- Swiss Ornithological Institute, Sempach, Switzerland
Rodents are classical model species to investigate spatial synchrony in population fluctuation. Yet, previous studies have been strongly biased geographically toward high latitude (boreal ecosystem) and limited in their spatial scale, i.e., few sampling sites separated by a few tens of kilometers. Both aspects currently limit our understanding of rodent population dynamics across space. In this study we investigate vole population synchrony at a large spatial scale in central Europe. We used long-term breeding success of a vole-eating raptor specialist, the European kestrel, as an indicator of vole abundance. We first demonstrate that the productivity of kestrels is highly dependent on the availability of voles and as such is a good proxy of vole abundance. Secondly, we assessed the spatial synchrony of kestrel productivity and its scaling. We found that kestrel productivity fluctuated synchronously at a large spatial scale, up to a distance of 300 km. This result suggests that vole populations in central Europe varied in synchrony at large spatial scales, similarly as in northern latitudes. The most likely mechanism resulting in such large scale synchrony of vole populations is synchronized density-independent environmental conditions.
Introduction
Spatial population synchrony, i.e., the fact that populations in different locations exhibit correlated fluctuations, has been subject to intensive research since the early-stage of ecological research (Elton, 1924; Moran, 1953). For a given species, spatial population synchrony may occur as a consequence of density-independent synchronized environmental conditions (i.e., Moran effect; Moran, 1953; Hudson and Cattadori, 1999), individual dispersal among populations (Bjørnstad et al., 1999; Koenig, 1999) or due to the synchronizing effect of density-dependent trophic interaction, e.g., highly mobile predators or parasite–host systems (Norrdahl and Korpimäki, 1996; Koenig, 1999). Over the last decade studies have tried to disentangle the respective contribution of these three mechanisms to population synchrony (Bjørnstad et al., 1999; Liebhold et al., 2004). It appeared that their relative importance is largely a matter of scale and location. Although it is generally accepted that the Moran effect is dominating the overall level of synchrony, dispersal could be non-negligible at small spatial scales (Sutcliffe et al., 1996; Heino et al., 1997; Hudson and Cattadori, 1999; Bellamy et al., 2003; Pardikes et al., 2017). The underlying mechanism driving spatial population synchrony may also change according to location. For instance, while predators are likely to be a key factor for vole population synchrony in southeastern Norway (Ims and Andreassen, 2000), they hardly have any effect on vole populations in northern England (Petty et al., 2000; Graham and Lambin, 2002). As a consequence, it is necessary to study population synchrony at different locations and spatial scales if we want to reach a comprehensive understanding of mechanisms driving population dynamics (Oli, 2019).
Arvicoline species (voles and lemmings) are historical model species in the study of spatial population synchrony (Elton, 1924; Bjørnstad et al., 1999). However, our current understanding of population synchrony in this taxon is still incomplete owing to two major limiting factors. First, while many studies have investigated rodent population dynamics locally, far less have investigated dynamics at larger spatial scales (i.e., >100 km, but see Huitu et al., 2008) due to the financial and logistical difficulties associated with such population monitoring (Steen et al., 1996). Second, our understanding of arvicoline population dynamics is strongly biased toward high latitude in boreal and tundra ecosystems with very few studies from lower latitudes (Steen et al., 1996; Ims and Andreassen, 2000; Angerbjörn et al., 2001; Krebs et al., 2002; Huitu et al., 2003b, 2008; Cheveau et al., 2004; Sundell et al., 2004).
In this study we investigated vole population synchrony at a large spatial scale in central Europe (Switzerland). We challenge the difficulty in obtaining large-scale vole abundance data by studying the breeding performance of a raptor species specialized in voles, the European kestrel Falco tinnunculus. Because diurnal raptors and owls specialized in rodents are strongly dependent on the availability of their prey, their breeding success or population density are very good indicators of the population dynamics of small mammals (Elton, 1924; Petty et al., 2000; Cheveau et al., 2004; Sundell et al., 2004; Solonen et al., 2015), and have already previously been used to investigate population synchrony of voles (Sundell et al., 2004). We first demonstrate the strong relationship between vole availability and kestrel breeding performance in our study area. Then we study variation in kestrel breeding performance at the scale of entire Switzerland to provide new insights into vole spatial population synchrony in central Europe.
