- Department of Biological Sciences, University of Pittsburgh, Pittsburgh, PA, United States
Eavesdropping, the interception of signals by unintended receivers, is an important component of the ecology and evolution of communication systems. Plants and microbes have complex communication systems with important consequences for agriculture, human health, and ecosystem functioning. Eavesdropping, however, has mostly been studied in animal systems. In this review, we argue that eavesdropping is an important force shaping the ecology and evolution of communication in these non-animal systems. To date, studying eavesdropping in plants and microbes has been limited by the fact that signaler “intention” is often unclear: distinguishing signals that evolved to convey information from unintended cues is particularly difficult in plants and microbes, and the fitness consequences of signaling are rarely measured. We describe some of the main examples of eavesdropping in plant and microbial communication and point out other murkier cases were the molecular and physiological basis of communication are well-understood, but the evolutionary implications have not been addressed. We argue that these systems provide experimental tractability to test some of the predicted ecological and evolutionary consequences of eavesdropping, and that the particularities of these systems can lead to an increased understanding of eavesdropping, and its importance in biological communication.
1. Introduction
Although the bright colors and conspicuous vocalizations of animal signals are most apparent to human observers, communication is fundamental to plant, fungal, and even microbial biology. The floral volatiles that plants produce to attract pollinators are one familiar example of communication in a non-animal system. Although plants and microbes differ from animals in the main sensory modalities they use to transmit and receive information, all signals are unified by a common theme: they evolved to convey information to an intended receiver, and are associated with a fitness benefit for the signaler (Laidre and Johnstone, 2013). And all signals, across the tree of life, are susceptible to a common threat: interception by unintended receivers known as “eavesdroppers.”
The term “eavesdropping” evokes auditory communication, but eavesdroppers can exploit any sensory modality through which signals are conveyed. In addition to vocalizations, unintended receivers intercept visual (Earley and Dugatkin, 2002), olfactory (Nieh et al., 2004), vibrational (Laumann et al., 2007), electric (Stoddard, 1999), and chemical (Hsueh et al., 2013) signals. In animal communication, the evolutionary and ecological significance of eavesdropping has long been appreciated (Zuk and Kolluru, 1998; Searcy and Nowicki, 2005). By contrast, studies of eavesdropping in non-animal systems are much rarer. As a result, animal communication has disproportionately influenced our understanding of the ecological and evolutionary significance of eavesdropping. Moreover, our models of signal form and function in non-animal systems often overlook eavesdropping as a crucial evolutionary force.
Here, we argue that eavesdropping is a fundamental force shaping the ecology and evolution of communication in non-animal systems. We begin by reviewing evidence for eavesdropping in non-animal systems, primarily focusing on plants and microbes. We identify hypotheses in the animal eavesdropping literature that have yet to be rigorously evaluated in non-animal systems, and highlight how plants and microbes are poised to advance our understanding of eavesdropping. We conclude by outlining productive avenues for future research.
1.1. Eavesdroppers, Signals, and Cues
Eavesdropping implies a communication system with a signaler, a signal, and one or more intended receivers. The raison d'etre for a signal is information transfer (Figure 1A). Signals have evolved to elicit a response in an intended receiver (Maynard-Smith and Harper, 2004). Generally, the response a signal elicits benefits both the signaler and the intended receiver (Figure 1A). Eavesdropping does not necessarily have an effect on the fitness of the signaler (Figure 1D) but often it is costly (Figure 1E). Competitors, or even worse, predators and parasites can gain information through eavesdropping that results in damage to the signaler. Most eavesdropping literature—including this review—focuses primarily on eavesdropping that is costly for the signaler, like eavesdropping predators and parasites, because it imposes conflicting selection on the signaler: the signaler benefits from its signal reaching the intended receiver, but pays a fitness cost when the signal is intercepted by an eavesdropper.
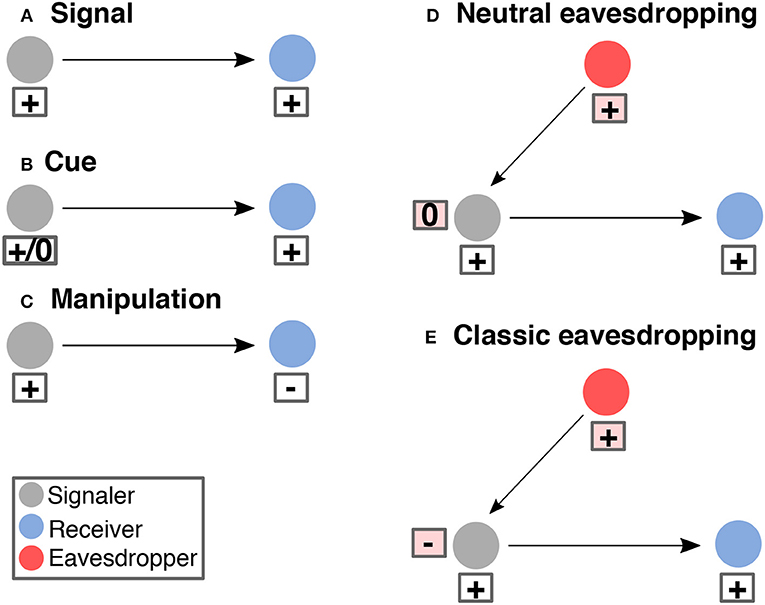
Figure 1. Different kinds communication systems. A signal is produced by a signaler to obtain a response from a receiver (A). Both signaler and receiver benefit from this interactions. Cues are unintended information that can be useful for the receiver (B). A signaler can also exploit sensory biases in the receiver to obtain a benefit at the cost of this receiver (C). Eavesdroppers intercept a signal to obtain beneficial information for them (D,E) often at the cost of the signaler (E).
Drawing from the animal communication literature, in this review we will distinguish between signals and cues. We use “cue” to refer to information produced inadvertently by a “signaler.” Cues are any piece of information from the environment that organisms use to change their physiology or behavior (Laidre and Johnstone, 2013). Cues are distinct from signals in that they did not evolve to convey information; instead, cues are unintended byproducts of other organismal functions (Figure 1B). Cues can convey a great deal of information about the organism that produced them (its proximity, nutritional state, sex, etc.), but communication is not their primary function. Chemical cues, for example, tend to be metabolic byproducts of other physiological processes that are passively emitted by the signaler (Figure 1B).
While discriminating signals from cues is certainly problematic in animals too, it can be especially difficult when plants and microbes act as receivers because they often respond to signals physiologically rather than behaviorally. Moreover, in contrast to the animal visual and auditory signals that we understand fairly well, the chemical signals that plants and microbes use to communicate with each other can be tricky to decode due to their high dimensionality (Raguso, 2008). Later in this review, we will highlight how principles and predictions from the animal eavesdropping literature can help us distinguish between signals and cues in plants and microbes.
2. How Do Plants and Microbes Communicate?
Plants produce a wide array of visual, olfactory, and chemical cues to communicate with animal and microbial mutualists, as well as with each other (Table 1) (Heil and Karban, 2010; Caruso and Parachnowitsch, 2016). Beyond the familiar bright colors, sweet-smelling volatiles, and nectar rewards associated with floral displays, plants also communicate via volatile organic compounds (“VOCs”) released from leaves, stems, and roots (Baldwin et al., 2006; Delory et al., 2016). These compounds diffuse through the air, water, or soil, and elicit responses from other plants, animals, and microbes (Baldwin et al., 2006; Heil and Karban, 2010). In addition to floral displays, an area in which plant communication is well-characterized is signal exchange between plants and mutualistic soil microbes. The root systems of nearly all land plants harbor mycorrhizal fungi that help their host plant tolerate drought and absorb soil nutrients (Bonfante and Genre, 2010). Legumes (plants in the family Fabaceae) also partner with soil bacteria called rhizobia to acquire nitrogen (Oldroyd, 2013). A crucial step in the formation of both mutualisms is chemical signal exchange, which plant and microbes use to locate and identify each other (Bonfante and Genre, 2010; Friesen, 2012; Oldroyd, 2013). These chemical conversations between plants and microbial mutualists occur via diffusible signals, such as flavonoids and strigolactones, and chemical gradients in the soil (Oldroyd, 2013).
Microbes also produce a wide array of secondary metabolites and respond to a broad range of chemicals in their environment and we are just starting to unravel the complex interactions and communication networks within microbial communities (Table 1) (e.g., Tan et al., 2014). Bacteria, for example, can transfer information through recognition of surface molecules (Blango and Mulvey, 2009), macromolecular contact-dependent delivery systems that transport effectors across cells (Hayes et al., 2010), small diffusible molecules and likely other physical forms of communication like sound waves (Matsuhashi et al., 1998), and electrical currents (Summers et al., 2010). The mechanisms of communication of microbes have mostly been described in bacteria; however, archaea, fungi, and protists all have their particular mechanisms of communication. In ascomycetes fungi, for example, complex chemical signaling is necessary to coordinate the fusion of germinating asexual spores in the formation and development of a fungal colony. This signaling system involves cell-cell contact and the alternation of signals and a coordinated rapid switch between two physiological stages (associated with signaling and response; Fleissner et al., 2009). And cell-cell signaling in social amoebas like Dictyostelium discoideum controls developmental programs (e.g., Bonner, 1970; Harwood et al., 1992; Pitt et al., 1992), kin recognition (Benabentos et al., 2009), multicellular aggregation (Tyson and Murray, 1989), behavioral changes (Darcy and Fisher, 1990; Dormann and Weijer, 2001), and the establishment of symbiosis (Shu et al., 2018).
