmission success (Vickerman, 2000; Combes, 2001; Leung and Poulin, 2008), there had to be a selective advantage to coordinating encystment with the molting cycle of the host. That advantage lies in the life history and behavior of gregarious cockroaches. Aggregations are compatible with parasite transmission events because emitters (molting juveniles), vehicles (cyst-filled feces) and receivers (other potential hosts) co-exist in time and space (Møller et al., 1993; Baquero, 2017). Juvenile cockroaches are typically gregarious, coprophagous (Bell et al., 2007) and the stages that molt. Although the life cycle of the flagellates would reach a dead end in infected adults, from the parasite point of view any juvenile host would assure the production of cysts and therefore continuity of the lineage (Figure 1).
Cost to Host
Gut flagellates must utilize host resources to meet their needs. In so doing alterations to the nutrient/energy budget of the infected host are expected. Parasites can cause anywhere from undetectable to drastic changes in host fitness, but harm can occur even with no measurable effect on the host. The cost may be slight, but it always exists (Combes, 2005; Poulin, 2007; Michalakis, 2009; Goater et al., 2014). To illustrate this point, Combes (2001) noted that an ant weighing 1 mg that flies on a Boeing 747 across the Atlantic costs the airline 10,000 molecules of fuel. The negative effect on host fitness is, however, more complex than the simple pilfering of host nutrients. An understanding of the mechanisms that link infection, host metabolism, immunity and life history are required to interpret the magnitude and direction of parasite-induced effects (Vickerman, 2000; Michalakis, 2009; Goater et al., 2014). Hosts may alter their physiology in a way that allows then to tolerate the presence of the parasite, and other microbes resident in the gut may play a role in shaping the way that the host body responds to infections. A variety of potentially virulent microbes are fairly harmless as long as they are part of a rich natural community of other symbionts in the same host (Poulin, 1995; Hudson et al., 2006). Avoidance of parasitism by the host is difficult because systems of recognizing parasites by hosts are limited (Perrot-Minnot and Cézilly, 2009), and in cockroaches, coprophagy has benefits that may outweigh any costs of parasitism. Cockroach feces offer fragmented, moistened and softened fare, and are enriched with lipids, carbohydrates, amino acids, unsaturated fatty acids, sterols, vitamins and cell debris originating from the excretor, its resident gut fauna, and the microbes that colonize the fecal pellet after its deposition in the external environment. The bacteria on feces ‘predigest’ recalcitrant foodstuffs, detoxify unpalatable chemicals, and are themselves utilized as food by the coprophage. Feces additionally serve as inoculum in the horizontal transmission of beneficial gut bacteria (reviewed by Nalepa et al., 2001; Bell et al., 2007). The presence of a mildly detrimental microbe may be ‘tolerated’ as a side effect of allowing beneficial species entry (Mushegian and Ebert, 2016). A parasitic flagellate of cockroaches that insinuates its cyst into this ingestible fecal bonanza has a high probability of finding a new host.
Transition From Parasitism to Mutualism
Symbioses are rarely static; they comprise a fluid spectrum of interactions from mutually beneficial to neutral to exploitative (Parmentier and Michel, 2013; Méthot and Alizon, 2014; Scharnagl, 2019). Although mutualistic relationships such as the one between Cryptocercus and its flagellates can arise in several ways from other interactions along the spectrum, many if not most cases of mutualism originate from an antagonistic, parasitic relationship (Mayhew, 2006; Weiblen and Treiber, 2015; Scharnagl, 2019). Mutualism differs from parasitism in only one way: instead of one partner in the association exploiting the other without reciprocity, in mutualism each partner exploits the other (Combes, 2005). The most commonly cited catalyst driving the change from parasitism to mutualism is the switch from horizontal to vertical transmission. That shift in transmission mode can lead not only to a reduction in parasite virulence (Ewald, 1987; Yamamura, 1993; Read, 1994; Thompson, 1994; Poulin, 2007; Schmid-Hempel, 2017), but also to evolution of host-symbiont interdependence. Parasites become totally dependent on host reproduction for their own transmission, pushing the flagellates to evolve metabolically in a way more favorable to the host (Yamamura, 1993; Herre et al., 1999; Weiblen and Treiber, 2015). Novel interactions of vertically transmitted symbionts with their host can originate and progress rapidly under the pressure of adapting to the transformed relationship, adjusting them as a unit to each other and to their shared selective environment (McLaughlin and Cain, 1983; Thompson, 1994; Leung and Poulin, 2008; Gilbert et al., 2010; Gerardo and Hurst, 2017).
Host Domination
Parasites are inextricably linked to the host that houses them, because the host is not only the resource base for a parasite, but also its habitat and vehicle (Combes, 2001). The establishment and maintenance of symbioses seem to be driven largely by the top–down influence of the host (Scharnagl, 2019). Although there are numerous examples of parasites driving changes in host behavior, these are predominantly in parasites with complex life cycles (i.e., those that require more than one host species to complete their life cycle) (Combes, 1991; Perrot-Minnot and Cézilly, 2009), not the basic two-stage life cycle of the flagellates in Cryptocercus. Selection may furthermore limit the evolution of host manipulation by any one microbial taxon in the gut microbiome, because of the large number of species and strains that compete with one another for space and resources (Johnson and Foster, 2018; Giudice, 2019).
The Behavioral Change
The shift from horizontal to vertical transmission in the termite lineage lies squarely at the intersection between the evolution of host family life and the co-evolution of the host–symbiont relationship. The change from a gregarious social structure with coprophagy as a mechanism of microbial transfer, to family life with parents directly transferring gut microbiota to offspring in a liquid medium with fleeting, if any, exposure to oxygen is here proposed as the catalyst that drove the change from the parasitic to the mutualistic end of the spectrum in flagellates.
If the relationship between host and protists was originally parasitic then the direct transfer of the flagellates from parents to neonates via trophallaxis should be unexpected; hosts are typically under selection to avoid parasitism (Moore, 2013), particularly in the case of parents infecting vulnerable juveniles. However, if the parasites were of low virulence (discussed below) and if there were benefits to the direct transfer of hindgut fluids for reasons having little to do with the parasitic effect of the flagellates, then that host behavioral change is capable of initiating a cascade of evolutionary events that eventually results not only in host-flagellate mutualism but also termite eusociality (Nalepa, 2015). It is the presence of fitness benefits for either the host or the parasite that remains the crucial criterion determining whether a behavioral change is adaptive (Poulin, 1995).
Host Fitness Benefits
A variety of fitness benefits accrue to a host that relies on trophallaxis rather than coprophagy to establish the gut microbiota in neonates. In addition to the above listed advantages of coprophagy, parental transfer of hindgut fluids would provide a more consistent transfer of the core prokaryotic assemblage associated with gregarious cockroach lineages (Schauer et al., 2014; Mikaelyan et al., 2016). Bacteria in cockroach guts display tremendous phylogenetic diversity, and include members of Bacteroidetes, Firmicutes, Fibrobacteres, and Proteobacteria (Dietrich et al., 2014; Mikaelyan et al., 2015b). The assemblage is dominated by obligate anaerobes, including those that break down structural polysaccharides of plant-based detritus (Bignell, 1977; Cruden and Markovetz, 1979, 1984, 1987), produce semiochemicals that influence host social behavior (Wada-Katsumata et al., 2015) and directly affect development by regulating gene expression in their host (Cruden and Markovetz, 1987; Jahnes et al., 2019). Another advantage of trophallaxis is that metabolic products of the parents and their resident microbial assemblage would not be compromised by exposure to the outside environment; these include hormones, enzymes, metabolites, and other chemicals that may serve as physiological or behavioral signals. Perhaps most importantly, vertical transmission of parasitic flagellate trophozoites would subject protists to the digestive activities of the cockroach at the receiving end of the interaction. While cysts of parasites associated with gregarious cockroaches evolved to withstand mastication, passage through the proventriculus (gizzard), and digestion by the host prior to reaching the hindgut, the large trophozoites are vulnerable and a potentially high quality, proteinaceous food source (Grassé and Noirot, 1945; Grassé, 1952; Machida et al., 2001; Nalepa et al., 2001; Brune and Ohkuma, 2011; Tokuda et al., 2014). This makes it impossible to separate symbiont transfer from nutrient transfer during gut fluid delivery (Nalepa, 2015) particularly when early juvenile stages are the recipients. The protozoan symbiosis is not established until the third instar in Cryptocercus (Nalepa, 1990); prior to that flagellate cytoplasm transferred from parents may help fuel their high nitrogen requirements for growth. Proctodeal trophallaxis also reinforces social structure because, unlike coprophagy, it requires physical contact and behavioral interaction (McMahan, 1969; Nalepa et al., 2001). Finally, the transfer of parental hindgut fluid frees young cockroaches from the necessity of seeking out fecal pellets, the distribution of which may be sporadic depending on the population dynamics and the size of the home aggregation.