Materials and Methods
Study Area and Data Collection
The study was carried out on the Swiss central plateau with a maximal extension of about 300 km from south-west (Geneva) to north-east (St-Gallen). The area is dominated by open agricultural landscape (Figure S1). We use data collected between 2008 and 2018 from a total of 6,187 broods that occurred in 879 nest boxes (Figure 1). Volunteers monitored kestrel broods and recorded the number of chicks reaching the ringing age in successful broods. Kestrel breeding performance is known to be strongly related to vole abundance (Village, 1990; Korpimäki and Norrdahl, 1991). To check the validity of this relationship in our study area, fresh prey remains in nest boxes have been quantified in a part of the study area (Figure S1). The total number of voles and birds found each year in nest boxes at the ringing time were recorded (n = 1,641). These data were used to estimate the relative abundance of voles in the kestrel diet which is known to mirror the relative densities of the voles in the field (Village, 1982; Korpimäki, 1986). Among vole items identified at the species level (n = 615), Microtus arvalis was by far the most abundant species representing 86% of the prey. The other identified species were Arvicola amphibious, Microtus agrestis, and Clethrionomys glareolus.
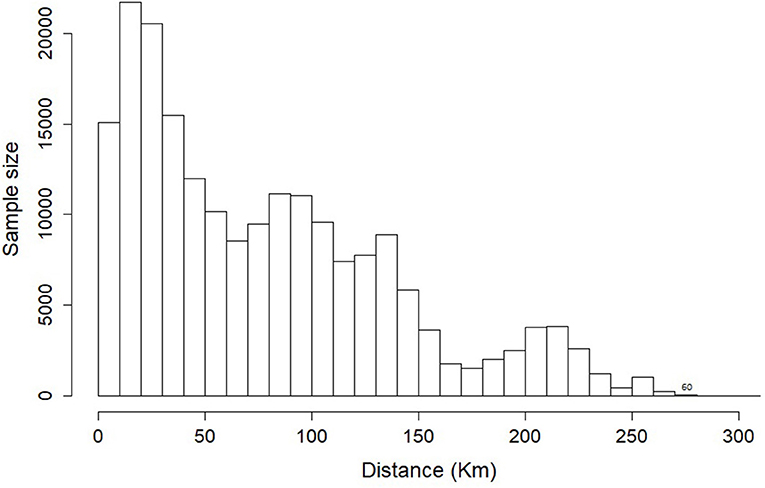
Figure 1. Number of unique pairs of nestbox comparisons by 10 km distance classes. Distances from 280 to 310 km were pooled into a single class due to small sample size.
Relationship Between Productivity and Vole Abundance
We modeled productivity, defined as the number of chicks at the ringing age in successful broods, using a Normal distribution: where Ψi, t is the observed productivity in nest box i in year t, δt is the estimated average productivity in year t and is the residual variance. We used a Normal instead of a Poisson distribution because only the former fitted the observed data well. Voles are the preferred prey of kestrels whereas birds are more opportunistically hunted and mostly when vole abundance is low, since they are more difficult to catch (Village, 1990). Thus, we used the proportion of voles in prey remains as an index of the annual vole abundance. We modeled the number of voles in prey remains using a Binomial model: N.volet ~ Bin(γt, N.preyt) where N.volet is the number of voles counted in prey remains in year t, N.preyt is the total number of prey remains (both birds and voles) counted in year t, and γt is the estimated index of vole abundance in year t. Finally, the relationship between productivity and estimated vole abundance is tested in a joint linear model:
where δt is the estimated average productivity in year t, β0 is the intercept, β1 the slope describing the relationship between productivity and the estimated index of the vole abundance (γt) in year t and is the residual term that we assumed to be normally distributed with mean 0 and variance .
