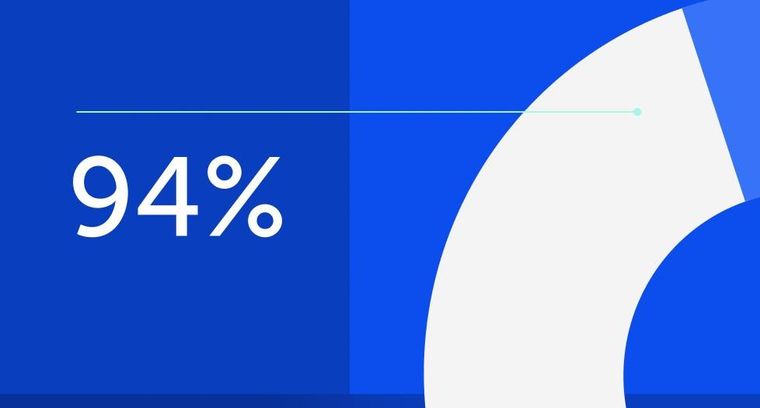
94% of researchers rate our articles as excellent or good
Learn more about the work of our research integrity team to safeguard the quality of each article we publish.
Find out more
ORIGINAL RESEARCH article
Front. Ecol. Evol., 13 December 2019
Sec. Biogeography and Macroecology
Volume 7 - 2019 | https://doi.org/10.3389/fevo.2019.00467
This article is part of the Research TopicEcological Development and Functioning of Biological Soil Crusts After Natural and Human DisturbancesView all 16 articles
Wildfires destabilize biocrust, requiring decades for most biological constituents to regenerate, but bacteria may recover quickly and mitigate the detrimental consequences of burnt soils. To evaluate the short-term recovery of biocrust bacteria, we tracked shifts in bacterial community form and function in Cyanobacteria/lichen-dominated (shrub interspaces) and Cyanobacteria/moss-dominated (beneath Artemisia tridentata) biocrusts 1 week, 2 months, and 1 year following a large-scale burn manipulations in a cold desert (Utah, USA). We found no evidence of the burned bacterial community recovering to a burgeoning biocrust. The foundational biocrust phyla, Cyanobacteria, dominated by Microcoleus viginatus (Microcoleaceae), disappeared from burned soils creating communities void of photosynthetic taxa. One year after the fire, the burned biocrust constituents had eroded away and the bare soils supported the formation of a convergent community of chemoheterotrophic copiotrophs regardless of location. The emergent community was dominated by a previously rare Planococcus species (family Planococcaceae, Firmicutes) and taxa in the Cellulomonadaceae (Actinobacteria), and Oxalobacteraceae (Betaproteobacteria). Previously burnt soils maintained similar levels of bacterial biomass, alpha diversity, and richness as unburned biocrusts, but supported diffuse, poorly-interconnected communities with 75% fewer species interactions. Nitrogen fixation declined at least 3.5-fold in the burnt soils but ammonium concentrations continued to rise through the year, suggesting that the exhaustion of organic C released from the fire, and not N, may diminish the longevity of the emergent community. Our results demonstrate that biocrust bacteria may recover rapidly after burning, albeit along a different community trajectory, as rare bacteria become dominant, species interconnectedness diminishes, and ecosystem services fail to rebound.
Fire may dramatically alter soil bacteria communities depending on the biome being burned (Pressler et al., 2019); fire characteristics [e.g., type (i.e., low-intensity vs. high-intensity, Xiang et al., 2014; Koster et al., 2016) and frequency (e.g., single vs. multiple, Hawkes and Flechtner, 2002; Guenon and Gros, 2013)], and depth of burned soil (Kim et al., 2004). Following the fire, burned communities begin to recover, with recovery defined as a return in biomass, community composition, and/or function to original levels prior to the disturbance. The rate of recovery, in any form, is moderated by a series of interacting factors, such as soil hydrophobicity (Faille et al., 2002; Fernelius et al., 2017), rainfall intensity and frequency (Guenon and Gros, 2013; Hinojosa et al., 2019), nutrient concentrations (Prendergast-Miller et al., 2017; Rodriguez et al., 2017), and soil erodibility (Williams et al., 2012). Within deserts, the impact of wildfires on soil bacteria is potentially immense. The surfaces of desert soils are often covered with biocrusts, which are complex mosaics of Cyanobacteria, other bacteria, green algae, lichens, mosses, and fungi. Biocrusts are autochthonally driven with photosynthate and fixed N2 from Cyanobacteria and organic C from other photosynthetic organisms creating a nutrient-rich zone, the “cyanosphere” (Couradeau et al., 2019; Warren et al., 2019) that supports a relatively high level of bacterial biomass and diversity (Chilton et al., 2018). However, these “living skins of the desert” occupy soil surfaces in close proximity to fuels (e.g., shrubs and grass litter, woody debris) that readily burn (Hilty et al., 2003; Balch et al., 2013) and biocrust constituents themselves are often desiccated and may burn during fire. If burned, biocrusts may lose the ability to armor soils against wind and water erosion (Eldridge and Leys, 2003; Rodriguez-Caballero et al., 2015), enhance hydrologic function (Chamizo et al., 2016), and fix N for chronically N-poor desert systems (Belnap, 2002). Taken together, the loss of biocrusts to fire may detrimentally alter desert ecosystem form and function.
Wildfires may kill many biocrust constituents, but bacteria, in particular Cyanobacteria, may recover more quickly. A lichen- or moss-dominated biocrust may require decades to fully recover depending on disturbance type, intensity, and precipitation variability (Johansen et al., 1984; Belnap, 2003; Root et al., 2017). However, soil bacteria are relatively resistant to fire even among other soil biota (e.g., fungi and mesofauna, Pressler et al., 2019). For example, surviving bacteria in burnt soils may enter a state of dormancy to weather the harsh conditions induced by fire. Dormancy is extremely common bet-hedging strategy, with upwards of 90% of microbial biomass and 50% of all bacterial taxa potentially being dormant at a given time (Alvarez et al., 1998; Lennon and Jones, 2011; Wang et al., 2014). Cyanobacteria may become dormant (Rajeev et al., 2013) and survive fires by potentially employing hydrotaxis to recolonize disturbed soils (Pringault and Garcia-Pichel, 2004). Besides dormancy, bioaerosols and unburned soils immediately below the burn may serve as seed banks to aid in biocrust recovery. Bioaerosols in dust harbor an immense diversity of bacteria (Choudoir et al., 2018; Dastrup et al., 2018) and may retain a taxonomical signature of the originating soil surfaces (Boose et al., 2016; Weil et al., 2017; Dastrup et al., 2018). Further, Microcoleus vaginatus, the foundational Cyanobacterium in many cold desert biocrusts (Garcia-Pichel et al., 2013), is a pioneering primary producer (Belnap, 2002) that may recolonize soils from dust, provide photosynthate, and shape the heterotrophic bacterial community. Soils millimeters below burnt soils harbor some of the same taxa (Steven et al., 2013; Maier et al., 2014) and may serve as inoculum for recovering biocrusts. In the short-term, bacteria components of biocrusts have the potential to recover relatively rapidly.
The recovering Cyanobacteria-dominated biocrust may mitigate the detrimental ecosystem consequences of burnt soil surfaces. Cyanobacteria colonize the top millimeters of soils, physically weaving soil particles together with sheathed filaments and, along with other bacteria, produce exopolymeric substances that glue soil particles together (Mazor et al., 1996; Costa et al., 2018). For example, foundational Cyanobacteria like M. vaginatus deters wind erosion (Kuske et al., 2012; Duniway et al., 2019) by rapidly proliferating filaments through unconsolidated surfaces ultimately increasing the threshold friction velocity of surface materials (Hu et al., 2002). Additionally, exopolymeric substance produced by cyanobacteria-dominated crust bacteria may enhances soil structure, improves infiltration (Costa et al., 2018), and may lower runoff and sediment loss due to increased soil aggregation (Faist et al., 2017). Alternatively, colonizing Cyanobacteria are often non-heterocystous incapable of performing N-fixation (Belnap, 1996; Yeager et al., 2007). Thus, once burgeoning Cyanobacteria-dominated biocrust communities mineralize and exhaust the residual unburned materials in soils (Prietofernandez et al., 1993), N availability may limit the ecosystem benefits of bacterial function.
In this study, we evaluated the potential for a burgeoning biocrust to recover and provide ecosystem services within a year following fire. We experimentally burned tracked the form and function of Cyanobacteria/lichen-dominated biocrusts and Cyanobacteria/moss-dominated biocrusts in a cold desert (UT, USA). Specifically, in a large-scale field manipulation, we burned the plant community and soil surfaces and evaluated shifts in bacterial community composition metrics such as richness, alpha diversity and taxa co-occurrence patterns 1 week, 2 months, and 1 year after the fire. We also measured N fixation rates and soil inorganic N availability, infiltration rates, and aggregate stability over the same time scale. The two biocrust forms occupied distinct locations across the landscape-Cyanobacteria/moss crust dominated the surfaces beneath and adjacent to canopies of Artemisia tridentata ssp. Wyomingensis shrub-islands, while Cyanobacteria/lichen crust dominated the shrub interspaces, which also supported a relatively low grass cover. We evaluated the cover of lichens, mosses, and surface cyanobacteria in burned and unburned plots. We hypothesized that, post-fire, a simplified Cyanobacteria-dominant crust will form from M. vaginatus and heterotrophic bacteria. We also hypothesized that bacterial biomass, richness, and diversity will approach unburned crust levels due to the resistance and resilience of soil bacteria to harsh conditions and high dispersal capabilities. Last, we hypothesized that within a year, along with a rudimentary crust, soil infiltration and stability, but not N-fixation, will begin to recover.
