- 1Department of Biological Sciences, University of Alberta, Edmonton, AB, Canada
- 2College of Forestry and Conservation, University of Montana, Missoula, MT, United States
Partial migration, a phenomenon wherein only some individuals within a population migrate, is taxonomically widespread. While well-studied in birds and fish, partial migration in large herbivores has come into the spotlight only recently due to the decline of migratory behavior in ungulate species around the world. We explored whether partial migration in ungulates is maintained at the population level through frequency-dependence, an environmental-genetic threshold, or a conditional strategy. Through a review of studies describing individual variation in migratory behavior, we then addressed how density-dependent and -independent factors such as social constraints, competition for forage, and escape from predators or pathogens, alone or together, could lead to occurrence of both migrants and residents within a population. We searched for evidence that intrinsic and extrinsic factors could combine with genetic predispositions and individual differences in temperament or life experience to promote migratory tendencies of individuals. Despite the long-held assumption for ungulates that migration is a fixed behavior of individuals, evidence suggested that flexibility in migratory behavior is more common than previously thought. Partial migration maintained by a conditional strategy results in changes in movement tactics as state-dependent responses of individuals. Data are needed to empirically demonstrate which factors determine the relative costs and benefits to using migratory vs. resident tactics. We outline what types of long-term data could address this need and urge those studying migration to meet these challenges in the interest of conserving partially migratory populations.
Introduction
Dramatic declines in populations of migratory ungulates and the disappearance of migratory behavior in many ungulate species are now recognized as a global conservation challenge (Berger, 2004; Bolger et al., 2008; Tucker et al., 2018). Population reductions have been well-documented in migratory species ranging from antelope (Antidorcas marsupialis, Child and Le Riche, 1969; Saiga tatarica, Milner-Gulland et al., 2001) and buffalo (Syncerus caffer caffer, Bennitt et al., 2016) to wildebeest (Connochaetes taurinus, Gasaway et al., 1996) and zebra (Equus burchelli antiquorum, Bartlam-Brooks et al., 2013). Loss of migratory behavior in ungulates is attributed primarily to human-induced changes to landscapes, which may be exacerbated by climate change (Lendrum et al., 2013). Loss of migration can have significant ecological impacts, potentially resulting in collapse of whole ecosystems, extending from alteration of plant composition and ecosystem processes such as grassland production and nitrogen mineralization (McNaughton et al., 1988; Frank, 1998; Holdo et al., 2006), to declines in other species including apex predators (Packer et al., 2005; Lee et al., 2016), to loss of wildlife tourism-based dollars normally used for environmental protection of Africa's most iconic species (Harris et al., 2009; Holdo et al., 2011). Given the potential severity of these ecological impacts and their associated economic consequences, identifying the processes that lead to migratory behavior should be a primary focus of biodiversity research and conservation efforts to address the loss of migration in ungulate populations (Bolger et al., 2008).
Migratory movements of individuals are expected to arise in variable environments wherein ungulates migrate to enhance lifetime reproductive fitness by gaining access to critical resources such as nutrients or water, reduce their likelihood of predation, or escape parasites (Fryxell and Sinclair, 1988a; Mysterud et al., 2011, 2016; Qviller et al., 2013). However, anthropogenic disturbances and environmental changes have sometimes altered the relative benefits of migrating in large herbivores to make residency more profitable (Berger, 2004; Hebblewhite et al., 2006; Jones et al., 2014). Partial migration is a population-level phenomenon in which a population is comprised of both resident and migrant individuals (Chapman et al., 2011a). Partial migration has become a focus for studies on ungulates only recently, and is presumed to result from trade-offs between the costs and benefits of migration (Eggeman et al., 2016). Although several studies have described the pattern of partial migration, the underlying ecological processes, which we review below, for maintaining partial migration are theoretical or empirically correlative. Experimental manipulations needed to identify mechanisms driving migratory tendency in large mammals may be unethical and are difficult (but see below), which creates an urgent need to better synthesize existing information on partial migration in ungulates. A better understanding of the worldwide decline in migratory behavior of ungulates will offer directions for future studies and inform associated conservation actions (Bolger et al., 2008).
We explore this topic with a review that begins by defining migrant and resident behavior in the context of partial migration. We then review the evidence for population-level mechanisms described by others to explain why partial migration occurs and is maintained in diverse populations of ungulates that inhabit variable environments. We explain how changes in proportions of migrants and residents within populations might occur both across generations, through either a frequency- or density-dependent fitness equilibrium, and within generations, via behavioral switching between migrant and resident behavior by individuals. Then we review the factors operating on individuals that might promote migration vs. residency. We focus primarily on genetic variability, social interactions and cultural inheritance, intrinsic factors such as age and nutritional condition, and extrinsic or environmental factors such as forage and predation risk. We conducted the review by searching the published literature for all ungulate species listed in Ultimate Ungulate (Huffman, 2018) and by Groves and Grubb (2011) within the orders Perissodactyla (odd-toed ungulates) and Cetartiodactyla (even-toed ungulates). We used “Web of Science” and “Google Scholar” search engines to find articles by the common and Latin name and/or genus in combination with “migra*,” “resid*,” “partia* migra*,” “facultative migra*,” or “conditional migra*.” In particular, we retained any article that described partial migration (i.e., the article needed to state that a portion of the population remained resident/sedentary, and another portion of the population migrated, irrespective of the form of migration observed) and addressed or speculated on the reasons behind the observed differences in migratory behavior. We chose not to include gray literature due to variability in data types and rigor. The hypotheses we evaluated are not mutually exclusive and two or more proximate mechanisms for migration are likely to operate simultaneously (Ketterson and Nolan, 1983; Smith and Nilsson, 1987; Avgar et al., 2014). The review focused on migration in female ungulates because adult female survival is thought to have the greatest influence on large ungulate population dynamics (Gaillard et al., 1998; Raithel et al., 2007) and because few articles concentrated on males or compared factors affecting migratory behavior between the sexes; we included migratory tendency in males if new mechanisms arose and there were adequate data (see Supplementary Tables 1, 2). We end by challenging researchers to collect the long-term data necessary to test the mechanisms underlying maintenance of partial migration to bring us closer to conserving ungulate populations in the face of ongoing environmental change.
What is a Migrant?
Migration as a phenomenon is not easy to define because of variation in both terminology and types of animal movement among taxa (Sinclair, 1983; Fryxell et al., 2011). The term migration is also used differently when it is applied to individuals vs. populations (Dingle and Drake, 2007; Dingle, 2014b). In either context, associating migration with a trait or a behavioral syndrome (sensu Sih et al., 2004) requires that migration responds to natural selection (Dingle, 2014b), but it may do so as part of a correlated suite of behavioral, physiological, or life history traits (Réale et al., 2010). In this review, we define migration as a behavioral tactic (sensu Dominey, 1984; Gross, 1996; Dawkins, 1999) describing a movement type that is exhibited by individuals (Table 1). We call it a tactic, rather than assume it is a genetically fixed strategy (sensu Maynard Smith, 1982) because of the information we synthesized during our review (below). Consistent with this definition as a tactic, the migratory tendency of an individual could be rigid and result from conditions during a key developmental window (i.e., phenotypic plasticity or reaction norm) or change over time (i.e., ongoing behavioral flexibility; Piersma and Drent, 2003). We explore the evidence for these mechanisms below.
Additional confusion about the meaning of migration stems from spatial definitions. Ungulates are among the taxa for which migration is thought to be movement, most commonly, but not always, as a round-trip between discrete seasonal ranges (Sinclair, 1983; Fryxell and Sinclair, 1988a). The spatiotemporal separation between ranges and the emphasis on return movement makes migration different from: (1) dispersal, a relatively short-term, one-time movement to a new population or a new range primarily for the purpose of reproduction; (2) nomadism or roaming, where animals follow resource pulses with little spatial predictability; and (3) residency, where there is continuous, overlapping use of the same range (McPeek and Holt, 1992; Hjeljord, 2001; Abrahms et al., 2017). Distinguishing between migratory tactics using seasonal ranges becomes challenging when individuals exhibit more idiosyncratic or mixed movements, such as returning to a seasonal range soon after leaving it (Dingle and Drake, 2007; Dingle, 2014b). Describing migration as a round-trip is problematic when individuals switch among multiple ranges and do not return to the same seasonal range they used the summer or winter before (e.g., Eggeman et al., 2016). Variation in the spatial extent of migratory movement reinforces that partial migration is not the simple dichotomy that is implied by terms like migrant vs. resident or short-distance (<10–50 km) vs. long-distance migrant (>50–150 km; Table 2). Indeed, some authors consider the choice to migrate as one point in a continuum of movement responses that occur over multiple scales of spatiotemporal variability (Cagnacci et al., 2011).
Greater latitude in the way migration is defined, behaviorally and spatially, may lessen the need for several quantitative methods used to distinguish migration from other types of movements and to classify variation in migratory movements (Cagnacci et al., 2016; Singh et al., 2016; Abrahms et al., 2017; Peters et al., 2019). Migrants are often distinguished from residents based on criteria such as the amount of seasonal home range overlap (Mysterud, 1999; Ball et al., 2001; Fieberg and Kochanny, 2005), trajectory segmentation (Buchin et al., 2013), or algorithms that cluster seasonal locations (Cagnacci et al., 2011, 2016; Damiani et al., 2016). A second approach is based on Correlated Random Walk (CRW) models (Bergman et al., 2000), including the increasingly popular Net Squared Displacement (NSD), measured as the cumulative squared displacement from a starting point (Turchin, 1998; Nouvellet et al., 2009; Bunnefeld et al., 2011). The drawback to NSD is that it can be computationally complex and often requires ad hoc reclassification of the migratory status of an individual (Spitz et al., 2017). On the other hand, this method is capable of quantifying different types of movement along a continuum, overcoming the problem of simplistic dichotomies (Singh et al., 2016). Despite the limitations in methodologies, quantifying animal movements as migratory behavior is a first step in exploring how partial migration is maintained.