3. Examples of Eavesdropping in Plants and Microbes
3.1. Plant-Animal Interactions
In plants, eavesdropping is most thoroughly understood in the context of plant-animal interactions mediated by floral displays (Table 2) (Schaefer et al., 2004). In many ways, flowers are analogous to the elaborate courtship displays that form the foundation of the animal eavesdropping literature. Flowers are visual (colorful) and chemical (scented) signals plants use to attract the animal pollinators they need to reproduce. The fitness benefit of floral signals for the plant, as well as the identity of the intended receiver, are clear and straightforward to measure. Species whose flowers share similar suites of traits (e.g., color, smell, shape) tend to be pollinated by the same animal taxa, a phenomenon known as a “pollination syndrome” (Fenster et al., 2004). The receiver of floral signals can often be identified based on these suites of shared traits, as well as models of animal sensory ecology that identify which species are capable of perceiving a given signal (Raguso, 2008; Schiestl and Johnson, 2013).
Flowers attract eavesdropping herbivores that damage leaf and stem tissue, rob pollen and nectar, and consume seeds. Using traps baited with individual components of the volatile cocktail produced by Canada thistle flowers, Theis (2006) demonstrated that some compounds attracted only pollinators, others attracted only herbivores, and some attracted both. Similarly, the dominant compound in the scent of flowers of the Neotropical orchid Dichaea pendula attracted bee pollinators and florivorous weevils (Nunes et al., 2016). These eavesdropping herbivores are a major source of selection on floral signals (Hanley et al., 2009; Kessler et al., 2013; Johnson et al., 2015; Santangelo et al., 2018). In a common garden, Santangelo et al. (2018) demonstrated that herbivores imposed stronger selection on inflorescence production and size than pollinators. A recent meta-analysis of phenotypic selection analyses came to a similar conclusion, finding that herbivore-mediated selection on floral traits was stronger than pollinator-mediated selection in two-thirds of cases (Johnson et al., 2015).
3.2. Plants as Eavesdroppers
Although controversial when first proposed, the idea that plants can receive and react to information is now firmly established (Heil and Karban, 2010; Kegge and Pierik, 2010; Karban et al., 2014; Caruso and Parachnowitsch, 2016). For example, plants respond to volatile compounds released by their herbivore-damaged neighbors by increasing their own herbivore resistance (Arimura et al., 2000; Dolch and Tscharntke, 2000; Dicke et al., 2003; Kegge and Pierik, 2010). Information is also transmitted from plant to plant through networks of mycorrhizal fungi that connect their root systems (Dicke et al., 2003; Babikova et al., 2013; Song et al., 2014).
Parasitic plants use plant-emitted volatiles to locate new hosts (Runyon et al., 2006). Dodder (Cuscuta pentagona) is a obligately parasitic plant that does not photosynthesize: instead, it obtains nutrients by attaching itself to the shoots and leaves of other plants. Runyon and colleagues found that dodder seedlings grow toward the volatile cocktails of their host plants. Incredibly, this response is host-specific: seedlings could distinguish between preferred and non-preferred hosts on the basis of their volatiles (Runyon et al., 2006). In at least one instance, plant eavesdropping on belowground signals helps them avoid parasitism. Many plants are attacked by parasitic nematodes that inflict substantial damage by grazing on plant roots or invading the root system to live as endoparasites. A recent study found that nematode pheromones called ascarosides, intraspecific signals regulating nematode social behavior and development, trigger defensive responses in plants (Table 2) (Manosalva et al., 2015). Plants aren't the only eavesdroppers exploiting these nematode pheromones. Ascarosides also trigger nematophagous fungi, predators of soil nematodes, to form the trapping structures they use to ensnare their nematode prey (Hsueh et al., 2013).
3.3. Quorum-Sensing in Bacteria
One of the most studied mechanisms of microbial communication is quorum-sensing in bacteria (Table 1). Quorum sensing refers to genetic regulation based on the concentration of a signal called an autoinducer. Autoinducers are produced in small concentration by the signaler bacterial cell and only cause a response after they have reached a certain threshold concentration (Miller and Bassler, 2001; Waters and Bassler, 2005; Keller and Surette, 2006). This bacterial response involves the production of more autoinducer, causing a positive feedback loop (Figure 2). Quorum-sensing signals are hypothesized to help microbes evaluate both the physical (i.e., diffusion) and social (i.e., population density) properties of the environment (Cornforth et al., 2014). Microbes can use these concentration-based systems to evaluate p how fast an extracellular compound will diffuse away (Redfield, 2002), and to regulate metabolite production based on population density; in other words, it allows bacteria to invest in a coordinated response only once a threshold density has been reached (Miller and Bassler, 2001; Waters and Bassler, 2005; Keller and Surette, 2006; Hawver et al., 2016).
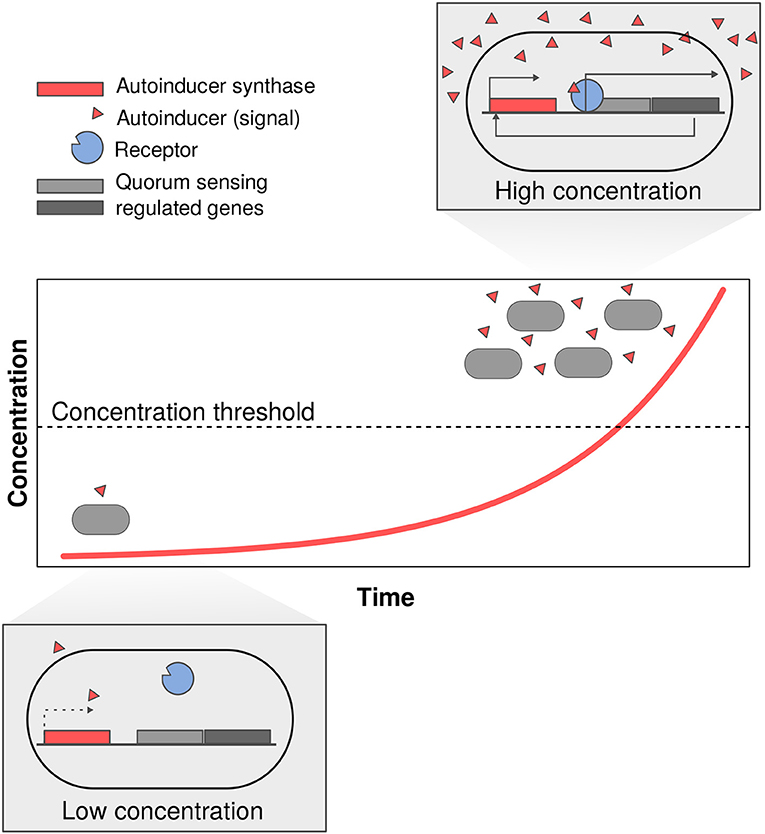
Figure 2. Quorum sensing is a system of communication that relies on the concentration of an autoinducer. In this system bacteria are constantly producing a small amount of signal (autoinducers; red triangles). As bacteria reach a larger density the concentration of autoinducer increases until it crosses a threshold after which it binds to the receptor and induces changes in expression. This changes often lead to an increase expression of autoinducer and thus act as positive feedbacks in high concentration of signal.
One of the clearest examples of eavesdropping in a microbial system involves quorum sensing. Myxococcus xanthus, a soil bacteria that feeds on other bacteria, eavesdrops on other bacterial signals to find its prey. It actively chases its prey and then is able to lyse and consume a wide range of bacterial species (Morgan et al., 2010). Like many animal predators, M. xanthus is able to eavesdrop on its prey signals and change its behavior accordingly. In this case, M. xanthus is able to recognize different quorum-sensing molecules (AHLs; Table 1) produced by Gram-negative bacteria, and respond by increasing predation activity (through increased motility, slower development of spores, increased germination of spores, and overall increased predation activity; Lloyd and Whitworth, 2017).
Eavesdropping on quorum sensing signals has been shown (through experiments and models) to increase eavesdropper fitness in soil bacteria, a hallmark of true eavesdropping (Chandler et al., 2012). Finally, LuxR/LuxI pair of genes show concordant patterns of evolution, suggesting co-evolution of signal and receptor (Lerat and Moran, 2004). Many bacteria have “solo” homologs of the LuxR-type protein (the response regulator) (i.e., in excess of those of the LuxI-type protein that functions as AHL synthase). Therefore, these bacteria can potentially respond to an autoinducer signal of another strain, but cannot produce their own, suggesting that eavesdropping could be on the purposes of these “solo” LuxR homologues (Case et al., 2008).