We conducted our study in the Great Basin Desert in Rush Valley, UT (40°05′27.43″N−112°18′18.24″W). Rugose crusts consisting of one moss, Syntrichia caninervis (9% cover) multiple cyanolichen and green algal lichen species (26% cover), and one Cyanobacterium, M. vaginatus (50% cover) were found in the shrub interspaces, while S. caninervis (6% cover) M. vaginatus (17% cover) and plant litter (70% cover) were found beneath shrubs. The shrub community was dominated by A. tridentata, ssp. Wyomingensis, and a native perennial grass Elymus elymoides (Raf.) Swezey. Mean annual precipitation (MAP) at the site is 27 cm year−1 [±1.5, n = 30, (mean and SEM) years 1978–2018] and mean annual temperature (MAT) is 8.8°C [±0.16, n = 30, years 1978–2018; Vernon Utah COOP Station 429133]. Based on limited climate data available from the station during our year-long experiment, the cold desert was slightly warmer and drier in the fall and winter than the 30-year mean. For example, monthly temperatures were within 1°C of MAT (months: October, November, August, September, and October), except in December when the mean daily temperatures was 3°C higher than MAT. Cumulative precipitation was a total of 2.5 cm lower in October, August, September, and October than the MAP levels for these same months. Soils were derived from Lake Bonneville sediments and are strongly alkaline. The series consists of well-drained, fine-loamy, mixed, mesic Xerollic Calciorthids with 3 to 15% calcium carbonate.
To investigate the post-fire response of biocrust bacteria, we created burned and unburned control plots. Treatments were assigned in a complete randomized block design for a total of 20 experimental plots (10 burned, 10 control plots), each 30 m width × 30 m length. Within each plot, we sampled two biocrust locations: Cyanobacteria/lichen-dominated crusts residing in interspaces ~30 cm away from a shrub (interspace); and, Cyanobacteria/moss-dominated crusts beneath A. tridentata at the edge of the shrub canopy (shrub). We sampled plots and crust locations 1 week (27th September), 2 months (4th November), and 1 year (1st October) following the fire. To facilitate a thorough and even burn, straw was spread onto the soil surface before burning (Esque et al., 2010). Also, the ash from burned straw blew away from soil surfaces within days, reducing the likelihood of long-term increases of soil C substrates or nutrients from burnt straw.
To evaluate the effects of fire on Cyanobacteria and heterotrophic bacteria, we characterized bacterial community composition using target-metagenomics based on the 16S rRNA gene. Bacterial communities were evaluated from a composite surface soil sample from three subsamples (2 cm width × 2 mm depth) with a soil corer (2 fire treatments × 2 biocrust microsites × 3 time points × 3 replicates = 36 samples). Subsamples were: composited by biocrust location and treatment combinations within a plot, flash frozen in the field with liquid N, and stored at −20°C until DNA analysis We extracted genomic DNA from 0.5 g of soil using the PowerSoil DNA Isolation Kit (MoBio, Carlsbad, CA) and amplified the V4–V5 region of the 16S rRNA gene using the bacterial specific primer set 515F and 806R with a 12-nt error correcting Golay barcodes (Aanderud et al., 2019). We used the following thermal cycle for PCR reactions: an initial denaturation step at 94°C for 3 min followed by 35 cycles of denaturation at 94°C for 45 s, annealing at 55°C for 30 s, and an extension at 72°C for 90 s. The amplified DNA was purified (Agencourt AMPure XP PCR Purification, Beckman Coulter Inc., Brea, CA, USA), pooled at approximately equimolar concentrations (Quant-iT PicoGreen dsDNA Kit, Invitrogen Corporation, Carlsbad, CA, USA), and sequenced at the Brigham Young University DNA Sequencing Center (http://dnasc.byu.edu/) using a 454 Life Sciences Genome Sequence FLX (Roche, Branford, CT, USA). We analyzed all sequences using mothur (v. 1.29.2) to remove barcodes and short reads, chimeras, and non-bacterial sequences (Schloss et al., 2009). Specifically, we excluded sequences < 260 bp with homopolymers longer than 8 bp, removed chimeras using UCHIME (Edgar et al., 2011), and eliminated chloroplast, mitochondria, archaeal, and eukaryotic 16S rRNA gene sequences based on reference sequences from the Ribosomal Database Project (Cole et al., 2009). We then aligned sequences against the SILVA database (silva.nr_v128.align; Pruesse et al., 2007) with the SEED aligner and created operational taxonomic units (OTUs) based on uncorrected pairwise distances using a minimum coverage of 99% and minimum pairwise sequence similarity of 97%.
To analyze shifts in bacterial communities following the burn manipulation, first, we used Principal Coordinates Analysis (PCoA) and permutational multivariate analyses of variance (PERMANOVA, Anderson, 2001). The PCoA was based on a Bray-Curtis distance matrix using the “vegan” package in R (R Development Core Team, 2017). The PCoA aided in the visualization of communities, but we tested for the main effects and interactions between burn treatment and time since the fire with PERMANOVA using the adonis function also in the vegan package of R. Second, we calculated the relative recovery (i.e., relative abundance) of 10 phyla and three subclasses to identify differences in the distribution of major taxonomical groups (recovery ≥ 1.0%) among the burned and unburned biocrust types through time. Next to further evaluated shifts in bacterial communities, taxonomic trends of 20 families (recovery ≥ 1.0% in at least one replicate) were visualized in a heat map with hierarchal clustering using the heatmap function in the “gplot” package in R. Last, we quantified the alpha diversity of communities as the inverse Shannon index and richness as the total number of OTUs based on 1,000 iterations of 900 random resampled sequences from each replicate. We examined differences in alpha diversity and richness among fire treatments and biocrust locations through time using two-way, repeated measures ANOVA (RM-ANOVA) in R.
To evaluate the recovery of bacterial biomass in the burn manipulations, we estimated abundance of bacteria using quantitative PCR and a universal bacterial 16S rRNA primer set [EUB 338 (forward) and Eub518 (reverse)] (Aanderud et al., 2018). In 12.5 μl reactions using KAPA2G Robust PCR Kits (KAPA Biosystems, Wilmington, MA, USA), we amplified targeted genes using the thermocycler condition: an initial denaturation step at 94°C for 3 min followed by 35 cycles of denaturation at 94°C for 45 s, anneal at 60°C for 30 s, and an extension at 72°C for 90 s. We generated qPCR standards from a crust soil bacterium using the TOPO TA Cloning Kit (Invitrogen) and extracted plasmids from transformed cells (Qiagen Sciences, Germantown, MD, USA). The coefficient of determination (r2) for our assay was 0.98, while amplification efficiency was 1.5. We evaluated shifts in biomass with two-way, RM-ANOVA in R.
To assess interactions among Cyanobacteria and other bacteria taxa, we created network co-occurrence models for the burned and unburned crust communities for each biocrust type based on maximal information coefficient (MIC) analysis. We calculated all possible linear and non-linear associations between OTUs using “Minerva” package in R, which belongs to a class of maximal information-based non-parametric exploration statistics for identifying and classifying relationships (Reshef et al., 2011). For all models, we included burned and unburned biocrust from the 2-month and 1-year sampling dates (n = 6 for each model), as the 1-week bacterial communities demonstrated little difference between the treatments in the PCoA. The nodes in the networks represented individual OTUs at 97% identity, while edges corresponded to valid or robust co-occurrence connections that occurred in at least 75% of all samples and had a MIC that was both > 0.7 and statistically significant (P-value = 0.01; Barberan et al., 2014). The filtering facilitated the determination of the core soil community responding to fire and removed poorly represented OTUs reducing network complexity. To describe the topology of the networks, we calculated the mean path length, mean degree, and modularity (Freedman et al., 2016). Network graphs in the graphml format were generated using “igraph” package in R and were visualized with Gephi (v. 0.8.2-beta).
To describe changes on the soil surface, we used a sixteen-point grid and a modified, step point-intercept transect technique (Bowker et al., 2008) to estimate the mean percent ground cover of Cyanobacteria biocrusts; species of cyanolichen, green algal lichen, and mosses; bare ground; plant material covering soil surfaces, and rock in burned and unburned treatments. The grids were placed in fixed locations for each sampling time point to more accurately re-evaluate crust components through time. Cyanobacterial biocrust cover was estimated visually, light and dark surface coloration, and structurally by dropping a pin onto the soil surface to ensure that the crust was in fact a crust created by cyanobacterial colonies weaving through soil surfaces (Rosentreter et al., 2007).
To determine the effects of fire on soil N inputs, we measured N fixation (μmol h−1 m−2) using the acetylene reduction assay (ARA) and evaluated soil ammonium (mg kg soil−1). The ARA assay was measured in intact soil core (2 cm width × 1 cm depth), while inorganic N was assessed from a composite surface soil sample from three subsamples (2 cm width × 2 mm depth) with a soil corer. Both N determinations were evaluated on all 10 field replicates through time (2 fire treatments × 2 biocrust microsites × 3 time points × 10 replicates = 120 samples). For ARA, we followed the protocols outlined by Belnap (2002). Briefly, we: incubated cores for 2 days on a 12 h light, 12 h dark schedule with daily water additions of 1 ml; sealed the cores and created a 10% acetylene atmosphere in the headspace by injecting 5 ml of pure acetylene through a septum with a gas-tight syringe; 4 h later, we removed a 4 ml headspace gas sample and measured the concentration (ppm) of ethylene with an Agilent Technologies 6890A gas chromatograph with a PoraPak R column (Agilent Technologies, Santa Clara, CA, USA) with an attached flame ionization detector. We used the ideal gas law to convert ppm ethylene to μmol ethylene h−1 m−2. We measured ammonium in soil extracts (2 g soil) with 4 ml 0.5 M K2SO4 (1:2 w/v) and quantified the using a SpectraMax Plus 384 (Molecular Devices Corporation, Sunnyside, CA, USA; Miranda et al., 2001). We tested for the effects of the fire treatment and biocrusts location on N fixation through time using two-way, RM-ANOVA.