Trophallaxis as Parental Care
In insects, shifts in behavior play a central role in the regulation of microbial associations and are contingent on the evolutionary history of both the host and symbionts (Poulin, 1995; Ezenwa et al., 2012). Two aspects of cockroach evolutionary history are relevant here. First, because coprophagy was already established in the gregarious ancestor, the transition to proctodeal trophallaxis was seamless. It is not a huge leap from feeding on a fresh fecal pellet to feeding directly on hindgut fluids, making trophallaxis a simple shift in an existing behavior (Nalepa, 1994; Nalepa et al., 2001). Second, there are a number of independent origins of parental care in cockroaches where adults feed neonates on bodily secretions (Bell et al., 2007: Table 8.4). Given the recalcitrance of cellulose-based substrates, the onset of trophallaxis can be envisioned as the most efficient way to transfer nutritional resources to offspring (Nalepa, 1994). This suggestion is reinforced by the observation of adults of the wood-feeding cockroach Salganea feeding their young on oral fluids but without evidence of symbiont transfer (Shimada and Maekawa, 2011). Regardless of the selection pressure driving the evolution of trophallaxis-based parental care in the termite lineage, it had monumental consequences for the host–symbiont relationship and is a prime example of how host social organization can affect parasite transmission dynamics (Ezenwa et al., 2016).
Evolution of the Mutualism
Ecological Basis
The sign and magnitude of a host–symbiont interaction is largely dependent on ecological context (Michalakis et al., 1992; Bronstein, 1994; Méthot and Alizon, 2014; Coyte et al., 2015; Bogitsh et al., 2018; Tipton et al., 2019): both that of the host within its habitat, and of the host as habitat for its microbiota (Bush et al., 2001; Goater et al., 2014). Trace fossils indicate that the early ancestors of cockroaches and termites fed on decaying plant matter, based on coprolite structure and distinctive pith borings in the stems of tree ferns (Labandeira and Phillips, 2002). Extant cockroach species have furthermore maintained their close association with rotting plant detritus in the natural environment, with plant structural polymers playing a significant role in their nutritional ecology (Roth and Willis, 1960; Nalepa and Bandi, 2000; Bell et al., 2007). Given that protists are major decomposers, contributing substantially to organic carbon mineralization and nutrient recycling (Wetzel, 2001; Corliss, 2002; Geisen et al., 2017), and that the liquid, anaerobic environment of the cockroach hindgut is not far removed from that of the anoxic sediments of water bodies, it seems likely that a shared capacity for utilizing cellulose-based material was the basis for the parasitic relationship in the gregarious ancestor. The flagellates would be preadapted for feeding and survival in the host digestive system, and the host gut would be a concentrated and continuous source of macerated plant detritus not only small enough for flagellates to phagocytose (Watanabe and Tokuda, 2010), but also accessible with few travel costs. The ingestion of particulate food via food vacuoles and its subsequent digestion by enzymes is thought to be a primitive feature of eukaryotic cells (Sleigh, 2000). Both the parasite and the gregarious host, then, likely possessed at least some capacity for cellulolytic digestion, either inherited from their respective ancestors or acquired via horizontal gene transfer from prokaryotes sometime during their evolutionary history (Lo et al., 2003b; Davison and Blaxter, 2005; Todaka et al., 2010; Sato et al., 2014). Bacteria are an important source of new genetic sequences for eukaryotes (Brugerolle and Müller, 2000; Sieber et al., 2017), and include those that confer the ability to degrade plant carbohydrates, to live anaerobically, and to adopt a parasitic life style. Each of these is documented in members of the Excavata: in Giardia intestinalis, Trichomonas vaginalis, Leishmania spp., and Trypanosoma spp. (Husnik and McCutcheon, 2018).
Parasitism to Mutualism
The basis of the parasitic exploitation of the gregarious host by the flagellates is suggested to be the diversion of host-ingested detritus and associated prokaryotes for their own nourishment, while providing few metabolic products that increased fitness in the cockroach. If so, the parasites would be of relatively low virulence because hosts could compensate by changing their feeding behavior (Ponton et al., 2011; Goater et al., 2014). A modest increase in feeding rate by an ancestral gregarious cockroach may have been sufficient to provide adequate nourishment for both the host and its intestinal parasites.
If we accept this cellulolytic parasite scenario, then the mechanisms leading to mutualism were present before these single-celled organisms made the transition to that lifestyle. The flagellates were not only adapted to the gut environment but also dependent on the host, with their two-stage life cycle highly integrated into the physiological underpinnings of host development. It was a stable interaction, with the flagellate potentially obtaining more fitness benefits than the host. Cellulose digestion became a cooperative endeavor after the flagellates were taken into host custody via vertical transmission, pushing flagellates to the mutualism end of the symbiotic spectrum. Parasites are adept at changing metabolic pathways and evolve rapidly in response to new selective pressures (Poulin, 1998; Tachezy and Šmíd, 2008); they have a surprising stem cell network controlled by intrinsic and extrinsic mechanisms of cell conversion and differentiation (Niculescu, 2014). The importance of protists in biogeochemical nutrient cycling in the external environment (Geisen et al., 2017) furthermore suggests that they have a wealth of metabolic capacities that could be exploited by the host.
The currency exchanged (Wein et al., 2019) between the ancestral cockroach and its flagellates depends on knowing the physiological basis of the symbiotic partnership, a difficulty given the complex cocktail of metabolites involved and the derived nature of the microbiome in extant members of the lineage. Nonetheless, many animal groups produce cellulases on their own, but these are generally incomplete and must be supplemented by symbiotic microorganisms (Lo et al., 2003b, 2011). Current evidence suggests that cellulose degradation in the lower termites is initiated by enzymes in the host salivary glands, then advanced in the hindgut by the flagellates; this dual system has been proposed as a model of efficient cellulose hydrolysis (Sugio et al., 2006; Ni and Tokuda, 2013). A reasonable assumption is that the flagellates shifted from exploiting cellulose in the host gut, to making its digestion a more efficient cooperative endeavor by integrating themselves into the cellulolytic metabolic pathways of the host. Vertical transmission (= proctodeal trophallaxis) was a first step leading to the extraordinarily complex division of labor in the termite-flagellate symbiosis, just as proctodeal trophallaxis (= vertical transmission) was the first step eventually leading to division of labor in the eusocial host (Nalepa, 2015).
Downstream Effects
An expected consequence of vertical transmission is the initiation of a positive feedback loop between the newly minted host-flagellate digestive capabilities and host food choices. Increasing ability to metabolize lignocellulose would push the host to include more of it in its diet, feeding back on the metabolic contributions of the flagellate. A host diet high in structural polysaccharides and the development of the mutualistic partnership to fully digest it each establish the conditions for the development of the other. Eventually an ancestral cockroach that included rotted plant debris in its diet could make the transition to an exclusively wood diet, taking advantage of a relatively competition poor ecological niche and allowing the host–symbiont partnership to avail itself of the most abundant biomass on earth (Ni and Tokuda, 2013).
Another evolutionary consequence of the direct transfer of hindgut fluids was increased opportunity for protists to form symbiotic relationships with prokaryotes (Nalepa et al., 2001). Few symbiotic associations are recognized between bacteria and free-living obligate anaerobic flagellates (Fenchel and Finlay, 2010), but eukaryote-prokaryote relationships are both prevalent and increasingly well documented in the guts of Cryptocerus/lower termites. Their guts harbor a large pool of diverse bacteria associated with protists (Noda et al., 2009), and proctodeal feeding assures passage of established microbial consortia. The relationship between gut eubacteria and archaea with flagellates can be ecto- or endosymbiotic, and may be coevolved and stable or exhibit frequent host switches. Integration ranges from transient affairs to permanent, obligatory partnerships, each of which provides an opportunity for cross-feeding, for communication, and for genes to move to a new genome (Hongoh et al., 2005; Noda et al., 2007, 2018; Keeling and Palmer, 2008; Desai et al., 2010; Hongoh and Ohkuma, 2018).
Functionally, the cellulose-rich diet of the cockroach host ensures that the currency exchanged in these inter-microbe relationships would involve overcoming the deficits of food that has an excess of structural polysaccharides and a deficit of nitrogen. Cellulose digestion is advanced in the hindgut by the flagellates, but also by their associated bacteria (Tokuda et al., 2014; Yuki et al., 2015; Treitli et al., 2019), as well as by prokaryotes free-living in hindgut fluids (Ni and Tokuda, 2013). Gut bacteria acquire, conserve and recycle nitrogen, as well as serve as direct sources of protein (Thong-On et al., 2009; Desai and Brune, 2012; Tokuda et al., 2014; Tai et al., 2016). The duo of nitrogen-fixing bacterial endosymbionts and their cellulolytic protist host together enable the highly efficient growth of their host termite (Hongoh et al., 2008). Although the specifics of exchanged metabolites are yet largely unknown, recent studies are starting to reveal the astounding degree of complexity embodied in just one flagellate-bacteria relationship. Flagellates not known to engulf wood particles, like Streblomastix strix in the termite Zootermopsis, may have bacterial associates that fix nitrogen, provide amino acids and cofactors, and digest cellulose, some of which may fuel their flagellate host. The bacteria in turn lack at least one essential enzyme, which may be overcome by an exchange of intermediates with the flagellate. Other members of the gut flagellate community seem to play a role, and Streblomastix additionally internalizes and digests its epibiotic bacteria (Treitli et al., 2019). These insect-protist-bacterial relationships have been called triplex or tripartite (Brune and Stingl, 2005; Noda et al., 2007) but recent work indicates that additional terms may be needed. Utami et al. (2018) recently took the nested symbioses to a fourth level: Treponema spirochetes living on oxymonad protists in the gut of Cryptocercus/lower termites have ectosymbiotic bacteria of their own (see also Pramono et al., 2017).