3.4. Host-Microbe Interactions
Bacteria are not the only ones that benefit from spying in microbial communication. Hosts also gather and react to crucial information about their biotic environment through eavesdropping in microbial quorum-sensing. The model legume Medicago truncatula modulates its gene expression in response to quorum-sensing autoinducers of a mutualist (Sinorhizobium meliloti) and a pathogen (P. aeruginosa) (Mathesius et al., 2003). Information flows in the other direction as well. Pathogenic microbes can eavesdrop on host signals to coordinate infection. In plant roots, for example, oomycete parasites can more easily find their host by eavesdropping on signals involved in attracting rhizobial partners (Hosseini et al., 2014). Inside the human gut E. coli and Salmonella respond to adrenaline and noradrenaline activating genes involved in virulence and motility (Lyte, 1992; Clarke et al., 2006). Similar eavesdropping mechanisms have been identified in plant pathogens. Xanthomonas oryzae pv. oryzae responds to the defense phytohormone salicylic acid by activating its quorum-sensing controlled virulence. More recently, Lopes and Sourjik (2018) showed that E. coli responds through chemotaxis to a wide array of hormones, and that the response (movement toward, or away from) is hormone-specific. The authors thus hypothesized that movement away from these hormones might allow the bacteria to escape certain host defenses (Table 2).
4. Why Study Eavesdropping in Non-animal Systems?
Organisms constantly respond to information in their environment, changing their growth, use of resources and ecological interactions. Thus, information plays an important role in ecological systems, mediating interactions, coexistence and community assembly (Kerényi et al., 2013; Juhász et al., 2017), and even affecting ecosystem functioning (Resco et al., 2009). Given the keystone ecological roles occupied by microbes and plants—both groups disproportionately influence nutrient cycling and overall ecosystem functioning—it is important to understand how they transmit and use information, and how as well as their information use shapes the stability of those systems. These communication networks pose a very interesting system to evaluate the ecological and evolutionary consequences of information transfer, signaling systems and eavesdropping.
Studying eavesdropping in non-animal systems has the potential to advance biological information theory in several ways. First, given the ecological and evolutionary significance of eavesdropping in animal systems, incorporating eavesdropping into plant and microbial biology is likely to yield new insights about the forces that shape information transfer. Second, expanding the study of eavesdropping beyond its traditional purview of behavioral ecology has the potential to generate new perspectives, and to test hypotheses that are difficult or impossible to test in most animal systems. Below, we outline how studies of eavesdropping could advance our understanding of plant and microbial communication biology, and how plant and microbial systems can be deployed to deepen our understanding of the evolutionary and ecological significance of eavesdropping.
4.1. Lessons From Animal Eavesdroppers for Plants and Microbes
What predictions and hypotheses from the animal eavesdropping literature are poised to advance our understanding communication biology in plants and microbes? With the notable exception of flowers and floral signals, it is often hard to distinguish between signals and cues in plants and microbes. Second, little is known about the fitness consequences of signaling in plants and microbes. With this in mind, we highlight two lessons from the animal eavesdropping literature that could significantly advance our understanding of the evolutionary forces shaping plant and microbial communications systems: (1) How to distinguish between signals and cues; and (2) Are a eavesdroppers a major agent of selection shaping the evolution of plant and microbial signals?
4.1.1. Distinguishing Between Signals and Cues
Distinguishing between signals and cues, as we noted earlier in this review, is a key step in establishing signaler-receiver relationships, and discriminating between intended receivers and eavesdroppers. In both plant and microbial systems, it remains challenging to establish that the compounds that elicit responses from plants and microbes are true signals, rather than cues. For example, while some volatile organic compounds released by plant leaves in response to herbivores are probably produced with the purpose of signaling—they are synthesized de novo in response to damage—others are mere byproducts of rupturing tissues and cells (Baldwin et al., 2002). From an evolutionary perspective, the distinction between signals and cues is an important one, given that signals and cues are governed by distinct selective pressures. By definition, signals and cues are associated with different fitness consequences for the signaler: while the receiver's response to a signal increases the signaler's fitness, cues may be selectively neutral, beneficial, or harmful for the individual that produced it (Laidre and Johnstone, 2013). Therefore, eavesdroppers almost always impose conflicting selection on signals, but not necessarily on cues.
There are three key properties of signals that distinguish them from cues. First, signals tend to be costly and specific, while cues are often cheap and non-specific (Table 1) (Grafen, 1990; Endler, 1992; Johnstone, 1998; Searcy and Nowicki, 2005). For example, flowers attract specific pollinators, and there is strong evidence that elements of floral displays are costly to produce (Knauer and Schiestl, 2015). The same is true for some of the molecules bacteria employ for quorum sensing (Table 1).
Second, relative to cues, signals are detectable. The reliability and detectability of cues is highly variable. Detectability depends on the state of the receiver (e.g., which receptors are being expressed), its genotype and the environmental context. Selection for signaling, by contrast, makes signals readily detectable by the intended receiver and in the appropriate context (Endler, 1992). As an example, it remains ambiguous whether the volatiles plants release are true signals, or merely cues whose primary function is something other than communication. Addressing this question can be tricky because herbivore damage necessarily releases some compounds as a direct result of wounding; many compounds implicated in plant-plant communication are plant hormones that play important roles in internal plant signaling as well (Heil and Karban, 2010); and most laboratory-based experiments that measure plant responses to volatiles use much higher volatile concentrations than are ecologically realistic (Baldwin et al., 2002). One possible way to resolve this question is to search for volatiles that exhibit heightened detectability (e.g., released at high initial concentrations or over especially long time periods) (Baldwin et al., 2002, 2006; Caruso and Parachnowitsch, 2016).
Finally, signal production (and specifically, the response a signal elicits in a receiver) increases signaler fitness. In plants and microbes, while the fitness consequences for the receiver are clear, the fitness consequences for the emitter are murkier (Karban and Maron, 2002; Heil and Karban, 2010). In quorum-sensing bacteria, it is not always clear who benefits from these interactions and at what cost for the other players. In mammals, for example, the immune system responds to P. aeruginosa quorum-sensing signals, but increasing evidence suggests that this response is not beneficial for the host and instead promotes P. aeruginosa establishment (reviewed in Kariminik et al., 2017). In leaf-epiphyte communities there are a number of strains that produce the same AHL, influencing motility in Pseudomonas syringae and therefore reducing its ability to colonize the host (Dulla and Lindow, 2009). However, the fitness consequences of this signaling system for the different players involved are not clear, nor why cross-communication has been maintained in different species that compete for some of the same resources.
Clever experimental manipulations have recently been deployed to decode the fitness consequences of information transfer in non-animal systems. Two powerful tools—available in model systems amenable to genetic manipulation—are “mute” organisms, which do not emit volatiles, and “deaf” organisms that lack the chemical receptors necessary to respond to them (Pierik et al., 2003; Baldwin et al., 2006; Paschold et al., 2006; Dicke and Baldwin, 2010). Comparing the fitnesses of mute and wild-type individuals is one way to quantify the benefits and costs of signaling for the signaler. An alternative approach is to measure the fitness consequences of being paired with a deaf partner that is unresponsive to a signaler's signal.
4.1.2. Do Eavesdroppers Shape Signal Evolution in Plants and Microbes?
The extent to which eavesdropping has shaped the evolution of information transfer systems deserves more consideration in non-animal systems. In animals, eavesdropping is a significant evolutionary force (Burk, 1982, 1988; Zuk and Kolluru, 1998). Signals that are intercepted by eavesdroppers are shaped evolutionary time by receiver-imposed selection favoring conspicuousness and eavesdropper-imposed selection favoring limited detectability (Zuk and Kolluru, 1998). The evolutionary impact of eavesdroppers is most evident in animal sexual signals. When eavesdropping predators are common, sexual signals tend to be less conspicuous than in populations where predation is rare (Endler, 1978). Over longer timescales, coevolutionary arms races between signalers, receivers, and eavesdroppers have been hypothesized to drive signal elaboration, facilitate speciation, and favor the evolution of novel signals (West-Eberhard, 1983; Zuk and Kolluru, 1998; Hoskin and Higgie, 2010). Finally, eavesdroppers may contribute to the maintenance of variation in signals by imposing fitness costs on the individuals that produce the most attractive signals (Strauss and Irwin, 2004; Heath and Stinchcombe, 2014; Wood et al., 2018). In the case of microbes, facultative cheating (eavesdropping) has favored the coexistence of multiple quorum sensing alleles in Bacillus subtilis, a classic case of balancing selection (Pollak et al., 2016).