Spatial Synchrony and Relationship With Distance
We used pairwise temporal correlations of productivity between nest boxes as a measure of synchrony in kestrel productivity to infer vole population synchrony. Given the number of nest boxes (n = 879) we could potentially estimate 385,881 pairwise temporal correlations of productivity. However, not all nest boxes were occupied or successful in each year. We only estimated such pairwise temporal correlation when productivity was available for at least three common years. For these reasons the final number of pairwise correlation was reduced to 199,502 (Figure 1). We estimated pairwise correlations of productivity between nest boxes using a hierarchical model derived from the residual decomposition technique (Johnson and Hoeting, 2003). First, for each nest box, we computed the standardized productivity as follows:
where Ψi, t is the observed productivity in nest box i in year t, μi is the average productivity in nest box i over the study duration and σi is the standard deviation of the productivity in nest box i over the study duration.
To estimate the pairwise temporal correlation of productivity between nest boxes i and j, we expressed the standardized productivity in nest box i in year t as a linear effect of the standardized productivity in nest box j the same year.
where cori,j is the pairwise temporal correlation of productivity between nest boxes i and j and εi,j,t is the residual that we assumed to be normally distributed with mean 0 and variance .
Finally, the pairwise temporal correlation is expressed as a linear effect of distance:
where β0 is the intercept, β1 is the slope describing the relationship between pairwise temporal correlations (cori,j) and the standardized distance between these nest boxes (di, j) and is the residual that we assumed to be normally distributed with mean 0 and variance . We considered both linear and quadratic relationships.
Models were fitted with a Bayesian approach using Markov Chain Monte Carlo (MCMC) techniques in software JAGS (Plummer, 2003). We used vague Normal prior distributions for the regression coefficients, a Uniform prior distribution between −1 and 1 for correlation coefficient and a Uniform prior distribution for the standard deviation of the random effect (Appendix S1).
Results
The proportion of voles in prey remains showed strong variation over the years from 30 to 85% (Figure S2). This index of vole abundance was strongly positively related to productivity (0.56 [0.40, 0.72], Figure 2), explaining 86% of the temporal variability. Thus, we consider kestrel productivity to be a reliable predictor of vole abundance in our study area.
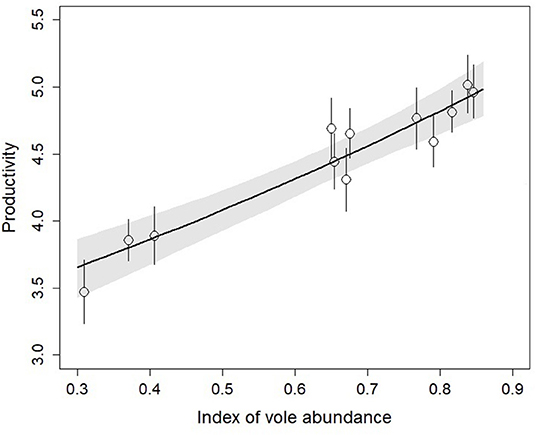
Figure 2. Relationship between the index of vole abundance and productivity of kestrels from 2007 to 2018. Open circles show productivity estimates obtained from a model with a fixed time effect and the bold line shows the productivity estimates based on a linear function of the index of vole abundance. Vertical line and the shaded area show ±95% CRI.
Mean pairwise correlation of kestrel productivity between nest boxes was strictly positive (95% CRI [0.12, 0.13]). This indicates that kestrel productivity and thus vole dynamics showed consistent fluctuations at the scale of Switzerland. Furthermore, the correlation of productivity between nests clearly declined with distance following a quadratic form (Table 1). Positive synchrony persisted over large distances, decreased by 50% around 200 km and vanished at around 300 km (Figure 3).
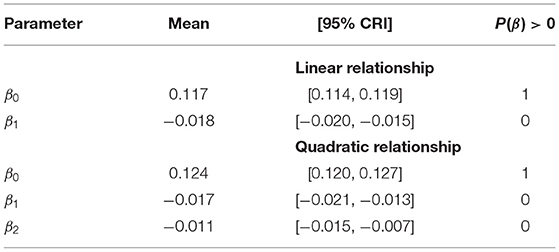
Table 1. Parameters estimates of the regression of the correlation in kestrel productivity against distance between pairs of nest-boxes.
