- 1Instituto de Biodiversidade e Sustentabilidade (NUPEM), Universidade Federal do Rio de Janeiro, Campus Macaé, Rio de Janeiro, Brazil
- 2Institut de Génomique Fonctionelle de Lyon - CNRS UMR 5242, Lyon, France
- 3Laboratório de Biologia Molecular do Desenvolvimento, Instituto de Ciências Biomédicas, Universidade Federal do Rio de Janeiro, Cidade Universitária Ilha do Fundão, Rio de Janeiro, Brazil
- 4Instituto Nacional de Ciência e Tecnologia em Entomologia Molecular - INCT-EM. INCT-EM, Rio de Janeiro, Brazil
- 5Laboratório de Química e Função de Proteínas e Peptídeos, Centro de Biociências e Biotecnologia, Universidade Estadual do Norte Fluminense Darcy Ribeiro, Rio de Janeiro, Brazil
Genes encoding small open-reading frames (smORFs) have been characterized as essential players of developmental processes. The smORF Tarsal-less/mille-pattes/polished-rice has been thoroughly investigated in holometabolous insects, such as the fruit fly Drosophila melanogaster and the red flour beetle Tribolium castaneum, while its function in hemimetabolous insects was only recently investigated. Thus, we analyzed the function of the tal/pri/mlpt ortholog in a hemimetabolous insect, the kissing bug Rhodnius prolixus (Rp). Sequence analysis shows that Rp-mlpt polycistronic mRNA encodes two small peptides (11–14 amino acids) containing a LDPTG(L/Q/T)Y motif. Interestingly, a new hemipteran-specific conserved peptide of ~80 amino acids was also identified by in silico analysis. In silico docking analysis supports the binding of the small Mlpt peptides to the transcription factor Shavenbaby. Rp-mlpt in situ hybridization and knockdown via RNA interference showed a conserved role of Rp-mlpt during embryogenesis, with a major role in the regulation of thoracic vs. abdominal segmentation, leg development, and head formation. Altogether, our study shows that mlpt segmentation role is conserved in the common ancestor of Paraneoptera and suggests that polycistronic genes might generate order specific smORFs.
Introduction
A large number of essential genes required for biological processes have been discovered by genetic screenings in model organisms such as the fruit fly Drosophila melanogaster (e.g., Nüsslein-Volhard and Wieschaus, 1980). While most loci important for developmental processes were identified in these original screenings, recent genetic and expression analyses in D. melanogaster and in the beetle Tribolium castaneum showed that genes previously classified as putative non-coding RNAs encode functional small open reading frames (smORFs) or sORFs (Pueyo et al., 2016a; Chekulaeva and Rajewsky, 2018). smORFs, ORFs smaller than 100 amino acids, have been described as being fundamental for several developmental processes of insects (Savard et al., 2006; Galindo et al., 2007; Kondo et al., 2007), although gene prediction methods have, in general, discarded smORFs in genome-wide predictions (reviewed in Saghatelian and Couso, 2015). Comparative genomic analysis of Drosophilid species showed an unexpected conservation of smORF-containing genes, suggesting important biological roles for this new class of genes (Ladoukakis et al., 2011).
Later on, experimental analysis of conserved smORFs such as sarcolamban (scl), a conserved peptide involved in Ca2+ uptake at the sarco-endoplasmic reticulum (Magny et al., 2013), and hemotin (Pueyo et al., 2016b), a conserved phagocytosis regulator, provided further evidence that genes containing smORFs might constitute a reservoir of important players in metazoan genomes. In the past years, direct evidence of large-scale smORF translation has been obtained by ribosomal profiling of polysomal fractions in Drosophila, using the Poly Ribo-Seq technique (Aspden et al., 2014). This study was able to classify smORFs in two groups: “longer” smORFs of around 80 amino acids resembling canonical proteins, mostly containing transmembrane motifs, and shorter (“dwarf”) smORFs. These “dwarf” smORFs are generally shorter (around 20 amino-acid long), less conserved and mostly found in 5′-UTRs and non-coding RNAs.
While bioinformatic studies point to hundreds or thousands of genes containing putative smORFs, only a few functional studies have been performed. In insects, the function of the smORF founding member mille-pattes (mlpt) was only investigated in detail in holometabolous insects, such as the D. melanogaster and T. castaneum (Savard et al., 2006; Galindo et al., 2007; Kondo et al., 2007), and more recently in basally branching Diptera (Jiménez-Guri et al., 2018) and Lepidoptera (Cao et al., 2018). In T. castaneum, mlpt acts as a gap gene during the process of embryonic segmentation, regulating Hox genes and thoracic vs. abdominal specification; knockdown of mlpt leads to embryos with multiple legs, mille-pattes in French (Savard et al., 2006). In D. melanogaster, tarsal-less (tal) was identified in a spontaneous mutant with defects in the distal part of the legs, the tarsus (Galindo et al., 2007). Independently, in the same year, Polished Rice (Pri) peptides were shown to be required for the F-actin organization during trichome morphogenesis; embryos lacking pri show external cuticle defects, resembling polished rice (Kondo et al., 2007). More recently, Mlpt peptides were shown to be regulated by ecdysone, defining the onset of epidermal trichome development, through post-translational control of the Shavenbaby (Svb) transcription factor (Chanut-Delalande et al., 2014). Altogether, Mlpt peptides display context-specific roles and interactions within different developmental processes.
While in D. melanogaster, mlpt early embryonic gene expression is segmental displaying a pair-rule pattern, mutants do not display segmentation or homeotic alterations as reported in T. castaneum. D. melanogaster embryonic mutant phenotypes include broken trachea, loss of cephalopharyngeal skeleton, abnormal posterior spiracles and lack of denticle belts, structures of late embryonic mlpt expression (Galindo et al., 2007).
To clarify whether the segmentation function of mlpt is ancestral but has been lost in the lineage giving rise to D. melanogaster, or whether it is a recently arisen specialization of T. castaneum, it is important to study mlpt function in other insect groups. Since hemipterans, as hemimetabolous, comprise the sister group of holometabolous insects (reviewed in Panfilio and Angelini, 2018), a functional characterization of mlpt in the emergent hemiptera model, the kissing bug Rhodnius prolixus would be important (Nunes-da-Fonseca et al., 2017). R. prolixus available genomic and transcriptomic resources (Medeiros et al., 2011; Ribeiro et al., 2014; Mesquita et al., 2015; Marchant et al., 2016; Brito et al., 2018), an established embryonic staging system (Berni et al., 2014) and the recent availability of in situ hybridization and RNA interference techniques are great advantages of this model system (Nunes-da-Fonseca et al., 2017). Recently, mlpt function has been investigated in several insects, including two hemipteran species, the water strider Gerris buenoi (Hemiptera, Gerridae) and the milkweed bug Oncopeltus fasciatus (Hemiptera, Lygaeidae) (Ray et al., 2019). This analysis provided evidence that Mlpt peptides, the ubiquitin-ligase Ubr3 and the transcription factor Shavenbaby (Svb), constitute an ancient developmental module required for early insect embryo patterning.
Here, we report interesting conserved and new functional aspects of the prototypic smORF mlpt gene of R. prolixus. First, sequence analysis identified a new hemiptera-specific peptide in the polycistronic mRNA of mlpt, a gene conserved throughout the Pancrustacean clade. Second, molecular docking analysis indicates that the small peptide containing a LDPTG(L/Q/T)Y consensus motif interacts with the N-terminus of the transcription factor Svb, as in D. melanogaster. Third, expression and functional analysis shows that the ortholog of mlpt acts during embryonic segmentation, regulating the transition between thoracic and abdominal identity, similarly to its role previously described in T. castaneum. Fourth, a conserved role in tarsal patterning was also observed. Overall, segmentation and tarsal patterning are conserved and ancestral roles of mlpt and our data provide evidence that generation of new peptides from smORFs might constitute an underestimated mechanism for the evolution of new genes.
Results
The recent description of several biologically important genes encoding smORFs among metazoans opened new avenues for molecular biology and functional genomics research (Saghatelian and Couso, 2015; Pueyo et al., 2016a; Zanet et al., 2016). mlpt function has been largely investigated in holometabolous insects, while its function in hemimetabolous insects has only been recently reported (Ray et al., 2019). In the current manuscript, we describe a bioinformatic and functional analysis of mlpt function in the kissing bug R. prolixus.
mlpt Polycistronic Gene and Peptide Distribution Among Arthropods
Previous BLAST searches for genes encoding mlpt peptides against different arthropod genomes and transcriptomes provided evidence that this gene containing smORFs is restricted to insects and crustaceans (Galindo et al., 2007). The increase in genome sequences in the past years (Tribolium Genome Sequencing Consortium et al., 2008; Schwager et al., 2017; Panfilio et al., 2019) allowed us to perform a complete search in the available arthropod genomes. Using a non-stringent BLAST approach (see methods for details), we identified mlpt orthologs in available insect genomes (Supplementary Table 1). These orthologs encode between two and several copies of a peptide of 11–32 amino acids, containing a LDPTG(L/Q/T)Y consensus motif (Figure 1A–red boxes, Figure 1B). These peptides have been previously shown to mediate the switch of the svb transcription factor from a repressor to an activator (Kondo et al., 2010) via the ubiquitin-conjugating complex, UbcD6-Ubr3, and proteasome recruitment (Zanet et al., 2015). Remarkably, hemiptera mlpt orthologs not only contain two smORFs encoding the LDPTG(L/Q/T)Y consensus motif (Figure 1A–red boxes), but also contain a larger hemiptera-specific smORF of about 80 amino acids (Figure 1A–green boxes and Figure 1C). We named this new peptide smHemiptera due to its restricted phylogenetic distribution. Interestingly, a polycistronic transcript containing all the aforementioned smORFs was identified in a digestive tract R. prolixus transcriptome (Ribeiro et al., 2014 and our own observations). This peptide appears to be smaller and less conserved in basally branching hemipteran species with circa 60 amino acids in the genome of the pea aphid Acyrthosiphon pisum and Pseudococcus longispinus and larger than 80 amino acids in triatomes, such as Rhodnius prolixus and Triatoma pallidipennis. smHemiptera contains a conserved stretch of 14 amino acids (GHR(S/N)WMTHLPLSRP) in almost all derived hemipteran species with available transcriptomes (Figures 1A,C). The unique exception is the genome of the milkweed bug, O. fasciatus (Panfilio et al., 2019), which lacks a mlpt gene sequence in the current assembly. Of-mlpt transcript recently published by Ray et al. (2019) does not contain smHemiptera. Thus, either O. fasciatus mlpt gene lost smHemiptera or the published transcript is not complete. We favor the latter possibility since smHemiptera is also present in mlpt genes of all species analyzed, including the recently published mlpt sequence of water strider, Gerris buenoi (Hemiptera, Gerridae) (Ray et al., 2019). Lastly, another peptide of 14 amino acids was identified at the 3′ region of two triatomine species (Figure 1A- pink boxes and Figure 1D).