Maintenance of Partial Migration in Ungulate Populations
Historically, partial migration was simply described as a kind of within-population variation in movement behavior in which just a part of the population migrates (Lack, 1943) with speculation about causation (e.g., Lack, 1943; Lundberg, 1988). Modern assessments have since evolved to developing theoretical frameworks for hypotheses that need to be tested with empirical data (Kokko, 2007, 2011; Lundberg, 2013). Both past and modern interpretations assume that migration results from natural selection such that the occurrence of partial migration requires the long-term balancing of Darwinian fitness between migrant and resident tactics under different ecological conditions. Such polymorphisms in life history tactics are maintained over evolutionary time only if fitness varies with population densities, environmental conditions, or similar phenomena (Swingland and Lessells, 1979). More specifically, natural selection could favor the maintenance of partial migration within a population via: (1) a frequency-dependent mixed evolutionarily stable strategy (ESS; Swingland, 1983; Dingle, 2014b), (2) an environmental-genetic threshold, a variant of a gene-environment interaction that accommodates changing environments (Pulido, 2011), or (3) a conditional strategy in which an individual's choice of migratory tactic varies with other aspects of phenotype, individual state, or the behavior of other individuals in the population (Lundberg, 1987; Chapman et al., 2011b, 2012; Pulido, 2011). Each of these mechanisms might prevail under different environmental conditions.
A frequency-dependent evolutionarily stable state (ESSt) assumes that migratory behavior is fixed, and residents are favored when migrants are at a high frequency and vice versa. At some specific equilibrium frequency, the migratory and non-migratory alternatives should have the same average pay-off; that is, if one alternative increases in frequency, its pay-off should decrease (i.e., fitness is negatively frequency-dependent; Swingland, 1983; Dingle, 2014a). The evolution of partial migration has been examined using frequency-dependent ESS modeling especially in birds (Lundberg, 1987; Kaitala et al., 1993; Kokko, 2011). However, empirical support for frequency-dependent ESSts in most species is lacking (Chapman et al., 2011b; Lundberg, 2013), perhaps because negative frequency-dependence may be observable only when the population is at or above the carrying capacity.
In partially migratory ungulates, many authors assume that migration is a fixed trait (Hebblewhite and Merrill, 2011; Gaillard, 2013; Middleton et al., 2013b). Fixed migration would necessarily mean that the ratio of migrants and residents in a population would need to be balanced by density- or frequency-dependence in a mixed-ESS at the population level (Lundberg, 1988; Kaitala et al., 1993), as described above. That is, individuals are not able to change their behavior, but the relative demographic success of each separate tactic determines the relative fitness of each behavior, which then changes in some stabilizing way as densities or frequencies change. Without such a stabilizing mechanism, a population would be expected to reach fixation for a single behavior. The rarity of “pure” migrant or resident populations itself rejects this notion. Further, partial migration through an ESSt could not happen if there is switching between tactics, which has been reported in deer (Odocoileus virginianus, Nelson, 1995), elk (Cervus elaphus, Eggeman et al., 2016), impala (Aepyceros melampus, Gaidet and Lecomte, 2013), moose (Alces alces, White et al., 2014), pronghorn (Antilocapra americana, White et al., 2007), Sierra Nevada bighorn sheep (Ovis canadensis sierrae, Spitz, 2015), and Svalbard reindeer (Rangifer tarandus platyrhynchus, Hansen et al., 2010; Meland, 2014; Table 3). In these studies, the average annual rate of switching was ~20%, although most studies had limited ability to detect switching due to inadequate sample size or infrequent monitoring over the course of entire lifetimes. If the results of these few switching studies are representative of the many long-lived ungulates with lifespans >10 years, the evidence suggests that individuals may switch tactics several times during their lifetime.
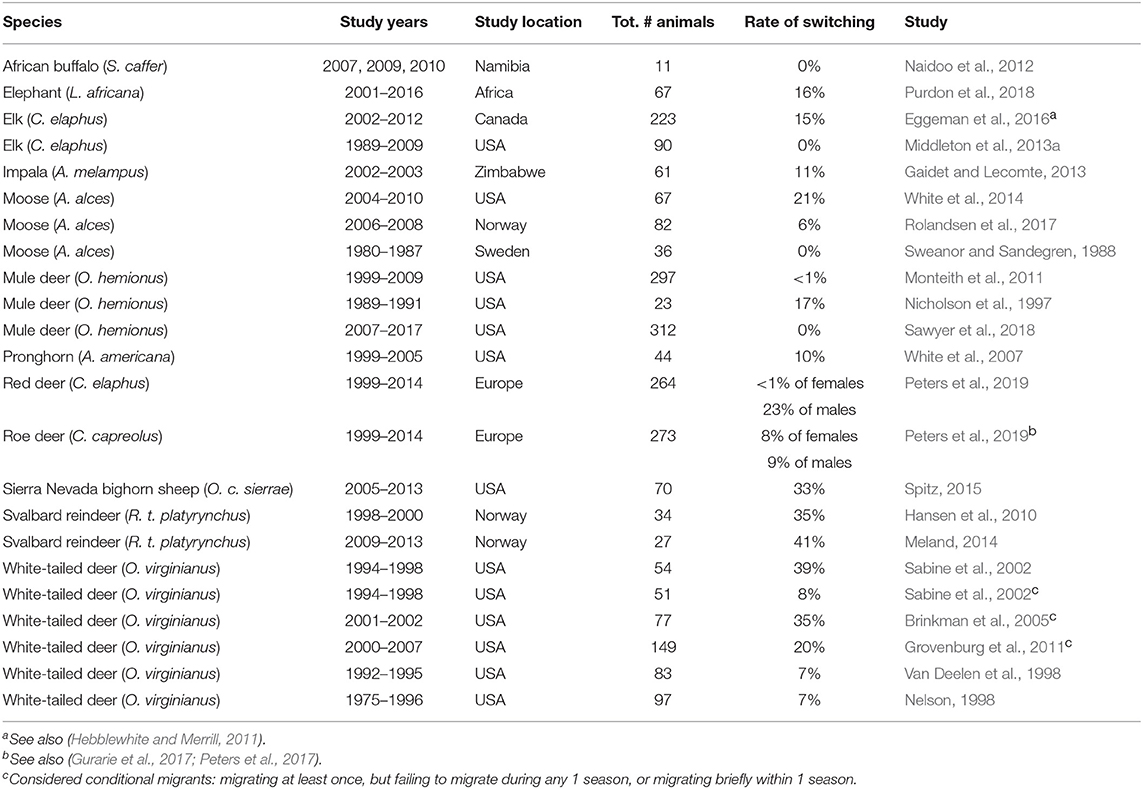
Table 3. Reported rates of switching between migratory tactics in partially migratory populations of large ungulates.
In contrast, the environmental-genetic threshold describes a mechanism in which a number of additive, environmental variables may interact with a number of genes to contribute toward expression of an underlying phenotypic, behavioral liability (i.e., migratory tendency) or trait that is normally distributed within a population (Figure 1, Pulido, 2011). According to the environmental-genetic threshold model, individuals have a genetically determined propensity for migration that is triggered, or not, by environmental conditions. A threshold exists below which individuals are sedentary, whereas those above the threshold are migratory (Berthold, 1991; Pulido et al., 1996). Migratory traits may not be fixed, even under strong, directional selection, because as the distribution of migratory propensity shifts below the threshold, migratory traits will not be phenotypically expressed (Pulido, 2011). Environmental variables such as food, social dominance, or body condition may affect individuals with liability values close to the threshold, causing them to change migratory tactic. This conceptual model has not been used to address partial migration in ungulates, and testing its predictions would require long-term studies once the genetic basis or a correlate for migration propensity was identified. Even if further work identifies genetically controlled, regulatory pathways of complex traits linked to migration, monitoring the interaction of these traits with environmental conditions over a sufficiently long period in free-ranging ungulates remains a formidable challenge (Pulido, 2011).
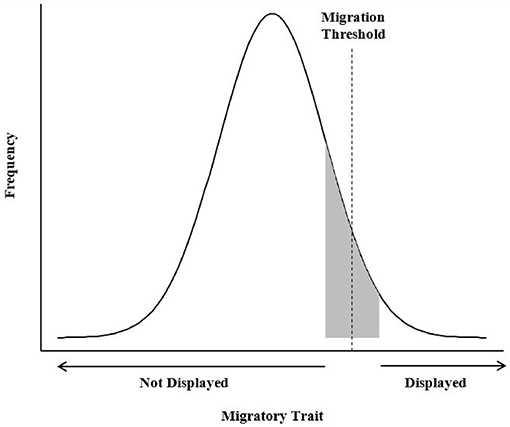
Figure 1. The environmental-genetic threshold model assumes that a dichotomous trait is displayed as a result of an underlying continuous character or liability (i.e., migratory tendency) that is normally distributed within a population (Pulido, 2011). The combined effects of genetic influences and environmental effects on the threshold position can push a facultative migrant (represented in gray), with a liability close to the threshold, to either migrate or remain resident, depending on the direction of the environmental shift.