A Conundrum
If flagellates are so adept at switching metabolic pathways, it is a mystery as to why they retain their two-stage life cycle in Cryptocercus. The metabolic and genomic complexity of the encystment cycle suggests that it entails a substantial cost, and construction of a cyst wall requires considerable investment in protein and chitin (Eichinger, 2001). Cysts became largely defunct for intergenerational transmission at the onset of anal trophallaxis in the ancestral hosts, but the flagellates could not revert to a functional two-stage life cycle via horizontal transmission because of the concomitant change in host social structure (Figure 2). Nonetheless, vertical transmission of flagellates in Cryptocercus is unlikely to be as tightly coordinated as vertically transmitted, obligate intracellular symbionts (e.g., Sacchi et al., 2000; Arab et al., 2020). There may always be some noise in a transmission system that relies on host behavior, and vertical transmission in Cryptocercus should be considered high fidelity but not perfect (Tai et al., 2015). The life history of Cryptocercus does not rule out horizontal transmission but it is probably rare. Older, melanized juveniles may leave the natal log and shelter temporarily in abandoned or short, self-excavated galleries (Nalepa and Grayson, 2011). Because these subadults have a molt or two left before maturity, feces that contain cysts may be deposited outside their natal gallery. It is unknown how long these cysts are viable (but is amenable to testing).
It could be that a two-stage parasitic life-cycle is a difficult habit to break. The flagellates may be trapped in a maladaptive state because, like a variety of protozoan parasites (e.g., Clopton et al., 2016) cysts are an obligate stage in the life cycle. Ancient events in phylogeny can commit a lineage forever, particularly if the protist is dependent on its host (Poulin, 2007). Although difficult to explain in evolutionary terms, there are other parasites with a dead-end in their life cycle (Vickerman, 2000; Mushegian and Ebert, 2016). In the human parasite Entamoeba histolytica, for example, those amoeba that end up in deep tissue sites are not further involved in the life cycle and reach a blind alley (Goater et al., 2014).
Because trophallaxis eases selection pressure to retain costly encystment cycles in the flagellates, a plausible explanation for their existence is that their deep integration with host physiology cannot be disengaged. This would accord with the idea of host domination in the evolution of the lineage, and with the ‘flagellate as victim’ hypothesis (Nalepa, 2017). Cysts in the feces of molting Cryptocercus nymphs, as well of vestiges of the encystment process in termites may be a legacy of their distant gregarious past. Response of protists to the molting hormones of the host nonetheless was strongly influential further along their shared evolutionary trajectory (Nalepa, 2017).
The Power of Host Behavioral Change
Figure 4 illustrates some of the self-reinforcing co-evolutionary networks resulting from one host behavioral change: the shift from horizontal to vertical transmission. The principle outcomes are the transition from parasitism to mutualism in the flagellates, host-flagellate interdependence, and the origination of their cooperative partnership in processing lignocellulose. The latter feeds back on host food choices, and continuous residency of the flagellates in host gut fluids allowed for increased multilayered prokaryotic-eukaryotic integration and collaboration. Such feedback processes cement mutualisms and can accelerate during co-evolution (Biedermann and Rohlfs, 2017).
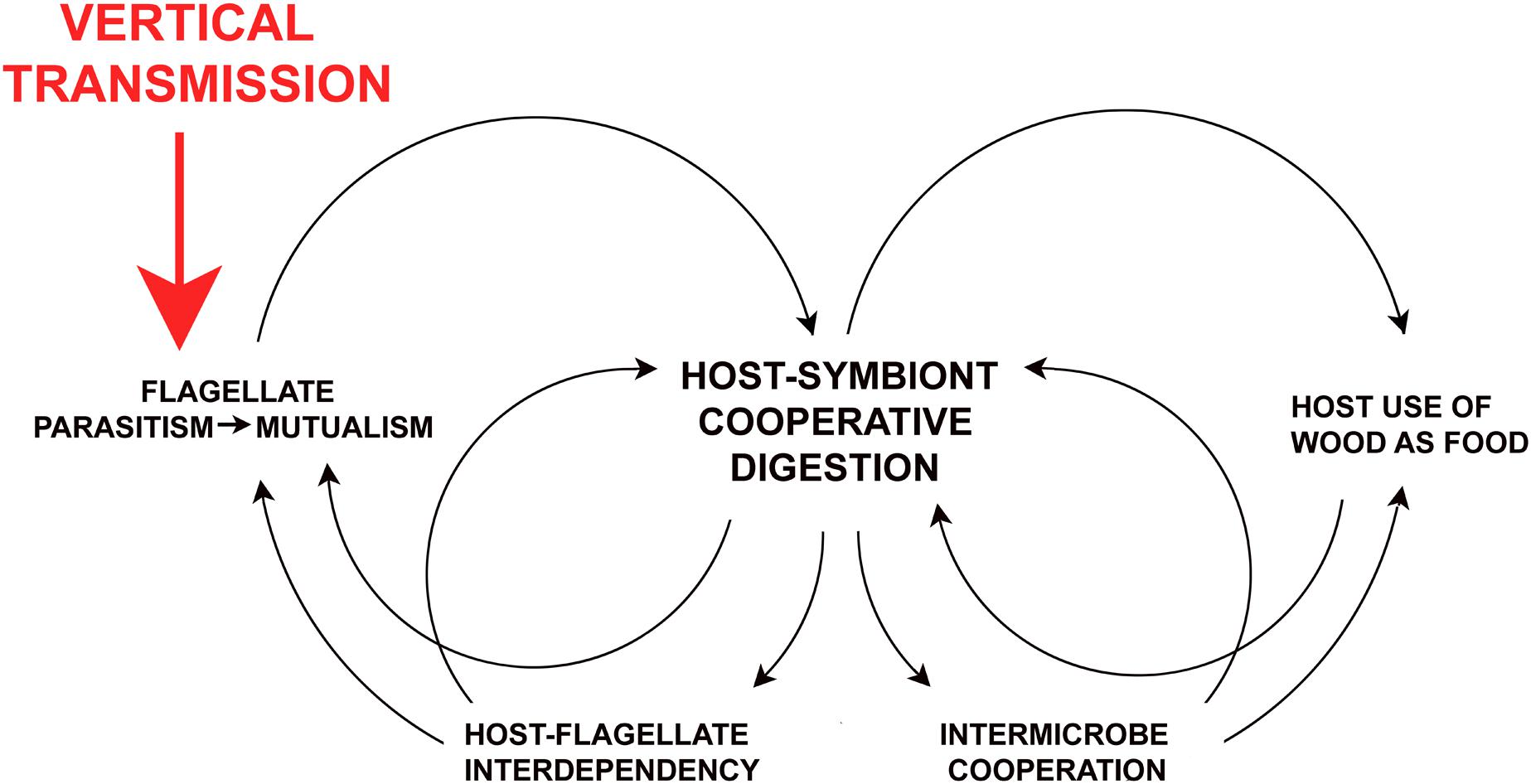
Figure 4. Self-reinforcing dynamics of vertical transmission on host-gut symbiont relationships in the termite ancestors. Consequences of vertical transmission (proctodeal trophallaxis) on social evolution and life history of the host are addressed in Nalepa (2011, 2015, 2017).
It should be noted that the host behavioral change from coprophagy to trophallaxis also had potent evolutionary consequences for host social structure, as it was the genesis of obligate subsociality and trophallactic exchanges in the lineage. Hosts became dependent on flagellates, neonates became dependent on parents, and eventually, termite colony members became dependent on each other (Nalepa, 2015, 2017). Vertical transmission additionally instigated or facilitated division of labor on three known levels: between hosts and their gut microbiota, among the diverse array of microbes that settled into the host gut, and eventually, among the members of the host social group. Arguably, then, it was the single key event in the genesis of termites from cockroaches, reinforcing the idea of behavioral change as a potent influence on the evolutionary trajectory of host-symbiont lineages.
Author Contributions
The author confirms being the sole contributor of this work and has approved it for publication.
Conflict of Interest
The author declares that the research was conducted in the absence of any commercial or financial relationships that could be construed as a potential conflict of interest.
Acknowledgments
I thank the editor PB for the invitation to write this paper, Aram Mikaelyan for insightful comments on the manuscript, Pat Rand for feedback on the figures, and Conrad Labandeira for references. The comments of reviewers considerably improved the manuscript.
References
Aanen, D. K., Eggleton, P., Rouland-Lefevre, C., Guldberg-Frøslev, T., Rosendahl, S., and Boomsma, J. J. (2002). The evolution of fungus-growing termites and their mutualistic fungal symbionts. Proc. Nat. Acad. Sci. U.S.A. 99, 14887–14892. doi: 10.1073/pnas.222313099
Alizon, S., Hurford, A., Mideo, N., and Van Baalen, M. (2009). Virulence evolution and the trade-off hypothesis: history, current state of affairs and the future. J. Evol. Biol. 22, 245–259. doi: 10.1111/j.1420-9101.2008.01658.x
Altermatt, F., Fronhofer, E. A., Garnier, A., Giometto, A., Hammes, F., Klecka, J., et al. (2015). Big answers from small worlds: a user’s guide for protist microcosms as a model system in ecology and evolution. Methods Ecol. Evol. 6, 218–231. doi: 10.1111/2041-210x.12312
Arab, D. A., Bourguignon, T., Wang, Z., Ho, S. Y. W., and Lo, N. (2020). Evolutionary rates are correlated between cockroach symbionts and mitochondial genomes. Biol. Lett. 16:20190702. doi: 10.1098/rsbl.2019.0702
Armer, J. M. (1944). Influence of the diet of Blattidae on some of their intestinal Protozoa. J. Parasitol. 30, 131–142.