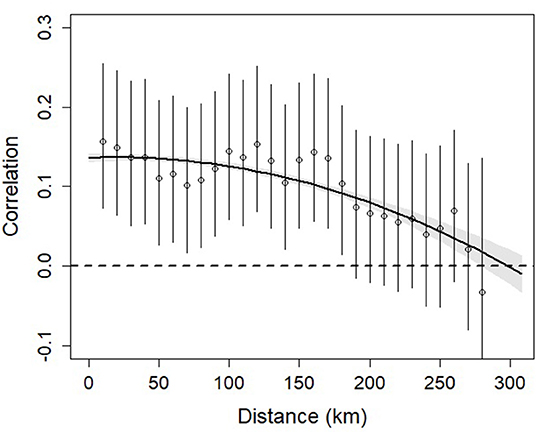
Figure 3. Correlation in kestrel productivity against distance between pairs of nest-boxes. Open circles show the correlation estimated by 10 km distance classes. Distances from 280 to 310 km were pooled into a single class due to sample size. The bold line shows the correlation in kestrel productivity estimates based on a quadratic function of the distance between pairs of nest-boxes. Vertical lines and the shaded areas show ±95% CRI.
Discussion
Our study suggests that vole population dynamics in central Europe are synchronized at a large spatial scale, i.e., until 300 km, but the strength of synchrony declines with increasing distance. While different mechanisms could have generated this pattern we suggest that correlated environmental condition is the most likely underlying causal factor.
Large Scale Vole Population Synchrony
The spatial scaling of synchrony of kestrel productivity reported in this study suggests that vole populations fluctuate synchronously over large distances in Switzerland (until 300 km). Previous studies reported population synchrony of voles at only medium distances, i.e., from 10 to 50 km (Steen et al., 1996; Bjørnstad et al., 1999; MacKinnon et al., 2001; Berthier et al., 2014), whereas others suggested synchrony at larger spatial scales. However, studies conducted at larger spatial scales were generally unable to identify at which distance the positive correlation vanished because of the limited size of the study area, i.e., up to 60 km (Lambin et al., 2006), 80 km (Huitu et al., 2003b), 120 km (Huitu et al., 2008), or 250 km (Gouveia et al., 2016). In the current study we used the breeding performance of a raptor specialized in voles to infer vole population synchrony with the advantage that data could more easily be collected over large spatial scales. We were able to identify the distance upon which synchrony stopped, which was around 300 km. Using a similar approach, Sundell et al. (2004) found that vole population synchrony in Finland vanish at around 500 km, demonstrating that a study should cover a huge area to be able to identify the extent of population synchrony in this taxon. Our study also confirms that large scale vole population synchrony is not limited to northern ecosystems but is also present at lower latitudes (Lambin et al., 2006; Gouveia et al., 2016).
What May Cause Large-Scale Population Synchrony of Voles in Switzerland?