To investigate the effect of fire on soil infiltration rates, we measured changes in soil infiltration rates (cm s−1) with a Decagon Device's Mini Disk Tension Infiltrometer (METER Group, Pullman, WA) and calculated infiltration following the method outlined by Zhang (1997). Due to the rugose nature of the Cyanobacteria/moss-dominated biocrusts at this site, we added ~10 g of quartz sand to help the infiltrometer form a seal with the soil surface. To assess soil aggregate stability, we measured soil aggregate (6–8 mm in diameter) stability according to the Jornada Experimental Range Test (Herrick et al., 2001) with a kit designed by Synergy Resource Solutions, Inc. (Montana, USA). Briefly, aggregates were assigned to a stability class (1–6) based on a combination of visual observation of slaking following the immersion of the aggregate in distilled water and the percent aggregate remaining on a 1.5 mm sieve after five dipping cycles. We tested for treatment effects though time using two-way, RM-ANOVA.
The impact of fire on Cyanobacteria/lichen- and Cyanobacteria/moss-dominant crusts was dramatic, with no visual recovery of biocrusts on burned soil surfaces during any of our sample times. None of the Cyanobacteria (light or dark surface coloration or surface structure), cyanolichens, green algal lichens, or mosses that composed the visible portion of biocrusts recovered in a year (Supplemental Table 1). One year after the fire, bare soils covered 93% ± 5.1 of the interspaces and 86% ± 5.4 of the surfaces beneath shrub canopies, with a sparse cover of perennial grass, E. elymoides, annual exotic grass, Bromus tectorum (interspace = 0; shrub = 3.8% ± 3.8) and/or the noxious exotic annual forb Halogeton glomeratus (interspace = 1.9% ± 1.9; shrub = 3.1% ± 1.4).
One year after the fire, burned soil biocrusts eroded away with the wind. Burned mosses and lichens remained attached to soil surfaces 2-months after the fire (data not shown); however, after 1 year all that remained was bare soil. Based on the new exposure of burned A. tridentata stems 1-year after the fire and the barren surfaces denuded of moss and lichen cover, anywhere from 0 to 2 cm of biocrusts constituents and some soil potentially eroded away. The unburned biocrusts remained intact over the year. Cyanobacteria/lichen crusts were dominated by one cyanolichen, Collema tenax (16% ± 3.5), and a green algal lichen, Toninia sedifolia (3.1% ± 1.4).
A year after the fire, the burned biocrusts, regardless of type, converged to a common bacterial community. The PCoA results clearly separated burned from unburned communities in ordination space along axis 1, which explained 23.6% of the variation (Figure 1). By the end of the year, the soil communities residing in the now mostly barren soils of burned interspace Cyanobacteria/lichen- and shrub Cyanobacteria/moss-dominated crusts were similar and grouped together. Conversely, unburned communities retained a signature of their biocrust type in ordination space along axis 2 (15.6% of the variation) with Cyanobacteria/lichen- and cyanobacteria/moss-dominated crusts creating unique bacterial communities. PERMANOVA results supported the ordination; there was a fire treatment × time interaction on bacterial composition (PERMANOVA, F = 2.39, R2 = 0.09, P = 0.001), suggesting that fire effect persisted even 1 year, with limited recovery. Also, there was a fire treatment × location on bacterial community composition (PERMANOVA, F = 2.00, R2 = 0.04, P = 0.02), demonstrating that fire altered bacterial communities differently in two biocrust types. This interaction was highlighted as 2-month-old, burned shrub communities were the first to shift from its unburned crust counterpart. All community inferences were based on the recovery of 127,616 quality sequences and 3,345 unique OTUs with samples possessing an average sequencing coverage of 89% ± 0.004 (mean and SEM) and normalized to 3,345 sequences. All sequences are available through NCBI as BioProject SUB6427289.
Figure 1. Bacterial communities in burned Cyanobacteria/lichen-dominated (interspaces) and Cyanobacteria/moss-dominated (shrubs) biocrusts converged to a common composition 1 year after the fire. The multivariate ordination was generated using principle coordinate analysis (PCoA) on a sample × OTU matrix of 16S rRNA gene community libraries (97% similarity cut-off).
Across both biocrusts, fire caused one dominant phylum to disappear and another to appear. Beneath shrubs, the abundance of Cyanobacteria was 72-times lower in the burned (0.12% ± 0.11) than unburned treatment (8.4% ± 2.6) 2 months after the fire, and after 1 year, was undetectable (Figure 2). Similarly, in interspaces, Cyanobacterial abundance was thirteen-times lower in the burned (1.1% ± 0.31) than unburned treatment (14% ± 5.2) 2 months post-fire, and after 1 year, was only barely detectable in burned soils (0.01% ± 0.01). Within the Cyanobacteria, the family Microcoleaceae, more specifically M. vaginatus, was the foundational Cyanobacterial taxon, constituting 13% (± 2.7) of bacterial abundance in Cyanobacteria/lichen crusts and 4.7% (± 1.4) in Cyanobacteria/moss crusts, regardless of sampling time (Figure 3). Nostoc and Chrooccoccidiopsis species also occurred in crusts but their abundance rarely exceeded 1% relative recovery. The resulting bacterial gap in burned biocrusts was filled by rare Firmicutes (Figure 2). Firmicutes were not detectable in unburned Cyanobacteria/lichen and only barely present in Cyanobacterial/moss biocrusts (abundance across all time points = 0.3% ± 0.2); however, 2 months following the fire, Firmicutes constituted 12% ± 5.2 of burned interspace bacteria and 35% ± 16 of burned shrub communities' bacteria. One year after the fire, Firmicutes remained a dominant phylum in burned interspaces (13% ± 5.8) and beneath burned shrubs (9.1% ± 6.0). The Planococcaceae, specifically a Planococcus species, within the Firmicutes differentiated the two burned community types and dominated burnt soils (Figure 3). The recovery of the Planococcaceae in the 2-month- and 1-year-old burnt communities = 12% ± 3.5 in interspace and 19% ± 7.5 in shrub crusts).
Figure 2. Abundant Cyanobacteria were replaced by rare Firmicutes in burnt crusts. The distribution of OTUs (%) is presented for the 10 phyla and three Proteobacteria subclasses that contributed ≥ 1% to the total recovery of biocrust communities. Values are means (n = 3) based on 16S rRNA gene libraries.
Figure 3. Burning and time since fire selected for specific bacterial families based on heat map analysis and hierarchical clustering of the relative recovery of 20 bacterial families. Values are based on means with hierarchal clustering of the burned treatments, and shrub and interspace microsites (top) and families (left). Only families that contributed ≥ 1.0% to the total recovery of communities are presented with recovery based on 16S rRNA gene libraries. Values are means (n = 3).
Other heterotrophic bacteria distinguished burned from unburned biocrusts, especially families from the Actinobacteria (Figure 3). Burned soil conditions enhanced the recovery of the Cellulomonadaceae (Actinobacteria), which was at least 9.0-times higher in burned than unburned biocrusts, with recovery reaching 8.8% ± 3.1 and 8.3% ± 4.6 in 1-year-old burned and now bare interspace and shrub soils respectively. The Oxalobacteraceae (Betaproteobacteria) also dominated burnt soils after 1 year, with abundance increasing from 0.11% (± 0.09) in unburned to 5.7% (± 1.6) burned interspace and 0.33% (± 0.09) in unburned to 6.9% (± 4.1) burned shrub soils. One-year post-fire, the abundance of the Micromonosporaceae (Actinobacteria), Rubrobacteriaceae (Actinobacteria), and Chitinophagaceae (Bacteroidetes) was at least 1.8-times lower in bare soils than unburned biocrusts, regardless of type. In Cyanobacteria/moss-dominated biocrusts the abundance of Sphingomonadaceae (Alphaproteobacteria) was depressed 4.2-fold one year after fire.
Bacterial biomass in the bare surface soils recovered 1 year after the fire, in contrast to richness or diversity that remained relatively stable. In burned shrub soils, bacterial biomass (16S rRNA gene copy number) declined an order of magnitude 1 week, and two orders of magnitude 2-months post-fire but recovered after 1 year (two-way RM-ANOVA, interaction: fire × time, F = 296, P < 0.004, df = 2, Figure 4). In interspace soils, bacterial gene copy numbers were consistently lower in burned than unburned cyanobacteria/lichen-dominated biocrusts. In both crust types, OTU richness in all biocrusts and burnt soils was lowest 1 week (273 ± 34.7), highest 2 month (461 ± 27.51), and moderate 1 year (357 ± 13.5) following the fire (two-way RM-ANOVA, main effect: time, F = 6.9, P = 0.004, df = 2, data not shown). No trend was visible for diversity, which ranged from 2.7 (± 0.77) in 2-month, burnt shrub to 4.2 (± 0.05) in 2-month, unburned shrub crusts (data not shown).