Baquero, F. (2017). Transmission as a basic process in microbial biology. Lwoff Award Prize Lecture. Fems Microbiol. Rev. 41, 816–827. doi: 10.1093/femsre/fux042
Beckage, N. E. (1991). Host-parasite hormonal relationships: a common theme? Exp. Parasitol. 72, 332–338. doi: 10.1016/0014-4894(91)90153-n
Bell, W. J., Roth, L. M., and Nalepa, C. A. (2007). Cockroaches: Behavior, Ecology and Evolution. Baltimore, MA: The Johns Hopkins University Press.
Biedermann, P. H. W., and Rohlfs, M. (2017). Evolutionary feedbacks between insect sociality and microbial management. Curr. Opin. Ins. Sci. 22, 92–100. doi: 10.1016/j.cois.2017.06.003
Bieniarz, J. (1950). Influence of vertebrate gonadotropic hormones upon the reproductive cycle of certain protozoa in frogs. Nature 165, 650–651. doi: 10.1038/165650a0
Bignell, D. E. (1977). An experimental study of cellulose and hemicellulose degradation in the alimentary canal of the American cockroach. Canad. J. Zool. 55, 579–589. doi: 10.1139/z77-073
Bignell, D. E. (2011). “Morphology, physiology, biochemistry and functional design of the termite gut: an evolutionary wonderland,” in Biology of Termites: A Modern Synthesis, eds D. E. Bignell, Y. Roisin, and N. Lo (Berlin: Springer), 375–412. doi: 10.1007/978-90-481-3977-4_14
Bogitsh, B. J., Carter, C. E., and Oeltmann, T. N. (2018). Human Parasitology. Cambridge, MA: Academic Press.
Bourguignon, T., Lo, N., Cameron, S. L., Sobotnik, J., Hayashi, Y., Shigenobu, S., et al. (2015). The evolutionary history of termites as inferred from 66 mitochondrial genomes. Molec. Biol. Evol. 32, 406–421. doi: 10.1093/molbev/msu308
Bradbury, P. C. (1987). “Protozoan adaptations for survival,” in Survival and Dormancy of Microorganisms, ed. Y. Henis (Hoboken, NJ: John Wiley and Sons), 267–299.
Breznak, J. A., and Brune, A. (1994). Role of microorganisms in the digestion of lignocellulose by termites. Ann. Rev. Entomol. 39, 453–487. doi: 10.1007/s00284-018-1502-4
Bronstein, J. L. (1994). Conditional outcomes in mutualistic interactions. Trends Ecol. Evol. 9, 214–217. doi: 10.1016/0169-5347(94)90246-1
Brown, A., and Akçay, E. (2019). Evolution of transmission mode in conditional mutualisms with spatial variation in symbiont quality. Evolution 73, 128–144. doi: 10.1111/evo.13656
Brugerolle, G., and Müller, M. (2000). “Amitochondriate flagellates,” in Flagellates: Unity, Diversity and Evolution, eds B. Leadbeater and J. Green (Milton Park: Taylor and Francis), 166–189.
Brugerolle, G., and Radek, R. (2006). “Symbiotic protozoa of termites,” in Intestinal microorganisms of termites and other invertebrates, eds H. Konig, and A. Varma (Berlin: Springer-Verlag), 243–269.
Brune, A. (1998). Termite guts: the world’s smallest bioreactors. Trends Biotechnol. 16, 16–21. doi: 10.1016/s0167-7799(97)01151-7
Brune, A. (2011). “Microbial symbioses in the digestive tract of lower termites,” in Beneficial Microorganisms in Multicellular Life Forms, eds E. Rosenberg, and U. Gophna (Berlin: Springer), 3–25. doi: 10.1007/978-3-642-21680-0_1
Brune, A. (2014). Symbiotic digestion of lignocellulose in termite guts. Nat. Rev. Microbiol. 12, 168–180. doi: 10.1038/nrmicro3182
Brune, A., and Friedrich, M. (2000). Microecology of the termite gut: structure and function on a microscale. Curr. Opin. Microbiol. 3, 263–269. doi: 10.1016/s1369-5274(00)00087-4
Brune, A., and Ohkuma, M. (2011). “Role of the termite gut microbiota in symbiotic digestion,” in Biology of Termites: A Modern Synthesis, eds D. E. Bignell, Y. Roisin, and N. Lo (Berlin: Springer), 439–475. doi: 10.1007/978-90-481-3977-4_16
Brune, A., and Stingl, U. (2005). “Prokaryotic symbionts of termite gut flagellates: phylogenetic and metabolic implications of a tripartite symbiosis,” in Prokaryotic Symbionts of Termite gut Flagellates: Phylogenetic and Metabolic Implications of a Tripartite Symbiosis. Molecular Basis of Symbiosis eds J. Overmann, and J. Overmann (Berlin: Springer), 39–60. doi: 10.1007/3-540-28221-1_3
Bush, A. O., Fernández, J. C., Esch, G. W., and Seed, J. R. (2001). Parasitism: The Diversity And Ecology Of Animal Parasites. Cambridge: Cambridge University Press.
Caron, D. A., Worden, A. Z., Countway, P. D., Demir, E., and Heidelberg, K. B. (2009). Protists are microbes too: a perspective. ISME J. 3:4. doi: 10.1038/ismej.2008.101
Carpenter, K. J., Chow, L., and Keeling, P. J. (2009). Morphology, phylogeny, and diversity of Trichonympha (Parabasalia: Hypermastigida) of the wood−feeding cockroach Cryptocercus punctulatus. J. Eukary. Microbiol. 56, 305–313. doi: 10.1111/j.1550-7408.2009.00406.x
Carpenter, K. J., Weber, P. K., Davisson, M. L., Pett-Ridge, J., Haverty, M. I., and Keeling, P. J. (2013). Correlated SEM, FIB-SEM, TEM, and NanoSIMS imaging of microbes from the hindgut of a lower termite: methods for in situ functional and ecological studies of uncultivable microbes. Microsc. Microanal. 19, 1490–1501. doi: 10.1017/S1431927613013482
Casadevall, A., and Pirofski, L.-A. (2015). What is a host? Incorporating the microbiota into the damage-response framework. Infect. Immun. 83, 2–7. doi: 10.1128/IAI.02627-14
Casadevall, A., and Pirofski, L.-A. (2019). Benefits and costs of animal virulence for microbes. mBio 10, e863–e819.
Chabé, M., Lokmer, A., and Ségurel, L. (2017). Gut protozoa: friends or foes of the human gut microbiota? Trends Parasitol. 33, 925–934. doi: 10.1016/j.pt.2017.08.005
Chávez-Munguía, B., Omaña-Molina, M., González-Lázaro, M., González-Robles, A., Cedillo-Rivera, R., Bonilla, P., et al. (2007). Ultrastructure of cyst differentiation in parasitic protozoa. Parasitol. Res. 100, 1169–1175. doi: 10.1007/s00436-006-0447-x
Cleveland, L. R. (1926). Symbiosis among animals with special reference to termites and their intestinal flagellates. Quart. Rev. Biol. 1, 51–59.
Cleveland, L. R., Hall, S. R., Sanders, E. P., and Collier, J. (1934). The wood feeding roach Cryptocercus, its protozoa, and the symbiosis between protozoa and roach. Mem. Amer. Acad. Arts Sci. 17, 185–342. doi: 10.1111/j.1550-7408.2011.00564.x
Clopton, R. E., Steele, S. M., and Clopton, D. T. (2016). Environmental persistence and infectivity of oocysts of two species of Gregarines, Blabericola migrator and Blabericola cubensis (Apicomplexa: Eugregarinida: Blabericolidae), parasitizing blaberid cockroaches (Dictyoptera: Blaberidae). J. Parasitol. 102, 169–173. doi: 10.1645/15-934
Combes, C. (1991). Ethological aspects of parasite transmission. Amer. Nat. 138, 866–880. doi: 10.1086/285257
Combes, C. (2001). Parasitism: The Ecology And Evolution Of Intimate Interactions. Chicago, IL: University of Chicago Press.
Corliss, J. O. (2002). Biodiversity and biocomplexity of the protists and an overview of their significant roles in maintenance of our biosphere. Acta Protozool. 41, 199–220.
Corliss, J. O., and Esser, S. C. (1974). Comments on the role of the cyst in the life cycle and survival of free-living protozoa. Trans. Amer. Microscop. Soc. 93, 578–593.
Coyte, K. Z., Schluter, J., and Foster, K. R. (2015). The ecology of the microbiome: Networks, competition, and stability. Science 350, 663–666. doi: 10.1126/science.aad2602
Cruden, D. L., and Markovetz, A. J. (1979). Carboxymethyl cellulose decomposition by intestinal bacteria of cockroaches. Appl. Environ. Microbiol. 38, 369–372. doi: 10.1128/aem.38.3.369-372.1979
Cruden, D. L., and Markovetz, A. J. (1984). Microbial aspects of the cockroach hindgut. Arch. Microbiol. 138, 131–139. doi: 10.1007/bf00413013
Cruden, D. L., and Markovetz, A. J. (1987). Microbial ecology of the cockroach gut. Ann. Rev. Microbiol. 41, 617–643. doi: 10.1146/annurev.mi.41.100187.003153
Davison, A., and Blaxter, M. (2005). Ancient origin of glycosyl hydrolase family 9 cellulase genes. Molec. Biol. Evol. 22, 1273–1284. doi: 10.1093/molbev/msi107
Desai, M. S., and Brune, A. (2012). Bacteroidales ectosymbionts of gut flagellates shape the nitrogen-fixing community in dry-wood termites. ISME J. 6:1302. doi: 10.1038/ismej.2011.194
Desai, M. S., Strassert, J. F., Meuser, K., Hertel, H., Ikeda−Ohtsubo, W, Radek, R., et al. (2010). Strict cospeciation of devescovinid flagellates and Bacteroidales ectosymbionts in the gut of dry−wood termites (Kalotermitidae). Environ. Microbiol. 12, 2120–2132.