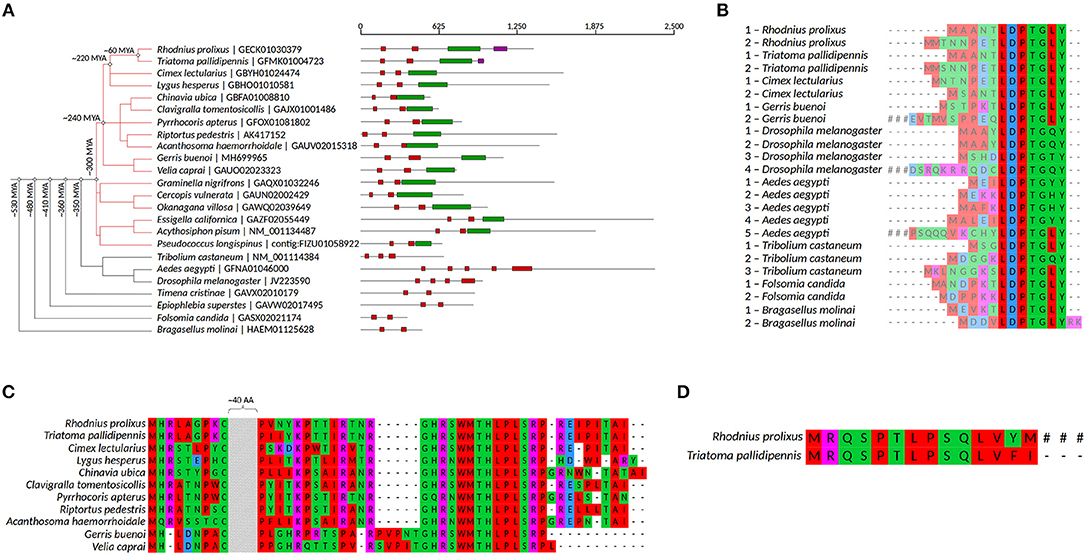
Figure 1. mlpt evolutionary analysis and putative regulation. (A) Phylogenetic representation of mlpt distribution among arthropod species and approximate time of node speciation based on the online server TimeTree (Kumar et al., 2017). mlpt polycistronic mRNA organization. Black lines comprise the whole polycistronic mRNA containing the smORFs encoding peptides displayed in red, green, and pink boxes. (B) Alignment of the previously described small peptide (red boxes in A) containing an LDPTG domain (Savard et al., 2006; Galindo et al., 2007; Kondo et al., 2007), conserved in several arthropod species and whose number of paralogs in a single polycistronic gene varies among arthropod species. (C) Alignment of a hemipteran specific peptide of about 80 amino acids (green boxes in A). (D) Alignment of triatomine conserved smORF encoded peptides (pink boxes). #indicates that translation is not interrupted in this sequence.
Shavenbaby-mlpts Binding Affinity, Interaction Modes, and Hotspots Predictions
Previous work in D. melanogaster identified the 31 N-terminal residues of the Shavenbaby (Svb) transcription factor (TF) as a Mlpt-dependent degradation signal, or degron (Zanet et al., 2015). In order to investigate the interaction modes between the peptides encoded by the polycistronic mRNA of mlpt and the Svb TF from D. melanogaster (Dmel-Svb) and R. prolixus (Rprol-Svb), 3D structures were predicted by an ab initio approach and protein-peptide docking assays were performed. First, exhaustive analyses about the secondary structure profile and disordered regions on Dmel-Svb and Rprol-Svb amino acid sequences were performed. Our results suggest that both Dmel-Svb and Rprol-Svb display mostly disordered and coiled regions (Supplementary Figures 1, 2), as previously suggested (Zanet et al., 2015). The disordered and coiled regions appear in large domains of both proteins, being interspersed by small alpha-helices and short beta-sheets (Figures 2A–R; Supplementary Figures 2A,B). According to DISOPRED3, around to C-terminal in both Svbs, the disorder probability decreased, indicating that the Zinc-finger double domain located in this region is more ordered. Smaller peptides containing LDPTG(L/Q/T)Y motifs, Dmel-pptd1 (Supplementary Figure 2C), Rprol-Mlpt1 (Supplementary Figure 2E) and Rprol-Mlpt2 (Supplementary Figure 2F) assumed disordered conformations. In contrast, Dmel-Mlpt4 (Supplementary Figure 2D), a peptide with 32 aa and unknown function (Galindo et al., 2007; Kondo et al., 2007), presented an ordered structure with two alpha-helices, intercalated by two small disordered regions. Moreover, DISOPRED3 program also predicted the protein-binding sites on the Dmel-Svb N-terminal ranging from Leu19 to Asn23 and in Rprol-Svb from Ser24 to Leu42 (Supplementary Figures 1A,B). Interestingly, our findings about Dmel-Svb-Mlpt interactions corroborates previous findings of Zanet et al. (2015), which showed that the region between the first 11 (N11) and 31 (N31) amino acids are required for Mlpt—mediated processing. Therefore, we further conducted docking assays with Svb and Mlpts, taking into account the data prediction and previously published biological data (Zanet et al., 2015).
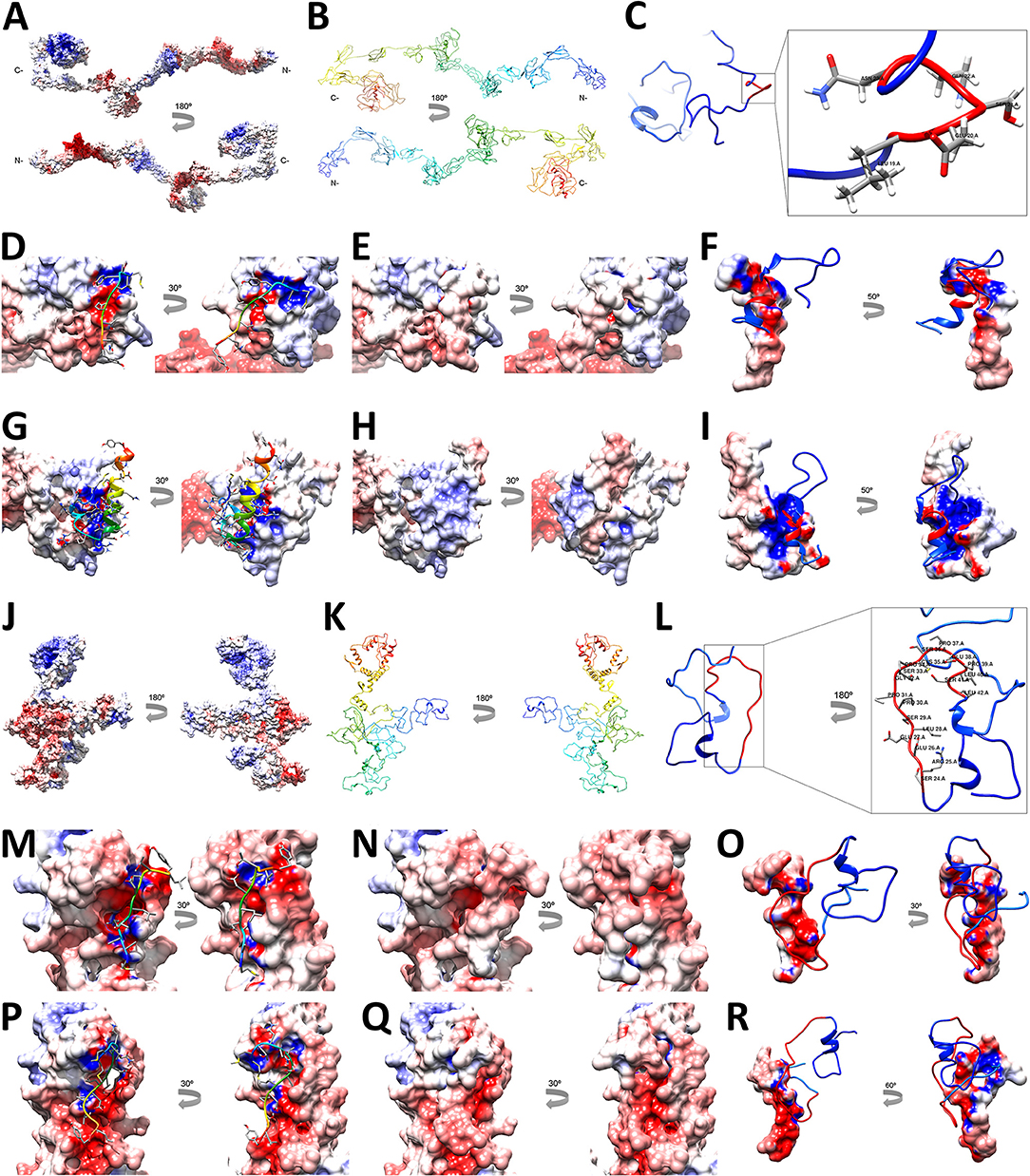
Figure 2. Shavenbaby-Mlpts interaction prediction. The Svbs-Mlpts docking assays were based on (Zanet et al., 2015) findings and on the analysis of protein binding sites prediction in the Svbs N-termini by DISOPRED. The electrostatic potential was represented on the molecular surface of Dmel-Svb (A,D,E,G,H), Dmel-Mlpt1 (E,F), Dmel-Mlpt4 (H,I), Rprol-Svb (D,J,M,N,P,Q), Rprol-Mlpt1 (N,O), Rprol-Mlpt2 (Q,R), with negative values (−10 keV) in red, neutral in white, and positive values (+10 keV) in blue. Dmel-Svb (B) and Rprol-Svb (K) ribbons (figure downside) were colored from blue to red to indicate the progression of the N- to C-terminal, respectively. The N-terminal (C,L) was enlarged and the predicted protein-binding site region Leu19-Asn23 was depicted in red for Dmel-Svb (C) and the region Ser24-Leu42 for Rprol-Svb (L). Dmel-Mlpt1 (D), Dmel-Mlpt4 (G), Rprol-Mlpt1 (M) and Rprol-Mlpt2 (P) are represented as ribbon, colored from blue to red as the polypeptide chain progresses from the N- to the C-terminus, each one in your respective docking region on Shavenbaby surface. The Mlpts side-chains residues are shown as sticks.
Docking assays focused in the predicted protein-binding sites on the Svb N-terminal. Our results suggest that at least half of LDPTG(L/Q/T)Y motifs residues from Mlpts peptides are involved with complex formation (Supplementary Table 2). The possible interactions at the complex interfaces (Supplementary Figure 3), the binding free energy contribution per-residue (Supplementary Table 2) as well as their theoretical interaction affinities (Supplementary Table 2), were predicted. The binding affinity measured by HawkDock program, using the MM/GBSA method, showed that Dmel-Svb_Dmel-Mlpt4 has the highest affinity, followed by Rprol-Svb_Rprol-Mlpt1, after by Rprol-Svb_Rprol-Mlpt2 and Dmel-Svb_Dmel-Mlpt1 (Supplementary Table 3). The dissociation constant (Kd) was measured by PRODIGY program and suggests a low affinity between them (Supplementary Table 3). The amount of bonds and contacts were measured by PDBsum (Supplementary Figure 3; Supplementary Table 3), and the results suggest that the interaction model Rprol-Svb_Rprol-Mlpt1 has the greatest number of non-bonded contacts, followed by Rprol-Svb_Rprol-Mlpt2. The Rprol-Svb_Rprol-Mlpt1 and Dmel-Svb_Dmel-Mlpt4 established the greatest number of hydrogens bonds but Dmel-Svb_Dmel-Mlpt4 has the greatest number of salt-bridges. Further analysis by in silico site-directed mutagenesis validated the contribution of each amino acid for peptide-TF binding (Supplementary Figure 4). Importantly, the predictions of binding affinity changes upon mutations indicate that globally at least 30% of residues at the interface are critical for protein complex stabilization. Analysis of binding affinity changes upon mutations corroborate previous biological findings of Zanet et al. (2015), since single mutations of Leucines (L24, L25, and L19) and Glutamic Acid (E20) at the N-terminal region of Dmel-Svb affect this region's interaction with Dmel-Mlpt1 (Supplementary Figure 4). These amino acids lie on the region previously experimentally demonstrated to be required for Dmel-Svb processing, mediated by Dmel-Mlpt1 (Zanet et al., 2015). To summarize, the proposed model was able to recover previously data of Dmel-Svb_Dmel-Mlpt biological interaction (Zanet et al., 2015) and to suggest the putative regions of interaction between Rprol-Svb and Rprol-Mlpts.