Do plant and microbial signals reflect an evolutionary compromise between communicating with intended receivers and avoiding eavesdropping antagonists, as is the case with animal sexual signals (Zuk and Kolluru, 1998)? With the exception of floral signals, this question is relatively unexplored. Approaches that leverage the tools of evolutionary genomics have the potential to shed light on these processes. For example, a comparison of evolutionary rates of autoinducer synthetases and receptors between allopatric and sympatric bacteria could provide some insight into the importance of eavesdropping for microbial competition. In plants, the role of eavesdroppers in driving prezygotic reproductive isolation merits further investigation. If neighboring plant populations encounter different eavesdroppers that are attracted to different characteristics of a signal, selection to evade detection by local eavesdroppers could drive signal divergence, resulting in a breakdown of communication between signaler-receiver pairs from different populations.
Two plant signals where the evolutionary consequences of eavesdropping deserve to be explored in more detail are the root exudates plants use to communicate with mutualistic microbes, and floral volatiles. Many signals that plants use to communicate with microbial mutualists are also implicated in pathogenesis (Oldroyd, 2013). Furthermore, the ability to form mycorrhizal and rhizobial mutualisms is associated with susceptibility to parasites (Miller, 1993; Wood et al., 2018), consistent with the hypothesis that parasites rely on some of the same signals as mutualists to locate plant hosts. Second, in a recent review, Caruso and Parachnowitsch (2016) proposed a new eavesdropper on floral volatiles: other plants. They suggested that plants may eavesdrop on their neighbors' volatiles, arguing that eavesdropping on floral volatiles would be an effective way for plants to gain information about mating opportunities in their local neighborhood. Whether this eavesdropping occurs and how it might shape the evolution of floral signals remains to be tested.
Signal honesty is another foundational concept from the animal literature that deserves more extensive exploration in microbes and plants. Dishonest or manipulative signals are those where the signaler obtains a benefit at a cost to the receiver (Figure 1C). For example, flowers without pollinator rewards might either mimic other high reward flowers or exploit the sensory biases of the intended pollinators (e.g., Schiestl, 2004; Peter and Johnson, 2008). Manipulative signals exploit sensory biases to elicit a response in the intended receiver, usually at a fitness cost for the receiver (Laidre and Johnstone, 2013). These dishonest signals are a central component of plant reproductive biology: many plants produce deceptive flowers that advertise but do not offer a food reward; others mimic the smell of rotting flesh to attach carrion-feeding insects (Raguso, 2008). It is important to empirically determine whether a signal is dishonest. Some apparently honest signals may in fact be manipulative, and signals that appear to be dishonest may not be. For example, floral nectar is often assumed to function as a pollinator attractant, but its evolutionary ecology is surprisingly poorly understood (Parachnowitsch et al., 2019). Pyke (2016) argues that floral nectar should be considered a manipulative signal rather than a pollinator attractant, because it influences pollinator behavior. These studies illustrate that a detailed understanding of a signal's natural history—its ecology and function—is crucial.
Whether eavesdroppers intercept dishonest or manipulative signals, and what the fitness consequences of interception are for the signaler, receiver, and eavesdropper remain unknown. The maintenance of honest signals has a deep history of study in animal signals, and, generally speaking, the costs associated with producing a signal that honestly reflects the signaler's condition are thought to prevent cheaters from “faking it” (Hamilton and Zuk, 1982; Laidre and Johnstone, 2013). By imposing additional costs on the signaler, eavesdroppers may play an important role in enforcing signal honesty. However, signal honesty in general, and the role of eavesdroppers in particular, have received relatively little attention in non-animal systems (Knauer and Schiestl, 2015). One intriguing reason that manipulative signals deserve more attention in the context of eavesdropping is that eavesdropping on deceptive signals is likely to be costly for eavesdroppers, limiting the potential for eavesdroppers to exploit the signal. Generally speaking, data on the costs of eavesdropping are scarce in plants and microbes. One case in which costs limit the potential for eavesdroppers to capitalize on signal interception is found in a Pseudomonas example outlined earlier in this review. Mutant cells that fail to produce a quorum signal pay a high pleiotropic cost because the signal is required to regulate internal metabolic processes as well (Dandekar et al., 2012). Future studies should explore the generality of this result: are pleiotropic costs of eavesdropping primarily a characteristic in quorum-sensing bacteria, or does pleiotropy constrain eavesdropping in other systems and circumstances?
4.2. Plants and Microbes Unlock New Questions: Non-animal Models of Eavesdropping and Communication
Theories of animal communication can help guide research in the much less known world of plant and microbial communication. Often the literature in these communication systems comes from physiological and molecular studies that are more interested in the molecular mechanisms than in the ecological and evolutionary consequences. This means, on the one hand, that plant and microbial communication theory is lacking with respect to our knowledge in animal systems. But, on the other hand, it means that the mechanisms of signaling and perception are often well described—the molecular tools to study these systems and their consequences have already been developed. This provides multiple advantages for using microbial and plant systems to explore the ecological and evolutionary consequences of eavesdropping from new perspectives.
4.2.1. Evolutionary Genetics of Eavesdropping
To date, work on the evolutionary implications of eavesdropping has focused disproportionately on selection imposed by eavesdroppers on signals and signalers. However, selection is only one of two factors that influence evolutionary change (Figure 3). In the classic framework of quantitative genetics, trait evolution is determined by selection acting on the trait and its genetic correlation with other traits (Figure 3) (Lande and Arnold, 1983; Via and Lande, 1985; Brodie III et al., 1995). A genetic correlation between two traits arises when the same genes influence both traits, or when the genes influencing the two traits are tightly linked to each other (Falconer and Mackay, 1996). Genetic correlations are evolutionarily important because selection on a correlated trait causes indirect change in the focal trait by targeting the same underlying genes.
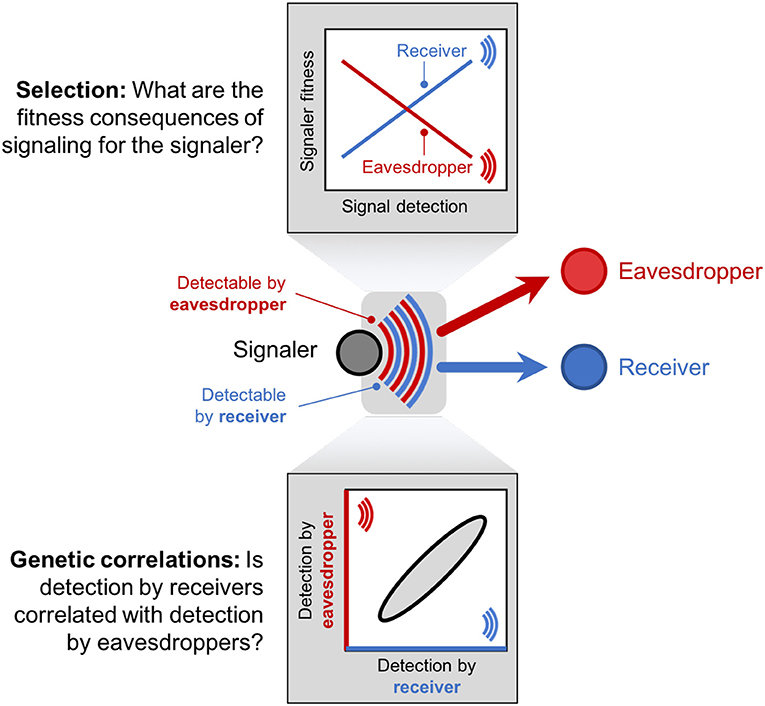
Figure 3. Signal evolution is governed by two elements: selection (the fitness consequences of signaling for the signaler) and genetic correlations (the relationship between signal detectability by receivers and signal detectability by eavesdroppers). Selection (top) imposed by eavesdroppers can be quantified by measuring the relationship between signaler fitness and signal detection by eavesdroppers. Genetic correlations (bottom) can be measured by treating signal detectability by eavesdroppers and receivers (the blue and red components of the signal in this diagram) as two separate traits in the signaler (i.e., two separate properties of the signal itself). When signal detection by eavesdroppers is positively correlated with detection by receivers, as illustrated in this example, eavesdroppers will influence how signaler-receiver communication evolves.
Using this quantitative genetic framework, eavesdropping can be incorporated into models of signal evolution by modeling signal detectability by intended receivers and eavesdroppers as two separate traits of the signaler that are genetically correlated (Figure 3) (Via and Lande, 1985). For example, attractiveness to pollinators and apparency to herbivores are both traits that can be measured on a focal plant. If, pollinators and herbivores home in on the same floral volatile, attractiveness to pollinators is genetically correlated with apparency to herbivores, and evolution of increased volatile production will attract more herbivores alongside pollinators. However, if pollinators and herbivores detect different volatiles, an evolutionary change in the volatiles that attract pollinators will not increase the plant's apparency to eavesdropping herbivores. Modeling signal detectability by receivers and eavesdroppers as traits of the signaler means that this framework is generalizable and can be applied to any signaler-receiver-eavesdropper triangle, regardless of study system.