The alternative to genetically fixed traits or liabilities is the possibility that migration varies between individuals as a function of state, such as age, nutritional condition, or other circumstances. As we discuss below, state-dependent migration may be relatively fixed intrinsically (e.g., dependent on an individual's age or sex or personality), or highly plastic based on nutritional state (e.g., fat reserves or other physiological mechanisms of the metabolic, immune, or endocrine systems) or extrinsic conditions (e.g., predation risk, parasite loads, or climate). If fitness varies temporally with environmental conditions (Rolandsen et al., 2017), then fitness balancing is not necessary over the short term. In this case, a single condition-dependent strategy could produce 2 (or more) tactics. Each individual should adopt the migratory tactic that is best for it at the time (Swingland, 1983), in some cases, making the “best of a bad job” (Lundberg, 1987) and resulting in relative pay-offs that may not be equal across individuals. For example, dominant or more competitive individuals may optimize fitness by remaining resident, whereas less competitive or sub-dominant individuals may optimize fitness by trading the cost of migration in return for a habitat where there is less competition (Swingland, 1983; Lundberg, 1987; Chapman et al., 2011b).
Consequently, both migratory and non-migratory tactics may be maintained within a population due to differential density-dependent regulation of vital rates that must counteract each other over the long term, such that any differences in reproductive success between migrants and residents must be countered by differences in survival (Figure 2). Hebblewhite and Merrill (2011) found that despite higher pregnancy rates and winter calf weights, migratory elk were more at risk during migration. In contrast, residents reduced predation risk by remaining in areas of human activity, which resulted in lower pregnancy and calf weights, but slightly higher adult and calf survival. Similarly, White et al. (2014) also found that calf survival was higher in migratory moose, but that there was no difference in body fat accumulation between residents and migrants. Both studies were suggestive of demographic balancing between the two tactics (Hebblewhite and Merrill, 2011; White et al., 2014). Peters et al. (2019) suggested that the probability of migrating should increase under high-density conditions; with increasing density, density-dependent or environmentally-driven switching between tactics would maintain partial migration within a population. Indeed, recent evidence from elk supports the notion of density-dependent migration being a potentially stabilizing mechanism regulating partial migration in populations (Eggeman et al., 2016). On the other hand, stochastic environmental events could cause mortality for the more successful tactic, independent of density, but if the increase in mortality is only to the level of survival of the alternative behavior, partial migration can be maintained (Grayson et al., 2011). The balance between these conflicting costs and benefits leads to individuals remaining in a range year-round, or moving to new areas. In the next section, we identify and assess the support for the most commonly hypothesized mechanisms shaping individual variation in migratory tendency in ungulates.
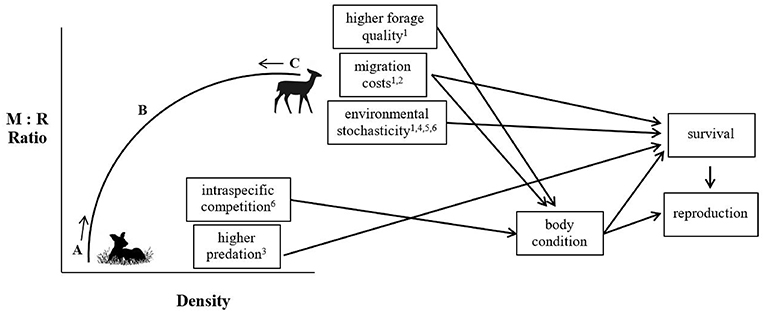
Figure 2. Conceptual framework depicting how the density-dependent and density-independent factors may interact on the vital rates of migrants and residents to maintain partial migration within a population. Migrants (M) may do better in one vital rate at high density, but residents (R) will do better in another vital rate at low density. This “demographic balancing” may not happen every year, but will prevent fixation of the proportion of migrants at either 0 or 1, and provide the mechanism for the long-term average ratio of M:R in a population. (A) With each additional R individual, individual fitness for each R individual declines, and density dependence in predation or competition, or alternatively, stochastic climate events, shift the M:R ratio back toward (B), the point at which partial migration is maintained. (C) With each additional M individual, fitness for each M individual declines. However, very little empirical evidence to support demographic balancing is found in the partially migratory ungulate literature because most studies are not long-term, or examine only 1 or 2 vital rates. For examples, see (1) Nicholson et al. (1997) (2) Hebblewhite and Merrill (2011) (3) White et al. (2014) (4) Middleton et al. (2013a) (5) Fieberg et al. (2008) (6) Plumb et al. (2009).
Why Do Some Individuals Migrate?
In this section, we summarize results from a range of field studies focused on ungulate migration to address what factors promote migration in an individual animal. We summarize evidence for a genetic basis to migration, evidence for the role of learning and cultural transmission, and factors related to individual state or environmental conditions and/or their interactions (Tables 4, 5).
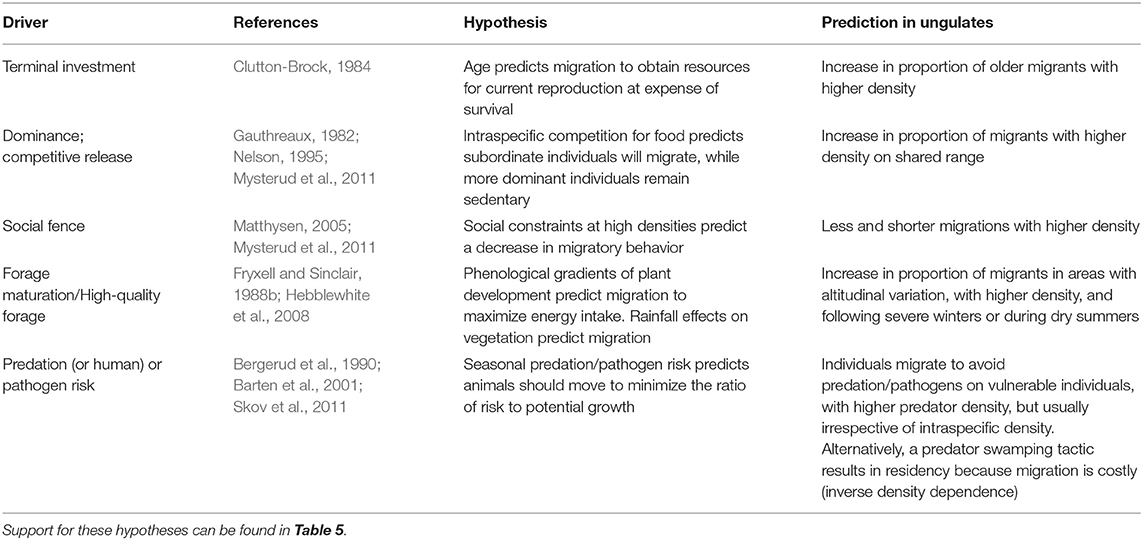
Table 4. State- or condition-dependent hypotheses to explain individual variation in migratory tendency within partially migratory ungulate populations.
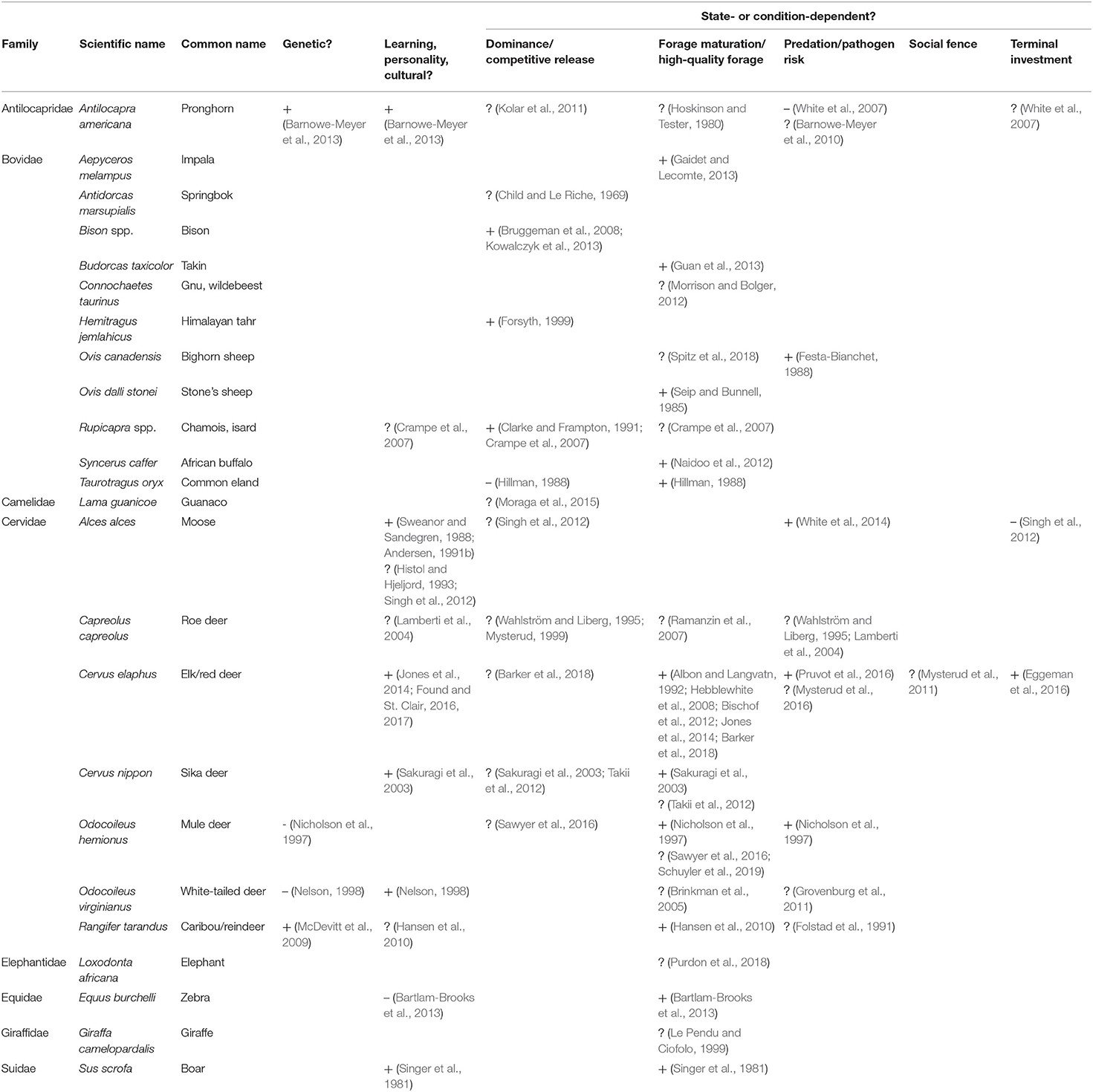
Table 5. Support (+ positive/likely, ? potentially but untested/suppositional, – negative/evidence against) for mechanisms explaining individual variation in migratory tendency within partially migratory ungulate populations, including genetics, learning, personality or cultural transmission, and state- or condition-dependence.