Dietrich, C., Köhler, T., and Brune, A. (2014). The cockroach origin of the termite gut microbiota: patterns in bacterial community structure reflect major evolutionary events. Appl. Environ. Microbiol. 80, 2261–2269. doi: 10.1128/AEM.04206-13
Diouf, M., Herve, V., Mora, P., Robert, A., Frechault, S., Rouland-Lefevre, C., et al. (2018). Evidence from the gut microbiota of swarming alates of a vertical transmission of the bacterial symbionts in Nasutitermes arborum (Termitidae, Nasutitermitinae). Anton. Leeuw. Int. J.G. 111, 573–587. doi: 10.1007/s10482-017-0978-4
Eichinger, D. (2001). Encystation in parasitic protozoa. Curr. Opin. Microbiol. 4, 421–426. doi: 10.1016/s1369-5274(00)00229-0
El Mofty, M., and Smyth, J. (1964). Endocrine control of encystation in Opalina ranarum parasitic in Rana temporaria. Exper. Parasitol. 15, 185–199. doi: 10.1016/0014-4894(64)90015-3
Engel, P., and Moran, N. A. (2013). The gut microbiota of insects–diversity in structure and function. FEMS Microbiol. Rev. 37, 699–735. doi: 10.1111/1574-6976.12025
Ewald, P. W. (1987). Transmission modes and evolution of the parasitism-mutualism continuum. Ann. N. Y. Acad. Sci. 503, 295–306. doi: 10.1111/j.1749-6632.1987.tb40616.x
Ezenwa, V. O., Archie, E. A., Craft, M. E., Hawley, D. M., Martin, L. B., Moore, J., et al. (2016). Host behaviour - parasite feedback: an essential link between animal behaviour and disease ecology. Proc. Roy. Soc. B Biol. Sci. 283:9. doi: 10.1098/rspb.2015.3078
Ezenwa, V. O., Gerardo, N. M., Inouye, D. W., Medina, M., and Xavier, J. B. (2012). Animal behavior and the microbiome. Science 338, 198–199.
Fakhar, M., Nakhaei, M., Sharifpour, A., Kalani, H., Banimostafavi, E. S., Abedi, S., et al. (2019). First molecular diagnosis of lophomoniasis: the end of a controversial story. Acta Parasitol. 64, 360–393. doi: 10.2478/s11686-019-00084-2
Fenchel, T. (1987). Ecology of the Protozoa. The Biology of Free-living Phagotrophic Protists. Berlin: Springer-Verlag.
Fenchel, T., and Finlay, B. J. (2010). “Free-living protozoa with endosymbiotic methanogens,” in Endo Symbiotic Methanogenic Archaea, ed. J. H. P. Hackstein (Berlin: Springer-Verlag), 1–11. doi: 10.1007/978-3-642-13615-3_1
Fisher, R. M., Henry, L. M., Cornwallis, C. K., Kiers, E. T., and West, S. A. (2017). The evolution of host-symbiont dependence. Nat. Commun. 8, 8.
Foissner, W. (2006). Biogeography and dispersal of micro-organisms: a review emphasizing protists. Acta Protozool. 45, 111–136.
Foissner, W. (2007). Dispersal and biogeography of protists: recent advances. Jpn. J. Protozool. 40, 1–16.
Gao, X., Chen, F., Niu, T., Qu, R., and Chen, J. (2015). Large-scale identification of encystment-related proteins and genes in Pseudourostyla cristata. Sci. Rep. 5:11360. doi: 10.1038/srep11360
Geisen, S., Mitchell, E. A. D., Wilkinson, D. M., Adl, S., Bonkowski, M., Brown, M. W., et al. (2017). Soil protistology rebooted: 30 fundamental questions to start with. Soil Biol. Biochem. 111, 94–103. doi: 10.1016/j.soilbio.2017.04.001
Genta, F. A., Terra, W. R., and Ferreira, C. (2003). Action pattern, specificity, lytic activities, and physiological role of five digestive β-glucanases isolated from Periplaneta americana. Ins. Biochem. Molec. Biol. 33, 1085–1097. doi: 10.1016/s0965-1748(03)00121-8
Gerardo, N., and Hurst, G. (2017). Q and A: friends (but sometimes foes) within: the complex evolutionary ecology of symbioses between host and microbes. BMC Biol. 15:126. doi: 10.1186/s12915-017-0455-6
Gilbert, S. F., Mcdonald, E., Boyle, N., Buttino, N., Gyi, L., Mai, M., et al. (2010). Symbiosis as a source of selectable epigenetic variation: taking the heat for the big guy. Phil. Trans. Roy. Soc. London BBiol. Sci. 365, 671–678. doi: 10.1098/rstb.2009.0245
Gile, G. H., and Slamovits, C. H. (2012). Phylogenetic position of Lophomonas striata Butschli (Parabasalia) from the hindgut of the cockroach Periplaneta americana. Protist 163, 274–283. doi: 10.1016/j.protis.2011.07.002
Giudice, M. D. (2019). Invisible designers: brain evolution through the lens of parasite manipulation. Quart. Rev. Biol. 94, 249–282. doi: 10.1086/705038
Goater, T. M., Goater, C. P., and Esch, G. W. (2014). Parasitism: The Diversity And Ecology Of Animal Parasites. Cambridg: Cambridge University Press.
Graf, J. (2016). Lessons from digestive-tract symbioses between bacteria and invertebrates. Ann. Rev.Microbiol. 70, 375–393. doi: 10.1146/annurev-micro-091014-104258
Grassé, P. P. (1952). Role des flagellés symbiotique chez les Blattes et les Termites. Tijdschr. Entomol. 95, 70–80.
Grassé, P.-P., and Noirot, C. (1945). La transmission des flagellés symbiotiques et les aliments des termites. Biol. Bull. France Belg. 79, 273–297.
Gutiérrez, J., Callejas, S., Borniquel, S., Benítez, L., and Martín-González, A. (2001). Ciliate cryptobiosis: a microbial strategy against environmental starvation. Inter. Microbiol. 4, 151–157. doi: 10.1007/s10123-001-0030-3
Gutiérrez, J. C., Martin-Gonzalez, A., and Callejas, S. (1998). Nuclear changes, macronuclear chromatin reorganization and DNA modifications during ciliate encystment. Eur. J. Protist. 34, 97–103. doi: 10.1016/s0932-4739(98)80018-7
Hackstein, J. H. P., and Stumm, C. K. (1994). Methane production in terrestrial arthropods. Proc. Nat. Acad. Sci. 91, 5441–5445. doi: 10.1073/pnas.91.12.5441
Herre, E., Knowlton, N., Mueller, U., and Rehner, S. (1999). The evolution of mutualisms: exploring the paths between conflict and cooperation. Trends Ecol. Evol. 14, 49–53. doi: 10.1016/s0169-5347(98)01529-8
Hongoh, Y. (2010). Diversity and genomes of uncultured microbial symbionts in the termite gut. Biosci. Biotechnol. Biochem. 74, 1145–1151. doi: 10.1271/bbb.100094
Hongoh, Y. (2011). Toward the functional analysis of uncultivable, symbiotic microorganisms in the termite gut. Cell. Molec. Life Sci. 68, 1311–1325. doi: 10.1007/s00018-011-0648-z
Hongoh, Y., Deevong, P., Inoue, T., Moriya, S., Trakulnaleamsai, S., Ohkuma, M., et al. (2005). Intra- and interspecific comparisons of bacterial diversity and community structure support coevolution of gut microbiota and termite host. Appl. Environ. Microbiol. 71, 6590–6599. doi: 10.1128/aem.71.11.6590-6599.2005
Hongoh, Y., and Ohkuma, M. (2018). “Termite gut flagellates and their methanogenic and eubacterial symbionts,” in (Endo)symbiotic Methanogenic Archaea, ed. J. H. P. Hackstein (Berlin: Springer), 55–80. doi: 10.1007/978-3-319-98836-8_5
Hongoh, Y., Sharma, V. K., Prakash, T., Noda, S., Toh, H., Taylor, T. D., et al. (2008). Genome of an endosymbiont coupling N2 fixation to cellulolysis within protist cells in termite gut. Science 322, 1108–1109. doi: 10.1126/science.1165578
Honigberg, B. M. (1970). “Protozoa associated with termites and their role in digestion,” in Biology of Termites, eds K. Krishna, and F. M. Weesner (New York, NY: Academic Press), 1–36.