Rp-mlpt Spatial Expression Pattern and Relative Expression During Rhodnius prolixus Embryogenesis
mlpt embryonic expression was originally described in the beetle T. castaneum and has been characterized by a very dynamic patterning (Savard et al., 2006). In D. melanogaster, mlpt expression was correlated with tissue folding, acting as a connection between patterning and morphogenesis (Galindo et al., 2007). Early expression of D. melanogaster mlpt starts as seven blastodermal stripes and a cluster of cells in the anterior part of the embryo, although segmentation is not affected in mlpt mutants (Galindo et al., 2007). Later, after segmentation, mlpt is present in the trachea, posterior spiracles, pharynx, hindgut, and presumptive denticle belts. D. melanogaster mlpt mutants display reduced cuticular structures and ectopic expression of mlpt in the head induces extra skeleton components (Galindo et al., 2007). In hemipterans, O. fasciatus and G. buenoi mlpt is expressed in an anterior domain at early stages, during germ band elongation through recently added segments anterior to the growth zone, and later expression is observed in presumptive neurons in the central nervous system, as well as in the limb buds and mouth parts (Ray et al., 2019).
To investigate mlpt expression in R. prolixus during embryogenesis, in situ hybridization of several embryonic stages was performed. The original data of Rp-mlpt expression using a colorimetric substrate and DAPI stainings are provided in Supplementary Figure 5, while, for simplicity, overlay images of pseudo-fluorescence expression and DAPI stainings are provided in Figure 3. Rp-mlpt distinct expression can be observed at stage 1B, between 6 and 12 h after egg laying (AEL), in an anterior domain (Figure 3A), which corresponds to the presumptive head lobes, followed by the appearance of a posterior expression domain shortly before the posterior invagination at stage 2A (Figure 3B 12–18 h AEL). Hemipterans, such as R. prolixus, undergo an embryonic inversion movement called anatrepsis (Panfilio, 2008). At stage 3A (24–30 h AEL), during this inversion movement (Figures 3C,C′), and at the beginning of germ band elongation at stage 3B (Figures 3D,D′ 30–36 h AEL), Rp-mlpt is expressed on the newly formed thoracic and abdominal segments, but not at the posterior-most region, the segment addition zone (SAZ) or growth-zone (Auman et al., 2017; Paese et al., 2018). At stage 4, during gem band elongation, Rp-mlpt expression remains in front of the SAZ and in an anterior expression domain of the head (Figures 3E–J 36–48 h AEL). At stage 5 (48–60 h AEL), shortly before the end of segmentation and when segmental buds are about to appear, Rp-mlpt is expressed only at the posterior-most region (Figure 3K 48–60 h AEL). Between stage 5 and stage 6 (60–72 h), abdominal segmentation is completed and Rp-mlpt expression is observed at the tips of the legs and in the head lobes (Figure 3L). Between stage 7 (72–84 h AEL) and stage 8 (84–96 h AEL), which is characterized by leg proximal-distal axis growth and abdominal segments thickening, an expression at the lateral, future dorsal embryonic region can be visualized (Figure 3M). At stage 9 (96–108 h AEL), at the beginning of germ band retraction, Rp-mlpt is only observed at the antenna, an organ undergoing extensive increase in size during stage 9 and 10 (Figure 3N; Berni et al., 2014). Altogether, mlpt expression is highly dynamic, suggesting multiple roles during R. prolixus embryonic development.
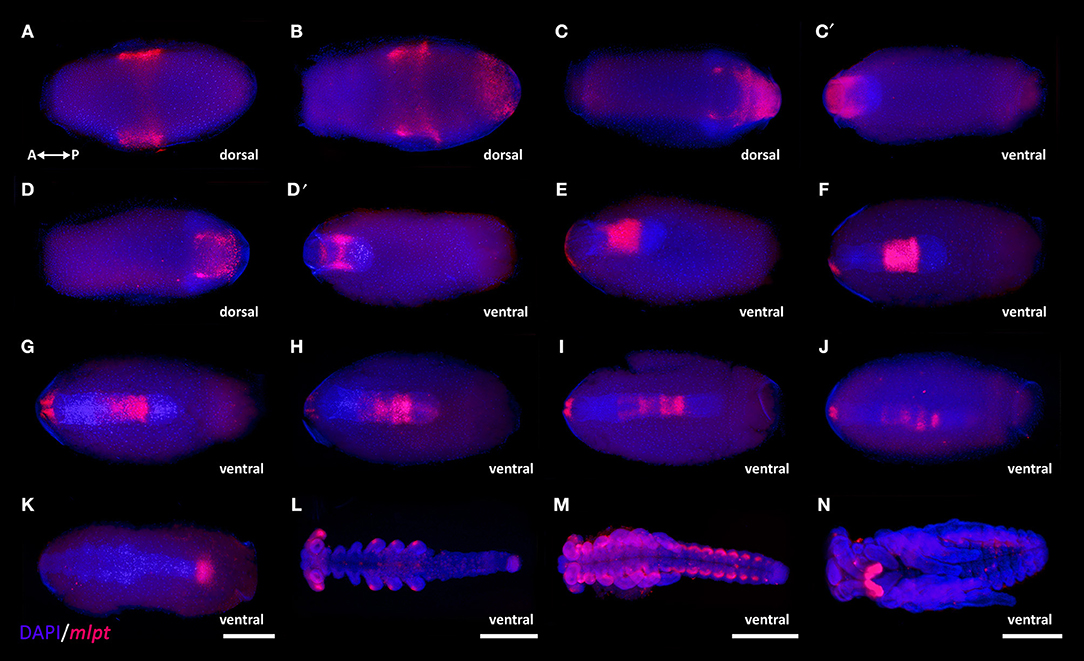
Figure 3. mlpt expression during R. prolixus embryogenesis. Anterior of the egg to the left in all panels. During the processes of anatrepis and katatrepsis embryo orientation shifts along the AP and DV axis (see Nunes-da-Fonseca et al., 2017 and Berni et al., 2014 for details). mlpt expression is displayed in false-colored red and DAPI in blue. Ventral egg view (A,B,C′,D′,G,H,I). Eggs dissected from the yolk (L,M,N) (A) Stage 1B, ~10 h after egg laying (AEL) mlpt expression is detected as a broad middle-anterior domain, which overlaps with to the future head. (B) A few hours later (stage 2A- 12–18 h AEL) besides the anterior domain, a posterior domain is also observed. (C,C′,D,D′) At stage 3A (24–30 h AEL) during anatrepsis, the posterior expression domain is at the surface (ectoderm) but does not extend toward the posterior region of the germ band. (E–J) At stage 3B (30–36 h AEL) the expression remains anterior to the posterior region and in a small bilateral domain in the head and starts to fade as elongation is finished. (K) Stage 4 (36–48 h) at beginning of head and thoracic segmentation, expression is observed at the posterior tip. (L) Stage 5 (48–60 h AEL) during leg growth a staining is specifically observed at the tips of the legs and in the head lobes. (M) At stage 7 (72–84 h AEL) abdominal segmentation is evident and expression is observed at lateral segmental cell clusters potentially corresponding to peripheral neurons as previously suggested for T. castaneum (Savard et al., 2006). (N) Stage 9 (96–108 h AEL) during germ band retraction expression is restricted to the antennae. R. prolixus embryonic staging system was described in Berni et al. (2014).
To investigate if levels of expression of mlpt also vary along embryonic development, we investigated its expression via Real-Time PCR (RT-qPCR). Small changes of Rp- mlpt expression were observed between non-fertilized (0–6 h NF) and early fertilized eggs (0–6 h AEL), while a 10-fold increase in expression was detected by the analysis of 36–48 h AEL eggs (Supplementary Figure 6A). Altogether, these RT-qPCR data show that Rp-mlpt expression is higher during zygotic segmentation stages than maternally deposited mRNAs.
Rp-mlpt Parental RNA Interference Is Efficient and Leads to a Series of Knockdown Phenotypes
Since Rp-mlpt gene displays a complex expression patterning during embryogenesis, we sought to analyze its function by parental RNA interference (pRNAi) in R. prolixus, as previously described (Berni et al., 2014). In this method, females are injected with double-stranded (dsRNA) synthesized against the gene of interest and phenotypic effects are evaluated in the offspring. Rp-mlpt dsRNA eggs showed a decrease up to 60–70% in Rp-mlpt expression when compared to its expression in the control dsRNA group, validating the knockdown (Supplementary Figure 6B). An increase in Rp-mlpt dsRNA injection concentration up to six micrograms per microliter (6 μg/μl) did not further increase knockdown efficiency (data not shown).
Upon egg collection and fixation, nuclear DAPI staining was performed and a plethora of embryonic mutant phenotypes were observed. A first phenotypic class of Rp-mlpt RNAi embryos comprises embryos with a wild-type number of segments, but with thoracic or gnathal segmental fusion. Embryos with this morphology were evident at stage 5 and 7 (Figures 4B,B′,C,C′). A second phenotypic class was constituted by embryos with four pairs of legs in the Rp-mlpt RNAi instead of the three pairs observed in controls (Figures 4E,E′). Last, a third phenotypic class of Rp-mlpt RNAi embryos showed improper germ band elongation and lack of the posterior region at stage 3 (Figures 4A,A′), which is more evident at stage 9, when a lack of abdominal segmentation and fused legs were observed (Figures 4D,D′).
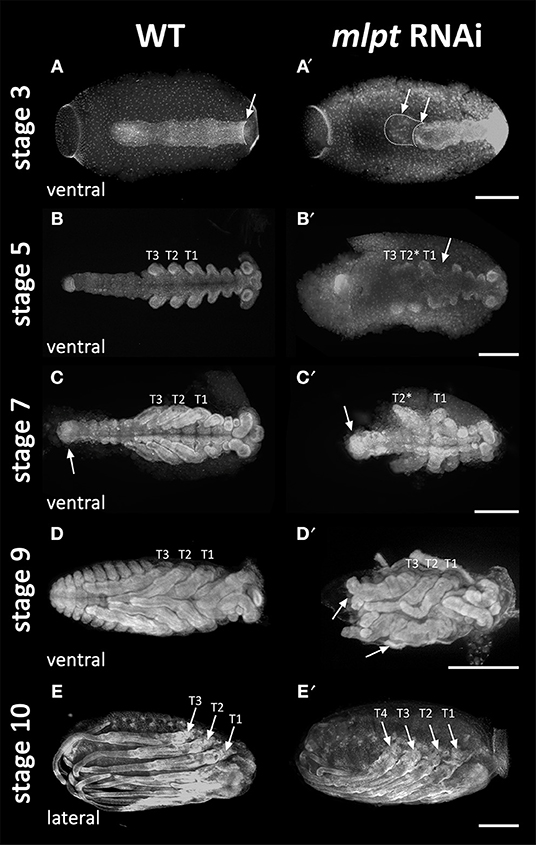
Figure 4. mlpt knockdown segmentation defects. DAPI staining of control and mlpt RNAi embryos. Anterior region of the egg toward to the left in all panels. (A,A′) Stage 3A. Germ band elongation appears irregular in the RNAi embryo when compared to the controls. (B,B′) Stage 5. Irregular thoracic and gnathal segmentation in the RNAi embryo. (C,C′) Stage 7. Thoracic segmental fusion and improper abdominal in the RNAi embryo segmentation when compared to the control. (D,D′) Stage 9. Third thoracic segment fused lack of abdominal segmentation and dorsal closure when compared to the control (E,E′) Stage 10. Late mlpt RNAi embryo showing four pairs of legs when compared to the three pairs of legs of the control. R. prolixus embryonic staging system was described in Berni et al. (2014).