Genetics
Evidence for a direct genetic basis for migration would require that behavioral traits of individuals were linked to specific alleles that differentiated groups or showed heritability, as demonstrated for migratory restlessness in captive birds (Berthold and Querner, 1982; Terrill, 1987; Berthold, 1991; Berthold and Pulido, 1994). Such experiments showing restless behavior related to migration have not been attempted and may not be feasible in ungulates, which are harder to hold in captivity and often express less spatial and temporal synchrony in their migration. Nonetheless, some authors have attempted more correlative approaches for exploring indirect genetic effects by using genetic surveys to distinguish individuals with different migratory tendencies. For example, authors used microsatellites to identify genetic differentiation between GPS-collared pronghorn antelope defined as migrants vs. residents in the Yellowstone Ecosystem (Barnowe-Meyer et al., 2013). Similar uses of microsatellites have revealed genetic structure in ungulates (e.g., Coltman et al., 2003; Colson et al., 2016), but inferences from microsatellite differentiation based on a few multi-loci (typically <20) were generally limited (Table 5). This scanning approach might better distinguish behavioral differences among individuals with new genomics approaches, such as amplified fragment length polymorphism (AFLP) markers in whole genome scans (Liedvogel et al., 2011).
A second approach for identifying genes associated with migration could attempt to isolate aspects of mitochondrial genotypes. For example, the probability of being migratory in a hybrid swarm of caribou (R. tarandus) in the Canadian Rockies was higher in individuals carrying a Beringian–Eurasian haplotype, which was mainly associated with the migratory, barren-ground subspecies, compared to the typically non-migratory woodland caribou (McDevitt et al., 2009). Interestingly, these animals could not be distinguished with microsatellite data, perhaps owing to interbreeding between diverged lineages since the last glaciation (McDevitt et al., 2009). The promise of an mtDNA approach was amplified by the correlation reported by Northrup et al. (2014) between the timing of migration in mule deer (O. hemionus) from 4 distinct winter ranges in the Piceance Basin of Colorado. These authors attributed the correlation to differences in mitochondrial efficiency associated with metabolic demands of migration.
Two other classic approaches for identifying the genetic basis of any behavior would be to compare parent-offspring pairs in long-term studies with known individuals (e.g., Gaillard, 2013) or to conduct cross-fostering experiments. To our knowledge, no authors have applied either technique to address migration in ungulates. Perhaps measuring gene expression in a species with fixed migrants, fixed residents, and individuals that switch migratory tactics within their lifetime could shed some light. A further challenge would be to consider alternative explanations for genetic correlations. For example, in the case of timing of migration in mule deer, Northrup et al. (2014) were able to reject a causative effect of sociality by controlling for the source of the individual's winter range, which showed little spatial clustering of haplotypes. Clearly, it would be challenging to disentangle alternative explanations such as fat levels or physiological status and social or cultural factors, which we discuss next, in correlative studies to support a genetic component for migration. In many cases, particularly in species for which animals switch migratory tactics within their lifetime, it is likely that genetic tendencies are moderated by environmental circumstances.
Learning, Culture, and Personality
Being able to discriminate genetic mechanisms from learning and cultural transmission is difficult but could be possible via studying mother-offspring pairs for long periods. Within and beyond these pairs, it is likely that information about navigation and migratory routes are passed from more experienced, key individuals to those that are less experienced (Dodson, 1988; Couzin et al., 2005; Fagan et al., 2012). Nelson (1998) reported that white-tailed deer fawns mimicked the migratory behavior of their mothers. Particularly in the first year of life, residency or migration can be assumed to be dependent on the migratory status of the parent because of the offspring-parent bond (Andersen, 1991b). However, we found few studies that addressed the potential effects of early learning or cultural inheritance on migration beyond the first year in ungulate populations (Sweanor and Sandegren, 1988; Nelson, 1998), although it has been documented in whales (Valenzuela et al., 2009). Translocated bighorn sheep and moose learned to increase knowledge and exploit green waves of forage growth in new environments where they had no previous knowledge of the landscape; as knowledge increased, so did the propensity to migrate (Jesmer et al., 2018).
Social learning that promotes migration does not need to be heritable to evolve (Boyce, 1991), although the ability to learn and mimic migratory behavior is likely partially hereditary (Nelson, 1998). Indeed, behavioral flexibility itself appears to be highly heritable (Laughlin et al., 2011) and might be especially important for partial migration. In the Canadian Rockies, resident elk exhibited bolder personalities that included greater exploration of novel objects relative to migratory individuals (Found and St. Clair, 2016). Bolder elk also exhibited lesser lateralization of hoof preferences when pawing the snow to forage, which potentially signals greater cerebral flexibility (Found and St. Clair, 2017). The same authors suggest that less lateralized animals had genetically determined temperaments that made them more responsive to environmental stimuli, which resulted in greater likelihood of them realizing the increasing benefits of residency and abandoning previous histories of migration (Found and Clair, in review). Similar metrics for studying personality traits in wild animals have proliferated in recent years (reviewed by Dingemanse et al., 2010 and Sih et al., 2012), creating much potential to explore their correlations with both migratory tactics and genotypic variation.
State and Physiological Condition
In reviewing potential intrinsic factors promoting migration, we found studies primarily addressed one specific hypothesis related to age, and very few studies directly tied physiological mechanisms to partial migration. The Terminal Investment Hypothesis states that older (past their prime) individuals are more likely to devote more resources toward ensuring successful reproduction than younger (yearling or prime-aged) individuals because they anticipate fewer future reproductive events (Clutton-Brock et al., 1982; Clutton-Brock, 1984; Bercovitch et al., 2009). When applied to migration, this hypothesis predicts that ungulates might have a propensity to remain resident while young so as to prioritize their own survival by avoiding risks that can occur during migration (e.g., from predation or anthropogenic factors; Nicholson et al., 1997; Hebblewhite and Merrill, 2011; Schuyler et al., 2019); as they age, they might accept greater risks to migrate to increase access to resources for investment in their terminal offspring (Fryxell and Sinclair, 1988a; Albon and Langvatn, 1992). The age at which this hypothesis might occur in ungulates could be quite old; indeed, Eggeman et al. (2016) showed potential evidence that elk became more likely to migrate with age in Alberta, Canada, but migrants rarely switched to a resident tactic after aging (>15 years old). However, the opposite pattern appears to occur in both pronghorn antelope, which became non-migratory as they aged (White et al., 2007), and moose, which migrated when young but were less likely to migrate as they aged (Singh et al., 2012). Evidence to support predictions of the terminal investment hypothesis could be confounded with other factors. For example, increasing costs of movement are associated with age-related changes in physiological condition (Ericsson and Wallin, 2001), and home ranges may become smaller with age due to experience gained (Allen et al., 2016).
Migration may also be state-dependent (Visscher and Merrill, 2018). If individuals were able to meet their nutritional demands satisfactorily without migrating, there may be no need to migrate if an individual were to incur additional costs or stress related to movement, predation risk, or social conflict (but see below). Because ungulate survival and reproductive efforts are closely tied to body fat reserves (Cook et al., 2004, 2016; Monteith et al., 2014), we would expect to see the propensity to migrate closely linked to nutritional state if condition buffers consequences (Spitz, 2015). Other physiological mechanisms differing between migratory tactics might include metabolic, immune, and endocrine systems, or oxidative stress associated with intense physical activity or fatigue (Jachowski and Singh, 2015; Hegemann et al., 2019). Although Jachowski et al. (2018) found individual mule deer occupying areas closer to peak forage quality during migration had decreased levels of fecal glucocorticoid metabolites, to our knowledge, there have been no studies comparing these mechanisms as related to partial migration in ungulates. Experiments would most likely be needed to identify the specific physiological mechanism, but even then, these could differ among species and environments (Hegemann et al., 2019). Further, recent evidence shows that transfer of the nutritional benefits that are normally associated with migration to residents, as can occur when irrigated agriculture supplements elk feeding, can promote resident behavior (Jones et al., 2014; Barker, 2018). In fact, reproducing and migrating every other year (Morrison and Bolger, 2012) may be a better tactic for ensuring survival and lifetime reproduction, and decisions surrounding migration in ungulates might be driven almost primarily by nutrition and reproductive status (e.g., Festa-Bianchet, 1988, described below).