Figure 4. Bacterial biomass declined following fire in both biocrust types. Values are means (n = 2–3) ± SEM, measured with quantitative polymerase chain reaction (qPCR) and reported as rRNA gene copy number. Differences between burned and unburned soils through time are based on two-way, repeated-measures RM-ANOVA.
Fire reduced the network complexity and connectedness present in Cyanobacteria/lichen- and Cyanobacteria/moss-dominated biocrusts. For example, network models for both burned compared to unburned biocrust types contained: 40–75% reduction in the number of significant correlations or edges between nodes or OTUs, up to a 57% increase in mean path length (number of steps between each node and any other node), and at least a 1.4-times smaller mean degree (average number of edges connected to a node, Figure 5, Table 1). Within the network models, 70–80% of the “hub” OTUs or the top 10 highest connected nodes (Table 1) were different between burned and unburned biocrusts. Species belonging to the Acidobacteriaceae (Acidobacteria), Chloroflexaceae, Thermomicrobia (Chloroflexi), and Gemmatimonadaceae (Gemmatimonadetes), were unique hubs in burned shrub soils, while species from the Acidobacteriaceae (Acidobacteria), Acidomicrobineae, Microbacteriaceae Micrococcacaceae, Micromonosporaceae, Solirubrobacteriaceae (Actinobacteria), Chitinophagaceae (Bacteroidetes), and Trueperaceae (Deinococcus) were unique hubs in burned interspace soils (Supplemental Table 2).
Figure 5. Network co-occurrence models using maximal information coefficient (MIC) analysis of unburned and burned biocrusts after fire. Nodes (circles) in the network models represent OTUs from 16S rRNA gene community libraries (97% similarity cut-off) with edges (connecting lines between nodes) representing significant co-occurrence connections that occur in at least 75% of the samples for a given microsite and have an MIC that is both > 0.7 and statistically significant (P < 0.01, n = 6). Colors represent different phyla as shown in the figure key. The size of the nodes indicates the number of significant connections between the given node and other taxa.
Table 1. Metrics from network co-occurrence models of burned and unburned of Cyanobacteria/lichen-dominated crusts occupying relatively plant-barren interspaces (interspaces) and Cyanobacteria/moss-dominated crusts the beneath shrub-islands (shrub).
M. vaginatus (Cyanobacteria) was present in both unburned models; however, it was not a hub species. The Firmicutes that dominated communities following fire failed to influence any OTU in the burned interspace model and was only slightly connected to several other nodes in burned shrub model.
Fire reduced the capacity of Cyanobacteria/lichen-dominated biocrusts to fix N, but inorganic N did accumulate in all burned crusts over time. In interspaces, N fixation rates were 6-times and thirty-one-times lower in burned than unburned treatments 1 week and 2 months after the fire, respectively (RM-ANOVA, interaction: fire × microsite × time, F = 10.4, P < 0.0001, df = 2, Figure 6A). Interspace N fixation rates were still depressed 1 year after the fire, being 3.5-times lower in burned than unburned cyanobacteria/lichen-dominated biocrusts. N fixation rates in all cyanobacteria/moss-dominated crusts were consistently low regardless of the fire. Fire increased concentrations in both burned biocrust types over the 1-year experiment (RM-ANOVA, interaction: fire × time, F = 60, P < 0.0001, df = 2) with concentrations slightly higher in interspace than shrub surface soils (RM-ANOVA interaction: microsites × time, F = 3.7, P = 0.03, df = 2, Figure 6B).
Figure 6. N fixation rates (A), and ammonium concentrations () (B) in unburned and burned biocrusts following fire. N fixation (μmol day−1 m−2) and inorganic N (μg g−1 soil) values are means (n = 10) ± SEM. Differences between treatments are based on RM-ANOVA results from three time intervals following fire (1-week, 1-month, and 1-year).
Fire depressed soil infiltration rates, especially shortly after the burn. Although infiltration was variable in unburned crusts, infiltration rates were at least 5.5-times lower in burned than unburned interspace and shrub soils 1 week after the fire (RM-ANOVA, interspace, fire × time, F = 31, P < 0.0001, df = 2, Figure 7). During the remainder of the year, infiltration rates in both biocrust types were only slightly depressed, at most 1.5-fold. Soil stability was barely impacted by fire, as stability was only depressed at the 1-week sample time in burned under-shrub Cyanobacteria/moss biocrusts (4.0 ± 0.70) compared to control treatment (7.1 ± 0.67, RM-ANOVA, interaction: fire × time, F = 3.0, P < 0.05, df = 2, data not shown).
Figure 7. Soil infiltration rates through biocrusts declined after fire. Values (cm min−1) are means (n = 5), ± SEM, reported in cm s−1. Differences are based on RM-ANOVA with attending F statistics and P-values for significant interactions.
Following fire, surviving taxa, colonizing species from soils immediately below the burn, and/or pioneering bacteria attached to bioaerosols may rapidly create unique communities in multiple ecosystems (Williams et al., 2012; Ferrenberg et al., 2013; Xiang et al., 2014; Li et al., 2019). Our cold desert was no different. Although aspects of the community (i.e., bacterial biomass, alpha diversity, and richness) recovered in the short-term, we found no evidence of the burned bacterial community becoming a burgeoning biocrust. After 1-year, the fire facilitated the formation of a unique convergent community of chemoheterotrophic copiotrophs in the resulting bare surface soils that were once the burnt shrub and interspace biocrusts. The emergent community still helped glue soil aggregates together, but N fixation and soil infiltration were depressed. Taken together, the loss of much of the photosynthetic and N2 fixation potential due the disappearance of Cyanobacteria and/or higher plants calls into question the longevity of the emergent community. The burgeoning community of chemoheterotrophic copiotrophs may only persist until the C and N released from the burn are exhausted. Our results demonstrate that biocrust bacteria may recover rapidly after fire, albeit along a different trajectory that results in fewer ecosystem services.
Contrary to our hypothesis, a simplified Cyanobacteria crust failed to form. M. vaginatus, the foundational Cyanobacteria in our unburned biocrusts, disappeared or was barely detectable in burned soils after 1 year. M. vaginatus did persist 2 months after the fire as an active member, dormant cell, or persistent exogenous DNA. The loss of Cyanobacteria was accompanied by a reduction in chemoheterotrophic copiotrophs, often associated with the “cyanosphere” (Couradeau et al., 2019). The cyanosphere is a nutrient-rich zone, analogous to the rhizosphere, where cyanobacteria enhance fertility of surface soils, primarily through increasing organic C availability via the addition of photosynthate, inorganic N through fixation, and soil moisture due to the specific ecohydrological benefits of crusts. Taxa from three of our families, in particular, were abundant in our unburned cyanosphere and likewise major components of other Cyanobacteria-, lichen-, and/or bryophyte-dominated crusts, Rubrobacteriaceae (Actinobacteria, Nagy et al., 2005; Gundlapally and Garcia-Pichel, 2006; Angel and Conrad, 2013; Maier et al., 2018), Chitinophagaceae (Bacteroidetes, Kuske et al., 2012; Maier et al., 2018), and Sphingomonadaceae (Alphaproteobacteria, Maier et al., 2014). Our sampling technique did not explicitly identify taxa in direct contact with Cyanobacterial filaments but captured taxa within the cyanosphere and in soil immediate surrounding the filaments. Even with our more course sampling, the cyanosphere and other biocrust constituents seem to generate a predictable set of soil conditions that may favor specific bacterial taxa.
The emergent community was dominated by a previously rare Planococcus species (family Planococcaceae, Firmicutes) that dominated all burned soils. In general, Firmicutes are favored in soils following fire, especially in the short-term (Ferrenberg et al., 2013). Our Planococcus species was no exception. This species appears able to occupy, rapidly populate, and dominate the same location as biocrusts constituents (the uppermost millimeters of soil), perhaps by exploiting nutrient-rich shifts in soils induced by fire. Planococcus are moderately halophilic heterotrophic (Ventosa et al., 1998) present in cold deserts around the world capable of hydrolyzing starch (Reddy et al., 2002; Mayilraj et al., 2005). In this instance, Planococcus appeared able to rapidly utilize relatively labile starches released after the burn fire without influencing other taxa, as this species was not a hub species in any network or only slightly connected to several other taxa in the shrub model network. Thus, Planococcus are most likely a copiotroph scrambler (Hibbing et al., 2010), better suited to capitalize on emerging resources by scrambling for nutrients instead of contesting/competing for existing ones. Other copiotrophs dominated burned soils, specifically a Cellulomonas species in the Cellulomonadaceae (Actinobacteria) and Massilia species in the Oxalobacteraceae. Both species were also not hub species, but may capitalized on partially burned plant/algal materials remaining in soils. The Massilia genera houses facultative anaerobes that are able to degrade long chain hydrocarbons in oil contaminated soils (Ali et al., 2016; Ren et al., 2018) and reduce nitrate in biocrusts (Bailey et al., 2014). The Cellulomonas generate houses Gram-positive, aerobic bacteria able to degrade cellulose (Anderson et al., 2012). The emerging dominant bacteria in our converged desert communities were presumably copiotrophs utilizing C resources released after fire. Once the partially burned and available organic C sources are consumed, we project that the community will shift once again, especially if there are no new inputs of C from photosynthetic organisms.