These phenotypic classes can also be observed by dissection of embryos at very late stages when nuclear DAPI staining is not possible due to cuticle formation (Figures 5A–D). Thoracic red pigmentation can be used to compare wild-type and RNAi embryos. In the first Rp-mlpt RNAi phenotypic class (20 out of 45 embryos−45%), embryos with two pairs of thoracic segments can be identified, indicating improper thoracic segmentation (Figures 5B-B‴). In the second class (15 out of 45 embryos−33%), four thoracic segments are present (Figures 5C-C‴), while in the third class (10 out of 45 embryos−22%), the strongest knockdown phenotype, the late embryo displays impairment of germ band elongation and most abdominal segmentation is lost, while dorsal closure shows clear defects (Figures 5D-D‴). We noticed that injection of higher concentrations of Rp-mlpt dsRNA increased the frequency of the third phenotypic class and the observation of head defects that were not identified in any of the previously described classes (see below).
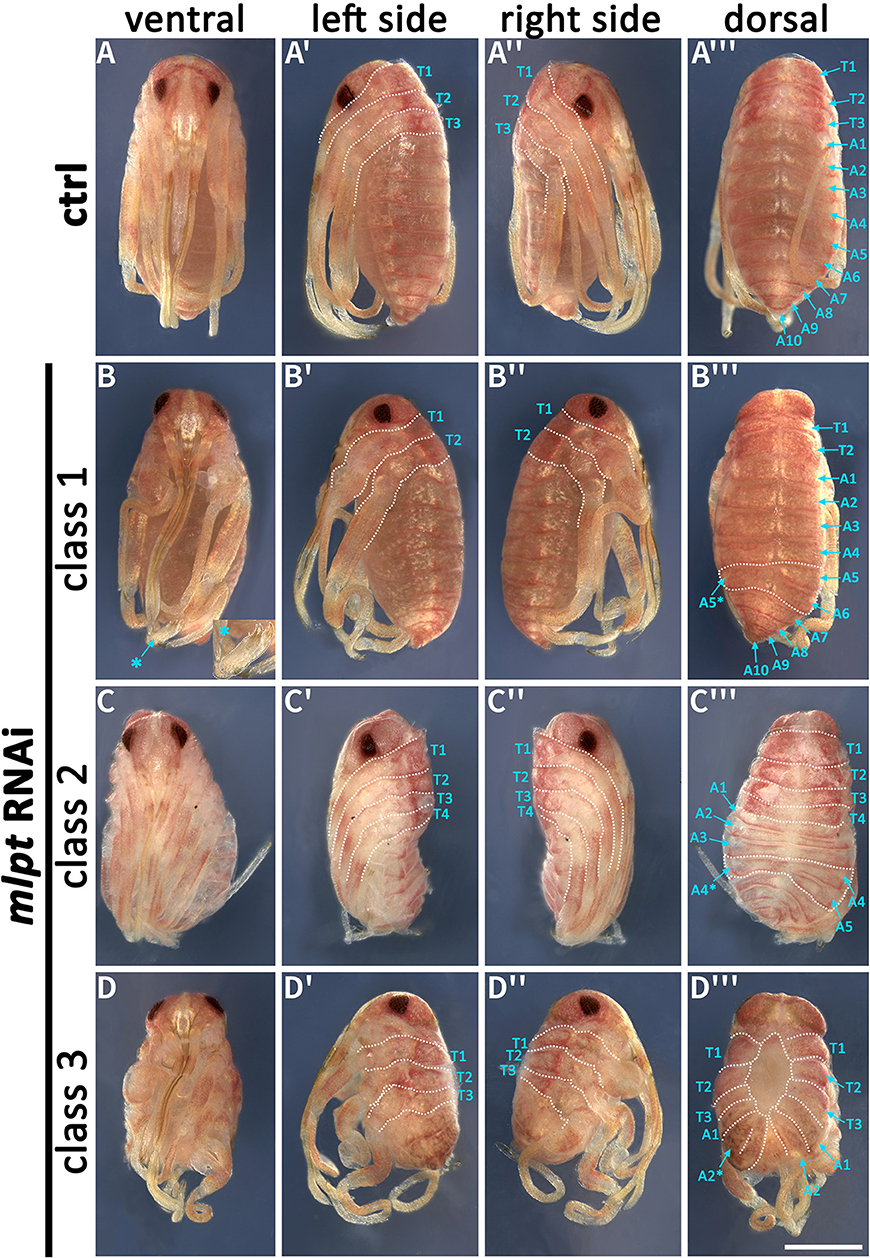
Figure 5. Phenotypic classes of mlpt RNAi late embryos. mlpt knockdown embryos were classified in three phenotypical classes when compared to the control (A-A‴). (B–B‴) Class 1—Embryos displaying only two thoracic segments and distal leg duplications (asterisk) (B–D). Abdominal segmentation appears normal with the exception of some asymmetrical segmental divisions on left and right sides (asterisk) (20 out of 45 embryos). (C–C‴) Class 2—Embryos displaying four thoracic segments and irregular abdominal segmentation (15 out of 45 embryos). (D–D‴) Class 3—Shorter embryos only displaying thoracic segments and fewer abdominal segments then controls (10 out of 45 embryos).
Interestingly, injection of Rp-mlpt dsRNA at lower concentrations (up to 1 μg/μL) into R. prolixus females lead to a small percentage of nymphs, which are able to leave the eggshell (10–15%). A few of these Rp-mlpt dsRNA nymphs display unilateral segmental fusion of first and second thoracic segments leading to abnormal distal leg morphology (Figures 6A,B). Distal regions of legs in wild-type R. prolixus are composed of a stereotypic pattern of tibia, tarsus and a pair of claws (Figure 6A). dsRNA Rp-mlpt nymphs display improper cuticle darkening and different types of leg deviations from the wild-type patterning, including lack of the proper distinction between tarsus and claws (Figures 6B′,B″). Altogether, our results show that Rp-mlpt is important for the distinction between thoracic and abdominal segments, for the process of germ band elongation and distal leg patterning.
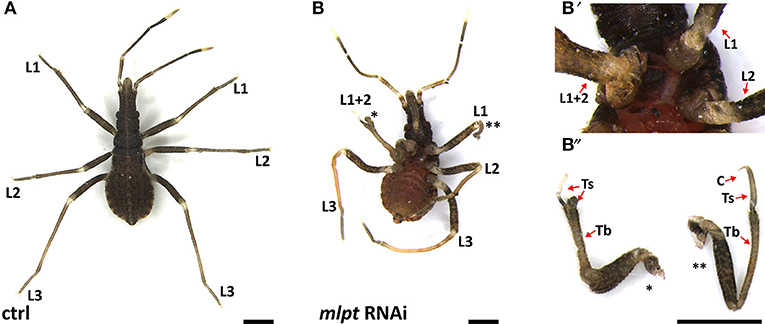
Figure 6. Segmental fusion and leg defects after mlpt RNAi. (A,B) Control (A) and mlpt RNAi nymph (B,B′,B″). (B') mlpt RNAi nymph showing unilateral leg fusion of L1 and L2. (B′,B″) Higher magnification of distal regions of fused legs show morphologies that deviate from the wild-type pattern. Several phenotypic changes from the wild-type pattern are observed after mlpt RNAi such as lack of distal regions such as tarsus (Ts) and claws as highlighted in (B″). Tb, tibia; Ts, tarsus; C, claws. Scale bar 500 μM.
Rp-mlpt dsRNA Injection Leads to Segmental Fusion and Impairment of Head Formation in the Strongest Phenotypes
Reduction in Rp-mlpt expression affected segment formation with clear phenotypic effects in the thorax and abdomen, before posterior invagination and at later segmentation stages (Figures 4, 5). Since a reduction or increase in the number of thoracic segments was observed in late embryonic stages after Rp-mlpt RNAi, we evaluated the expression of the ortholog of the segment polarity gene hedgehog (hh) in control and Rp-mlpt RNAi embryos. Like in other insects, the posterior region of every segment expresses Rp-hh during embryonic segmentation, and thoracic and abdominal segments can be identified by their differential widths (Figure 7A). A phenotypic series of Rp-mlpt RNAi embryos shows that the distance between segmental stripes is reduced and that the distinction between thoracic and abdominal segments is less clear. Remarkably, in apparently stronger knockdown phenotypes, a lack of the anterior-most segments of the head was also observed (Figure 7A, asterisk). hh stainings of these strongest mlpt phenotypes at stage 9 confirms that the head is affected at later stages (Figure 7A′). Phenotypic defects in the head have only been observed upon injection of higher dsRNA concentrations (four to 6 μg per microliter) and in a low frequency (~10% of the embryos). This strongest phenotype cannot be attributed to any of the previously described three classes. Altogether, Rp-hh expression analysis shows that Rp-mlpt is essential for proper thoracic and abdominal segmental distinction and for head formation.
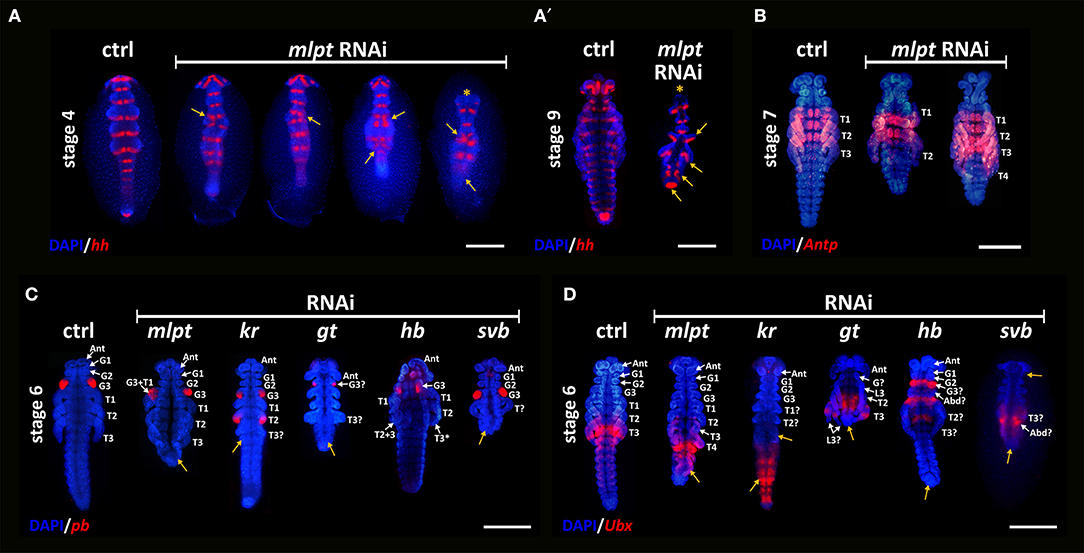
Figure 7. Segmental fusion and Hox gene expression in knockdown embryos of mlpt and other developmental genes. (A) In situ hybridization for hedgehog (hh) and nuclear counterstaining with DAPI of control (ctrl) in the left and progressive stronger mlpt dsRNA phenotypes from left to right. Yellow arrows highlight apparent segmental fusions at the thoracic regions. In the strongest phenotype (right most) the head is affected (asterisk) and germ band elongation did not occur. (A′) At stage 9 one control (ctrl) one strongly affected mlpt dsRNA embryo, showing segmentation and head defects. (B) ANTP antibody staining in control and Rp-mlpt RNAi embryos. ANTP antibody marks the first and the second legs (L1 and L2) and the three thoracic segments in control embryos. Middle row—Rp-mlpt embryos show two fused segments (L1 and L2) and a third leg (L3), which does not express ANTP. Rightmost embryo shows four legs with a probable duplication of L3, since the two most posterior legs do not express ANTP. (C) pb expression in control and RNAi embryos. In control embryos at stage 6, pb is expressed in the third gnathal segment, corresponding to the prospective labium. After mlpt RNAi pb is either non-affected (right side) or an irregular shape (left side). Duplications or lack of staining were not observed after mlpt RNAi. Kr RNAi embryos show duplication patterns, gt RNAi embryos show a smaller group of expressing cells and the lack of anterior gnathal segments. svb RNAi displays clear segmentation deviations from the wild-type pattern, but does not display changes in pb staining. (D) Ubx expression in control and RNAi embryos. Ubx expression is stronger at the first abdominal segment and in concentric rings of the third leg. After mlpt RNAi Ubx is still strongly expressed in the first abdominal segment and not in concentric rings of the third leg. Kr RNAi embryos show diffuse staining in the abdominal region. Strong phenotype of gt RNAi embryos lack abdominal Ubx expression, while this marker is expressed in two consecutive pairs of legs, suggesting duplication of the third leg (L3). Hb RNAi embryos display Ubx expression at anterior (gnathal) segments. No Ubx staining was observed in the legs. A strong svb RNAi embryo lacks the posterior abdominal but show expression of Ubx.