Our results suggest that vole population exhibit large scale (i.e., 100–300 km) spatial synchrony in Switzerland. Hypotheses explaining spatial synchrony are usually based on three classes of mechanisms: (1) individual dispersal, (2) density-dependent trophic interactions and (3) environmental conditions (i.e., Moran effect). The dispersal ability of small mammals is strongly limited in space. The average dispersal distance of voles roughly ranges from 10 m to 2 km (Le Galliard et al., 2012). Even considering sequential dispersal with several generations within a year, individual movements produce spatial patterns that are limited to local scales (Sherratt et al., 2000; MacKinnon et al., 2001). Two populations at distances of more than 20 km are generally assumed to be unconnected by local dispersal (Bjørnstad et al., 1999; Krebs et al., 2002) suggesting that dispersal cannot synchronize population size across large spatial areas (i.e., 100–300 km) as described in this study (Ims and Andreassen, 2005; Zhang et al., 2015). In rodent population dynamics, the trophic interaction mechanism (2) is often limited to the predator hypothesis, i.e., mobile predators may induce spatial synchrony in vole populations. Nomadic birds of prey are typically highly mobile predators that are expected to synchronize vole population in Scandinavia (Norrdahl and Korpimäki, 1996; Ims and Andreassen, 2000). However such aggregating avian predators as for example Asio flammeus, Buteo lagopus, Circus sp., are absent from our study area and local predators i.e., F. tinnunculus, Buteo buteo, Asio otus, do not show nomadic behavior like in Fennoscandia. Other predators such as mustelids may also prey heavily on voles. However, mammals are less mobile than birds and their numerical response to variation in local prey abundance has a time lag of at least 1 year (Gilg et al., 2003). Mammalian predators appear therefore ineffective to synchronize vole populations distant by hundreds of kilometers within a single year. Thus the predator hypothesis seems inoperable in our study area supporting the idea that predators are not synchronizing populations everywhere (Petty et al., 2000; Graham and Lambin, 2002; Lambin et al., 2006). Finally, among the synchronizing mechanisms, only the third involving density independent environmental effects (3) can satisfactorily explain the large scale vole population synchrony observed in our study area. This is in line with others studies conducted on vole and lemming populations (Bjørnstad et al., 1999; Angerbjörn et al., 2001; Krebs et al., 2002; Huitu et al., 2008). Climatic variables such as precipitation and temperature show large scaling of synchrony (up to continental scale; Koenig, 2002) that easily includes our complete study area (Figure S3). Furthermore, weather is an important factor of vole population regulation (Tkadlec et al., 2006). Effects of weather on vole demography is expected to be mainly indirectly mediated by plant productivity, i.e., food resource, which can be particularly limiting in winter (Hansen et al., 1999; Huitu et al., 2003a; Korslund and Steen, 2006; Tkadlec et al., 2006). Nutritional deficits during winter have been associated to a poor physiological condition in voles and to the decreasing phase of their population cycles (Huitu et al., 2007). Vole physiology is not adapted to food deprivation and fasting longer than some hours can lead to death (Mustonen et al., 2008). To conclude, although these mechanisms are not mutually exclusive, environmental effects are likely to be the key factor both necessary and sufficient to generate large scale synchrony of vole populations in Switzerland.
The Use of Breeding Performance of Raptors to Infer Vole Population Dynamics
The use of the breeding performance of a raptors can be a powerful approach to investigate patterns of prey population fluctuations across space (Sundell et al., 2004). This approach is particularly efficient regarding data collection. While productivity of raptors can be assessed by one or two nest controls, the direct estimation of vole abundance requires much more intense data collection (Steen et al., 1996; Lambin et al., 2006). However, the quality of data collected using the two approaches is not identical and the indirect approach as used here has some limitations. Raptors breeding performances reflect prey availability with a low temporal resolution, i.e., only a proxy of vole abundance over the duration of breeding season is obtained. Owing to their high mobility, raptors provide also information on vole abundance with a lower spatial resolution making the investigation of local and continuous process such as traveling wave not workable. Furthermore, even in a prey specialist the breeding performance is not completely determined by prey abundance but is affected also by additional factors. Thus, this indirect method provides more crude information compared to the direct estimation of vole abundance. Despite these limitations we think that breeding performance of raptors constitutes a useful complementary source of information to infer prey population dynamics especially at large spatial scales. This approach is currently underused and we hope it will be applied more often in the future to obtain further insights on prey population dynamics.
Data Availability Statement
The datasets generated for this study are available on request to the corresponding author.
Ethics Statement
Ethical review and approval was not required for the animal study because this study has been conducted in accordance with the Swiss legislation.
Author Contributions
SM, JJ, and JL coordinated data collection and management. RF and MS analyzed the data. RF led the writing of the manuscript. All authors contributed to the drafts and gave final approval for publication, conceived the ideas, and designed the methodology.
Funding
This work was funded by the Swiss Ornithological Institute.
Conflict of Interest
The authors declare that the research was conducted in the absence of any commercial or financial relationships that could be construed as a potential conflict of interest.