As long as eavesdroppers impose fitness costs on signalers, a positive genetic correlation between signal detection by receivers and eavesdroppers will constrain the evolution of signal detectability, because any change in the signal that increases detection by receivers will increase apparency to eavesdroppers. When the two traits are uncorrelated, however, selection is free to reshape signals by exaggerating the features perceptible by eavesdroppers and minimizing those perceptible by eavesdroppers. Understanding the genetic correlation between detection by receivers and eavesdroppers is crucial to fully incorporate eavesdropping into models of signal evolution. Yet while we know a great deal about the fitness consequences of eavesdropping for signalers (Figure 3, top panel), few studies have measured the genetic correlation between signal detection by receivers and eavesdroppers (Figure 3, bottom panel), perhaps because measuring genetic correlations requires breeding designs and large sample sizes (Falconer and Mackay, 1996). By leveraging the quantitative genetic approach described above to model evolutionary change in multiple correlated traits, we can fully incorporate eavesdroppers into our framework of signal evolution, and advance our understanding of how constraint imposed by eavesdroppers has shaped signals and communications systems over evolutionary time.
4.2.2. Experimental Ecology and Evolution of Signals
Due to the molecular characterization of communication systems in bacteria, and the ease with which their genomes can be engineered, bacteria communication can be easily manipulated to measure the fitness effects of different quorum-sensing mutations in different ecological contexts (see, for example, Popat et al., 2012. In plants, this kind of genetic engineering is restricted to a few organisms (e.g., Pierik et al., 2003; Paschold et al., 2006), but plant genetics are easy to manipulate through planned crosses.
In bacteria, for example, genetic manipulation could illuminate the fitness costs and benefits of eavesdropping in the context of cooperation and cheating within the same bacterial species. These systems are susceptible to invasion by cheaters that gain the benefits without paying the costs. This is analogous to intraspecific eavesdroppers in animals, like satellite males, that are able to obtain the overall benefits of other male calls without paying for the costs of producing the signal (e.g., Olzer and Zuk, 2018). However, given the particular properties of quorum-sensing, there can be different kinds of quorum sensing cheaters. They could be (1) insensitive to the signal and therefore not produce more signal and other public goods, (2) use information from the signal without secreting public goods (eavesdroppers), or (3) respond to the signal by producing more signal stimulating the production of more public goods by others (manipulation). The frequency and consequences of these different kinds of cheaters have rarely been studied and different mutations lead to different consequences and a diversity of ecological and evolutionary dynamics that would be hard to predict from communication theory alone. In Pseudomonas aeruginosa, for example, it is common to find mutants that are insensitive to quorum-sensing signals (e.g., Cabrol et al., 2003) and these cheaters readily evolve in environments were cheating is beneficial (Sandoz et al., 2007). In contrast, “eavesdropper” mutants that cannot produce the autoinducer (even if they can sense others responses) pay significant pleiotropic costs because this autoinducer is necessary for regulation of other metabolic processes (Dandekar et al., 2012).
Furthermore, the ease of manipulation in these systems can allow us to investigate the ecological evolutionary consequences of signals produced not by the signaler itself, but their associated bacteria. In hyenas, for example, social odors that indicate species identity, sex and reproductive state are produced by bacterial fermentation in their odor glands (Theis et al., 2013). Similarly, microbes from flowers can alter the volatile composition affecting pollinator visitation (Herrera et al., 2013; Good et al., 2014; Schaeffer et al., 2017). What are the consequences of these signaling symbiotic associations? What are their evolutionary consequences? Manipulating the association between microbes and plants can be a fruitful strategy to evaluate the contributions of the microbiome for fitness and how these associations might affect evolution (Lau and Lennon, 2011, 2012).
Finally, using experimental evolution, it is possible to evaluate the evolutionary consequences of eavesdropping in microbes (and even in plants). In a recent example, Kimura et al. (2016) conducted directed evolution to increase the receptor sensitivity to a particular autoinducer. This study was motivated by increasing our understanding of the receptor function and activity, but similar methods can be used to understand the fitness effects of increased sensitivity and specialization under different ecological contexts (e.g., in the presence/absence of eavesdroppers or in the presence of eavesdroppers with varying degrees of fitness impacts on the signaler).
5. Summary
There are few clear examples of eavesdropping in microbial and plant communication. In this review, we argue that studies in these systems have been focused on the immediate causes and molecular mechanisms, while the evolutionary consequences are often overlooked. It is often hard to determine the “intentions” of a signaler (even worse if they are so unlike us). These organisms, however, provide great systems to test theoretical predictions and advance our understanding of biological communication. Furthermore, plants and microbes play important roles in their environments shaping the function of whole ecosystems. In principle, we might expect eavesdropping to have large-scale consequences that ramify up to the ecosystem scale. For example, signal exploitation by eavesdroppers could influence community composition and assembly although to our knowledge such an effect has not yet been empirically demonstrated. Finally, understanding the ecological and evolutionary consequences of eavesdropping and communication in plants and microbes can have important effects on agriculture and human health. Intercepting quorum-sensing signals has been proposed as a potential alternative to classic antibiotics (Roy et al., 2011) but a better understanding of genetic correlations, costs, and pleiotropic effects would give us a better idea of the potential for these methods to work over time.
Author Contributions
MR-G and CW conceived the paper, reviewed the literature, and wrote the manuscript.
Funding
This work was supported by funding from the Department of Biological Sciences at the University of Pittsburgh to CW. MR-G was supported by a PEEP Postdoctoral Fellowship from the Department of Biological Sciences at the University of Pittsburgh.
Conflict of Interest
The authors declare that the research was conducted in the absence of any commercial or financial relationships that could be construed as a potential conflict of interest.
Acknowledgments
We are grateful to Ximena Bernal for inviting us to contribute this manuscript to this Research Topic.
References
Andersson, S. (2000). The cost of flowers in Nigella degenii inferred from flower and perianth removal experiments. Int. J. Pl 161, 903–908. doi: 10.1086/317558
Arimura, G., Ozawa, R., Shimoda, Y., Nishioka, T., Bolandß, W., and Takabayashi, J. (2000). Herbivore-induced volatiles from lima bean leaves elicit defense genes in uninfested leaves. Nature 406, 512–515. doi: 10.1038/35020072
Babikova, Z., Gilbert, L., Bruce, T. J., Birkett, M., Caulfield, J. C., Woodcock, C., et al. (2013). Underground signals carried through common mycelial networks warn neighbouring plants of aphid attack. Ecol. Lett. 16, 835–843. doi: 10.1111/ele.12115
Baldwin, I. T., Halitschke, R., Paschold, A., Dahl, C. C. V., and Preston, C. A. (2006). Volatile signaling in plant-plant in the genomics era. Science 812–816. doi: 10.1126/science.1118446
Baldwin, I. T., Kessler, A., and Halitschke, R. (2002). Volatile signaling in plant-plant-herbivore interactions: what is real? Curr. Opin. Plant Biol. 5, 1–4. doi: 10.1016/S1369-5266(02)00263-7
Benabentos, R., Hirose, S., Sucgang, R., Curk, T., Katoh, M., Ostrowski, E. A., et al. (2009). Polymorphic members of the lag gene family mediate kin discrimination in dictyostelium. Curr. Biol. 19, 567–572. doi: 10.1016/j.cub.2009.02.037
Blango, M. G., and Mulvey, M. A. (2009). Bacterial landlines: contact-dependent signaling in bacterial populations. Curr. Opin. Microbiol. 12, 177–181. doi: 10.1016/j.mib.2009.01.011
Bonfante, P., and Genre, A. (2010). Mechanisms underlying beneficial plant-fungus interactions in mycorrhizal symbiosis. Nat. Commun. 1, 1–11. doi: 10.1038/ncomms1046
Bonner, J. T. (1970). Induction of stalk cell differentiation by cyclic AMP in the cellular slime mold Dictyostelium discoideum. Proc. Natl. Acad. Sci. U.S.A. 65, 110–113. doi: 10.1073/pnas.65.1.110
Brodie, E. D. III, Moore, A. J., and Janzen, F. J. (1995). Visualizing and quantifying natural selection. Trends Ecol. Evol. 10, 313–318. doi: 10.1016/S0169-5347(00)89117-X
Burk, T. (1982). Evolutionary significance of predation on sexually signalling males. Florida Entomol. 65, 90–104. doi: 10.2307/3494148
Burk, T. (1988). Acoustic signals, arms races and the costs of honest signalling. Florida Entomol. 71, 400–409. doi: 10.2307/3494999
Cabrol, S., Olliver, A., Pier, G. B., Andremont, A., and Ruimy, R. (2003). Transcription of quorum-sensing system genes in clinical and environmental isolates of Pseudomonas aeruginosa. J. Bacteriol. 185, 7222–7230. doi: 10.1128/JB.185.24.7222-7230.2003
Caruso, C. M., and Parachnowitsch, A. L. (2016). Do plants eavesdrop on floral scent signals? Trends Plant Sci. 21, 9–15. doi: 10.1016/j.tplants.2015.09.001
Case, R. J., Labbate, M., and Kjelleberg, S. (2008). AHL-driven quorum-sensing circuits: their frequency and function among the Proteobacteria. ISME J. 2, 345–349. doi: 10.1038/ismej.2008.13
Chandler, J. R., Heilmann, S., Mittler, J. E., and Greenberg, E. P. (2012). Acyl-homoserine lactone-dependent eavesdropping promotes competition in a laboratory co-culture model. ISME J. 6, 2219–2228. doi: 10.1038/ismej.2012.69
Clarke, M. B., Hughes, D. T., Zhu, C., Boedeker, E. C., and Sperandio, V. (2006). The QseC sensor kinase: a bacterial adrenergic receptor. Proc. Natl. Acad. Sci. U.S.A. 103, 10420–10425. doi: 10.1073/pnas.0604343103
Cornforth, D. M., Popat, R., McNally, L., Gurney, J., Scott-Phillips, T. C., Ivens, A., et al. (2014). Combinatorial quorum sensing allows bacteria to resolve their social and physical environment. Proc. Natl. Acad. Sci. U.S.A. 111, 4280–4284. doi: 10.1073/pnas.1319175111
Dandekar, A. A., Chugani, S., and Greenberg, E. P. (2012). Bacterial quorum sensing and metabolic incentives to cooperate. Science 338, 264–266. doi: 10.1126/science.1227289
Darcy, P. K., and Fisher, P. R. (1990). Pharmacological evidence for a role for cyclic AMP signalling in Dictyostelium discoideum slug behaviour. J. Cell Sci. 96, 661–668.