Comparison of Knockdown Phenotypes of mlpt and Other Developmental Genes
Previous functional analysis of mlpt in the T. castaneum and D. melanogaster showed that this gene is required for early patterning in the beetle but not in fruit flies. In beetles, mlpt acts as a gap gene, while its role in fruit flies is restricted to later (post-segmental) embryonic stages (Savard et al., 2006; Galindo et al., 2007; Kondo et al., 2007, 2010; Ray et al., 2019). Recent analysis of mlpt function in two other hemipteran species and in a wasp showed a requirement for early patterning processes, suggesting that this is the ancestral function of the gene (Ray et al., 2019). From our phenotypic analysis, it remains open whether the knockdown of Rp-mlpt leads to similar morphological changes as the knockdown of classical gap genes, the transcription factors Krüppel (Kr), giant (gt), and hunchback (Hb). We performed parental RNAi against the orthologs of these three gap genes and analyzed the expression of Hox genes. Krüppel (Kr) and giant (gt) RNAi phenotypes have been published elsewhere (Lavore et al., 2012, 2014). Expression of the Hox gene proboscipedia (pb) in the third gnathal segment is comparable in control and mlpt RNAi embryos, although the posterior region in the knockdown has been clearly affected (Figure 7C). In contrast, pb expression is duplicated after Rp-kr RNAi, largely diminished after Rp-gt RNAi and slightly affected upon Rp-Hb RNAi (Figure 7C).
The expression of the Hox gene Ultrabithorax (Ubx) was also evaluated in control and in knockdowns of mlpt and other gap genes. In R. prolixus control embryos, Ubx is strongly expressed in the first abdominal segment and in concentric rings around the third leg (L3) (Figure 7C). As previously described, the effect of mlpt RNAi can generate at least three classes of embryonic phenotypes. Analysis of an embryo showing four pairs of legs (class 2 phenotype) showed that only the posterior-most leg expresses Ubx, suggesting that this leg corresponds to the L3 and that a duplication of L1 or L2 must have occurred. Interestingly, the expression of Ubx in the L3 does not occur as a concentric ring as in the control, but rather in one side of the legs, suggesting that leg patterning was also affected (Figure 7C). In addition, a slight expansion of Ubx toward posterior segments was observed in mlpt RNAi embryos (Figure 7C). Ubx expression was also analyzed upon knockdown of other gap genes, such as Kr, gt, and hunchback (Hb) pRNAi. Kr RNAi embryos show only two pairs of legs and lack of Ubx staining in the legs, suggesting that the third leg (L3) is absent. In addition, a large expansion of Ubx expression toward the posterior region in Kr RNAi embryos was observed. Rp-gt RNAi embryos lack gnathal and abdominal segments and show a duplication of L3. Rp-Hb RNAi embryos show a large expansion of the posterior fates toward the anterior region, also pointing to a major role of this gap gene in R. prolixus (Figure 7D). Altogether, these results suggest that the segmentation role Rp-mlpt role in R. prolixus is rather limited and mainly concentrated at the transition between the thoracic and abdominal region and that classical gap genes, such as kr, gt, hb, display a broader role in the segmental cascade of the hemiptera R. prolixus (Figure 7). Further support for the hypothesis that mlpt function is mainly required for thoracic vs. abdominal distinction is provided by the immunohistochemical analysis using a monoclonal antibody against the homeobox transcription factor ANTP. ANTP demarcates the thoracic segments (T1-T3) and the leg one and two (L1 and L2) in the controls (Figure 7B). As in controls, ANTP is only expressed in L1 and L2 in mlpt dsRNA embryos with four legs (L1–L4), suggesting that L4 in these embryos might be a duplication of L3 (Ray et al., 2019).
Lastly, morphological analysis of the knockdown of the mlpt interaction partner, the transcription factor svb (Kondo et al., 2010; Ray et al., 2019) was performed. As recently demonstrated by Ray et al. (2019) in two hemipteran species, mlpt, and svb, RNAi phenotypes appear remarkably similar. As observed for mlpt RNAi, svb RNAi did not show any alteration on the expression pattern of pb or Ubx either, although germ band elongation and the distinction between thoracic vs. abdominal segmentation was clearly affected (Figure 7C).
Discussion
Nucleotide and Peptide Sequence Analysis Provides Insights Into the Evolution of the mlpt Gene Function
In the past years, genes encoding smORFs have been characterized as new and important players of an unknown part of animal genomes (Saghatelian and Couso, 2015; Zanet et al., 2015; Pueyo et al., 2016a). mlpt is the most widely studied polycistronic gene encoding smORFs in insects. Previous studies and our own sequence analysis presented here has shown that mlpt is not present in chelicerate and myriapod genomes, being restricted to Pancrustacea.
Previous analyses identified specific roles for mlpt short peptides containing LDPTG(L/Q/T)Y motifs in the insect D. melanogaster, while the function of a second weakly conserved predicted peptide (ORF-B in Galindo et al., 2007), remains open since it is not translated in D. melanogaster cell culture nor functionally required in rescue experiments (Galindo et al., 2007; Kondo et al., 2007). Mechanistically, small peptides from mlpt containing LDPTG(L/Q/T)Y motifs are essential for a selective proteasome-mediated N-terminal processing and activation of the transcription factor svb (Kondo et al., 2010; Zanet et al., 2015). The new hemipteran specific peptide identified here (smHemiptera) is ~35% longer in R. prolixus than in the most basally branching species analyzed, Pseudococcus longispinus (Figures 1A,C). It has been recently proposed that smORFs could evolve to major ORFs via an “elongation” pattern (Couso and Patraquim, 2017). Although smHemiptera is not a major ORF, size distribution of the smHemiptera peptide in hemiptera phylogeny corroborates this hypothesis. Future studies should address the function of smHemiptera. It is unlikely that a predicted smORF peptide with a stretch of 14 amino acids with 100% identity over 240 million years would be non-functional. It must be considered that the pRNAi technique used in our study leads to the knockdown of the mature transcript and presumably of all predicted peptides encoded by the hemipteran mlpt gene. Cas9/CRISPR editing technology has been established in other non-model arthropod species (Gilles et al., 2015) and its establishment in R. prolixus might help to unveil the specific function of each small peptide present in Rp-mlpt transcript at different developmental stages. Recent studies demonstrated that mlpt is required for Svb activation in adult tissues, particularly in nephric and intestinal D. melanogaster stem cells (Al Hayek et al., 2019). Since mlpt is also expressed in R. prolixus digestive tract (Ribeiro et al., 2014 and our own observations), it will also be interesting to address the functional role of this gene containing smORFs in other developmental contexts.
To the best of our knowledge, this is the first study to provide a theoretical interaction model for the Svb-Mlpt complexes for D. melanogaster (Dmel) and R. prolixus (Rprol). Our predictions were able to capture previously published data for D. melanogaster (Zanet et al., 2015), even in the absence of experimentally validated 3D structures from D. melanogaster Svb. Secondary structure and fold for both Svb and Mlpts, and protein-binding sites on the N-terminal of Svbs were modeled and agree with (Zanet et al., 2015). Both Svbs (Dmel and Rprol) display around 80–90% conserved disordered regions, but the Zinc-finger double domain near the C-terminal were predicted to be more ordered (Supplementary Figure 1C). The results of the docking assays and binding affinity changes upon mutation on key residues at the interaction interface offer an interesting possibility to propose a theoretical interaction model for Svb-Mlpt of both species (Supplementary Figures 3, 4). Leucines (L24, L25, and L19) and Glutamic Acid (E20) at the N-terminal region of Dmel-Svb affects its interaction with Dmel-Mlpt1, amino acids previously identified in the region of interaction between Dmel-Svb and Dmel_Mlpt1 (Zanet et al., 2015). Additionally, our results suggest that both organisms use at least half of LDPTG(L/Q/T)Y motif residues to anchor on Shavenbaby N-terminal region. All these in silico data might be useful for future NMR approaches to test these structural predictions and to test the effects of mutations in smORF peptides using Cas9/CRISPR technology.
Conservation of mlpt Expression Among Insects
In situ hybridization analysis of the Rp-mlpt gene during embryonic development shows a complex pattern of spatial expression, similarly to previously reported expression patterns in beetles, fruit flies, and other holometabolous insects (Savard et al., 2006; Galindo et al., 2007; Cao et al., 2018; Jiménez-Guri et al., 2018; Zhu et al., 2018; Ray et al., 2019). Most differences in expression have been observed in early developmental stages. In D. melanogaster, the expression occurs in seven blastodermal stripes, a pair-rule pattern, while in most other insect species, including the basally branching Diptera midge Clogmia albipunctata, the expression appears as a gap domain (Jiménez-Guri et al., 2018). Thus, as observed for T. castaneum (Savard et al., 2006) and more recently in the hemiptera O. fasciatus and G. buenoi (Ray et al., 2019), Rp- mlpt is first expressed in an anterior domain overlapping with the head and shortly after expression in a posterior domain overlapping with the prospective thoracic segments (Figure 3). Later on, during germ band elongation and dorsal closure, the dynamic expression of Rp-mlpt is observed in several tissues including the tips of the legs, the head and the antenna (Figure 3). While expression in the head and in the legs have been reported in other species (Ray et al., 2019), expression in the antennae at later stages has, to the best of our knowledge, only been reported here and recently in another hemipteran species O. fasciatus (Ray et al., 2019).
Comparison of Rp-mlpt expression by RT-PCR between fertilized and non-fertilized eggs during the first hours of development (0–6 h AEL) and 36–48 AEL shows 10 times higher expression at later stages when compared to freshly laid or non-fertilized eggs (0–6 h AEL) (Supplementary Figure 6). Although the developmental time of the process of maternal-zygotic transition in R. prolixus is unknown, these results suggest that large changes in mlpt transcriptional levels occurs zygotically, at later stages (36–48 h AEL). It is possible that the translated Mlpt peptides might post-transcriptionally switch Svb from a repressor to an activator at 36–48 h AEL, as previously reported for D. melanogaster (Kondo et al., 2010; Zanet et al., 2015).
mlpt Functions Not as a Classical Gap Gene in R. prolixus but Rather Participates in Thoracic vs. Abdominal Segmental Identity
Three phenotypic classes were observed in mlpt knockdown embryos, mainly affecting segment formation (Figures 4, 5). Comparison of the morphological defects of mlpt knockdown embryos with the defects of transcription factor gap gene knockdowns, such as Kr, Hb, and Gt (Figure 7), showed clear differences (Figure 7). mlpt knockdown embryos display localized phenotypic changes mainly at the transition between thoracic and abdominal segments, while knockdown of the aforementioned transcription factors shows larger effects and changes in molecular marker expression (Figure 7). Our results can be reconciled with several recently published studies about mlpt in other insect orders (Figures 8A,B). In T. castaneum mlpt knockdown leads to extensive homeotic transformations and embryos show up to six leg pairs (Savard et al., 2006). Recent data in two other hemipterans showed that knockdown of three genes mlpt, Ubr3, and svb shows similar phenotypic effects as we describe here for R. prolixus such as posterior truncation, with the fusion/loss of thoracic segments, shortened legs and head appendages (Ray et al., 2019). While in R. prolixus, mlpt RNAi embryos with four pairs of legs were observed, O. fasciatus and G. buenoi knockdown embryos show thoracic segmental fusion, and an increase in the number of thoracic segments was not reported. Since ectopic segment formation after mlpt RNAi occurs in a low frequency in R. prolixus, it is possible that it also occurs at low frequency in O. fasciatus and G. buenoi mlpt knockdown. It is intriguing that, in these three species, mlpt is mainly required when germ band elongation and abdominal segmentation takes place and future studies using embryonic live imaging, as recently described for other insect species (Benton, 2018), might help to understand the role of mlpt during germ band elongation and thoracic vs. abdominal segment specification.