Acknowledgments
We are very grateful to all the volunteers who installed the nest boxes and collected data on productivity. This study would not have been possible without their great work. We acknowledge Martin Spiess, Markus Rudin†, Pierre-Alain Ravussin, and Alexandre Roulin for their collaboration to initiate this project.
Supplementary Material
The Supplementary Material for this article can be found online at: https://www.frontiersin.org/articles/10.3389/fevo.2019.00512/full#supplementary-material
Footnote
†^Passed away
References
Angerbjörn, A., Tannerfeldt, M., and Lundberg, H. (2001). Geographical and temporal patterns of lemming population dynamics in Fennoscandia. Ecography 24, 298–308. doi: 10.1034/j.1600-0587.2001.240307.x
Bellamy, P. E., Rothery, P., and Hinsley, S. A. (2003). Synchrony of woodland bird populations: the effect of landscape structure. Ecography 26, 338–348. doi: 10.1034/j.1600-0587.2003.03457.x
Berthier, K., Piry, S., Cosson, J.-F., Giraudoux, P., Foltête, J.-C., Defaut, R., et al. (2014). Dispersal, landscape and travelling waves in cyclic vole populations. Ecol. Lett. 17, 53–64. doi: 10.1111/ele.12207
Bjørnstad, O. N., Ims, R. A., and Lambin, X. (1999). Spatial population dynamics: analyzing patterns and processes of population synchrony. Trends Ecol. Evol. 14, 427–432. doi: 10.1016/S0169-5347(99)01677-8
Cheveau, M., Drapeau, P., Imbeau, L., and Bergeron, Y. (2004). Owl winter irruptions as an indicator of small mammal population cycles in the boreal forest of eastern North America. Oikos 107, 190–198. doi: 10.1111/j.0030-1299.2004.13285.x
Elton, C. S. (1924). Periodic fluctuations in the numbers of animals: their causes and effects. Br. J. Exp. Biol. 2, 119–163.
Gilg, O., Hanski, I., and Sittler, B. (2003). Cyclic dynamics in a simple vertebrate predator-prey community. Science 302, 866–868. doi: 10.1126/science.1087509
Gouveia, A. R., Bjørnstad, O. N., and Tkadlec, E. (2016). Dissecting geographic variation in population synchrony using the common vole in central Europe as a test bed. Ecol. Evol. 6, 212–218. doi: 10.1002/ece3.1863
Graham, I. M., and Lambin, X. (2002). The impact of weasel predation on cyclic field-vole survival: the specialist predator hypothesis contradicted. J. Anim. Ecol. 71, 946–956. doi: 10.1046/j.1365-2656.2002.00657.x
Hansen, T. F., Stenseth, N. C., and Henttonen, H. (1999). Multiannual vole cycles and population regulation during long winters: an analysis of seasonal density dependence. Am. Nat. 154, 129–139. doi: 10.1086/303229
Heino, M., Kaitala, V., Ranta, E., and Lindström, J. (1997). Synchronous dynamics and rates of extinction in spatially structured populations. Proc. R. Soc. Lond. Ser. B Biol. Sci. 264, 481–486. doi: 10.1098/rspb.1997.0069
Hudson, P. J., and Cattadori, I. (1999). The Moran effect: a cause of population synchrony. Trends Ecol. Evol. 14, 1–2. doi: 10.1016/S0169-5347(98)01498-0
Huitu, O., Jokinen, I., Korpimäki, E., Koskela, E., and Mappes, T. (2007). Phase dependence in winter physiological condition of cyclic voles. Oikos 116, 565–577. doi: 10.1111/j.0030-1299.2007.15488.x
Huitu, O., Koivula, M., Korpimäki, E., Klemola, T., and Norrdahl, K. (2003a). Winter food supply limits growth of northern vole populations in the absence of predation. Ecology 84, 2108–2118. doi: 10.1890/02-0040
Huitu, O., Laaksonen, J., Klemola, T., and Korpimäki, E. (2008). Spatial dynamics of microtus vole populations in continuous and fragmented agricultural landscapes. Oecologia 155, 53–61. doi: 10.1007/s00442-007-0885-x
Huitu, O., Norrdahl, K., and Korpimäki, E. (2003b). Landscape effects on temporal and spatial properties of vole population fluctuations. Oecologia 135, 209–220. doi: 10.1007/s00442-002-1171-6