Firmicutes are often only minor constituents in biocrusts but may become more abundant in disturbed biocrusts. In multiple metagenomic surveys of biocrust communities from hot and cold deserts, Firmicutes are relatively uncommon (Steven et al., 2014; Karaoz et al., 2018; Warren et al., 2019). Following multiple forms of disturbance however, these aerobic, desiccation-tolerant copiotrophs may dominate. For example, following rewetting in Cyanobacteria-dominated biocrusts, Microcoleus species were displaced by large blooms of Firmicutes from three families (i.e., Alicyclobacillaceae, Bacillaceae, and Planococcaceae) one of which, the with Planococcaceae houses our Planococcus species (Karaoz et al., 2018). Further, once Cyanobacterial-dominated crusts are disturbed due to grazing, bacterial communities in now bare soils associated with hoofprints contain a high contribution of Firmicutes (Abed et al., 2019). Firmicutes are often spore-formers and the ability of these taxa to weather adverse environmental conditions as endospores most likely contributes to their success in disturbed biocrusts. Our dominant Firmicutes, a Planococcus species, however, is from a non-spore forming genera. The ability of the Planococcus to exploit disturbed biocrusts may reside in their protein flexibility, resource efficiency, genomic plasticity, and osmotic-specific adaptive mechanisms that likely compensate for the desiccation and cold stresses present in cold deserts (Mykytczuk et al., 2013).
As hypothesized, bacterial biomass, richness, and diversity recovered to approximately unburned levels 1 year after the fire even in bare soils, but the resulting community was more diffuse and sparsely interconnected. In soils, disturbances that alter the quantity and quality of C and other resources may exert immense control over bacterial communities and microbial-mediated processes (Ma et al., 2015; Zechmeister-Boltenstern et al., 2015). With desert wildfires may come a flush of resources (Fuentes-Ramirez et al., 2015). Depending on fire severity, partially burnt plant materials deposited on soil surfaces and/or leached into the profile, along with defunct root systems, offers copious amounts of relatively labile and recalcitrant C for bacteria to harness. Additionally, fire may release phosphorus and other non-combustible nutrient potentially alleviating nutrient limitations and the availability of water may rise in the absence of transpiration by higher plants. In deserts, soils surrounding burned A. tridentata supported higher levels of bacterial biomass and increased concentrations of total organic C, total N, and dissolved organic C (Halvorson et al., 1997). Thus, burned soils may offer relatively nutrient-rich soil conditions and/or new niches for recovering bacteria to exploit. The dominant Planococcus species (Firmicutes) was not one of the top-ten most interconnected bacterial species in burnt soils. Fire created a unique set of interconnected hub species of chemoheterotrophic copiotrophs commonly found in biocrusts and/or other taxa well-adapted to weather and thrive in desert soil conditions. For example, new hubs, Acidobacteriaceae (Acidobacteria), Solirubrobacteriaceae (Actinobacteria), Chitinophagaceae (Bacteroidetes), are common copiotrophs within biocrusts (Kuske et al., 2012; Angel and Conrad, 2013; Maier et al., 2018) and potentially contribute/influence a consortium of taxa consuming cellulose, hemicellulose, and chitin within burnt soils. The Chloroflexi commonly associated with M. vaginatus in biocrusts are thermotolerant and non-photosynthetic scavenging organic acids derived from Cyanobacterial photosynthates (Maier et al., 2018). In burned soils beneath shrubs, the hub members of the Chloroflexaceae and Thermomicrobia were most likely thermophilic and cellulolytic taxa demonstrating photoheterotrophic and/or chemoheterotrophic metabolism (Houghton et al., 2015; Klatt et al., 2015). Thus, both hubs were possibly reliant or linked to other taxa generating organic acids in burned soils. Last, the Actinobacteria are desert cosmopolitan species, hosting taxa that are acidtolerant, alkalitolerant, psychrotolerant, thermotolerant, and halotolerant (Mohammadipanah and Wink, 2016), and able to produce dormant endospores under harsh conditions. Thus, we are assuming that our Gram-positive Actinobacteria hubs (i.e., Acidomicrobineae, Microbacteriaceae Micrococcacaceae, and Micromonosporaceae) were extremely well-adapted to weather the environmental extremes often present in deserts and potentially compete for resources under burned soil conditions.
Our last hypothesis was predicated on the services provided by a rudimentary M. vaginatus-dominated crust. In the absence of Cyanobacteria; however, the recovered bacterial community provided only an abbreviated set of ecosystem services. Soil aggregate stability was only marginally impacted by the burn, dipping slightly in Cyanobacteria/moss-dominated crusts 2 months after the fire. Bacteria commonly produce exopolymeric substances that glue soil particles together (Costa et al., 2018). Our data suggest that the post-fire soil community aggregated soils into particles or at least helped maintain aggregation in now surface soils as proficiently as either biocrust type. The benefits of soil aggregation by biota are substantial (Bronick and Lal, 2005), but the emerging soil communities we observed after fire are unlikely able to withstand the high erosional forces of desert winds. Well-developed biocrusts, those with high biomass of crust constituents, reduce soil erodibility, and armor soils against wind erosion (Belnap and Gardner, 1993; Mazor et al., 1996). Our burned soils support similar amounts of bacterial biomass but the surfaces are void of crusts. Thus, substantial erosion is likely, regardless of soil aggregate stability. Alternatively, both infiltration and N fixation failed to recover following fire. We found some evidence of a hydrophobic layer forming on the burned soil surfaces, as infiltration plummeted 1-week after the fire and remained depressed through the year. We expect that the burning of biocrusts, A. tridentata, or the straw that we added to carry the fire and released hydrophobic organic compounds or rearranged amphiphilic molecules (e.g., phytanols and fatty acids) already present in the soil (Ravi et al., 2007; Uddin et al., 2017). N fixation was basically non-existent in burned soils, but soil ammonium continued to accumulate in burned surface soils, suggesting that the emergent community had adequate access to N.
Our burnt soils will most likely never recover into a fully-developed biocrust. Although the burnt soils were only sparsely covered with exotic, annual grass B. tectorum and noxious invader H. glomeratus 1 year after the fire, both species had fully encroached into the disturbed soils after only 4 years (St. Clair et al., 2016). Cover of B. tectorum increased from 3.8% (± 5.2) to 23% (± 5.2) and H. glomeratus increased from 0.9% (± 1.1) to 13% (± 1.1) in unburned compared to burned plots. Fully formed biocrusts often inhibit exotic but not native plant establishment (Slate et al., 2019), but disturbance may suppress this process (Hernandez and Sandquist, 2011).
Such invasion results in a greater percentage of vascular plant cover and subsequent litter on the soil surface, both of which reduce light levels reaching the soil surface, effectively threatening essential phototrophic component of crusts (Brooks and Matchett, 2006). Additionally, increased plant cover may compete for essential nutrients (e.g., N and phosphorus) and soil moisture necessary for crust recovery (Evans et al., 2001; Ryel et al., 2010). Thus, if fire occur in close proximity to exotic plant seed sources and the disturbed soils are readily invaded, the probability of a fully-developed crust is unlikely.
An intensive wildfire inhibited the recovery of even a rudimentary biocrust in 1 year's time. Although wildfires changed biocrusts into bare soils with vastly different community composition, both bacterial communities supported similar level of bacterial biomass, alpha diversity, and richness 1 year after the fire. Rather than being dominated by the Cyanobacterium M. vaginatus, the two burned biocrust types converged to a common community dominated by heterotrophic copiotrophs most likely benefiting from the release of partially burned biocrust and plant materials. The fire created more diffuse and poorly connected communities than their unburned biocrust counterparts disrupting upwards of 75% of species interactions present in unburned crusts. One common ecosystem service supported by biocrusts potentially returned (i.e., exopolymeric substances gluing soil aggregates together); however, the seminal biocrust services of N fixation and improved soil ecohydrology, measured here as soil infiltration rates, remained substantially reduced 1 year after the burn. Our results suggest that the absence of the dominant and foundational taxa of biocrusts opened multiple new niches for rare bacteria to exploit creating poorly connected communities that provided only an abbreviated set of ecosystem services.
This manuscript contains previously unpublished data. All sequences are available through NCBI as BioProject SUB6427289.
ZA, JBa, DR, JBe, TC, RG, BM, and SC conducted the experiments and helped write and review the manuscript. ZA, JBa, DR, JBe, and SC analyzed and interpreted the data. ZA agrees to be accountable for all aspects of the work in ensuring that questions related to the accuracy or integrity of any part of the work are appropriately investigated and resolved.
Our research was supported by a USDA National Institute of Food and Agriculture: 2010-38415-21908.
The authors declare that the research was conducted in the absence of any commercial or financial relationships that could be construed as a potential conflict of interest.
We thank the Bureau of Land Management Salt Lake City Field Office for conducting NEPA clearance and performing the experimental burn manipulations. JBe thanks the Ecosystems and Land Use Change Mission Areas of U.S. Geological Survey. Any use of trade, firm, or product names is for descriptive purposes only and does not imply endorsement by the U.S. Government.