Delory, B. M., Delaplace, P., Fauconnier, M.-l., and Jardin, P. (2016). Root-emitted volatile organic compounds : can they mediate belowground plant-plant interactions? Plant Soil 402, 1–26. doi: 10.1007/s11104-016-2823-3
Denarie, J., and Debelle, F. (1996). Rhizobium lipo-chitooligosaccharide nodulation factors: signaling molecules mediating recognition and morphogenesis. Annu. Rev. Biochem. 65, 503–545. doi: 10.1146/annurev.bi.65.070196.002443
Dicke, M., Agrawal, A. A., and Bruin, J. (2003). Plants talk, but are they deaf? Trends Plant Sci. 8, 403–405. doi: 10.1016/S1360-1385(03)00183-3
Dicke, M., and Baldwin, I. T. (2010). The evolutionary context for herbivore-induced plant volatiles: beyond the 'cry for help'. Trends Plant Sci. 15, 167–175. doi: 10.1016/j.tplants.2009.12.002
Diggle, S. P., Gardner, A., West, S. A., and Griffin, A. S. (2007). Evolutionary theory of bacterial quorum sensing: when is a signal not a signal? Philos. Trans. R. Soc. B Biol. Sci. 362, 1241–1249. doi: 10.1098/rstb.2007.2049
Dolch, R., and Tscharntke, T. (2000). Defoliation of alders (Alnus glutinosa) affects herbivory by leaf beetles on undamaged neighbours. Oecologia 125, 504–511. doi: 10.1007/s004420000482
Dormann, D., and Weijer, C. J. (2001). Propagating chemoattractant waves coordinate periodic cell movement in Dictyostelium slugs. Development 128, 4535–4543.
Dulla, G. F. J., and Lindow, S. E. (2009). Acyl-homoserine lactone-mediated cross talk among epiphytic bacteria modulates behavior of pseudomonas syringae on leaves. ISME J. 3, 825–834. doi: 10.1038/ismej.2009.30
Earley, R. L., and Dugatkin, L. A. (2002). Eavesdropping on visual cues in green swordtail (Xiphophorus helleri) fights: a case for networking. Proc. R. Soc. B 269, 943–952. doi: 10.1098/rspb.2002.1973
Endler, J. A. (1992). Signals, signal conditions, and the direction of evolution. Am. Nat. 139(Suppl.), S125–S152. doi: 10.1086/285308
Falconer, D. S., and Mackay, T. F. (1996). Introduction to Quantitative Genetics, 4th Edn. Essex: Prentice Hall.
Fenster, C. B., Armbruster, W. S., Wilson, P., Dudash, M. R., and Thomson, J. D. (2004). Pollination syndromes and floral specialiation. Annu. Rev. Ecol. Evol. Syst. 35, 375–403. doi: 10.1146/annurev.ecolsys.34.011802.132347
Fleissner, A., Leeder, A. C., Roca, M. G., Read, N. D., and Glass, N. L. (2009). Oscillatory recruitment of signaling proteins to cell tips promotes coordinated behavior during cell fusion. Proc. Natl. Acad. Sci. U.S.A. 106, 19387–19392. doi: 10.1073/pnas.0907039106
Friesen, M. L. (2012). Widespread fitness alignment in the legume-rhizobium symbiosis. New Phytol. 194, 1096–1111. doi: 10.1111/j.1469-8137.2012.04099.x
Fuqua, C., Parsek, M. R., and Greenberg, E. P. (2001). Regulation of gene expression by cell-to-cell communication: acyl-homoserine lactone quorum sensing. Annu. Rev. Genet. 35, 439–468. doi: 10.1146/annurev.genet.35.102401.090913
Good, A. P., Gauthier, M. P. L., Vannette, R. L., and Fukami, T. (2014). Honey bees avoid nectar colonized by three bacterial species, but not by a yeast species, isolated from the bee gut. PLoS ONE 9:e86494. doi: 10.1371/journal.pone.0086494
Grafen, A. (1990). Biological signals and handicaps. J. Theor. Biol. 144, 517–546. doi: 10.1016/S0022-5193(05)80088-8
Hamilton, W. D., and Zuk, M. (1982). Heritable true fitness and bright birds: a role for parasites? Science 218, 384LP–387. doi: 10.1126/science.7123238
Hanley, M. E., Lamont, B. B., Armbruster, W. S., and Hanley, M. (2009). Pollination and plant defence traits co-vary in Western Australian Hakeas. New Phytol. 182, 251–260. doi: 10.1111/j.1469-8137.2008.02709.x
Harwood, A. J., Hopper, N. A., Simon, M. N., Bouzid, S., Veron, M., and Williams, J. G. (1992). Multiple roles for cAMP-dependent protein kinase during Dictyostelium development. Dev. Biol. 149, 90–99. doi: 10.1016/0012-1606(92)90266-J
Hawver, L. A., Jung, S. A., and Ng, W. L. (2016). Specificity and complexity in bacterial quorum-sensing systemsa. FEMS Microbiol. Rev. 40, 738–752. doi: 10.1093/femsre/fuw014
Hayes, C. S., Aoki, S. K., and Low, D. A. (2010). Bacterial contact-dependent delivery systems. Annu. Rev. Genet. 44, 71–90. doi: 10.1146/annurev.genet.42.110807.091449
Heath, K. D., and Stinchcombe, J. R. (2014). Explaining mutualism variation: a new evolutionary paradox? Evolution 68, 309–317. doi: 10.1111/evo.12292
Heil, M., and Karban, R. (2010). Explaining evolution of plant communication by airborne signals. Trends Ecol. Evol. 25, 137–144. doi: 10.1016/j.tree.2009.09.010
Herrera, C. M., Pozo, M. I., and Medrano, M. (2013). Yeasts in nectar of an early-blooming herb: sought by bumble bees, detrimental to plant fecundity. Ecology 94, 273–279. doi: 10.1890/12-0595.1
Hoballah, M. E., Kollner, T. G., Degenhardt, J., and Turlings, T. C. J. (2004). Costs of induced volatile production in maize. Oikos 105, 168–180. doi: 10.1111/j.0030-1299.2004.12831.x
Hoskin, C. J., and Higgie, M. (2010). Speciation via species interactions: the divergence of mating traits within species. Ecol. Lett. 13, 409–420. doi: 10.1111/j.1461-0248.2010.01448.x
Hosseini, S., Heyman, F., Olsson, U., Broberg, A., Funck Jensen, D., and Karlsson, M. (2014). Zoospore chemotaxis of closely related legume-root infecting Phytophthora species towards host isoflavones. Plant Pathol. 63, 708–714. doi: 10.1111/ppa.12137
Hsueh, Y.-P., Mahanti, P., and Schroeder, F. C. (2013). Nematode-trapping fungi eavesdrop on nematode pheromones. Curr. Biol. 23, 83–86. doi: 10.1016/j.cub.2012.11.035
Johnson, M. T., Campbell, S. A., and Barrett, S. C. (2015). Evolutionary interactions between plant reproduction and defense against herbivores. Annu. Rev. Ecol. Evol. Syst. 46, 191–213. doi: 10.1146/annurev-ecolsys-112414-054215
Johnstone, R. A. (1998). Conspiratorial whispers and conspicuous displays. Evolution 52, 1554–1563. doi: 10.1111/j.1558-5646.1998.tb02236.x
Juhász, J., Bihary, D., Jády, A., Pongor, S., and Ligeti, B. (2017). Differential signal sensitivities can contribute to the stability of multispecies bacterial communities. Biol. Direct 12, 1–10. doi: 10.1186/s13062-017-0192-3
Karban, R., and Maron, J. L. (2002). The fitness consequences of interspecific eavesdropping between plants. Ecology 83, 1209–1213. doi: 10.1890/0012-9658(2002)083[1209:TFCOIE]2.0.CO;2
Karban, R., Wetzel, W. C., Shiojiri, K., Ishizaki, S., Ramirez, S. R., and Blande, J. D. (2014). Deciphering the language of plant communication: volatile chemotypes of sagebrush. J. Physiol. 204, 380–385. doi: 10.1111/nph.12887
Kariminik, A., Baseri-Salehi, M., and Kheirkhah, B. (2017). Pseudomonas aeruginosa quorum sensing modulates immune responses: an updated review article. Immunol. Lett. 190, 1–6. doi: 10.1016/j.imlet.2017.07.002