Hoyte, H. M. D. (1961a). The protozoa occurring in the hind-gut of cockroaches. I. Responses to changes in environment. Parasitol. 51, 415–436. doi: 10.1017/s0031182000070700
Hoyte, H. M. D. (1961b). The protozoa occurring in the hindgut of cockroaches II. Morphology of Nyctotherus ovalis. Parasitol. 51, 437–463. doi: 10.1017/s0031182000070712
Hudson, P. J., Dobson, A. P., and Lafferty, K. D. (2006). Is a healthy ecosystem one that is rich in parasites? Trends Ecol. Evol. 21, 381–385. doi: 10.1016/j.tree.2006.04.007
Huitzil, S., Sandoval-Motta, S., Frank, A., and Aldana, M. (2018). Modeling the role of the microbiome in evolution. Front. Physiol. 9:14.
Husnik, F., and McCutcheon, J. P. (2018). Functional horizontal gene transfer from bacteria to eukaryotes. Nat. Rev. Microbiol. 16, 67–79. doi: 10.1038/nrmicro.2017.137
Ikeda-Ohtsubo, W., and Brune, A. (2009). Cospeciation of termite gut flagellates and their bacterial endosymbionts: Trichonympha species and ’Candidatus Endomicrobium trichonympha’. Molec. Ecol. 18, 332–342. doi: 10.1111/j.1365-294x.2008.04029.x
Jahnes, B. C., Herrmann, M., and Sabree, Z. L. (2019). Conspecific coprophagy stimulates normal development in a germ-free model invertebrate. Peerj 7, 18. doi: 10.7717/peerj.6914
Jeelani, G., Sato, D., Husain, A., Escueta-De Cadiz, A., Sugimoto, M., Soga, T., et al. (2012). Metabolic profiling of the protozoan parasite Entamoeba invadens revealed activation of unpredicted pathway during encystation. PLoS One 7:e37740. doi: 10.1371/journal.pone.0037740
Johnson, K. V. A., and Foster, K. R. (2018). Why does the microbiome affect behaviour? Nat. Rev. Microbiol. 16, 647–655. doi: 10.1038/s41579-018-0014-3
Keeling, P. J., and Palmer, J. D. (2008). Horizontal gene transfer in eukaryotic evolution. Nat. Rev. Genet. 9:605. doi: 10.1038/nrg2386
Kidder, G. W. (1937). The intestinal protozoa of the wood-feeding roach Panesthia. Parasitol 29, 163–203.
Klass, K.-D., Nalepa, C., and Lo, N. (2008). Wood-feeding cockroaches as models for termite evolution (Insecta: Dictyoptera): Cryptocercus vs. Parasphaeria boleiriana. Molec. Phylo. Evol. 46, 809–817. doi: 10.1016/j.ympev.2007.11.028
Kudo, R. (1926a). A cytological study of Lophomonas striata Butschli. Arch. Protistenkd. 55, 504–515.
Kudo, R. (1926b). Observations on Lophomonas blattarum, a flagellate inhabiting the colon of the cockroach. Blatta orientalis. Arch. Protistenkd. 53, 191–214.
Labandeira, C., and Phillips, T. L. (2002). Stem borings and petiole galls from Pennsylvanian tree ferns of Illinois, USA: implications for the origin of the borer and galler functional-feeding groups and holometabolous insects. Palaeontographica Abt. A 264, 1–84.
Lauwaet, T., Davids, B. J., Reiner, D. S., and Gillin, F. D. (2007). Encystation of Giardia lamblia: a model for other parasites. Curr. Opin. Microbiol. 10, 554–559. doi: 10.1016/j.mib.2007.09.011
Lawrence, P. O. (1986). Host-parasite hormonal interactions: an overview. J. Insect Physiol. 32, 295–298. doi: 10.1016/0022-1910(86)90042-9
Lawrence, P. O. (1991). Hormonal effects on insects and other endoparasites in vitro. In Vitro Cell. Develop. Biol. Animal. 27, 487–496. doi: 10.1007/bf02631150
Leung, T., and Poulin, R. (2008). Parasitism, commensalism, and mutualism: exploring the many shades of symbioses. Vie Milieu 58, 107.
Lin, N., and Michener, C. D. (1972). Evolution of sociality in insects. Quart. Rev. Biol. 47, 131–159.
Lo, N., Bandi, C., Watanabe, H., Nalepa, C., and Beninati, T. (2003a). Evidence for cocladogenesis between diverse dictyopteran lineages and their intracellular symbionts. Molec. Biol. Evol. 20, 907–913. doi: 10.1093/molbev/msg097
Lo, N., and Eggleton, P. (2011). “Termite phylogenetics and co-cladogenesis with symbionts,” in Biology of Termites: A Modern Synthesis, eds D. Bignell, Y. Roisin, and N. Lo (Berlin: Springer), 27–50. doi: 10.1007/978-90-481-3977-4_2
Lo, N., Tokuda, G., and Watanabe, H. (2011). “Evolution and function of endogenous termite cellulases,” in Biology of Termites: A Modern Synthesis, mus,, eds D. Bignell, Y. Roisin, and N. Lo (Berlin: Springer), pp. 51-67.
Lo, N., Watanabe, H., and Sugimura, M. (2003b). Evidence for the presence of a cellulase gene in the last common ancestor of bilaterian animals. Proc. Roy. Soc. B Biol. Sci. 270, S69–S72.
Lucas, C. L. (1927). Two new species of amoeba found in cockroaches: with notes on the cysts of Nyctotherus ovalis Leidy. Parasitol. 19, 223–235. doi: 10.1017/s003118200000562x
Machida, M., Kitade, O., Miura, T., and Matsumoto, T. (2001). Nitrogen recycling through proctodeal trophallaxis in the Japanese damp-wood termite Hodotermopsis japonica (Isoptera. Termopsidae). Ins. Soc. 48, 52–56. doi: 10.1007/pl00001745
Macke, E., Tasiemski, A., Massol, F., Callens, M., and Decaestecker, E. (2017). Life history and eco−evolutionary dynamics in light of the gut microbiota. Oikos 126, 508–531. doi: 10.1111/oik.03900
Martinez-Girón, R., Martinez-Torre, C., and Van Woerden, H. C. (2017). The prevalence of protozoa in the gut of German cockroaches (Blattella germanica) with special reference to Lophomonas blattarum. Parasitol. Res. 116, 3205–3210. doi: 10.1007/s00436-017-5640-6
Martinez-Girón, R., and Van Woerden, H. C. (2013). Lophomonas blattarum and bronchopulmonary disease. J. Med. Microbiol. 62, 1641–1648. doi: 10.1099/jmm.0.059311-0
Mayhew, P. J. (2006). Discovering Evolutionary Ecology: Bringing Together Ecology and Evolution. Oxford: Oxford University Press.
McLaughlin, G., and Cain, G. (1983). An inquiry into the nature of symbiosis. Evol. Theory 6, 185–196.
McMahan, E. A. (1969). “Feeding relationships and radioisotope techniques,” in Biology of Termites, eds K. Krishna, and F. M. Weesner (New York, NY: Academic Press), 387–406. doi: 10.1016/b978-0-12-395529-6.50016-7
Méthot, P.-O., and Alizon, S. (2014). What is a pathogen? Toward a process view of host-parasite interactions. Virulence 5, 775–785. doi: 10.4161/21505594.2014.960726
Michalakis, Y. (2009). Parasitism and the Evolution of Life-History Traits. Ecology and Evolution of Parasitism. Oxford: Oxford University Press, 19–30.
Michalakis, Y., Olivieri, I., Renaud, F., and Raymond, M. (1992). Pleiotropic action of parasites: how to be good for the host. Trends Ecol. Evol. 7, 59–62. doi: 10.1016/0169-5347(92)90108-N
Mikaelyan, A., Dietrich, C., Köhler, T., Poulsen, M., Sillam−Dussès, D., and Brune, A. (2015a). Diet is the primary determinant of bacterial community structure in the guts of higher termites. Molec. Ecol 24, 5284–5295. doi: 10.1111/mec.13376
Mikaelyan, A., Köhler, T., Lampert, N., Rohland, J., Boga, H., Meuser, K., et al. (2015b). Classifying the bacterial gut microbiota of termites and cockroaches: a curated phylogenetic reference database (DictDb). Syst. Appl. Microbiol. 38, 472–482. doi: 10.1016/j.syapm.2015.07.004
Mikaelyan, A., Meuser, K., and Brune, A. (2017a). Microenvironmental heterogeneity of gut compartments drives bacterial community structure in wood-and humus-feeding higher termites. FEMS Microbiol. Ecol. 93:fiw210. doi: 10.1093/femsec/fiw210
Mikaelyan, A., Thompson, C. L., Hofer, M. J., and Brune, A. (2016). Deterministic assembly of complex bacterial communities in guts of germ-free cockroaches. Appl. Environ. Microbiol. 82, 1256–1263. doi: 10.1128/AEM.03700-15
Mikaelyan, A., Thompson, C. L., Meuser, K., Zheng, H., Rani, P., Plarre, R., et al. (2017b). High−resolution phylogenetic analysis of Endomicrobia reveals multiple acquisitions of endosymbiotic lineages by termite gut flagellates. Environ. Microbiol. Rep. 9, 477–483. doi: 10.1111/1758-2229.12565
Møller, A. P., Dufva, R., and Allander, K. (1993). Parasites and the evolution of host social-behavior. Adv. Study Behav. 22, 65–102. doi: 10.1016/s0065-3454(08)60405-2
Moore, J. (2013). An overview of parasite-induced behavioral alterations - and some lessons from bats. J. Exp. Biol. 216, 11–17. doi: 10.1242/jeb.074088
Mushegian, A. A., and Ebert, D. (2016). Rethinking “mutualism” in diverse host−symbiont communities. Bioessays 38, 100–108. doi: 10.1002/bies.201500074
Nalepa, C. A. (1984). Colony composition, protozoan transfer and some life history characteristics of the woodroach Cryptocercus punctulatus Scudder. Behav. Ecol. Sociobiol. 14, 273–279. doi: 10.1007/bf00299498
Nalepa, C. A. (1990). Early development of nymphs and establishment of hindgut symbiosis in Cryptocercus punctulatus (Dictyoptera: Cryptocercidae). Ann. Entomol. Soc. Amer 83, 786–789. doi: 10.1093/aesa/83.4.786
Nalepa, C. A. (1991). Ancestral transfer of symbionts between cockroaches and termites: an unlikely scenario. Proc. Roy. Soc. Lon. Ser. B 246, 185–189. doi: 10.1098/rspb.1991.0143
Nalepa, C. A. (1994). “Nourishment and the evolution of termite eusociality,” in Nourishment and Evolution in Insect Societies, eds J. H. Hunt, and C. A. Nalepa (Boulder, CO: Westview Press), 57–104.