The Supplementary Material for this article can be found online at: https://www.frontiersin.org/articles/10.3389/fevo.2019.00467/full#supplementary-material
Aanderud, Z. T., Saurey, S., Ball, B. A., Wall, D. H., Barrett, J. E., Muscarella, M. E., et al. (2019). Stoichiometric shifts in soil C:N:P promote bacterial taxa dominance, maintain biodiversity, and deconstruct community assemblages (vol 9, 1401, 2018). Front. Microbiol. 10:391. doi: 10.3389/fmicb.2019.00391
Aanderud, Z. T., Smart, T. B., Wu, N., Taylor, A. S., Zhang, Y. M., and Belnap, J. (2018). Fungal loop transfer of nitrogen depends on biocrust constituents and nitrogen form. Biogeosciences 15, 3831–3840. doi: 10.5194/bg-15-3831-2018
Abed, R. M., Tamm, A., Hassenrück, C., Al-Rawahi, A. N., Rodríguez-Caballero, E., Fiedler, S., et al. (2019). Habitat-dependent composition of bacterial and fungal communities in biological soil crusts from Oman. Nat. Sci. Rep. 9:6468. doi: 10.1038/s41598-019-42911-6
Ali, N., Dashti, N., Salamah, S., Al-Awadhi, H., Sorkhoh, N., and Radwan, S. (2016). Autochthonous bioaugmentation with environmental samples rich in hydrocarbonoclastic bacteria for bench-scale bioremediation of oily seawater and desert soil. Environ. Sci. Pollut. Res. 23, 8686–8698. doi: 10.1007/s11356-016-6057-y
Alvarez, C. R., Alvarez, R., Grigera, S., and Lavado, R. S. (1998). Associations between organic matter fractions and the active soil microbial biomass. Soil Biol. Biochem. 30, 767–773. doi: 10.1016/s0038-0717(97)00168-5
Anderson, I., Abt, B., Lykidis, A., Klenk, H. P., Kyrpides, N., and Ivanova, N. (2012). Genomics of aerobic cellulose utilization systems in actinobacteria. PLoS ONE 7:e39331. doi: 10.1371/journal.pone.0039331
Anderson, M. J. (2001). A new method for non-parametric multivariate analysis of variance. Austral. Ecol. 26, 32–46. doi: 10.1111/j.1442-9993.2001.01070.pp.x
Angel, R., and Conrad, R. (2013). Elucidating the microbial resuscitation cascade in biological soil crusts following a simulated rain event. Environ. Microbiol. 15, 2799–2815. doi: 10.1111/1462-2920.12140
Bailey, C. A., Kellom, M., Poret-Peterson, A. T., Noonan, K., Hartnett, H. E., and Raymond, J. (2014). Draft genome sequence of Massilia sp., strain BSC265, isolated from biological soil crust of Moab, Utah. Genome Announc. 2:e01199-14. doi: 10.1128/genomeA.01199-14
Balch, J. K., Bradley, B. A., D'Antonio, C. M., and Gomez-Dans, J. (2013). Introduced annual grass increases regional fire activity across the arid western USA (1980-2009). Glob. Change Biol. 19, 173–183. doi: 10.1111/gcb.12046
Barberan, A., Bates, S. T., Casamayor, E. O., and Fierer, N. (2014). Using network analysis to explore co-occurrence patterns in soil microbial communities (vol 6, pg 343, 2012). ISME J. 8, 952–952. doi: 10.1038/ismej.2013.236
Belnap, J. (1996). Soil surface disturbances in cold deserts: Effects on nitrogenase activity in cyanobacterial-lichen soil crusts. Biol. Fert. Soils 23, 362–367. doi: 10.1007/BF00335908
Belnap, J. (2002). Nitrogen fixation in biological soil crusts from southeast Utah, USA. Biol. Fertil. Soils 35, 128–135. doi: 10.1007/s00374-002-0452-x
Belnap, J. (2003). The world at your feet: desert biological soil crusts. Front. Ecol. Environ. 1, 181–189. doi: 10.1890/1540-9295(2003)001
Belnap, J., and Gardner, J. S. (1993). Soil microstructure in soils of the Colorado Plateau-the role of Cyanobacterium Micorcoleus vaginatus. Great Basin Nat. 53, 40–47.
Boose, Y., Welti, A., Atkinson, J., Ramelli, F., Danielczok, A., Bingemer, H. G., et al. (2016). Heterogeneous ice nucleation on dust particles sourced from nine deserts worldwide - Part 1: immersion freezing. Atmos. Chem. Phys. 16, 15075–15095. doi: 10.5194/acp-16-15075-2016
Bowker, M. A., Belnap, J., Chaudhary, V. B., and Johnson, N. C. (2008). Revisiting classic water erosion models in drylands: the strong impact of biological soil crusts. Soil Biol. Biochem. 40, 2309–2316. doi: 10.1016/j.soilbio.2008.05.008
Bronick, C. J., and Lal, R. (2005). Soil structure and management: a review. Geoderma 124, 3–22. doi: 10.1016/j.geoderma.2004.03.005
Brooks, M. L., and Matchett, J. R. (2006). Spatial and temporal patterns of wildfires in the Mojave Desert, 1980-2004. J. Arid Environ. 67, 148–164. doi: 10.1016/j.jaridenv.2006.09.027
Chamizo, S., Canton, Y., Rodriguez-Caballero, E., and Domingo, F. (2016). Biocrusts positively affect the soil water balance in semiarid ecosystems. Ecohydrology 9, 1208–1221. doi: 10.1002/eco.1719
Chilton, A. M., Neilan, B. A., and Eldridge, D. J. (2018). Biocrust morphology is linked to marked differences in microbial community composition. Plant Soil 429, 65–75. doi: 10.1007/s11104-017-3442-3
Choudoir, M. J., Barberan, A., Menninger, H. L., Dunn, R. R., and Fierer, N. (2018). Variation in range size and dispersal capabilities of microbial taxa. Ecology 99, 322–334. doi: 10.1002/ecy.2094
Cole, J. R., Wang, Q., Cardenas, E., Fish, J., Chai, B., Farris, R. J., et al. (2009). The Ribosomal Database Project: improved alignments and new tools for rRNA analysis. Nucleic Acids Res. 37, D141–D145. doi: 10.1093/nar/gkn879
Costa, O. Y. A., Raaijmakers, J. M., and Kuramae, E. E. (2018). Microbial extracellular polymeric substances: ecological function and impact on soil aggregation. Front. Microbiol. 9:1636. doi: 10.3389/fmicb.2018.01636
Couradeau, E., Giraldo-Silva, A., De Martini, F., and Garcia-Pichel, F. (2019). Spatial segregation of the biological soil crust microbiome around its foundational cyanobacterium, Microcoleus vaginatus, and the formation of a nitrogen-fixing cyanosphere. Microbiome 7:55. doi: 10.1186/s40168-019-0661-2
Dastrup, D. B., Carling, G. T., Collins, S. A., Nelson, S. T., Fernandez, D. P., Tingey, D. G., et al. (2018). Aeolian dust chemistry and bacterial communities in snow are unique to airshed locations across northern Utah, USA. Atmos. Environ. 193, 251–261. doi: 10.1016/j.atmosenv.2018.09.016
Duniway, M. C., Pfennigwerth, A. A., Fick, S. E., Nauman, T. W., Belnap, J., and Barger, N. N. (2019). Wind erosion and dust from US drylands: a review of causes, consequences, and solutions in a changing world. Ecosphere 10:e02650. doi: 10.1002/ecs2.2650
Edgar, R. C., Haas, B. J., Clemente, J. C., Quince, C., and Knight, R. (2011). UCHIME improves sensitivity and speed of chimera detection. Bioinformatics 27, 2194–2200. doi: 10.1093/bioinformatics/btr381
Eldridge, D. J., and Leys, J. F. (2003). Exploring some relationships between biological soil crusts, soil aggregation and wind erosion. J. Arid Environ. 53, 457–466. doi: 10.1006/jare.2002.1068
Esque, T. C., Kaye, J. P., Eckert, S. E., DeFalco, L. A., and Tracy, C. R. (2010). Short-term soil inorganic N pulse after experimental fire alters invasive and native annual plant production in a Mojave Desert shrubland. Oecologia 164, 253–263. doi: 10.1007/s00442-010-1617-1
Evans, R. D., Rimer, R., Sperry, L., and Belnap, J. (2001). Exotic plant invasion alters nitrogen dynamics in an arid grassland. Ecol. Appl. 11, 1301–1310. doi: 10.1890/1051-0761(2001)011[1301:EPIAND]2.0.CO;2
Faille, C., Jullien, C., Fontaine, F., Bellon-Fontaine, M. N., Slomianny, C., and Benezech, T. (2002). Adhesion of Bacillus spores and Escherichia coli cells to inert surfaces: role of surface hydrophobicity. Can. J. Microbiol. 48, 728–738. doi: 10.1139/w02-063
Faist, A. M., Herrick, J. E., Belnap, J., Van Zee, J. W., and Barger, N. N. (2017). Biological soil crust and disturbance controls on surface hydrology in a semi-arid ecosystem. Ecosphere 8:13. doi: 10.1002/ecs2.1691
Fernelius, K. J., Madsen, M. D., Hopkins, B. G., Bansal, S., Anderson, V. J., Eggett, D. L., et al. (2017). Post-fire interactions between soil water repellency, soil fertility and plant growth in soil collected from a burned pition-juniper woodland. J. Arid Environ. 144, 98–109. doi: 10.1016/j.jaridenv.2017.04.005
Ferrenberg, S., O'Neill, S. P., Knelman, J. E., Todd, B., Duggan, S., Bradley, D., et al. (2013). Changes in assembly processes in soil bacterial communities following a wildfire disturbance. ISME J. 7, 1102–1111. doi: 10.1038/ismej.2013.11
Freedman, Z. B., Upchurch, R. A., and Zak, D. R. (2016). Microbial potential for ecosystem N loss is increased by experimental N deposition. PLoS ONE 11:e0164531. doi: 10.1371/journal.pone.0164531