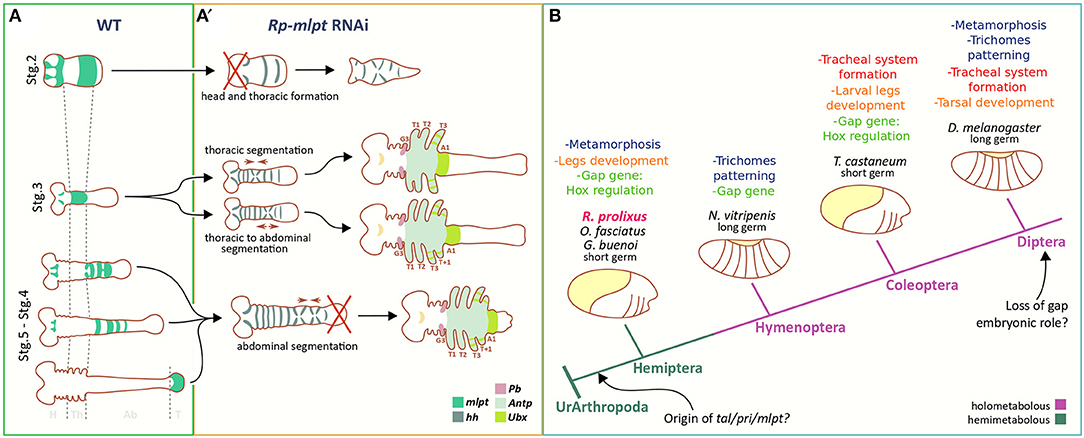
Figure 8. Comparison of mlpt function in R. prolixus and other insect species. (A,A′) In R. prolixus mlpt is required for the correct specification of thoracic vs. abdominal segments, patterning of the distal structures of the legs, and of head formation in strongly affected embryos. (B) Multiple roles of mlpt during insect evolution based on findings of Galindo et al. (2007), Pueyo and Couso (2011), Chanut-Delalande et al. (2014), Zanet et al. (2015), Cao et al. (2018), Jiménez-Guri et al. (2018), Zhu et al. (2018), and Ray et al. (2019) and the current manuscript.
Finally, several distal duplications of leg segments and leg malformations have been observed after Rp-mlpt RNAi (Figure 6). While several leg defects might be attributed to thoracic segment fusion, at least some examples of distal duplications are likely generated by local effects of Rp-mlpt in the legs. We did not detect multiple rings of Rp-mlpt expression in R. prolixus; however, such rings were observed in Periplaneta and also in other hemipteran species (Chesebro, 2013). In summary, our data is compatible with a model that mlpt is required during early stages of R. prolixus development for the distinction between thoracic and abdominal segmentation, during germ band elongation and for head formation (Figure 8A). Our data is important for defining the multiple roles of mlpt in R. prolixus embryogenesis, particularly during early patterning. Since recent analysis in other two hemipteran species, O. fasciatus, and G. buenoi, demonstrated a similar role of mlpt and its interaction partners for early developmental processes (Ray et al., 2019), one can infer that, in the common ancestor of Paraneoptera, mlpt already displayed multiple roles (Figure 8B). Most of these roles of mlpt have been described in Diptera and Coleoptera, and it remains open if all of them are conserved in hemimetabolous insects, as well as its ancestral function in insects (Figure 8B). Lastly, our data contribute to the study of mlpt evolution, providing the interesting finding of a new Hemipteran conserved peptide whose function should be addressed by future functional studies.
Materials and Methods
Bioinformatic Analyses
Mlpt peptide sequences from D. melanogaster and T. castaneum were used for BLAST searches (Altschul et al., 1997) against available arthropod genomes and transcriptomes using relaxed parameters to maximize the chances to obtain genes encoding smORFs. The species used for the smORF identification are depicted in Supplementary Table 1. Rp-mlpt/tal/pri was identified in R. prolixus genome and transcriptomes (Medeiros et al., 2011; Ribeiro et al., 2014; Mesquita et al., 2015; Marchant et al., 2016). BLAST results and domain architectures were manually annotated.
Structural Modeling and Shavenbaby-mlpts Docking Assays
The 3D models of Dmel-Svb (1351 aa; Uniprot: P51521-1) and Rprol-Svb (834 aa; VectorBase: GECK01059228) were predicted via ab initio modeling using the I-Tasser program (Yang et al., 2015). The 3D structures of Mlpts (Dmel-pptd1 and 4) were predicted via CABS-dock (Kurcinski et al., 2015) and I-Tasser, respectively; Rprol-pptd1 and 2, both via CABS-dock. The global and local stereochemical quality of all predicted models were performed by MolProbity (Chen et al., 2010), Verify 3D (Eisenberg et al., 1997), ProSA (Wiederstein and Sippl, 2007), VoroMQA (Olechnovic and Venclovas, 2017), ProQ3D (Uziela et al., 2017), Qprob (Cao and Cheng, 2016), DeepQA (Cao et al., 2016), and SVMQA (Manavalan and Lee, 2017). The most suited structural models were refined by ModRefiner (Xu and Zhang, 2011), 3Drefine (Bhattacharya et al., 2016), GalaxyRefine (Heo et al., 2013), and FG-MD (Zhang et al., 2011). The protein-peptide docking assays were directed to the Shavenbaby N-terminal region (first 31 aa) inspired by the findings of Zanet et al. (2015). The secondary structures, disordered regions and peptide-binding sites predictions on Shavenbabys were further analyzed by PSIPRED (Jones, 1999), Porter (Mirabello and Pollastri, 2013), SPOT-Disorder (Hanson et al., 2017), and DISOPRED3 (Jones and Cozzetto, 2015), respectively. The dockings were performed by HADDOCK (van Zundert et al., 2016). The best interaction complexes were selected after exhausting analyses with DockScore (Malhotra et al., 2015), PPCheck (Sukhwal and Sowdhamini, 2015), and CCharPPI (Moal et al., 2015) programs. After selection of the best complex, further refinements were performed via GalaxyRefineComplex (Heo et al., 2016). The refinements were completed using the “Energy minimization of side chains” function on PDB_Hydro webserver (Azuara et al., 2006). The binding free energy prediction (ΔG) using MM/GBSA method was carried out by HawkDock (Weng et al., 2019), dissociation constant (Kd) prediction was carried out by PRODIGY (Xue et al., 2016), and the analysis of hotspot amino acids located in the interaction interface were performed by HotRegion (Cukuroglu et al., 2012) and ANCHOR (Meireles et al., 2010), respectively. Calculations on the change in the binding energy of the protein-protein complex upon mutations in the residues on interaction interface were performed by BindProfX (Xiong et al., 2017). The electrostatic calculations were carried out by PDB2PQR and APBS (Dolinsky et al., 2004; Unni et al., 2011). Interatomic contacts and types of interactions maintained at Svbs-MLTPs interfaces were analyzed by PDBsum (Laskowski et al., 2018). The images of Svbs-MLTPs complexes were obtained by UCSF Chimera 1.13.1 (Pettersen et al., 2004).
Insect Rearing, Fixation and Dissection
Insect rearing was performed as described by Souza-Ferreira et al. (2014). Approximately 1 week after blood-feeding, eggs were collected daily and fixed in different stages of development. For fixation, up to 100 eggs were briefly washed with distilled water to remove the debris and then transferred to a 1.5 mL microtube containing 1 mL of distilled water. This microtube was maintained for 90 s in boiling water, and after this period, the water was replaced by 1 mL of paraformaldehyde 12% (PBS) and fixed for 1 h (6–8°C). Then, the embryos were incubated with 1 mL of paraformaldehyde 4% containing 0.1% of Tween 20 under agitation (200 rpm) for 1 h at room temperature. The eggs were then washed repeatedly with PBST (PBS 1X, Tween 20 0.1%). For long-term storage, embryos were gradually transferred to ethanol 100% and then stored at −20°C. For the dissections, two forceps (Dumont No. 5) were used. The egg is held with the help of one of them, while the other is used to apply pressure at the chorionic rim to remove the operculum. The chorionic rim is held and the shell is opened transversely, leading to the embryonic release. For later stages, when segmentation is complete, at least stage 6, the yolk is easily removed, either with the help of a thin forceps or a glass needle, without damaging the embryo.
In situ Hybridization, Nuclear, and Antibody Staining
Embryos stored in ethanol 100% at −20°C were gradually transferred to PBST at room temperature. In situ hybridization and probe synthesis were performed as described by (Sachs et al., 2015), including the proteinase K treatment. DAPI (4′,6-Diamidine-2′-phenylindole dihydrochloride, SIGMA) staining was performed as in Berni et al. (2014). Antibody staining followed the protocol described in Santos et al. (2013). All the images were acquired with the stereoscope Leica M205, and processed and analyzed with the software Leica Application Suite Advanced Fluorescence Version 0.4 (LAS AF v4—Leica Microsystems). Images were assembled and the in situ hybridization staining (NBT/BCIP) was converted to a false fluorescence as described in Benton et al. (2016).
RNA Interference and Real-Time PCR
The RNA interference (RNAi) was performed similarly to Berni et al. (2014) using a non-related dsRNA as a control (neomycin dsRNA-dsneo). For Rp-mlpt, two non-overlapping PCR fragments containing T7 promoter initiation sites at both ends were used as templates for dsRNA synthesis using Ambion T7 Megascript Kit (Cat. No. AM1334). The amount and integrity of the dsRNA samples were measured by spectrophotometry and agarose gel electrophoresis, respectively. For each silenced gene, between 6 and 12 μg of dsRNA were injected. The quantification of RNAi efficiency and comparison of gene expression after silencing was measured through real-time PCR (RT-PCR) as performed in Berni et al. (2014) using the gene Elongation factor-1 (Ef1) as endogenous reference gene. Gene bank or Vector base accession numbers of the genes analyzed by in situ hybridization or RT-PCR are provided in the Supplementary Table 4. For RT-PCR analysis, RNA was extracted in biological triplicates from eggs at appropriate hours after egg lay (AEL). Fertilized eggs (0–6 and 36–48 h AEL) and non-fertilized eggs obtained from female virgins (0–6 h AEL) were used for the analysis.
Data Availability Statement
The datasets generated for this study can be found in the NCBI.
Author Contributions
VT-S, DG-A, FM, LR, MB, HA, CL, NF, JS-M, EP, and RN: experimental data generation and interpretation. HA, CL, and RN: funding acquisition. VT-S and RN: manuscript draft.
Funding
RN was supported by CNPq (307952/2017-7 and 431354/2016-2) and FAPERJ (E-26/210-150/2016 and E-26/203.298/2016). VT-S and DG-A were master students of PPG-PRODBIO-Macaé (CAPES scholarships), MB a Ph.D. student from PPG-PCM-ICB-UFRJ and LR was a postdoc with CAPES scholarship from the National Institute of Molecular Entomology/CNPq (INCT-EM).