Nalepa, C. A. (2011). “Altricial development in wood-feeding cockroaches: the key antecedent of termite eusociality,” in Biology of Termites: A Modern Synthesis, eds D. Bignell, Y. Roisin, and N. Lo (Berlin: Springer), 69–96.
Nalepa, C. A. (2015). Origin of termite eusociality: trophallaxis integrates the social, nutritional, and microbial environments. Ecol. Entomol. 40, 323–335. doi: 10.1111/een.12197
Nalepa, C. A. (2017). What kills the hindgut flagellates of lower termites during the host molting cycle? Microorganisms 5:E82. doi: 10.3390/microorganisms5040082
Nalepa, C. A., and Bandi, C. (2000). “Characterizing the ancestors: paedomorphosis and termite evolution,” in Termites: Evolution, Sociality, Symbiosis, Ecology, eds T. Abe, D. E. Bignell, and M. Higashi (Dortrecht: Kluwar), 53–75. doi: 10.1007/978-94-017-3223-9_3
Nalepa, C. A., Bignell, D. E., and Bandi, C. (2001). Detritivory, coprophagy, and the evolution of digestive mutualisms in Dictyoptera. Ins. Soc. 48, 194–201. doi: 10.1007/pl00001767
Nalepa, C. A., Byers, G. W., Bandi, C., and Sironi, M. (1997). Description of Cryptocercus clevelandi (Dictyoptera: Cryptocercidae) from the northwestern United States, molecular analysis of bacterial symbionts in its fat body, and notes on biology, distribution, and biogeography. Ann. Entomol. Soc. Am. 90, 416–424. doi: 10.1093/aesa/90.4.416
Nalepa, C. A., and Grayson, K. L. (2011). Surface activity of the xylophagous cockroach Cryptocercus punctulatus (Dictyoptera: Cryptocercidae) based on collections from pitfall traps. Ann. Entomol. Soc. Amer. 104, 364–368. doi: 10.1603/an10133
Ni, J. F., and Tokuda, G. (2013). Lignocellulose-degrading enzymes from termites and their symbiotic microbiota. Biotech. Adv. 31, 838–850. doi: 10.1016/j.biotechadv.2013.04.005
Niculescu, V. F. (2014). The evolutionary history of eukaryotes: how the ancestral proto-lineage conserved in hypoxic eukaryotes led to protist pathogenicity. Microbiol. Disc. 2, 4. doi: 10.7243/2052-6180-2-4
Noda, S., Hongoh, Y., Sato, T., and Ohkuma, M. (2009). Complex coevolutionary history of symbiotic Bacteroidales bacteria of various protists in the gut of termites. BMC Evol. Biol. 9:158. doi: 10.1186/1471-2148-9-158
Noda, S., Inoue, T., Hongoh, Y., Kawai, M., Nalepa, C. A., Vongkaluang, C., et al. (2006). Identification and characterization of ectosymbionts of distinct lineages in Bacteroidales attached to flagellated protists in the gut of termites and a wood−feeding cockroach. Environ. Microbiol. 8, 11–20. doi: 10.1111/j.1462-2920.2005.00860.x
Noda, S., Kitade, O., Inoue, T., Kawai, M., Kanuka, M., Hiroshima, K., et al. (2007). Cospeciation in the triplex symbiosis of termite gut protists (Pseudotrichonympha spp.), their hosts, and their bacterial endosymbionts. Molec. Ecol. 16, 1257–1266. doi: 10.1111/j.1365-294x.2006.03219.x
Noda, S., Shimizu, D., Yuki, M., Kitade, O., and Ohkuma, M. (2018). Host-symbiont cospeciation of termite-gut cellulolytic protists of the genera Teranympha and Eucomonympha and their Treponema endosymbionts. Microbes Environ. 33, 26–33. doi: 10.1264/jsme2.ME17096
Noirot, C. (1995). The gut of termites (Isoptera). Comparative anatomy, sytematics, phylogeny. I. Lower termites. Ann. Soc. Entomol. FranceNouv. sér. 31, 197–226.
Ohkuma, M. (2008). Symbioses of flagellates and prokaryotes in the gut of lower termites. Trends Microbiol. 16, 345–352. doi: 10.1016/j.tim.2008.04.004
Ohkuma, M., and Brune, A. (2011). “Diversity, structure, and evolution of the termite gut microbial community,” in Biology of Termites: A Modern Synthesis, eds D. Bignell, Y. Roisin, and N. Lo (Berlin: Springer), 413–438. doi: 10.1007/978-90-481-3977-4_15
Ohkuma, M., Noda, S., Hongoh, Y., Nalepa, C. A., and Inoue, T. (2009). Inheritance and diversification of symbiotic trichonymphid flagellates from a common ancestor of termites and the cockroach Cryptocercus. Proc. Roy. Soc. London B: Biol. Sci. 276, 239–245. doi: 10.1098/rspb.2008.1094
Otani, S., Zhukova, M., Kone, N. A., Da Costa, R. R., Mikaelyan, A., Sapountzis, P., et al. (2019). Gut microbial compositions mirror caste-specific diets in a major lineage of social insects. Environ. Microbiol. Rep. 11, 196–205. doi: 10.1111/1758-2229.12728
Parfrey, L. W. (2015). Mock communities highlight the diversity of host-associated eukaryotes. Molec. Ecol. 24, 4337–4339. doi: 10.1111/mec.13311
Park, Y.-C., Grandcolas, P., and Choe, J. C. (2002). Colony composition, social behavior and some ecological characteristics of the Korean wood-feeding cockroach (Cryptocercus kyebangensis). Zool. Sci. 19, 1133–1139. doi: 10.2108/zsj.19.1133
Parmentier, E., and Michel, L. (2013). Boundary lines in symbiosis forms. Symbiosis 60, 1–5. doi: 10.1007/s13199-013-0236-0
Perrot-Minnot, M.-J., and Cézilly, F. (2009). “Parasites and behaviour,” in Ecology and Evolution of Parasitism, eds F. Thomas, J. F. Guégan, and F. Renaud (Oxford: Oxford University Press), 49–68.
Ponton, F., Lalubin, F., Fromont, C., Wilson, K., Behm, C., and Simpson, S. J. (2011). Hosts use altered macronutrient intake to circumvent parasite-induced reduction in fecundity. Int. J. Parasitol. 41, 43–50. doi: 10.1016/j.ijpara.2010.06.007
Popovic, A., and Parkinson, J. (2018). “Characterization of eukaryotic microbiome using 18S amplicon sequencing,” in Microbiome Analysis: Methods and Protocols, ed. R. G. E. A. Beiko (Berlin: Springer), 29–48. doi: 10.1007/978-1-4939-8728-3_3
Poulin, R. (1995). “Adaptive” changes in the behaviour of parasitized animals: A critical review. Int. J. Parasitol. 25, 1371–1383. doi: 10.1016/0020-7519(95)00100-x
Poulin, R. (1998). Evolutionary Ecology Of Parasites. From Individuals To Communities. London: Chapman and Hall.
Pramono, A. K., Kuwahara, H., Itoh, T., Toyoda, A., Yamada, A., and Hongoh, Y. (2017). Discovery and complete genome sequence of a bacteriophage from an obligate intracellular symbiont of a cellulolytic protist in the termite gut. Microbes Environment 32, 112–117. doi: 10.1264/jsme2.ME16175
Raven, J. (2000). “The flagellate condition,” in The Flagellates: Unity, Diversity and Evolution, eds B. Leadbeater, and J. Green (Milton Park: Taylor and Francis), 27–48.