Ims, R. A., and Andreassen, H. P. (2000). Spatial synchronization of vole population dynamics by predatory birds. Nature 408:194. doi: 10.1038/35041562
Ims, R. A., and Andreassen, H. P. (2005). Density-dependent dispersal and spatial population dynamics. Proc. R. Soc. B Biol. Sci. 272, 913–918. doi: 10.1098/rspb.2004.3025
Johnson, D. S., and Hoeting, J. A. (2003). Autoregressive models for capture-recapture data: a bayesian approach. Biometrics 59, 341–350. doi: 10.1111/1541-0420.00041
Koenig, W. D. (1999). Spatial autocorrelation of ecological phenomena. Trends Ecol. Evol. 14, 22–26. doi: 10.1016/S0169-5347(98)01533-X
Koenig, W. D. (2002). Global patterns of environmental synchrony and the moran effect. Ecography 25, 283–288. doi: 10.1034/j.1600-0587.2002.250304.x
Korpimäki, E. (1986). Diet variation, hunting habitat and reproductive output of the kestrel Falco tinnunculus in the light of the optimal diet theory. Ornis Fennica 63, 84–90.
Korpimäki, E., and Norrdahl, K. (1991). Numerical and functional responses of kestrels, short-eared owls, and long-eared owls to vole densities. Ecology 72, 814–826. doi: 10.2307/1940584
Korslund, L., and Steen, H. (2006). Small rodent winter survival: snow conditions limit access to food resources. J. Anim. Ecol. 75, 156–166. doi: 10.1111/j.1365-2656.2005.01031.x
Krebs, C. J., Kenney, A. J., Gilbert, S., Danell, K., Angerbjörn, A., Erlinge, S., et al. (2002). Synchrony in lemming and vole populations in the Canadian Arctic. Can. J. Zool. 80, 1323–1333. doi: 10.1139/z02-120
Lambin, X., Bretagnolle, V., and Yoccoz, N. G. (2006). Vole population cycles in northern and southern Europe: is there a need for different explanations for single pattern? J. Anim. Ecol. 75, 340–349. doi: 10.1111/j.1365-2656.2006.01051.x
Le Galliard, J.-F., Remy, A., Ims, R. A., and Lambin, X. (2012). Patterns and processes of dispersal behaviour in arvicoline rodents. Mol. Ecol. 21, 505–523. doi: 10.1111/j.1365-294X.2011.05410.x
Liebhold, A., Koenig, W. D., and Bjørnstad, O. N. (2004). Spatial synchrony in population dynamics. Annu. Rev. Ecol. Evol. Syst. 35, 467–490. doi: 10.1146/annurev.ecolsys.34.011802.132516
MacKinnon, J. L., Petty, S. J., Elston, D. A., Thomas, C. J., Sherratt, T. N., and Lambin, X. (2001). Scale invariant spatio-temporal patterns of field vole density. J. Anim. Ecol. 70, 101–111. doi: 10.1046/j.1365-2656.2001.00479.x
Moran, P. A. (1953). The statistical analysis of the Canadian lynx cycle. Aust. J. Zool. 1, 291–298. doi: 10.1071/ZO9530291
Mustonen, A.-M., Saarela, S., and Nieminen, P. (2008). Food deprivation in the common vole (Microtus arvalis) and the tundra vole (Microtus oeconomus). J. Comp. Physiol. B 178, 199–208. doi: 10.1007/s00360-007-0213-0
Norrdahl, K., and Korpimäki, E. (1996). Do nomadic avian predators synchronize population fluctuations of small mammals? Field Exp. Oecol. 107, 478–483. doi: 10.1007/BF00333938
Oli, M. K. (2019). Population cycles in voles and lemmings: state of the science and future directions. Mamm. Rev. 49, 226–239. doi: 10.1111/mam.12156
Pardikes, N. A., Harrison, J. G., Shapiro, A. M., and Forister, M. L. (2017). Synchronous population dynamics in California butterflies explained by climatic forcing. R. Soc. Open Sci. 4:170190. doi: 10.1098/rsos.170190
Petty, S. J., Lambin, X., Sherratt, T. N., Thomas, C. J., Mackinnon, J. L., Coles, C. F., et al. (2000). Spatial synchrony in field vole Microtus agrestis abundance in a coniferous forest in northern England: the role of vole-eating raptors. J. Appl. Ecol. 37, 136–147. doi: 10.1046/j.1365-2664.2000.00473.x
Plummer, M. (2003). “JAGS: a program for analysis of bayesian graphical models using gibbs sampling,” in Proceedings of the 3rd International Workshop on Distributed Statistical Computing (DSC 2003), eds K. Hornik, F. Leisch, and A. Zeileis (Vienna), 1–10.