Competition, Forage, Predation, and Pathogens
Competition may promote migration but how and where competition influences the tendency for an individual to migrate may vary. The Dominance or Competitive Release Hypothesis (Ketterson and Nolan, 1976; Fudickar et al., 2013) is based on intraspecific competition, with an individual's propensity to migrate expected to increase at higher density on sympatric range. Although competition for food on high-density, sympatric range is likely, it is difficult to demonstrate directly, but might be inferred. For example, white-tailed deer have shown flexible migratory behavior in which they do not remain after fall arrival on sympatric winter ranges, and instead move back to summer ranges during years of little snow and mild weather, suggesting avoidance of competition on the less nutritional winter ranges (Nelson, 1995). Similarly, the distance migrated by elk and red deer in summer has been shown to increase with density, suggesting avoidance of competition on seasonal ranges (Mysterud et al., 2011; Eggeman et al., 2016). Sawyer et al. (2016) also showed that long-distance migrants spent more time migrating and may have initiated spring migration 3 weeks earlier than moderate- or short-distance migrants to escape intraspecific competition by lessening time spent on winter range. In contrast to competition that occurs on sympatric winter ranges, if population densities also increase on allopatric summer ranges, leading to occupation of all the summer areas, migration tendency can be restricted due to competition and social aggression according to the Social Fence Hypothesis (Matthysen, 2005). For example, Mysterud et al. (2011) reported that a lower proportion of red deer migrated at high density during summer, consistent with this hypothesis. However, the authors only contrasted areas of differing densities and did not measure variation in habitat quality, which is needed to determine the level of competition (Fretwell and Lucas, 1969). Because fall migration was delayed at high density, Mysterud et al. (2011) further suggested that a combination of the competitive release and social fence hypotheses were needed to explain migratory tendency in ungulates. Constraints on distribution and changes in sociality and aggressive behaviors of individuals would need to be documented on both sympatric and allopatric ranges as ungulate densities increased to support these hypotheses.
In seasonal environments, the Forage Maturation Hypothesis predicts that spatiotemporally varying resources promote migration to maximize nutrient intake where there are phenological gradients of plant development (Fryxell and Sinclair, 1988a; Fryxell et al., 1988; Albon and Langvatn, 1992; Hebblewhite et al., 2008). Whereas the classic example may be the Serengeti wildebeest following new green growth to the plains during the wet season (Holdo et al., 2009), many cervids in temperate systems show migrations tied to elevational gradients in plant green up (Sawyer and Kauffman, 2011; Bischof et al., 2012; Merkle et al., 2016; Aikens et al., 2017). If migrants “surf” or “jump” an altitudinal green wave, they are predicted to enter winter with heavier masses and in better body condition than residents as a consequence of higher-quality forage (Albon and Langvatn, 1992; Hebblewhite et al., 2008). Only a handful of studies focused on partially migratory ungulates have demonstrated that females or their young were fatter when they were migratory (e.g., Mysterud et al., 2001; Hebblewhite et al., 2008; Hebblewhite and Merrill, 2011). Yet, this conclusion for elk in the Greater Yellowstone Ecosystem was driven largely by non-lactating females with no data on calf survival and whether release from nutritional costs associated with calf loss contributed to their better condition; in addition, an influence of surfing on autumn fat levels was not detected for lactating elk so results remained somewhat inconclusive (Middleton et al., 2018). Even fewer efforts have linked the tactic of migration to life-time reproductive success. Such studies would require not only long-term studies but additionally evaluating other costs or benefits of migration.
The major hypothesis posed as an alternative to ungulate migration as a response to forage maturation is the Predation Risk Hypothesis, which states that ungulates migrate to escape or minimize predation or other risk factors, such as human hunting or parasites (Fryxell and Sinclair, 1988a; Bergerud et al., 1990; Hebblewhite and Merrill, 2007). Evidence we found to support this hypothesis focused on ungulates moving outside of predator ranges and denning territories (Bergerud, 1988) or by using terrain where predators travel less frequently (Bergerud and Page, 1987). For example, pregnant bighorn sheep in Alberta moved from relatively higher-quality forage to rugged high-elevation summer range earlier than non-pregnant ewes and before plant growth started, which Festa-Bianchet (1988) argued was to avoid predation on vulnerable newborn lambs. On the coast of Alaska, migrant moose showed almost 3 times higher neonatal calf survival by migrating to avoid predation but did not obtain nutritional benefits through accumulation of body fat (White et al., 2014). Recent theoretical work suggests that parasites and pathogens could be drivers of partial migration, either as escape from infected areas or individuals, through loss of infected individuals during migration, or as recovery from infection when parasites cannot adjust to environmental changes that occur during migration (Altizer et al., 2011; Fritzsche McKay and Hoye, 2016; Shaw and Binning, 2016). In support of these mechanisms, Pruvot et al. (2016) showed that migratory elk herds in Canada were potentially less likely to be infected with giant liver flukes (Facioloides magna) when compared with resident elk, and lower intensities of warble fly larvae (Hypoderma tarandi) were found in reindeer the farther they migrated post-calving (Folstad et al., 1991).
While comparing the costs and benefits of migratory tactics represents an important first step to understanding what promotes the tendency to migrate, explaining migration by only 2 hypotheses (predation risk avoidance vs. forage maturation, which tend to be the focus of many studies) limits the possibility that other intrinsic or extrinsic factors could also be influential as we've described above. However, results do demonstrate that there may be no straightforward, easy answer because the top-down benefits of avoiding risk through migration may be complicated by life history trade-offs (the cost of rearing offspring to subsequent fecundity), or which may be at times compete with, or modulate, the bottom-up effects of increased access to forage.
Conclusions and Future Directions
We have shown that flexibility in migratory behavior by ungulates is more common than previously appreciated, amplifying the suggestion by others that migration should evolve under widely varying environmental conditions in response to the advantages and disadvantages of different life-history strategies (Holt and Fryxell, 2011; Fryxell and Holt, 2013; Avgar et al., 2014). Migration is a complex phenomenon (Alerstam et al., 2003) determined by a number of traits, in turn affected by several genes with pleiotropic effects (Sutherland, 1998). We conclude that migration is not determined by a direct mapping of genotype to phenotype, making it a flexible tactic adopted within a broader strategy. Establishing that partial migration is common in ungulates, and that it appears to respond to diverse genetic, environmental, and demographic correlates, increases the range of techniques that might be applied to study it. Achieving these advances will require use of clear, universal definitions (Avgar et al., 2014; Cagnacci et al., 2016) and classification methods (e.g., Bunnefeld et al., 2011; Naidoo et al., 2012). In fact, the longer individual white-tailed deer were monitored, the more likely they were to be classified by researchers as conditional migrants as opposed to fixed migrants or residents (Fieberg et al., 2008).
Limitations of past studies of migration might be overcome with an understanding that migration is often flexible. Very few of the studies we found were set up to examine how density could lead to a long-term demographic balancing of migrants and residents within a population, but viewing migration as a conditional tactic in a broader strategy to maximize forage intake increases the range of experimental studies that might be applied to this problem. For example, related hypotheses might be tested by manipulating forage or ungulate access to it in protected areas (e.g., Most et al., 2015) or in managed herds (Rivrud et al., 2016). Similarly, few studies have tested explicitly for a genetic basis for differences in migratory and resident individuals within partially migratory populations. The few studies that mentioned learning or cultural inheritance (Singer et al., 1981; Sweanor and Sandegren, 1988; Andersen, 1991a; Barnowe-Meyer et al., 2013) did not conduct them with detailed behavioral observations or controlled experiments to test related hypotheses. A broader view of the genetic and environmental correlates of migratory tactics increases the relevance of many associated metrics.
The decision to migrate or not is made by individuals, but rarely do studies examine individual decision-making in migratory populations (Ball et al., 2001). Nonetheless, some authors attempt such an approach, as with the characterization of multiannual movement patterns by more than 300 moose in 10 different populations (Allen et al., 2016). But many authors whose studies we reviewed characterized migration dichotomously at the level of single populations. Emerging is the view that migration may be a continuum (Ball et al., 2001), both as a behavior (e.g., individuals may exhibit intermediate tactics or variability in timing and distance) and as a population metric (i.e., 1 to 99% of the population may be migratory). Based on our review, migration as a continuum means the reasons for migration were often hard to detect and characterize (Cagnacci et al., 2016). In particular, instances in behavioral switching between migratory tactics should be explored for their potential intrinsic and extrinsic correlates.
Unfortunately, it is difficult to link multiple, interacting intrinsic and extrinsic variables to the occurrence of migration when there is strong environmental variation (Fieberg et al., 2008). In contrast to fixed migrants in other species that show predictable movements as a result of physiological processes (neuroendocrine and endocrine systems), linking environmental cues (day length, photoperiod) to the mechanisms controlling facultative migration in highly variable environments is challenging (Ramenofsky et al., 2012). We found speculative support for state- or condition-dependent migration in ungulates in our review, but relatively little experimental data, despite several indirect lines of evidence. We know that differences in habitat quality can lead to corresponding differences in physiology, nutritional condition, and reproductive success in ungulates, and that these can be modified by density (Weber et al., 1984; Becker et al., 2010). More studies are needed that relate habitat use to resulting nutritional acquisition, and measures of body condition and reproductive success, to identify the fitness consequences of migratory tactics. Given new advances in remote monitoring of physiological traits in free-ranging animals, studies on not only how body fat at time of capture, but also physiological mechanisms, differ between migrants and residents and contribute to switching between tactics are warranted (Hegemann et al., 2019). Further, studies that track migratory traits of mothers and their offspring could separate the genetic and learned components of migratory behavior from environmental effects.
Current knowledge of partial migration in ungulates is sometimes limited by their large size, long lives, and wide-ranging use of habitats, but these traits also confer advantages of observability, long-term study, and generalization across spatial scales. These advantages will be further amplified by using methodologies that are increasingly cost-effective and tractable over the long term in space and time, and in remote environments, to test the relative fitness-related consequences of partially migratory behavior (Bolger et al., 2008; Gaillard, 2013). Long-term, demographic studies and population models tracking the life-history traits of co-existing individuals along the resident-migrant gradient through the year will allow for calculating the costs and benefits of their migration patterns (Bolger et al., 2008). Given the potential ecological and evolutionary significance of partial migration, and that ever-increasing anthropogenic disturbance and environmental change may alter or eliminate the benefits of migration altogether (Bischof et al., 2012), understanding the genetic, environmental, and density-driven trade-offs underlying partial migration is of the utmost importance.