Fuentes-Ramirez, A., Schafer, J. L., Mudrak, E. L., Schat, M., Parag, H. A., Holzapfel, C., et al. (2015). Spatio-temporal impacts of fire on soil nutrient availability in Larrea tridentata shrublands of the Mojave Desert, USA. Geoderma 259, 126–133. doi: 10.1016/j.geoderma.2015.05.016
Garcia-Pichel, F., Loza, V., Marusenko, Y., Mateo, P., and Potrafka, R. M. (2013). Temperature drives the continental-scale distribution of key microbes in topsoil communities. Science 340, 1574–1577. doi: 10.1126/science.1236404
Guenon, R., and Gros, R. (2013). Frequent-wildfires with shortened time-since-fire affect soil microbial functional stability to drying and rewetting events. Soil Biol. Biochem. 57, 663–674. doi: 10.1016/j.soilbio.2012.07.006
Gundlapally, S. R., and Garcia-Pichel, F. (2006). The community and phylogenetic diversity of biological soil crusts in the Colorado Plateau studied by molecular fingerprinting and intensive cultivation. Microb. Ecol. 52, 345–357. doi: 10.1007/s00248-006-9011-6
Halvorson, J. J., Bolton, H., and Smith, J. L. (1997). The pattern of soil variables related to Artemisia tridentata in a burned shrub-steppe site. Soil Sci. Soc. Am. J. 61, 287–294. doi: 10.2136/sssaj1997.03615995006100010041x
Hawkes, C. V., and Flechtner, V. R. (2002). Biological soil crusts in a xeric Florida shrubland: composition, abundance, and spatial heterogeneity of crusts with different disturbance histories. Microb. Ecol. 43, 1–12. doi: 10.1007/s00248-001-1017-5
Hernandez, R. R., and Sandquist, D. R. (2011). Disturbance of biological soil crust increases emergence of exotic vascular plants in California sage scrub. Plant Ecol. 212, 1709–1721. doi: 10.1007/s11258-011-9943-x
Herrick, J. E., Whitford, W. G., de Soyza, A. G., Van Zee, J. W., Havstad, K. M., Seybold, C. A., et al. (2001). Field soil aggregate stability kit for soil quality and rangeland health evaluations. Catena 44, 27–35. doi: 10.1016/s0341-8162(00)00173-9
Hibbing, M. E., Fuqua, C., Parsek, M. R., and Peterson, S. B. (2010). Bacterial competition: surviving and thriving in the microbial jungle. Nat. Rev. Microbiol. 8, 15–25. doi: 10.1038/nrmicro2259
Hilty, J. H., Eldridge, D. J., Rosentreter, R., and Wicklow-Howard, M. C. (2003). Burning and seeding influence soil surface morphology in an Artemisia shrubland in southern Idaho. Arid Land Res. Manage. 17, 1–11. doi: 10.1080/15324980390169028
Hinojosa, M. B., Laudicina, V. A., Parra, A., Albert-Belda, E., and Moreno, J. M. (2019). Drought and its legacy modulate the post-fire recovery of soil functionality and microbial community structure in a Mediterranean shrubland. Glob. Change Biol. 25, 1409–1427. doi: 10.1111/gcb.14575
Houghton, K. M., Morgan, X. C., Lagutin, K., MacKenzie, A. D., Mitchell, K. A., McDonald, I. R., et al. (2015). Thermorudis pharmacophila sp. nov., a novel member of the class Thermomicrobia isolated from geothermal soil, and emended descriptions of Thermomicrobium roseum, Thermomicrobium caboxidum, Thermomicrobium peleae, and Sphaerobacter thermophilus. Int. J. Syst. Evol. Microbiol. 65, 4479–4487. doi: 10.1099/ijsem.0.000598
Hu, C. X., Liu, Y. D., Song, L. R., and Zhang, D. K. (2002). Effect of desert soil algae on the stabilization of fine sands. J. Appl. Phycol. 14, 281–292. doi: 10.1023/a:1021128530086
Johansen, J. R., St. Clair, L. L., Webb, B. L., and Nebeker, G. T. (1984). Recovery patterns of cyrptogamic soil crusts in desert rangelands following fire disturbance. Bryologist 87, 238–243. doi: 10.2307/3242798
Karaoz, U., Couradeau, E., da Rocha, U. N., Lim, H., Northen, T., Garcia-Pichel, F., et al. (2018). Large blooms of Bacillales (Firmicutes) underlie the response to rewetting of Cyanobacterial biocrusts at various stages of maturity. mBio 9, 1–17. doi: 10.1128/mBio.01366-16
Kim, O. S., Yoo, J. J., Lee, D. H., Ahn, T. S., and Song, H. G. (2004). Monitoring of bacterial community in a coniferous forest soil after a wildfire. J. Microbiol. 42, 278–284.
Klatt, G. C., Zhenfeng, L., Ludwig, M., Kuhl, M., Jensen, S. I., Dryant, D. A., et al. (2015). Temporal metatranscriptomic patterning in phototrophic Chloroflexi inhabiting a microbial mat in a geothermal spring. ISME J. 7, 1775–1789. doi: 10.1038/ismej.2013.52
Koster, K., Berninger, F., Heinonsalo, J., Linden, A., Koster, E., Ilvesniemi, H., et al. (2016). The long-term impact of low-intensity surface fires on litter decomposition and enzyme activities in boreal coniferous forests. Int. J. Wildland Fire 25, 213–223. doi: 10.1071/wf14217
Kuske, C. R., Yeager, C. M., Johnson, S., Ticknor, L. O., and Belnap, J. (2012). Response and resilience of soil biocrust bacterial communities to chronic physical disturbance in arid shrublands. ISME J. 6, 886–897. doi: 10.1038/ismej.2011.153
Lennon, J. T., and Jones, S. E. (2011). Microbial seed banks: the ecological and evolutionary implications of dormancy. Nat. Rev. Microbiol. 9, 119–130. doi: 10.1038/nrmicro2504
Li, W. K., Niu, S. K., Liu, X. D., and Wang, J. M. (2019). Short-term response of the soil bacterial community to differing wildfire severity in Pinus tabulaeformis stands. Sci. Rep. 9:10. doi: 10.1038/s41598-019-38541-7
Ma, C., Liu, M. Q., Wang, H., Chen, C. Y., Fan, W. Q., Griffiths, B., et al. (2015). Resource utilization capability of bacteria predicts their invasion potential in soil. Soil Biol. Biochem. 81, 287–290. doi: 10.1016/j.soilbio.2014.11.025
Maier, S., Schmidt, T. S. B., Zheng, L. J., Peer, T., Wagner, V., and Grube, M. (2014). Analyses of dryland biological soil crusts highlight lichens as an important regulator of microbial communities. Biodivers. Conserv. 23, 1735–1755. doi: 10.1007/s10531-014-0719-1
Maier, S., Tamm, A., Wu, D. M., Caesar, J., Grube, M., and Weber, B. (2018). Photoautotrophic organisms control microbial abundance, diversity, and physiology in different types of biological soil crusts. ISME J. 12, 1032–1046. doi: 10.1038/s41396-018-0062-8
Mayilraj, S., Prasad, G. S., Suresh, K., Saini, H. S., Shivaji, S., and Chakrabarti, T. (2005). Planococcus stackebrandtii sp nov., isolated from a cold desert of the Himalayas, India. Int. J. Syst. Evol. Microbiol. 55, 91–94. doi: 10.1099/ijs.0.63290-0
Mazor, G., Kidron, G. J., Vonshak, A., and Abeliovich, A. (1996). The role of cyanobacterial exopolysaccharides in structuring desert microbial crusts. FEMS Microbiol. Ecol. 21, 121–130. doi: 10.1016/0168-6496(96)00050-5
Miranda, K. M., Espey, M. G., and Wink, D. A. (2001). A rapid, simple spectrophotometric method for simultaneous detection of nitrate and nitrite. Nitric Oxide Biol. Chem. 5, 62–71. doi: 10.1006/niox.2000.0319
Mohammadipanah, F., and Wink, J. (2016). Actinobacteria from arid and desert habitats: diversity and biological activity. Front. Microbiol. 6:1541. doi: 10.3389/fmicb.2015.01541
Mykytczuk, N. C. S., Foote, S. J., Omelon, C. R., Southam, G., Greer, C. W., and Whyte, L. G. (2013). Bacterial growth at −15 °C; molecular insights from the permafrost bacterium Planococcus halocryophilus Or1. ISME J. 7, 1211–1226. doi: 10.1038/ismej.2013.8
Nagy, M. L., Johansen, J. R., St. Clair, L. L., and Webb, B. L. (2005). Recovery patterns of microblotic soil crusts 70 years after arsenic contamination. J. Arid Environ. 63, 304–323. doi: 10.1016/j.jaridenv.2005.03.029
Prendergast-Miller, M. T., de Menezes, A. B., Macdonald, L. M., Toscas, P., Bissett, A., Baker, G., et al. (2017). Wildfire impact: natural experiment reveals differential short-term changes in soil microbial communities. Soil Biol. Biochem. 109, 1–13. doi: 10.1016/j.soilbio.2017.01.027
Pressler, Y., Moore, J. C., and Cotrufo, M. F. (2019). Belowground community responses to fire: meta-analysis reveals contrasting responses of soil microorganisms and mesofauna. Oikos 128, 309–327. doi: 10.1111/oik.05738
Prietofernandez, A., Villar, M. C., Carballas, M., and Carballas, T. (1993). Short-term effects of a wildfire on the nitrogen status and ite mineralization kinetics in an Atlantic forest soils. Soil Biol. Biochem. 25, 1657–1664. doi: 10.1016/0038-0717(93)90167-a
Pringault, O., and Garcia-Pichel, F. (2004). Hydrotaxis of cyanobacteria in desert crusts. Microb. Ecol. 47, 366–373. doi: 10.1007/s00248-002-0107-3
Pruesse, E., Quast, C., Knittel, K., Fuchs, B. M., Ludwig, W. G., Peplies, J., et al. (2007). SILVA: a comprehensive online resource for quality checked and aligned ribosomal RNA sequence data compatible with ARB. Nucleic Acids Res. 35, 7188–7196. doi: 10.1093/nar/gkm864.