Kegge, W., and Pierik, R. (2010). Biogenic volatile organic compounds and plant competition. Trends Plant Sci. 15, 126–132. doi: 10.1016/j.tplants.2009.11.007
Keller, L., and Surette, M. G. (2006). Communication in bacteria: an ecological and evolutionary perspective. Nat. Rev. Microbiol. 4, 249–258. doi: 10.1038/nrmicro1383
Kerényi, Á., Bihary, D., Venturi, V., and Pongor, S. (2013). Stability of multispecies bacterial communities: signaling networks may stabilize microbiomes. PLoS ONE 8:e57947. doi: 10.1371/journal.pone.0057947
Kessler, D., Diezel, C., and Thomas, D. G. C. (2013). Petunia flowers solve the defence/apparency dilemma of pollinator attraction by deploying complex floral blends. Ecol. Lett. 16, 299–306. doi: 10.1111/ele.12038
Kimura, Y., Tashiro, Y., Saito, K., Kawai-Noma, S., and Umeno, D. (2016). Directed evolution of Vibrio fischeri LuxR signal sensitivity. J. Biosci. Bioeng. 122, 533–538. doi: 10.1016/j.jbiosc.2016.04.010
Knauer, A. C., and Schiestl, F. P. (2015). Bees use honest floral signals as indicators of reward when visiting flowers. Ecol. Lett. 18, 135–143. doi: 10.1111/ele.12386
Laidre, M. E., and Johnstone, R. A. (2013). Animal signals. Curr. Biol. 23, 829–833. doi: 10.1016/j.cub.2013.07.070
Lande, R., and Arnold, S. J. (1983). the Measurement of selection on correlated characters. Evolution 37, 1210–1226. doi: 10.1111/j.1558-5646.1983.tb00236.x
Lau, J. A., and Lennon, J. T. (2011). Evolutionary ecology of plant-microbe interactions: soil microbial structure alters selection on plant traits. New Phytol. 192, 215–224. doi: 10.1111/j.1469-8137.2011.03790.x
Lau, J. A., and Lennon, J. T. (2012). Rapid responses of soil microorganisms improve plant fitness in novel environments. Proc. Natl. Acad. Sci. U.S.A. 109, 14058–14062. doi: 10.1073/pnas.1202319109
Laumann, R. A., Blassioli Moraes, M. C., Cokl, A., and Borges, M. (2007). Eavesdropping on sexual vibratory signals of stink bugs (Hemiptera : Pentatomidae) by the egg parasitoid Telenomus podisi. Anim. Behav. 73, 637–649. doi: 10.1016/j.anbehav.2006.09.011
Lerat, E., and Moran, N. A. (2004). The evolutionary history of quorum-sensing systems in bacteria. Mol. Biol. Evol. 21, 903–913. doi: 10.1093/molbev/msh097
Lloyd, D. G., and Whitworth, D. E. (2017). The myxobacterium Myxococcus xanthus can sense and respond to the quorum signals secreted by potential prey organisms. Front. Microbiol. 8:439. doi: 10.3389/fmicb.2017.00439
Lopes, J. G., and Sourjik, V. (2018). Chemotaxis of Escherichia coli to major hormones and polyamines present in human gut. ISME J. 12, 2736–2747. doi: 10.1038/s41396-018-0227-5
Lyon, G. J., and Novick, R. P. (2004). Peptide signaling in Staphylococcus aureus and other Gram-positive bacteria. Peptides 25, 1389–1403. doi: 10.1016/j.peptides.2003.11.026
Lyte, M. (1992). Catecholamine induced growth of gram negative bacteria. Life Sci. 50, 203–212. doi: 10.1016/0024-3205(92)90273-R
Manosalva, P., Manohar, M., Von Reuss, S. H., Chen, S., Koch, A., Kaplan, F., et al. (2015). Conserved nematode signalling molecules elicit plant defenses and pathogen resistance. Nat. Commun. 6, 1–8. doi: 10.1038/ncomms8795
Mathesius, U., Mulders, S., Gao, M., Teplitski, M., Caetano-anolle, G., Rolfe, B. G., et al. (2003). Extensive and specific responses of a eukaryote to bacterial quorum-sensing signals. Proc. Natl. Acad. Sci. U.S.A. 100, 1444–1449. doi: 10.1073/pnas.262672599
Matsuhashi, M., Pankrushina, A. N., Takeuchi, S., Ohshima, H., Miyoi, H., Endoh, K., et al. (1998). Production of sound waves by bacterial cells and the response of bacterial cells to sound. J. Gen. Appl. Microbiol. 44, 49–55. doi: 10.2323/jgam.44.49
Miller, M. B., and Bassler, B. L. (2001). Quorum sensing in bacteria. Annu. Rev. Microbiol. 55, 165–169. doi: 10.1146/annurev.micro.55.1.165
Miller, R. M. (1993). Nontarget and ecological effects of transgenically altered disease resistance in crops–possible effects on the mycorrhizal symbiosis. Mol. Ecol. 2, 327–335. doi: 10.1111/j.1365-294X.1993.tb00025.x
Morgan, A. D., MacLean, R. C., Hillesland, K. L., and Velicer, G. J. (2010). Comparative analysis of myxococcus predation on soil bacteria. Appl. Environ. Microbiol. 76, 6920–6927. doi: 10.1128/AEM.00414-10
Nieh, J. C., Barreto, L. S., Contrera, F. A. L., and Imperatriz-fonseca, V. L. (2004). Olfactory eavesdropping by a competitively foraging stingless bee, Trigona spinipes. Proc. R. Soc. B 271, 1633–1640. doi: 10.1098/rspb.2004.2717
Nunes, C. E., Peñaflor, M. F. G., Bento, J. M. S., Salvador, M. J., and Sazima, M. (2016). The dilemma of being a fragrant flower: the major floral volatile attracts pollinators and florivores in the euglossine-pollinated orchid Dichaea pendula. Oecologia 182, 933–946. doi: 10.1007/s00442-016-3703-5
Oldroyd, G. E. D. (2013). Speak, friend, and enter: Signalling systems that promote beneficial symbiotic associations in plants. Nat. Rev. Microbiol. 11, 252–263. doi: 10.1038/nrmicro2990
Olzer, R., and Zuk, M. (2018). Obligate, but not facultative, satellite males prefer the same male sexual signal characteristics as females. Anim. Behav. 144, 37–43. doi: 10.1016/j.anbehav.2018.07.014
Parachnowitsch, A. L., Manson, J. S., and Sletvold, N. (2019). Evolutionary ecology of nectar. Ann. Bot. 123, 247–261. doi: 10.1093/aob/mcy132
Paschold, A., Halitschke, R., and Baldwin, I. T. (2006). Using ‘mute’ plants to translate volatile signals. Plant J. 45, 275–291. doi: 10.1111/j.1365-313X.2005.02623.x
Peter, C. I., and Johnson, S. D. (2008). Mimics and magnets : the importance of color and ecological facilitation in floral deception. Ecology 89, 1583–1595. doi: 10.1890/07-1098.1
Pierik, R., Visser, E. J. W., Kroon, H. D. E., and Voesenek, L. A. C. J. (2003). Ethylene is required in tobacco to successfully compete with proximate neighbours. Plant Cell Environ. 26, 1229–1234. doi: 10.1046/j.1365-3040.2003.01045.x
Pitt, G. S., Milona, N., Borleis, J., Lin, K. C., Reed, R. R., and Devreotes, P. N. (1992). Structurally distinct and stage-specific adenylyl cyclase genes play different roles in Dictyostelium development. Cell 69, 305–315. doi: 10.1016/0092-8674(92)90411-5
Pollak, S., Omer-Bendori, S., Even-Tov, E., Lipsman, V., Bareia, T., Ben-Zion, I., et al. (2016). Facultative cheating supports the coexistence of diverse quorum-sensing alleles. Proc. Natl. Acad. Sci. U.S.A. 113, 2152–2157. doi: 10.1073/pnas.1520615113
Popat, R., Crusz, S. A., Messina, M., Williams, P., West, S. A., and Diggle, S. P. (2012). Quorum-sensing and cheating in bacterial biofilms. Proc. Biol. Sci. R. Soc. 279, 4765–4771. doi: 10.1098/rspb.2012.1976
Pyke, G. H. (1991). What does it cost a plant to produce floral nectar? Nature 350, 58–59. doi: 10.1038/350058a0
Pyke, G. H. (2016). Floral nectar: pollinator attraction or manipulation? Trends Ecol. Evol. 31, 339–341. doi: 10.1016/j.tree.2016.02.013