Author Contributions
JB designed and wrote the first draft of the manuscript. All authors contributed to manuscript revision and read and approved the submitted version.
Funding
This work would not have been possible without the financial and in-kind support from: Alberta Environment and Parks; Parks Canada; Natural Sciences and Engineering Research Council; University of Alberta; University of Montana; Alberta Conservation Association; Minister's Special License—Hunting for Tomorrow and Alberta Fish & Game Association; Rocky Mountain Elk Foundation; Safari Club International Foundation; Safari Club—Northern Alberta Chapter; NASA (USA, NNX11A047G to MH); National Science Foundation (USA) Long-term Research in Environmental Biology grant (LTREB) to MH and EM (1556248); and the Nestor and Sue Cebuliak, Bill Samuel, and William Wishart Graduate Awards.
Conflict of Interest Statement
The authors declare that the research was conducted in the absence of any commercial or financial relationships that could be construed as a potential conflict of interest.
Acknowledgments
We thank Tal Avgar, Andrew Derocher, and Kevin Monteith for comments on an earlier version of the manuscript.
Supplementary Material
The Supplementary Material for this article can be found online at: https://www.frontiersin.org/articles/10.3389/fevo.2019.00325/full#supplementary-material
References
Abrahms, B., Seidel, D. P., Dougherty, E., Hazen, E. L., Bograd, S. J., Wilson, A. M., et al. (2017). Suite of simple metrics reveals common movement syndromes across vertebrate taxa. Mov. Ecol. 5:12. doi: 10.1186/s40462-017-0104-2
Aikens, E. O., Kauffman, M. J., Merkle, J. A., Dwinnell, S. P. H., Fralick, G. L., and Monteith, K. L. (2017). The greenscape shapes surfing of resource waves in a large migratory herbivore. Ecol. Lett. 20, 741–750. doi: 10.1111/ele.12772
Albon, S. D., and Langvatn, R. (1992). Plant phenology and the benefits of migration in a temperate ungulate. Oikos 65, 502–513. doi: 10.2307/3545568
Alerstam, T., Hedenström, A., and Åkesson, S. (2003). Long-distance migration: evolution and determinants. Oikos 103, 247–260. doi: 10.1034/j.1600-0706.2003.12559.x
Allen, A. M., Månsson, J., Sand, H., Malmsten, J., Ericsson, G., and Singh, N. J. (2016). Scaling up movements: from individual space use to population patterns. Ecosphere 7:e01524. doi: 10.1002/ecs2.1524
Altizer, S., Bartel, R., and Han, B. A. (2011). Animal migration and infectious disease risk. Science 331, 296–302. doi: 10.1126/science.1194694
Andersen, R. (1991a). Habitat changes in moose ranges: effects on migratory behavior, site fidelity and size of summer home-range. Alces 27, 85–92.
Andersen, R. (1991b). Habitat deterioration and the migratory behaviour of moose (Alces alces L.) in Norway. J. Appl. Ecol. 28, 102–108. doi: 10.2307/2404117
Avgar, T., Street, G., and Fryxell, J. M. (2014). On the adaptive benefits of mammal migration. Can. J. Zool. 92, 481–490. doi: 10.1139/cjz-2013-0076
Ball, J. P., Nordengren, C., and Wallin, K. (2001). Partial migration by large ungulates: characteristics of seasonal moose Alces alces ranges in northern Sweden. Wildl. Biol. 7, 39–47. doi: 10.2981/wlb.2001.007
Barker, K. J. (2018). Home is where the food is: Causes and consequences of partial migration in elk (MSc thesis). University of Montana, Missoula, MT, USA.
Barker, K. J., Mitchell, M. S., Proffitt, K. M., and Devoe, J. D. (2018). Land management alters traditional nutritional benefits of migration for elk. J. Wildl. Manage. 83, 167–174. doi: 10.1002/jwmg.21564
Barnowe-Meyer, K. K., White, P. J., Davis, T. L., Smith, D. W., Crabtree, R. L., and Byers, J. A. (2010). Influences of wolves and high-elevation dispersion on reproductive success of pronghorn (Antilocapra americana). J. Mammal. 91, 712–721. doi: 10.1644/09-MAMM-A-057.1
Barnowe-Meyer, K. K., White, P. J., Waits, L. P., and Byers, J. A. (2013). Social and genetic structure associated with migration in pronghorn. Biol. Conserv. 168, 108–115. doi: 10.1016/j.biocon.2013.09.022
Barten, N. L., Bowyer, R. T., and Jenkins, K. J. (2001). Habitat use by female caribou: tradeoffs associated with parturition. J. Wildl. Manage. 65, 77–92. doi: 10.2307/3803279
Bartlam-Brooks, H. L. A., Beck, P. S. A., Bohrer, G., and Harris, S. (2013). In search of greener pastures: using satellite images to predict the effects of environmental change on zebra migration. J. Geophys. Res. Biogeosci. 118, 1427–1437. doi: 10.1002/jgrg.20096
Becker, S. A., Kauffman, M. J., and Anderson, S. H. (2010). Nutritional condition of adult female Shiras moose in northwest Wyoming. Alces 46, 151–166. Retrieved from: https://www.alcesjournal.org/alces/index.php/alces/article/view/65.
Bennitt, E., Bonyongo, M. C., and Harris, S. (2016). Effects of divergent migratory strategies on access to resources for Cape buffalo (Syncerus caffer caffer). J. Mammal. 97, 1682–1698. doi: 10.1093/jmammal/gyw134
Bercovitch, F. B., Loomis, C. P., and Rieches, R. G. (2009). Age-specific changes in reproductive effort and terminal investment in female Nile lechwe. J. Mammal. 90, 40–46. doi: 10.1644/08-MAMM-A-124.1
Berger, J. (2004). The last mile: how to sustain long-distance migration in mammals. Conserv. Biol. 18, 320–331. doi: 10.1111/j.1523-1739.2004.00548.x
Bergerud, A. T. (1988). Caribou, wolves and man. Trends Ecol. Evol. 3, 68–72. doi: 10.1016/0169-5347(88)90019-5
Bergerud, A. T., Ferguson, R., Butler, S. E., and Butler, H. E. (1990). Spring migration and dispersion of woodland caribou at calving. Anim. Behav. 39, 360–368. doi: 10.1016/S0003-3472(05)80882-6
Bergerud, A. T., and Page, R. E. (1987). Displacement and dispersion of parturient caribou at calving as antipredator tactics. Can. J. Zool. 65, 1597–1606. doi: 10.1139/z87-249
Bergman, C. M., Schaefer, J. A., and Luttich, S. N. (2000). Caribou movement as a correlated random walk. Oecologia 123, 364–374. doi: 10.1007/s004420051023
Berthold, P. (1991). Genetic control of migratory behaviour in birds. Trends Ecol. Evol. 6, 254–257. doi: 10.1016/0169-5347(91)90072-6
Berthold, P., and Pulido, F. (1994). Heritability of migratory activity in a natural bird population. Proc. R. Soc. B Biol. Sci. 257, 311–315. doi: 10.1098/rspb.1994.0131
Berthold, P., and Querner, U. (1982). Partial migration in birds: experimental proof of polymorphism as a controlling system. Experimentia 38, 20–21. doi: 10.1007/BF01972282
Bischof, R., Loe, L. E., Meisingset, E. L., Zimmermann, B., Van Moorter, B., and Mysterud, A. (2012). A migratory northern ungulate in the pursuit of spring: jumping or surfing the green wave? Am. Nat. 180, 407–424. doi: 10.1086/667590
Bolger, D. T., Newmark, W. D., Morrison, T. A., and Doak, D. F. (2008). The need for integrative approaches to understand and conserve migratory ungulates. Ecol. Lett. 11, 63–77. doi: 10.1111/j.1461-0248.2007.01109.x
Börger, L., Matthiopoulos, J., Holdo, R. M., Morales, J. M., Couzin, I. D., and McCauley, E. (2011). “Migration quantified: constructing models and linking them with data,” in Animal Migration: A Synthesis, eds E. J. Milner-Gulland, J. M. Fryxell, and A. R. E. Sinclair (Oxford: Oxford University Press, 111–128.
Boyce, M. S. (1991). Migratory behavior and management of elk (Cervus elaphus). Appl. Anim. Behav. Sci. 29, 239–250. doi: 10.1016/0168-1591(91)90251-R
Brinkman, T. J., Deperno, C. S., Jenks, J. A., Haroldson, B. S., and Osborn, R. G. (2005). Movement of female white-tailed deer: effects of climate and intensive row-crop agriculture. J. Wildl. Manage. 69, 1099–1111. doi: 10.2193/0022-541X(2005)069[1099:MOFWDE]2.0.CO;2
Bruggeman, J. E., White, P. J., Garrott, R. A., and Watson, F. G. R. (2008). “Partial migration in central Yellowstone bison,” in The Ecology of Large Mammals in Central Yellowstone: Sixteen Years of Integrated Field Studies, eds R. A. Garrott, P. J. White, and F. G. R. Watson (San Diego, CA: Academic Press), 217–235.
Buchin, M., Kruckenberg, H., and Kolzsch, A. (2013). “Segmenting trajectories by movement states,” in Advances in Spatial Data Handling: Geospatial Dynamics, Geosimulation and Exploratory Visualization, eds S. Timpf and P. Laube (Heidelberg: Springer-Verlag Berlin Heidelberg, 15–25.