Conflict of Interest
The authors declare that the research was conducted in the absence of any commercial or financial relationships that could be construed as a potential conflict of interest.
Acknowledgments
The authors thank Roland Zimm for helpful suggestions in the manuscript.
Supplementary Material
The Supplementary Material for this article can be found online at: https://www.frontiersin.org/articles/10.3389/fevo.2019.00379/full#supplementary-material
References
Al Hayek, S., Alsawadi, A., Kambris, Z., Boquete, J.-P., Bohère, J., Ronsin, B., et al. (2019). Shavenbaby protein isoforms orchestrate the self-renewal versus differentiation of Drosophila ntestinal stem cells. bioRxiv. doi: 10.1101/627554
Altschul, S. F., Madden, T. L., Schäffer, A. A., Zhang, J., Zhang, Z., Miller, W., et al. (1997). Gapped BLAST and PSI-BLAST: a new generation of protein database search programs. Nucleic Acids Res. 25, 3389–3402. doi: 10.1093/nar/25.17.3389
Aspden, J. L., Eyre-Walker, Y. C., Phillips, R. J., Amin, U., Mumtaz, M. A., Brocard, M., et al. (2014). Extensive translation of small open reading frames revealed by Poly-Ribo-Seq. eLife 3:e03528. doi: 10.7554/eLife.03528
Auman, T., Vreede, B. M. I., Weiss, A., Hester, S. D., Williams, T. A., Nagy, L. M., et al. (2017). Dynamics of growth zone patterning in the milkweed bug Oncopeltus fasciatus. Development 144, 1896–1905. doi: 10.1242/dev.142091
Azuara, C., Lindahl, E., Koehl, P., Orland, H., and Delarue, M. (2006). PDB_Hydro: incorporating dipolar solvents with variable density in the Poisson-Boltzmann treatment of macromolecule electrostatics. Nucleic Acids Res. 34, W38–42. doi: 10.1093/nar/gkl072
Benton, M. A. (2018). A revised understanding of Tribolium morphogenesis further reconciles short and long germ development. PLoS Biol. 16:e2005093. doi: 10.1371/journal.pbio.2005093
Benton, M. A., Pechmann, M., Frey, N., Stappert, D., Conrads, K. H., Chen, Y. T., et al. (2016). Toll genes have an ancestral role in axis elongation. Curr. Biol. 26, 1609–1615. doi: 10.1016/j.cub.2016.04.055
Berni, M., Fontenele, M. R., Tobias-Santos, V., Caceres-Rodrigues, A., Mury, F. B., Vionette-do-Amaral, R., et al. (2014). Toll signals regulate dorsal-ventral patterning and anterior-posterior placement of the embryo in the hemipteran Rhodnius prolixus. Evodevo 5:38. doi: 10.1186/2041-9139-5-38
Bhattacharya, D., Nowotny, J., Cao, R., and Cheng, J. (2016). 3Drefine: an interactive web server for efficient protein structure refinement. Nucleic Acids Res. 44, W406–W409. doi: 10.1093/nar/gkw336
Brito, T., Julio, A., Berni, M., de Castro Poncio, L., Bernardes, E. S., Araujo, H., et al. (2018). Transcriptomic and functional analyses of the piRNA pathway in the chagas disease vector Rhodnius prolixus. PLoS Negl Trop Dis 12:e0006760. doi: 10.1371/journal.pntd.0006760
Cao, G., Gong, Y., Hu, X., Zhu, M., Liang, Z., Huang, L., et al. (2018). Identification of tarsal-less peptides from the silkworm Bombyx mori. Appl. Microbiol. Biotechnol. 102, 1809–1822. doi: 10.1007/s00253-017-8708-4
Cao, R., Bhattacharya, D., Hou, J., and Cheng, J. (2016). DeepQA: improving the estimation of single protein model quality with deep belief networks. BMC Bioinformatics 17:495. doi: 10.1186/s12859-016-1405-y
Cao, R., and Cheng, J. (2016). Protein single-model quality assessment by feature-based probability density functions. Sci. Rep. 6:23990. doi: 10.1038/srep23990
Chanut-Delalande, H., Hashimoto, Y., Pelissier-Monier, A., Spokony, R., Dib, A., Kondo, T., et al. (2014). Pri peptides are mediators of ecdysone for the temporal control of development. Nat. Cell Biol. 16, 1035–1044. doi: 10.1038/ncb3052
Chekulaeva, M., and Rajewsky, N. (2018). Roles of long noncoding RNAs and circular RNAs in translation. Cold Spring Harb. Perspect. Biol. 11:a032680doi: 10.1101/cshperspect.a032680
Chen, V. B., Arendall, W. B. III., Headd, J. J., Keedy, D. A., Immormino, R. M., Kapral, G. J., et al. (2010). MolProbity: all-atom structure validation for macromolecular crystallography. Acta Crystallogr D Biol. Crystallogr. 66, 12–21. doi: 10.1107/S0907444909042073
Chesebro, J. (2013). Mechanisms of segmentation in the American cockroach, Periplaneta americana (Doctoral thesis, PhD). Sussex: University of Sussex.
Couso, J. P., and Patraquim, P. (2017). Classification and function of small open reading frames. Nat. Rev. Mol. Cell Biol. 18, 575–589. doi: 10.1038/nrm.2017.58
Cukuroglu, E., Gursoy, A., and Keskin, O. (2012). HotRegion: a database of predicted hot spot clusters. Nucleic Acids Res. 40, D829–D833. doi: 10.1093/nar/gkr929
Dolinsky, T. J., Nielsen, J. E., McCammon, J. A., and Baker, N. A. (2004). PDB2PQR: an automated pipeline for the setup of Poisson-Boltzmann electrostatics calculations. Nucleic Acids Res. 32, W665–W667. doi: 10.1093/nar/gkh381
Eisenberg, D., Lüthy, R., and Bowie, J. U. (1997). VERIFY3D: assessment of protein models with three-dimensional profiles. Methods Enzymol. 277, 396–404. doi: 10.1016/S0076-6879(97)77022-8
Galindo, M. I., Pueyo, J. I., Fouix, S., Bishop, S. A., and Couso, J. P. (2007). Peptides encoded by short ORFs control development and define a new eukaryotic gene family. PLoS Biol. 5:e106. doi: 10.1371/journal.pbio.0050106
Gilles, A. F., Schinko, J. B., and Averof, M. (2015). Efficient CRISPR-mediated gene targeting and transgene replacement in the beetle Tribolium castaneum. Development 142, 2832–2839. doi: 10.1242/dev.125054
Hanson, J., Yang, Y., Paliwal, K., and Zhou, Y. (2017). Improving protein disorder prediction by deep bidirectional long short-term memory recurrent neural networks. Bioinformatics 33, 685–692. doi: 10.1093/bioinformatics/btw678
Heo, L., Lee, H., and Seok, C. (2016). GalaxyRefineComplex: refinement of protein-protein complex model structures driven by interface repacking. Sci. Rep. 6:32153. doi: 10.1038/srep32153
Heo, L., Park, H., and Seok, C. (2013). GalaxyRefine: protein structure refinement driven by side-chain repacking. Nucleic Acids Res. 41, W384–W388. doi: 10.1093/nar/gkt458
Jiménez-Guri, E., Wotton, K. R., and Jaeger, J. (2018). tarsal-less is expressed as a gap gene but has no gap gene phenotype in the moth midge Clogmia albipunctata. R. Soc. Open Sci. 5:180458. doi: 10.1098/rsos.180458
Jones, D. T. (1999). Protein secondary structure prediction based on position-specific scoring matrices. J. Mol. Biol. 292, 195–202. doi: 10.1006/jmbi.1999.3091
Jones, D. T., and Cozzetto, D. (2015). DISOPRED3: precise disordered region predictions with annotated protein-binding activity. Bioinformatics 31, 857–63. doi: 10.1093/bioinformatics/btu744
Kondo, T., Hashimoto, Y., Kato, K., Inagaki, S., Hayashi, S., and Kageyama, Y. (2007). Small peptide regulators of actin-based cell morphogenesis encoded by a polycistronic mRNA. Nat. Cell Biol. 9, 660–665. doi: 10.1038/ncb1595
Kondo, T., Plaza, S., Zanet, J., Benrabah, E., Valenti, P., Hashimoto, Y., et al. (2010). Small peptides switch the transcriptional activity of Shavenbaby during Drosophila embryogenesis. Science 329, 336–339. doi: 10.1126/science.1188158
Kumar, S., Stecher, G., Suleski, M., and Hedges, S. B. (2017). TimeTree: a resource for timelines, timetrees, and divergence times. Mol. Biol. Evol. 34, 1812–1819. doi: 10.1093/molbev/msx116
Kurcinski, M., Jamroz, M., Blaszczyk, M., Kolinski, A., and Kmiecik, S. (2015). CABS-dock web server for the flexible docking of peptides to proteins without prior knowledge of the binding site. Nucleic Acids Res. 43, W419–W424. doi: 10.1093/nar/gkv456
Ladoukakis, E., Pereira, V., Magny, E. G., Eyre-Walker, A., and Couso, J. P. (2011). Hundreds of putatively functional small open reading frames in Drosophila. Genome Biol. 12:R118. doi: 10.1186/gb-2011-12-11-r118
Laskowski, R. A., Jabłonska, J., Pravda, L., Vareková, R. S., and Thornton, J. M. (2018). PDBsum: structural summaries of PDB entries. Protein Sci. 27, 129–134. doi: 10.1002/pro.3289
Lavore, A., Esponda-Behrens, N., Pagola, L., and Rivera-Pomar, R. (2014). The gap gene Kruppel of Rhodnius prolixus is required for segmentation and for repression of the homeotic gene sex comb-reduced. Dev. Biol. 387, 121–129. doi: 10.1016/j.ydbio.2013.12.030
Lavore, A., Pagola, L., Esponda-Behrens, N., and Rivera-Pomar, R. (2012). The gap gene giant of Rhodnius prolixus is maternally expressed and required for proper head and abdomen formation. Dev. Biol. 361, 147–155. doi: 10.1016/j.ydbio.2011.06.038
Magny, E. G., Pueyo, J. I., Pearl, F. M., Cespedes, M. A., Niven, J. E., Bishop, S. A., et al. (2013). Conserved regulation of cardiac calcium uptake by peptides encoded in small open reading frames. Science 341, 1116–1120. doi: 10.1126/science.1238802
Malhotra, S., Mathew, O. K., and Sowdhamini, R. (2015). DOCKSCORE: a webserver for ranking protein-protein docked poses. BMC Bioinformatics 16:127. doi: 10.1186/s12859-015-0572-6
Manavalan, B., and Lee, J. (2017). SVMQA: support-vector-machine-based protein single-model quality assessment. Bioinformatics 33, 2496–2503. doi: 10.1093/bioinformatics/btx222
Marchant, A., Mougel, F., Mendonça, V., Quartier, M., Jacquin-Joly, E., da Rosa, J. A., et al. (2016). Comparing de novo and reference-based transcriptome assembly strategies by applying them to the blood-sucking bug Rhodnius prolixus. Insect Biochem. Mol. Biol. 69, 25–33. doi: 10.1016/j.ibmb.2015.05.009
Medeiros, M. N., Logullo, R., Ramos, I. B., Sorgine, M. H., Paiva-Silva, G. O., Mesquita, R. D., et al. (2011). Transcriptome and gene expression profile of ovarian follicle tissue of the triatomine bug Rhodnius prolixus. Insect Biochem. Mol. Biol. 41, 823–831. doi: 10.1016/j.ibmb.2011.06.004