Sherratt, T. N., Lambin, X., Petty, S. J., Mackinnon, J. L., Coles, C. F., and Thomas, C. J. (2000). Use of coupled oscillator models to understand synchrony and travelling waves in populations of the field vole Microtus agrestis in northern England. J. Appl. Ecol. 37, 148–158. doi: 10.1046/j.1365-2664.2000.00472.x
Solonen, T., Ahola, K., and Karstinen, T. (2015). Clutch size of a vole-eating bird of prey as an indicator of vole abundance. Environ. Monit. Assess. 187:588. doi: 10.1007/s10661-015-4783-0
Steen, H., Ims, R. A., and Sonerud, G. A. (1996). Spatial and temporal patterns of small-rodent population dynamics at a regional scale. Ecology 77, 2365–2372. doi: 10.2307/2265738
Sundell, J., Huitu, O., Henttonen, H., Kaikusalo, A., Korpimäki, E., Pietiäinen, H., et al. (2004). Large-scale spatial dynamics of vole populations in Finland revealed by the breeding success of vole-eating avian predators. J. Anim. Ecol. 73, 167–178. doi: 10.1111/j.1365-2656.2004.00795.x
Sutcliffe, O. L., Thomas, C. D., and Moss, D. (1996). Spatial synchrony and asynchrony in butterfly population dynamics. J. Anim. Ecol. 65, 85–95. doi: 10.2307/5702
Tkadlec, E., Zboril, J., Losík, J., Gregor, P., and Lisická, L. (2006). Winter climate and plant productivity predict abundances of small herbivores in central Europe. Clim. Res. 32, 99–108. doi: 10.3354/cr032099
Village, A. (1982). The diet of kestrels in relation to vole abundance. Bird Study 29, 129–138. doi: 10.1080/00063658209476747
Keywords: Falco tinnunculus, population dynamics, prey abundance, spatial synchrony, spatial autocorrelation
Citation: Fay R, Michler S, Laesser J, Jeanmonod J and Schaub M (2020) Large-Scale Vole Population Synchrony in Central Europe Revealed by Kestrel Breeding Performance. Front. Ecol. Evol. 7:512. doi: 10.3389/fevo.2019.00512
Received: 16 September 2019; Accepted: 17 December 2019;
Published: 21 January 2020.
Edited by:
Mauricio Lima, Pontifical Catholic University of Chile, ChileReviewed by:
Nigel Yoccoz, Arctic University of Norway, NorwayJuan Jose Luque-Larena, University of Valladolid, Spain
Copyright © 2020 Fay, Michler, Laesser, Jeanmonod and Schaub. This is an open-access article distributed under the terms of the Creative Commons Attribution License (CC BY). The use, distribution or reproduction in other forums is permitted, provided the original author(s) and the copyright owner(s) are credited and that the original publication in this journal is cited, in accordance with accepted academic practice. No use, distribution or reproduction is permitted which does not comply with these terms.
*Correspondence: Rémi Fay, ZmF5LnJlbWkmI3gwMDA0MDtnbWFpbC5jb20=