Bunnefeld, N., Börger, L., van Moorter, B., Rolandsen, C. M., Dettki, H., Solberg, E. J., et al. (2011). A model-driven approach to quantify migration patterns: individual, regional and yearly differences. J. Anim. Ecol. 80, 466–476. doi: 10.1111/j.1365-2656.2010.01776.x
Cagnacci, F., Focardi, S., Ghisla, A., van Moorter, B., Merrill, E. H., Gurarie, E., et al. (2016). How many routes lead to migration? Comparison of methods to assess and characterize migratory movements. J. Anim. Ecol. 85, 54–68. doi: 10.1111/1365-2656.12449
Cagnacci, F., Focardi, S., Heurich, M., Stache, A., Hewison, A. J. M., Morellet, N., et al. (2011). Partial migration in roe deer: migratory and resident tactics are end points of a behavioural gradient determined by ecological factors. Oikos 120, 1790–1802. doi: 10.1111/j.1600-0706.2011.19441.x
Chapman, B. B., Bronmark, C., Nilsson, J.-Å., and Hansson, L.-A. (2011a). Partial migration: an introduction. Oikos 120, 1761–1763. doi: 10.1111/j.1600-0706.2011.20070.x
Chapman, B. B., Bronmark, C., Nilsson, J.-Å., and Hansson, L.-A. (2011b). The ecology and evolution of partial migration. Oikos 120, 1764–1775. doi: 10.1111/j.1600-0706.2011.20131.x
Chapman, B. B., Hulthen, K., Brodersen, J., Nilsson, P. A., Skov, C., Hansson, L.-A., et al. (2012). Partial migration in fishes: causes and consequences. J. Fish Biol. 81, 456–478. doi: 10.1111/j.1095-8649.2012.03342.x
Child, G., and Le Riche, J. D. (1969). Recent springbok treks (mass movements) in south-western Botswana. Mammalia 33, 499–504. doi: 10.1515/mamm.1969.33.3.499
Clarke, C. M. H., and Frampton, C. M. (1991). Structural changes in an apparently stable chamois population in Basin Creek, Canterbury, New-Zealand. N. Z. J. Zool. 18, 233–241. doi: 10.1080/03014223.1991.10418042
Clutton-Brock, T. H. (1984). Reproductive effort and terminal investment in iteroparous animals. Am. Nat. 123, 212–229. doi: 10.1086/284198
Clutton-Brock, T. H., Guinness, F. E., and Albon, S. D. (1982). Red Deer: Behavior and Ecology of Two Sexes. Chicago, IL: University of Chicago Press.
Colson, K. E., White, K. S., and Hundertmark, K. J. (2016). Parturition site selection in moose (Alces alces): evidence for social structure. J. Mammal. 97, 788–797. doi: 10.1093/jmammal/gyw006
Coltman, D. W., Pilkington, J. G., and Pemberton, J. M. (2003). Fine-scale genetic structure in a free-living ungulate population. Mol. Ecol. 12, 733–742. doi: 10.1046/j.1365-294X.2003.01762.x
Cook, J. G., Cook, R. C., Davis, R. W., and Irwin, L. L. (2016). Nutritional ecology of elk during summer and autumn in the Pacific Northwest. Wildl. Monogr. 195, 1–81. doi: 10.1002/wmon.1020
Cook, J. G., Johnson, B. K., Cook, R. C., Riggs, R. A., Delcurto, T., Bryant, L. D., et al. (2004). Effects of summer-autumn nutrition and parturition date on reproduction and survival of elk. Wildl. Monogr. 155, 1–61. doi: 10.2193/0084-0173(2004)155[1:EOSNAP]2.0.CO;2
Couzin, I. D., Krause, J., Franks, N. R., and Levin, S. A. (2005). Effective leadership and decision-making in animal groups on the move. Nature 433, 513–516. doi: 10.1038/nature03236
Crampe, J.-P., Bon, R., Gerard, J.-F., Serrano, E., Caens, P., Florence, E., et al. (2007). Site fidelity, migratory behaviour, and spatial organization of female isards (Rupicapra pyrenaica) in the Pyrenees National Park, France. Can. J. Zool. 85, 16–25. doi: 10.1139/z06-185
Damiani, M. L., Issa, H., Fotino, G., Heurich, M., and Cagnacci, F. (2016). Introducing ‘presence' and ‘stationarity index' to study partial migration patterns: an application of a spatio-temporal clustering technique. Int. J. Geogr. Inf. Sci. 30, 907–928. doi: 10.1080/13658816.2015.1070267
Dawkins, R. (1999). The Extended Phenotype: The Long Reach of the Gene. Oxford: Oxford University Press.
Dingemanse, N. J., Kazem, A. J. N., Réale, D., and Wright, J. (2010). Behavioural reaction norms: animal personality meets individual plasticity. Trends Ecol. Evol. 25, 81–89. doi: 10.1016/j.tree.2009.07.013
Dingle, H. (ed.) (2014a). “Behavioral and life-history variability in migration,” in Migration: The Biology of Life on the Move (Oxford: Oxford University Press), 211–230.
Dingle, H. (ed.) (2014b). “Migration: definition and scope,” in Migration: The Biology of Life on the Move (Oxford: Oxford University Press), 13–23.
Dingle, H., and Drake, V. A. (2007). What is migration? Bioscience 57, 113–121. doi: 10.1641/B570206
Dodson, J. J. (1988). Nature and role of learning in the orientation and migratory behavior of fishes. Environ. Biol. Fishes 23, 161–182. doi: 10.1007/BF00004908
Dominey, W. J. (1984). Alternative mating tactics and evolutionarily stable strategies. Am. Zool. 24, 385–396. doi: 10.1093/icb/24.2.385
Eggeman, S. L., Hebblewhite, M., Bohm, H., Whittington, J., and Merrill, E. H. (2016). Behavioural flexibility in migratory behaviour in a long-lived large herbivore. J. Anim. Ecol. 85, 785–797. doi: 10.1111/1365-2656.12495
Ericsson, G., and Wallin, K. (2001). Age-specific moose (Alces alces) mortality in a predator-free environment: evidence for senescence in females. Ecoscience 8, 157–163. doi: 10.1080/11956860.2001.11682641
Fagan, W. F., Cantrell, R. S., Cosner, C., Mueller, T., and Noble, A. E. (2012). Leadership, social learning, and the maintenance (or collapse) of migratory populations. Theor. Ecol. 5, 253–264. doi: 10.1007/s12080-011-0124-2
Festa-Bianchet, M. (1988). Seasonal range selection in bighorn sheep: conflicts between forage quality, forage quantity, and predator avoidance. Oecologia 75, 580–586. doi: 10.1007/BF00776423
Fieberg, J. R., and Kochanny, C. O. (2005). Quantifying home-range overlap: the importance of the utilization distribution. J. Wildl. Manage. 69, 1346–1359. doi: 10.2193/0022-541X(2005)69[1346:QHOTIO]2.0.CO;2
Fieberg, J. R., Kuehn, D. W., and DelGiudice, G. D. (2008). Understanding variation in autumn migration of northern white-tailed deer by long-term study. J. Mammal. 89, 1529–1539. doi: 10.1644/07-MAMM-A-277.1
Folstad, I., Nilssen, A. C., Halvorsen, O., and Andersen, J. (1991). Parasite avoidance: the cause of post-calving migrations in Rangifer? Can. J. Zool. 69, 2423–2429. doi: 10.1139/z91-340
Forsyth, D. M. (1999). Long-term harvesting and male migration in a New Zealand population of Himalayan tahr Hemitragus jemlahicus. J. Appl. Ecol. 36, 351–362. doi: 10.1046/j.1365-2664.1999.00410.x
Found, R., and St. Clair, C. C. (2016). Behavioural syndromes predict loss of migration in wild elk. Anim. Behav. 115, 35–46. doi: 10.1016/j.anbehav.2016.02.007
Found, R., and St. Clair, C. C. (2017). Ambidextrous ungulates have more flexible behaviour, bolder personalities and migrate less. R. Soc. Open Sci. 4:160958. doi: 10.1098/rsos.160958
Frank, D. A. (1998). Ungulate regulation of ecosystem processes in Yellowstone National Park: direct and feedback effects. Wildl. Soc. Bull. 26, 410–418.
Fretwell, S. D., and Lucas, H. L. (1969). On territorial behavior and other factors influencing habitat distribution in birds. Acta Biotheor. 19, 16–36. doi: 10.1007/BF01601953
Fritzsche McKay, A., and Hoye, B. J. (2016). Are migratory animals superspreaders of infection? Integr. Comp. Biol. 56, 260–267. doi: 10.1093/icb/icw054
Fryxell, J. M., Greever, J., and Sinclair, A. R. E. (1988). Why are migratory ungulates so abundant? Am. Nat. 131, 781–798. doi: 10.1086/284822
Fryxell, J. M., and Holt, R. D. (2013). Environmental change and the evolution of migration. Ecology 94, 1274–1279. doi: 10.1890/12-0668.1
Fryxell, J. M., Milner-Gulland, E. J., and Sinclair, A. R. E. (2011). “Introduction,” in Animal Migration: A Synthesis, eds E. J. Milner-Gulland, J. M. Fryxell, and A. R. E. Sinclair (Oxford: Oxford University Press, 1–3.