Meireles, L. M., Dömling, A. S., and Camacho, C. J. (2010). ANCHOR: a web server and database for analysis of protein-protein interaction binding pockets for drug discovery. Nucleic Acids Res. 38, W407–W411. doi: 10.1093/nar/gkq502
Mesquita, R. D., Vionette-Amaral, R. J., Lowenberger, C., Rivera-Pomar, R., Monteiro, F. A., Minx, P., et al. (2015). Genome of Rhodnius prolixus, an insect vector of Chagas disease, reveals unique adaptations to hematophagy and parasite infection. Proc. Natl. Acad. Sci. U.S.A. 112, 14936–14941. doi: 10.1073/pnas.1506226112
Mirabello, C., and Pollastri, G. (2013). Porter, PaleAle 4.0: high-accuracy prediction of protein secondary structure and relative solvent accessibility. Bioinformatics 29, 2056–2058. doi: 10.1093/bioinformatics/btt344
Moal, I. H., Jiménez-García, B., and Fernández-Recio, J. (2015). CCharPPI web server: computational characterization of protein-protein interactions from structure. Bioinformatics 31, 123–125. doi: 10.1093/bioinformatics/btu594
Nunes-da-Fonseca, R., Berni, M., Tobias-Santos, V., Pane, A., and Araujo, H. M. (2017). Rhodnius prolixus: from classical physiology to modern developmental biology. Genesis 55, 1–11. doi: 10.1002/dvg.22995
Nüsslein-Volhard, C., and Wieschaus, E. (1980). Mutations affecting segment number and polarity in Drosophila. Nature 287, 795–801. doi: 10.1038/287795a0
Olechnovic, K., and Venclovas, C. (2017). VoroMQA: assessment of protein structure quality using interatomic contact areas. Proteins 85, 1131–1145. doi: 10.1002/prot.25278
Paese, C. L. B., Schoenauer, A., Leite, D. J., Russell, S., and McGregor, A. P. (2018). A SoxB gene acts as an anterior gap gene and regulates posterior segment addition in a spider. eLife 7:e37567. doi: 10.7554/eLife.37567
Panfilio, K. A. (2008). Extraembryonic development in insects and the acrobatics of blastokinesis. Dev. Biol. 313, 471–91. doi: 10.1016/j.ydbio.2007.11.004
Panfilio, K. A., and Angelini, D. R. (2018). By land, air, and sea: hemipteran diversity through the genomic lens. Curr. Opin. Insect Sci. 25, 106–115. doi: 10.1016/j.cois.2017.12.005
Panfilio, K. A., Vargas Jentzsch, I. M., Benoit, J. B., Erezyilmaz, D., Suzuki, Y., Colella, S., et al. (2019). Molecular evolutionary trends and feeding ecology diversification in the Hemiptera, anchored by the milkweed bug genome. Genome Biol. 20:64. doi: 10.1186/s13059-019-1660-0
Pettersen, E. F., Goddard, T. D., Huang, C. C., Couch, G. S., Greenblatt, D. M., Meng, E. C., et al. (2004). UCSF Chimera–a visualization system for exploratory research and analysis. J. Comput. Chem. 25, 1605–1612. doi: 10.1002/jcc.20084
Pueyo, J. I., and Couso, J. P. (2011). Tarsal-less peptides control Notch signalling through the Shavenbaby transcription factor. Dev. Biol. 355, 183–193. doi: 10.1016/j.ydbio.2011.03.033
Pueyo, J. I., Magny, E. G., and Couso, J. P. (2016a). New peptides under the s(ORF)ace of the Genome. Trends Biochem. Sci. 41, 665–678. doi: 10.1016/j.tibs.2016.05.003
Pueyo, J. I., Magny, E. G., Sampson, C. J., Amin, U., Evans, I. R., Bishop, S. A., et al. (2016b). Hemotin, a regulator of phagocytosis encoded by a small ORF and conserved across metazoans. PLoS Biol. 14:e1002395. doi: 10.1371/journal.pbio.1002395
Ray, S., Rosenberg, M. I., Chanut-Delalande, H., Decaras, A., Schwertner, B., Toubiana, W., et al. (2019). The mlpt/Ubr3/Svb module comprises an ancient developmental switch for embryonic patterning. eLife 8:e39748. doi: 10.7554/eLife.39748
Ribeiro, J. M., Genta, F. A., Sorgine, M. H., Logullo, R., Mesquita, R. D., Paiva-Silva, G. O., et al. (2014). An insight into the transcriptome of the digestive tract of the bloodsucking bug, Rhodnius prolixus. PLoS Negl. Trop. Dis. 8:e2594. doi: 10.1371/journal.pntd.0002594
Sachs, L., Chen, Y. T., Drechsler, A., Lynch, J. A., Panfilio, K. A., Lässig, M., et al. (2015). Dynamic BMP signaling polarized by Toll patterns the dorsoventral axis in a hemimetabolous insect. eLife 4:e05502. doi: 10.7554/eLife.05502
Saghatelian, A., and Couso, J. P. (2015). Discovery and characterization of smORF-encoded bioactive polypeptides. Nat. Chem. Biol. 11, 909–916. doi: 10.1038/nchembio.1964
Santos, V. T., Ribeiro, L., Fraga, A., de Barros, C. M., Campos, E., Moraes, J., et al. (2013). The embryogenesis of the tick Rhipicephalus (Boophilus) microplus: the establishment of a new chelicerate model system. Genesis 51, 803–818. doi: 10.1002/dvg.22717
Savard, J., Marques-Souza, H., Aranda, M., and Tautz, D. (2006). A segmentation gene in tribolium produces a polycistronic mRNA that codes for multiple conserved peptides. Cell 126, 559–569. doi: 10.1016/j.cell.2006.05.053
Schwager, E. E., Sharma, P. P., Clarke, T., Leite, D. J., Wierschin, T., Pechmann, M., et al. (2017). The house spider genome reveals an ancient whole-genome duplication during arachnid evolution. BMC Biol. 15:62. doi: 10.1186/s12915-017-0399-x
Souza-Ferreira, P. S., Moreira, M. F., Atella, G. C., Oliveira-Carvalho, A. L., Eizemberg, R., Majerowicz, D., et al. (2014). Molecular characterization of Rhodnius prolixus' embryonic cuticle. Insect Biochem. Mol. Biol. 51, 89–100. doi: 10.1016/j.ibmb.2013.12.005
Sukhwal, A., and Sowdhamini, R. (2015). PPCheck: a webserver for the quantitative analysis of protein-protein interfaces and prediction of residue hotspots. Bioinform Biol. Insights 9, 141–151. doi: 10.4137/BBI.S25928
Tribolium Genome Sequencing Consortium, Richards, S., Gibbs, R. A., Weinstock, G. M., Brown, S. J., Denell, R., et al. (2008). The genome of the model beetle and pest Tribolium castaneum. Nature 452, 949–955. doi: 10.1038/nature06784
Unni, S., Huang, Y., Hanson, R. M., Tobias, M., Krishnan, S., Li, W. W., et al. (2011). Web servers and services for electrostatics calculations with APBS and PDB2PQR. J. Comput. Chem. 32, 1488–1491. doi: 10.1002/jcc.21720
Uziela, K., Menéndez Hurtado, D., Shu, N., Wallner, B., and Elofsson, A. (2017). ProQ3D: improved model quality assessments using deep learning. Bioinformatics 33, 1578–1580. doi: 10.1093/bioinformatics/btw819
van Zundert, G. C. P., Rodrigues, J. P. G. L. M., Trellet, M., Schmitz, C., Kastritis, P. L., Karaca, E., et al. (2016). The HADDOCK2.2 web server: user-friendly integrative modeling of biomolecular complexes. J. Mol. Biol. 428, 720–725. doi: 10.1016/j.jmb.2015.09.014
Weng, G., Wang, E., Wang, Z., Liu, H., Zhu, F., Li, D., et al. (2019). HawkDock: a web server to predict and analyze the protein-protein complex based on computational docking and MM/GBSA. Nucleic Acids Res. 47, W322–W330. doi: 10.1093/nar/gkz397
Wiederstein, M., and Sippl, M. J. (2007). ProSA-web: interactive web service for the recognition of errors in three-dimensional structures of proteins. Nucleic Acids Res. 35, W407–W410. doi: 10.1093/nar/gkm290
Xiong, P., Zhang, C., Zheng, W., and Zhang, Y. (2017). BindProfX: assessing mutation-induced binding affinity change by protein interface profiles with pseudo-counts. J. Mol. Biol. 429, 426–434. doi: 10.1016/j.jmb.2016.11.022
Xu, D., and Zhang, Y. (2011). Improving the physical realism and structural accuracy of protein models by a two-step atomic-level energy minimization. Biophys. J. 101, 2525–2534. doi: 10.1016/j.bpj.2011.10.024
Xue, L. C., Rodrigues, J. P., Kastritis, P. L., Bonvin, A. M., and Vangone, A. (2016). PRODIGY: a web server for predicting the binding affinity of protein-protein complexes. Bioinformatics 32, 3676–3678. doi: 10.1093/bioinformatics/btw514
Yang, J., Yan, R., Roy, A., Xu, D., Poisson, J., and Zhang, Y. (2015). The I-TASSER Suite: protein structure and function prediction. Nat. Methods 12, 7–8. doi: 10.1038/nmeth.3213
Zanet, J., Benrabah, E., Li, T., Pélissier-Monier, A., Chanut-Delalande, H., Ronsin, B., et al. (2015). Pri sORF peptides induce selective proteasome-mediated protein processing. Science 349, 1356–1358. doi: 10.1126/science.aac5677
Zanet, J., Chanut-Delalande, H., Plaza, S., and Payre, F. (2016). Small peptides as newcomers in the control of Drosophila development. Curr. Top. Dev. Biol. 117, 199–219. doi: 10.1016/bs.ctdb.2015.11.004
Zhang, J., Liang, Y., and Zhang, Y. (2011). Atomic-level protein structure refinement using fragment-guided molecular dynamics conformation sampling. Structure 19, 1784–1795. doi: 10.1016/j.str.2011.09.022
Keywords: smORF, peptides, evo-devo, arthropod development, hemiptera, evolution, MZT, hemimetabolous insect
Citation: Tobias-Santos V, Guerra-Almeida D, Mury F, Ribeiro L, Berni M, Araujo H, Logullo C, Feitosa NM, de Souza-Menezes J, Pessoa Costa E and Nunes-da-Fonseca R (2019) Multiple Roles of the Polycistronic Gene Tarsal-less/Mille-Pattes/Polished-Rice During Embryogenesis of the Kissing Bug Rhodnius prolixus. Front. Ecol. Evol. 7:379. doi: 10.3389/fevo.2019.00379
Received: 12 June 2019; Accepted: 20 September 2019;
Published: 11 October 2019.
Edited by:
Elizabeth Jenness Duncan, University of Leeds, United KingdomReviewed by:
Leslie Pick, University of Maryland, College Park, United StatesMiriam Rosenberg, Hebrew University of Jerusalem, Israel
Copyright © 2019 Tobias-Santos, Guerra-Almeida, Mury, Ribeiro, Berni, Araujo, Logullo, Feitosa, de Souza-Menezes, Pessoa Costa and Nunes-da-Fonseca. This is an open-access article distributed under the terms of the Creative Commons Attribution License (CC BY). The use, distribution or reproduction in other forums is permitted, provided the original author(s) and the copyright owner(s) are credited and that the original publication in this journal is cited, in accordance with accepted academic practice. No use, distribution or reproduction is permitted which does not comply with these terms.
*Correspondence: Rodrigo Nunes-da-Fonseca, cm5mb25zZWNhQG1hY2FlLnVmcmouYnI=