Robinson, C. J., Bohannan, B. J. M., and Young, V. B. (2010). From structure to function: the ecology of host-associated microbial communities. Microbiol. Molec. Biol. Rev. 74, 453–476. doi: 10.1128/MMBR.0014-10
Roth, L. M., and Willis, E. R. (1960). The biotic associations of cockroaches. Smithson. Misc. Coll. 141, 1–470. doi: 10.1242/bio.20122683
Sacchi, L., Nalepa, C. A., Lenz, M., Bandi, C., Corona, S., Grigolo, A., et al. (2000). Transovarial transmission of symbiotic bacteria in Mastotermes darwiniensis Froggatt (Isoptera: Mastotermitidae): Ultrastructural aspects and phylogenetic implications. Ann. Entomol. Soc. Amer. 93, 1308–1313. doi: 10.1603/0013-8746(2000)093%5B1308:ttosbi%5D2.0.co;2
Sachs, J. L., Essenberg, C. J., and Turcotte, M. M. (2011). New paradigms for the evolution of beneficial infections. Trends Ecol. Evol. 26, 202–209. doi: 10.1016/j.tree.2011.01.010
Sato, T., Kuwahara, H., Fujita, K., Noda, S., Kihara, K., Yamada, A., et al. (2014). Intranuclear verrucomicrobial symbionts and evidence of lateral gene transfer to the host protist in the termite gut. ISME J. 8:1008. doi: 10.1038/ismej.2013.222
Schaap, P., and Schilde, C. (2018). Encystation: the most prevalent and underinvestigated differentiation pathway of eukaryotes. Microbiology 164, 727–739. doi: 10.1099/mic.0.000653
Schauer, C., Thompson, C., and Brune, A. (2014). Pyrotag sequencing of the gut microbiota of the cockroach Shelfordella lateralis reveals a highly dynamic core but only limited effects of diet on community structure. Plos One 9:e85861.
Schmid-Hempel, P. (2017). Parasites and their social hosts. Trends Parasitol. 33, 453–462. doi: 10.1016/j.pt.2017.01.003
Seelinger, G., and Seelinger, U. (1983). On the social organization, alarm and fighting in the primitive cockroach Cryptocercus punctulatus. Z. Tierpsychol. 61, 315–333. doi: 10.1111/j.1439-0310.1983.tb01347.x
Shimada, K., and Maekawa, K. (2011). Description of the basic features of parent−offspring stomodeal trophallaxis in the subsocial wood−feeding cockroach Salganea esakii (Dictyoptera, Blaberidae, Panesthiinae). Entomol. Sci. 14, 9–12. doi: 10.1111/j.1479-8298.2010.00406.x
Sieber, K. B., Bromley, R. E., and Hotopp, J. C. D. (2017). Lateral gene transfer between prokaryotes and eukaryotes. Exp. Cell Res. 358, 421–426. doi: 10.1016/j.yexcr.2017.02.009
Slaytor, M. (1992). Cellulose digestion in termites and cockroaches: what role do symbionts play? Comp. Biochem. Physiol. B. Comp. Biochem. 103, 775–784. doi: 10.1016/0305-0491(92)90194-v
Sleigh, M. A. (2000). “Trophic strategies,” in The Flagellates: Unity, Diversity And Evolution, eds B. Leadbeater, and J. Green (Milton Park: Taylor and Francis), 147-165.
Sugio, K., Shimojo, K., Isozaki, J., Itosu, W., Tsuha, A., Kakazu, S., et al. (2006). Distribution of cellulase activity in the salivary glands and the guts of pseudoworkers and soldiers of the drywood-feeding termite Neotermes koshunensis (Shiraki) and the effect of defaunation. Jap. J. Appl. Entomol. Zool. 50, 1–6. doi: 10.1303/jjaez.2006.1
Tachezy, J., and Šmíd, O. (2008). “Mitosomes in parasitic protists,” in Hydrogenosomes and Mitosomes: Mitochondria of Anaerobic Eukaryotes, ed. J. Tachezy (Berlin: Springer), 201–230. doi: 10.1007/7171_2007_113
Tai, V., Carpenter, K. J., Weber, P. K., Nalepa, C. A., Perlman, S. J., and Keeling, P. J. (2016). Genome evolution and nitrogen fixation in bacterial ectosymbionts of a protist inhabiting wood-feeding cockroaches. Appl. Environ. Microbiol. 82, 4682–4695. doi: 10.1128/AEM.00611-16
Tai, V., James, E. R., Nalepa, C. A., Scheffrahn, R. H., Perlman, S. J., and Keeling, P. J. (2015). The role of host phylogeny varies in shaping microbial diversity in the hindguts of lower termites. Appl. Environ. Microbiol. 81, 1059–1070. doi: 10.1128/AEM.02945-14
Thong-On, A., Suzuki, K., Noda, S., Inoue, J.-I., Kajiwara, S., and Ohkuma, M. (2009). Isolation and characterization of anaerobic bacteria for symbiotic recycling of uric acid nitrogen in the gut of various termites. Microbes Environ 27, 186–192. doi: 10.1264/jsme2.me11325
Tipton, L., Darcy, J. L., and Hynson, N. A. (2019). A developing symbiosis: enabling cross-talk between ecologists and microbiome scientists. Front. Microbiol. 10:292. doi: 10.3389/fmicb.2019.00292
Todaka, N., Inoue, T., Saita, K., Ohkuma, M., Nalepa, C. A., Lenz, M., et al. (2010). Phylogenetic analysis of cellulolytic enzyme genes from representative lineages of termites and a related cockroach. Plos One 5:10. doi: 10.1371/journal.pone.0008636
Tokuda, G., Kihara, K., Saitou, S., Moriya, S., Lo, N., et al. (2014). Metabolomic profiling of C-13 labelled cellulose digestion in a lower termite: insights into gut symbiont function. Proc. Roy. Soc. B-Biol. Sci. 281:20140990. doi: 10.1098/rspb.2014.0990
Treitli, S. C., Kolisko, M., Husník, F., Keeling, P. J., and Hampl, V. (2019). Revealing the metabolic capacity of Streblomastix strix and its bacterial symbionts using single-cell metagenomics. Proc. Nat. Acad. Sci. U.S.A. 116, 19675–19684. doi: 10.1073/pnas.1910793116
Treitli, S. C., Kotyk, M., Yubuki, N., Jirounkova, E., Vlasakova, J., Smejkalova, P., et al. (2018). Molecular and morphological diversity of the oxymonad genera Monocercomonoides and Blattamonas gen. nov. Protist 169, 744–783. doi: 10.1016/j.protis.2018.06.005
Utami, Y. D., Kuwahara, H., Igai, K., Murakami, T., Sugaya, K., Morikawa, T., et al. (2018). Genome analyses of uncultured TG2/ZB3 bacteria in ‘Margulisbacteria’ specifically attached to ectosymbiotic spirochetes of protists in the termite gut. ISME J. 13:455. doi: 10.1038/s41396-018-0297-4
Vickerman, K. (2000). “Adaptations to parasitism among flagellates,” in The Flagellates: Unity, Diversity and Evolution, eds B. Leadbeater, and J. Green (Milton Park: Taylor and Francis), 190–215.
Wada-Katsumata, A., Zurek, L., Nalyanya, G., Roelofs, W. L., Zhang, A. J., and Schal, C. (2015). Gut bacteria mediate aggregation in the German cockroach. Proc. Nat. Acad. Sci., U.S.A. 112, 15678–15683. doi: 10.1073/pnas.1504031112
Watanabe, H., and Tokuda, G. (2010). Cellulolytic systems in insects. Ann. Rev. Entomol. 55, 609–632. doi: 10.1146/annurev-ento-112408-085319
Weiblen, G. D., and Treiber, E. L. (2015). “Evolutionary origins and diversification of mutualism,” in Mutualism, ed. J. L. Bronstein (Oxford: Oxford University Press), 37–56.
Wein, T., Romero Picazo, D., Blow, F., Woehle, C., Jami, E., Reusch, T. B. H., et al. (2019). Currency, exchange, and inheritance in the evolution of symbiosis. Trends Microbiol. 27, 836–849. doi: 10.1016/j.tim.2019.05.010
Wenrich, D. (1935). Host-parasite relations between parasitic protozoa and their hosts. Proc. Amer. Philos. Soc. 75, 605–650.
Wilson, E (1975). Sociobiology. The New Synthesis. Cambridge, MA: Belknap Press of Harvard University Press.
Yamamura, N. (1993). Vertical transmission and evolution of mutualism from parasitism. Theor. Popul. Biol. 44, 95–109. doi: 10.1006/tpbi.1993.1020
Yuki, M., Kuwahara, H., Shintani, M., Izawa, K., Sato, T., Starns, D., et al. (2015). Dominant ectosymbiotic bacteria of cellulolytic protists in the termite gut also have the potential to digest lignocellulose. Environ. Microbiol. 17, 4942–4953. doi: 10.1111/1462-2920.12945
Keywords: parasitism, symbiosis, Cryptocercus, evolution, lignocellulose digestion, commensalism
Citation: Nalepa CA (2020) Origin of Mutualism Between Termites and Flagellated Gut Protists: Transition From Horizontal to Vertical Transmission. Front. Ecol. Evol. 8:14. doi: 10.3389/fevo.2020.00014
Received: 25 October 2019; Accepted: 17 January 2020;
Published: 06 February 2020.
Edited by:
Peter H. W. Biedermann, Julius Maximilian University of Würzburg, GermanyReviewed by:
Renate Radek, Free University of Berlin, GermanyPaul Eggleton, Natural History Museum, United Kingdom
Copyright © 2020 Nalepa. This is an open-access article distributed under the terms of the Creative Commons Attribution License (CC BY). The use, distribution or reproduction in other forums is permitted, provided the original author(s) and the copyright owner(s) are credited and that the original publication in this journal is cited, in accordance with accepted academic practice. No use, distribution or reproduction is permitted which does not comply with these terms.
*Correspondence: Christine A. Nalepa, Y2hyaXN0aW5lX25hbGVwYUBuY3N1LmVkdQ==