R Development Core Team (2017). R: A language and Environment for Statistical Computing. Vienna: R Foundation for Statistical Computing. Available online at: http://www.R-project.org.
Rajeev, L., da Rocha, U. N., Klitgord, N., Luning, E. G., Fortney, J., Axen, S. D., et al. (2013). Dynamic cyanobacterial response to hydration and dehydration in a desert biological soil crust. ISME J. 7, 2178–2191. doi: 10.1038/ismej.2013.83
Ravi, S., D'Odorico, P., Zobeck, T. M., Over, T. M., and Collins, S. L. (2007). Feedbacks between fires and wind erosion in heterogeneous arid lands. J. Geophys. Res. Biogeosci. 112:7. doi: 10.1029/2007jg000474
Reddy, G. S. N., Prakash, J. S. S., Vairamani, M., Prabhakar, S., Matsumoto, G. I., and Shivaji, S. (2002). Planococcus antarcticus and Planococcus psychrophilus spp. nov isolated from cyanobacterial mat samples collected from ponds in Antarctica. Extremophiles 6, 253–261. doi: 10.1007/s00792-001-0250-7
Ren, M. N., Li, X. Y., Zhang, Y. Q., Jin, Y., Li, S. Q., and Huang, H. D. (2018). Massilia armeniaca sp nov., isolated from desert soil. Int. J. Syst. Evol. Microbiol. 68, 2319–23624. doi: 10.1099/ijsem.0.002836
Reshef, D. N., Reshef, Y. A., Finucane, H. K., Grossman, S. R., McVean, G., Turnbaugh, P. J., et al. (2011). Detecting novel associations in large data sets. Science 334, 1518–1524. doi: 10.1126/science.1205438
Rodriguez, J., Gonzalez-Perez, J. A., Turmero, A., Hernandez, M., Ball, A. S., Gonzalez-Vila, F. J., et al. (2017). Wildfire effects on the microbial activity and diversity in a Mediterranean forest soil. Catena 158, 82–88. doi: 10.1016/j.catena.2017.06.018
Rodriguez-Caballero, E., Canton, Y., and Jetten, V. (2015). Biological soil crust effects must be included to accurately model infiltration and erosion in drylands: an example from Tabernas Badlands. Geomorphology 241, 331–342. doi: 10.1016/j.geomorph.2015.03.042
Root, H. T., Brinda, J. C., and Dodson, E. K. (2017). Recovery of biological soil crust richness and cover 12-16 years after wildfires in Idaho, USA. Biogeosciences 14, 3957–3969. doi: 10.5194/bg-14-3957-2017
Rosentreter, R., Bowker, M., and Belnap, J. (2007). A Field Guide to Biological Soil Crusts of Western U.S. Drylands: Common Lichens and Bryophytes. Denver, CO: U.S. Government Printing Office.
Ryel, R. J., Leffler, A. J., Ivans, C., Peek, M. S., and Caldwell, M. M. (2010). Functional differences in water-use patterns of contrasting life forms in Great Basin steppelands. Vadose Zone J. 9, 548–560. doi: 10.2136/vzj2010.0022
Schloss, P. D., Westcott, S. L., Ryabin, T., Hall, J. R., Hartmann, M., Hollister, E. B., et al. (2009). Introducing mothur: open-source, platform-independent, community-supported software for describing and comparing microbial communities. Appl. Environ. Microbiol. 75, 7537–7541. doi: 10.1128/aem.01541-09
Slate, M. L., Callaway, R. M., and Pearson, D. E. (2019). Life in interstitial space: biocrusts inhibit exotic but not native plant establishment in semi-arid grasslands. J. Ecol. 107, 1317–1327. doi: 10.1111/1365-2745.13117
St. Clair, S. B., O'Connor, R., Gill, R., and McMillan, B. (2016). Biotic resistance and disturbance: rodent consumers regulate post-fire plant invasions and increase plant community diversity. Ecology 97, 1700–1711. doi: 10.1002/ecy.1391
Steven, B., Gallegos-Graves, L., Belnap, J., and Kuske, C. R. (2013). Dryland soil microbial communities display spatial biogeographic patterns associated with soil depth and soil parent material. FEMS Microbiol. Ecol. 86, 101–113. doi: 10.1111/1574-6941.12143
Steven, B., Gallegos-Graves, L. V., Yeager, C., Belnap, J., and Kuske, C. R. (2014). Common and distinguishing features of the bacterial and fungal com- munities in biological soil crusts and shrub root zone soils. Soil Biol. Biochem. 69, 302–312. doi: 10.1016/j.soilbio.2013.11.008
Uddin, S. M. M., Daniel, N. R. R., Harper, R. J., and Henry, D. J. (2017). Why do biogenic volatile organic compounds (BVOCs) derived from vegetation fire not induce soil water repellency? Biogeochemistry 134, 147–161. doi: 10.1007/s10533-017-0352-7
Ventosa, A., Nieto, J. J., and Oren, A. (1998). Biology of moderately halophilic aerobic bacteria. Microbiol. Mol. Biol. Rev. 62, 504–544.
Wang, G. S., Mayes, M. A., Gu, L. H., and Schadt, C. W. (2014). Representation of dormant and active microbial dynamics for ecosystem modeling. PLoS ONE 9:e89252. doi: 10.1371/journal.pone.0089252
Warren, S. D., St. Clair, L. L., Stark, L. R., Lewis, L. A., Pombubpa, N., Kurbessoian, T., et al. (2019). Reproduction and dispersal of biological soil crust organisms. Front. Ecol. Evol. 7:344. doi: 10.3389/fevo.2019.00344
Weil, T., De Filippo, C., Albanese, D., Donati, C., Pindo, M., Pavarini, L., et al. (2017). Legal immigrants: invasion of alien microbial communities during winter occurring desert dust storms. Microbiome 5:11. doi: 10.1186/s40168-017-0249-7
Williams, R. J., Hallgren, S. W., and Wilson, G. W. T. (2012). Frequency of prescribed burning in an upland oak forest determines soil and litter properties and alters the soil microbial community. For. Ecol. Manage. 265, 241–247. doi: 10.1016/j.foreco.2011.10.032
Xiang, X. J., Shi, Y., Yang, J., Kong, J. J., Lin, X. G., Zhang, H. Y., et al. (2014). Rapid recovery of soil bacterial communities after wildfire in a Chinese boreal forest. Sci. Rep. 4:3829. doi: 10.1038/srep03829
Yeager, C. M., Kornosky, J. L., Morgan, R. E., Cain, E. C., Garcia-Pichel, F., Housman, D. C., et al. (2007). Three distinct clades of cultured heterocystous cyanobacteria constitute the dominant N2-fixing members of biological soil crusts of the Colorado Plateau, USA. FEMS Microbiol. Ecol. 60, 85–97. doi: 10.1111/j.1574-6941.2006.00265.x
Zechmeister-Boltenstern, S., Keiblinger, K. M., Mooshammer, M., Penuelas, J., Richter, A., Sardans, J., et al. (2015). The application of ecological stoichiometry to plant-microbial-soil organic matter transformations. Ecol. Monogr. 85, 133–155. doi: 10.1890/14-0777.1.sm
Keywords: biological soil crust, Bromus tectorum, disturbance, Great Basin Desert, rare biosphere, network co-occurrence model
Citation: Aanderud ZT, Bahr J, Robinson DM, Belnap J, Campbell TP, Gill RA, McMillian B and St. Clair S (2019) The Burning of Biocrusts Facilitates the Emergence of a Bare Soil Community of Poorly-Connected Chemoheterotrophic Bacteria With Depressed Ecosystem Services. Front. Ecol. Evol. 7:467. doi: 10.3389/fevo.2019.00467
Received: 28 June 2019; Accepted: 20 November 2019;
Published: 13 December 2019.
Edited by:
Nicole Pietrasiak, New Mexico State University, United StatesReviewed by:
Ferran Garcia-Pichel, Arizona State University, United StatesCopyright © 2019 Aanderud, Bahr, Robinson, Belnap, Campbell, Gill, McMillian and St. Clair. This is an open-access article distributed under the terms of the Creative Commons Attribution License (CC BY). The use, distribution or reproduction in other forums is permitted, provided the original author(s) and the copyright owner(s) are credited and that the original publication in this journal is cited, in accordance with accepted academic practice. No use, distribution or reproduction is permitted which does not comply with these terms.
*Correspondence: Zachary T. Aanderud, emFjaGFyeV9hYW5kZXJ1ZEBieXUuZWR1
Disclaimer: All claims expressed in this article are solely those of the authors and do not necessarily represent those of their affiliated organizations, or those of the publisher, the editors and the reviewers. Any product that may be evaluated in this article or claim that may be made by its manufacturer is not guaranteed or endorsed by the publisher.
Research integrity at Frontiers
Learn more about the work of our research integrity team to safeguard the quality of each article we publish.