Raguso, R. A. (2008). Wake up and smell the roses: the ecology and evolution of floral scent. Annu. Rev. Ecol. Evol. Syst. 39, 549–569. doi: 10.1146/annurev.ecolsys.38.091206.095601
Redfield, R. J. (2002). Is quorum sensing a side effect of diffusion sensing? Trends Microbiol. 10, 365–370. doi: 10.1016/S0966-842X(02)02400-9
Resco, V., Hartwell, J., and Hall, A. (2009). Ecological implications of plants' ability to tell the time. Ecol. Lett. 12, 583–592. doi: 10.1111/j.1461-0248.2009.01295.x
Roy, V., Adams, B. L., and Bentley, W. E. (2011). Developing next generation antimicrobials by intercepting AI-2 mediated quorum sensing. Enzyme Microb. Technol. 49, 113–123. doi: 10.1016/j.enzmictec.2011.06.001
Runyon, J. B., Mescher, M. C., and Moraes, C. M. D. (2006). Chemical plants cues guide host by location and host selection parasitic. Science 313, 1964–1967. doi: 10.1126/science.1131371
Sandoz, K. M., Mitzimberg, S. M., and Schuster, M. (2007). Social cheating in Pseudomonas aeruginosa quorum sensing. Proc. Natl. Acad. Sci. U.S.A. 104, 15876–15881. doi: 10.1073/pnas.0705653104
Santangelo, J. S., Thompson, K. A., and Johnson, M. T. J. (2018). Herbivores and plant defenses affect selection on plant reproductive traits more strongly than pollinators. J. Evol. Biol. 32, 4–18. doi: 10.1111/jeb.13392
Schaefer, H. M., Schaefer, V., and Levey, D. J. (2004). How plant-animal interactions signal new insights in communication. Trends Ecol. Evol. 19, 577–584. doi: 10.1016/j.tree.2004.08.003
Schaeffer, R. N., Mei, Y. Z., Andicoechea, J., Manson, J. S., and Irwin, R. E. (2017). Consequences of a nectar yeast for pollinator preference and performance. Funct. Ecol. 31, 613–621. doi: 10.1111/1365-2435.12762
Schiestl, F. P. (2004). Floral evolution and pollinator mate choice in a sexually deceptive orchid. J. Evol. Biol. 17, 67–75. doi: 10.1046/j.1420-9101.2003.00650.x
Schiestl, F. P., and Johnson, S. D. (2013). Pollinator-mediated evolution of floral signals. Trends Ecol. Evol. 28, 307–315. doi: 10.1016/j.tree.2013.01.019
Searcy, W. A., and Nowicki, S. (2005). The Evolution of Animal Communication: Reliability and Deception in Signaling Systems. Princeton, NJ: Princeton University Press.
Shu, L., Zhang, B., Queller, D. C., and Strassmann, J. E. (2018). Burkholderia bacteria use chemotaxis to find social amoeba Dictyostelium discoideum hosts. ISME J. 12, 1977–1993. doi: 10.1038/s41396-018-0147-4
Sletvold, N., Trunschke, J., Smit, M., Verbeek, J., and Agren, J. (2016). Strong pollinator-mediated selection for increased flower brightness. Evolution 70, 716–724. doi: 10.1111/evo.12881
Song, Y. Y., Ye, M., Li, C., He, X., Zhu-salzman, K., Wang, R. L., et al. (2014). Hijacking common mycorrhizal networks. Sci. Rep. 4, 1–8. doi: 10.1038/srep03915
Stoddard, P. K. (1999). Predation enhances complexity in the evolution of electric fish signals. Nature 400, 254–256. doi: 10.1038/22301
Strauss, S. Y., and Irwin, R. E. (2004). Ecological and evolutionary consequences of multispecies plant-animal interactions. Annu. Rev. Ecol. Evol. Syst. 35, 435–466. doi: 10.1146/annurev.ecolsys.35.112202.130215
Summers, Z. M., Fogarty, H. E., Leang, C., Franks, A., Mlvankar, N. S., and Lovley, D. R. (2010). Direct exchange of electrons within aggregates of an evolved syntrophic coculture of anaerobic bacteria. Science 330, 819–824. doi: 10.1126/science.1196526
Tan, C. H., Koh, K. S., Xie, C., Tay, M., Zhou, Y., Williams, R., et al. (2014). The role of quorum sensing signalling in EPS production and the assembly of a sludge community into aerobic granules. ISME J. 8, 1186–1197. doi: 10.1038/ismej.2013.240
Theis, K. R., Venkataraman, A., Dycus, J. A., Koonter, K. D., Schmitt-Matzen, E. N., Wagner, A. P., et al. (2013). Symbiotic bacteria appear to mediate hyena social odors. Proc. Natl. Acad. Sci. U.S.A. 110, 19832–19837. doi: 10.1073/pnas.1306477110
Theis, N. (2006). Fragrance of Canada thistle (Cirsium arvense) attracts both floral herbivores and pollinators. J. Chem. Ecol. 32, 917–927. doi: 10.1007/s10886-006-9051-x
Tyson, J. J., and Murray, J. D. (1989). Cyclic AMP waves during aggregation of Dictyostelium amoebae. Development 106, 421–426.
Vendeville, A., Winzer, K., Heurlier, K., Tang, C. M., and Hardie, K. R. (2005). Making 'sense' of metabolism: autoinducer-2, LuxS and pathogenic bacteria. Nat. Rev. Microbiol. 3, 383–396. doi: 10.1038/nrmicro1146
Via, S., and Lande, R. (1985). Genotype-environment interaction and the evolution of phenotypic plasticity author (s): sara via and russell lande reviewed Work (s): published by : society for the study of evolution stable URL : http://www.jstor.org/stable/2408649.EVOLUTIONOFPHE. Evolution 39, 505–522.
Waters, C. M., and Bassler, B. L. (2005). Awareness on ex-gratia compensation scheme among medical department staff in a tertiary government hospital in Kuala Lumpur. Annu. Rev. Cell Dev. Biol. 63, 319–346. doi: 10.1146/annurev.cellbio.21.012704.131001
Waters, M. T., Gutjahr, C., Bennett, T., and Nelson, D. C. (2017). Strigolactone signaling and evolution. Annu. Rev. Plant Biol. 68, 291–322. doi: 10.1146/annurev-arplant-042916-040925
West-Eberhard, M. J. (1983). Sexual selection, social competition, and speciation. Q. Rev. Biol. 58, 155–183.
Wood, C. W., Pilkington, B. L., Vaidya, P., Biel, C., and Stinchcombe, J. R. (2018). Genetic conflict with a parasitic nematode disrupts the legume-rhizobia mutualism. Evol. Lett. 2, 233–245. doi: 10.1002/evl3.51
Keywords: eavesdropping, signal, cue, plant volatiles, quorum-sensing, cooperation, plant communication, microbial communication
Citation: Rebolleda-Gómez M and Wood CW (2019) Unclear Intentions: Eavesdropping in Microbial and Plant Systems. Front. Ecol. Evol. 7:385. doi: 10.3389/fevo.2019.00385
Received: 25 January 2019; Accepted: 24 September 2019;
Published: 15 October 2019.
Edited by:
Ximena E. Bernal, Purdue University, United StatesReviewed by:
Kristina Linnea Hillesland, University of Washington Bothell, United StatesAmy L. Parachnowitsch, University of New Brunswick Fredericton, Canada
Copyright © 2019 Rebolleda-Gómez and Wood. This is an open-access article distributed under the terms of the Creative Commons Attribution License (CC BY). The use, distribution or reproduction in other forums is permitted, provided the original author(s) and the copyright owner(s) are credited and that the original publication in this journal is cited, in accordance with accepted academic practice. No use, distribution or reproduction is permitted which does not comply with these terms.
*Correspondence: María Rebolleda-Gómez, bWFyaWEucmVib2xsZWRhLWdvbWV6JiN4MDAwNDA7eWFsZS5lZHU=; Corlett Wolfe Wood, Y29ybGV0dC53b29kJiN4MDAwNDA7cGl0dC5lZHU=
†These authors have contributed equally to this work