Fryxell, J. M., and Sinclair, A. R. E. (1988a). Causes and consequences of migration by large herbivores. Trends Ecol. Evol. 3, 237–241. doi: 10.1016/0169-5347(88)90166-8
Fryxell, J. M., and Sinclair, A. R. E. (1988b). Seasonal migration by white-eared kob in relation to resources. Afr. J. Ecol. 26, 17–31. doi: 10.1111/j.1365-2028.1988.tb01125.x
Fudickar, A. M., Schmidt, A., Hau, M., Quetting, M., and Partecke, J. (2013). Female-biased obligate strategies in a partially migratory population. J. Anim. Ecol. 82, 863–871. doi: 10.1111/1365-2656.12052
Gaidet, N., and Lecomte, P. (2013). Benefits of migration in a partially-migratory tropical ungulate. BMC Ecol. 13:36. doi: 10.1186/1472-6785-13-36
Gaillard, J.-M. (2013). Assessing fitness consequences of migratory tactics requires long-term individually based monitoring. Ecology 94, 1261–1264. doi: 10.1890/12-0710.1
Gaillard, J.-M., Festa-Bianchet, M., and Yoccoz, N. G. (1998). Population dynamics of large herbivores: variable recruitment with constant adult survival. Trends Ecol. Evol. 13, 58–63. doi: 10.1016/S0169-5347(97)01237-8
Gasaway, W. C., Gasaway, K. T., and Berry, H. H. (1996). Persistent low densities of plains ungulates in Etosha National Park, Namibia: testing the food-regulating hypothesis. Can. J. Zool. 74, 1556–1572. doi: 10.1139/z96-170
Gauthreaux, S. A. Jr. (1982). “The ecology and evolution of avian migration systems” in Avian Biology, eds D. S. Farner, J. R. King, and K. C. Parkes (Orlando, FL: Academic Press, Inc., 93–168.
Grayson, K. L., Bailey, L. L., and Wilbur, H. M. (2011). Life history benefits of residency in a partially migrating pond-breeding amphibian. Ecology 92, 1236–1246. doi: 10.1890/11-0133.1
Gross, M. R. (1996). Alternative reproductive strategies and tactics: diversity within sexes. Trends Ecol. Evol. 11, 92–98. doi: 10.1016/0169-5347(96)81050-0
Grovenburg, T. W., Jacques, C. N., Klaver, R. W., DePerno, C. S., Brinkman, T. J., Swanson, C. C., et al. (2011). Influence of landscape characteristics on migration strategies of white-tailed deer. J. Mammal. 92, 534–543. doi: 10.1644/09-MAMM-A-407.1
Guan, T. P., Ge, B. M., McShea, W. J., Li, S., Song, Y. L., and Stewart, C. M. (2013). Seasonal migration by a large forest ungulate: a study on takin (Budorcas taxicolor) in Sichuan Province, China. Eur. J. Wildl. Res. 59, 81–91. doi: 10.1007/s10344-012-0650-2
Gurarie, E., Cagnacci, F., Peters, W. E., Fleming, C. H., Calabrese, J. M., Mueller, T., et al. (2017). A framework for modelling range shifts and migrations: asking when, whither, whether and will it return. J. Anim. Ecol. 86, 943–959. doi: 10.1111/1365-2656.12674
Hansen, B. B., Aanes, R., and Sæther, B.-E. (2010). Partial seasonal migration in high-arctic Svalbard reindeer (Rangifer tarandus platyrhynchus). Can. J. Zool. 88, 1202–1209. doi: 10.1139/Z10-086
Harris, G., Thirgood, S., Hopcraft, J. G. C., Cromsigt, J. P. G. M., and Berger, J. (2009). Global decline in aggregated migrations of large terrestrial mammals. Endanger. Species Res. 7, 55–76. doi: 10.3354/esr00173
Hebblewhite, M., and Merrill, E. H. (2007). Multiscale wolf predation risk for elk: does migration reduce risk? Oecologia 152, 377–387. doi: 10.1007/s00442-007-0661-y
Hebblewhite, M., and Merrill, E. H. (2011). Demographic balancing of migrant and resident elk in a partially migratory population through forage–predation tradeoffs. Oikos 120, 1860–1870. doi: 10.1111/j.1600-0706.2011.19436.x
Hebblewhite, M., Merrill, E. H., and McDermid, G. (2008). A multi-scale test of the forage maturation hypothesis in a partially migratory ungulate population. Ecol. Monogr. 78, 141–166. doi: 10.1890/06-1708.1
Hebblewhite, M., Merrill, E. H., Morgantini, L. E., White, C. A., Allen, J. R., Bruns, E., et al. (2006). Is the migratory behavior of montane elk herds in peril? The case of Alberta's Ya Ha Tinda elk herd. Wildl. Soc. Bull. 34, 1280–1294. doi: 10.2193/0091-7648(2006)34[1280:ITMBOM]2.0.CO;2
Hegemann, A., Fudickar, A. M., and Nilsson, J.-Å. (2019). A physiological perspective on the ecology and evolution of partial migration. J. Ornithol. 160, 893–905. doi: 10.1007/s10336-019-01648-9
Hillman, J. C. (1988). Home range and movement of the common eland (Taurotragus oryx Pallas 1766) in Kenya. Afr. J. Ecol. 26, 135–148. doi: 10.1111/j.1365-2028.1988.tb00964.x
Histol, T., and Hjeljord, O. (1993). Winter feeding strategies of migrating and nonmigrating moose. Can. J. Zool. 71, 1421–1428. doi: 10.1139/z93-196
Hjeljord, O. (2001). Dispersal and migration in northern forest deer - Are there unifying concepts? Alces 37, 353–370.
Holdo, R. M., Holt, R. D., Coughenour, M. B., and Ritchie, M. E. (2006). Plant productivity and soil nitrogen as a function of grazing, migration and fire in an African savanna. J. Ecol. 95, 115–128. doi: 10.1111/j.1365-2745.2006.01192.x
Holdo, R. M., Holt, R. D., and Fryxell, J. M. (2009). Opposing rainfall and plant nutritional gradients best explain the wildebeest migration in the Serengeti. Am. Nat. 173, 431–445. doi: 10.1086/597229
Holdo, R. M., Holt, R. D., Sinclair, A. R. E., Godley, B. J., and Thirgood, S. (2011). “Migration impacts on communities and ecosystems: empirical evidence and theoretical insights,” in Animal Migration: A Synthesis, eds E. J. Milner-Gulland, J. M. Fryxell, and A. R. E. Sinclair (Oxford: Oxford University Press, 131–143.
Holt, R. D., and Fryxell, J. M. (2011). “Theoretical reflections on the evolution of migration,” in Animal Migration: A Synthesis, eds E. J. Milner-Gulland, J. M. Fryxell, and A. R. E. Sinclair (New York, NY: Oxford University Press, 17–31.
Hoskinson, R. L., and Tester, J. R. (1980). Migration behavior of pronghorn in southeastern Idaho. J. Wildl. Manage. 44, 132–144. doi: 10.2307/3808359
Huffman, B. (2018). Ultimate Ungulate. Available online at: www.ultimateungulate.com (accessed December 20, 2018).
Jachowski, D. S., Kauffman, M. J., Jesmer, B. R., Sawyer, H., and Millspaugh, J. J. (2018). Integrating physiological stress into the movement ecology of migratory ungulates: a spatial analysis with mule deer. Conserv. Physiol. 6, 1–12. doi: 10.1093/conphys/coy054
Jachowski, D. S., and Singh, N. J. (2015). Toward a mechanistic understanding of animal migration: incorporating physiological measurements in the study of animal movement. Conserv. Physiol. 3, 1–12. doi: 10.1093/conphys/cov035
Jesmer, B. R., Merkle, J. A., Goheen, J. R., Aikens, E. O., Beck, J. L., Courtemanch, A. B., et al. (2018). Is ungulate migration culturally transmitted? Evidence of social learning from translocated animals. Science 361, 1023–1025. doi: 10.1126/science.aat0985
Jones, J. D., Kauffman, M. J., Monteith, K. L., Scurlock, B. M., Albeke, S. E., and Cross, P. C. (2014). Supplemental feeding alters migration of a temperate ungulate. Ecol. Appl. 24, 1769–1779. doi: 10.1890/13-2092.1
Kaitala, A., Kaitala, V., and Lundberg, P. (1993). A theory of partial migration. Am. Nat. 142, 59–81. doi: 10.1086/285529
Ketterson, E. D., and Nolan, V. Jr. (1976). Geographic variation and its climatic correlates in the sex ratio of eastern-wintering dark-eyed juncos (Junco hyemalis hyemalis). Ecology 57, 679–693. doi: 10.2307/1936182
Ketterson, E. D., and Nolan, V. Jr. (1983). “The evolution of differential bird migration,” in Current Ornithology, ed R. F. Johnston (New York, NY: Plenus Publishing Corporation, 357–402.
Kokko, H. (2007). Modelling for Field Biologists and Other Interesting People. Cambridge: Cambridge University Press.
Kokko, H. (2011). Directions in modelling partial migration: how adaptation can cause a population decline and why the rules of territory acquisition matter. Oikos 120, 1826–1837. doi: 10.1111/j.1600-0706.2011.19438.x
Kolar, J. L., Millspaugh, J. J., and Stillings, B. A. (2011). Migration patterns of pronghorn in southwestern North Dakota. J. Wildl. Manage. 75, 198–203. doi: 10.1002/jwmg.32
Kowalczyk, R., Krasinska, M., Kaminski, T., Górny, M., Struś, P., Hofman-Kaminska, E., et al. (2013). Movements of European bison (Bison bonasus) beyond the Białowieza Forest (NE Poland): range expansion or partial migrations? Acta Theriol. 58, 391–401. doi: 10.1007/s13364-013-0136-y
Lamberti, P., Mauri, L., and Apollonio, M. (2004). Two distinct patterns of spatial behaviour of female roe deer (Capreolus capreolus) in a mountainous habitat. Ethol. Ecol. Evol. 16, 41–53. doi: 10.1080/08927014.2004.9522653
Laughlin, R. E., Grant, T. L., Williams, R. W., and Jentsch, J. D. (2011). Genetic dissection of behavioral flexibility: reversal learning in mice. Biol. Psychiatry 69, 1109–1116. doi: 10.1016/j.biopsych.2011.01.014
Le Pendu, Y., and Ciofolo, I. (1999). Seasonal movements of giraffes in Niger. J. Trop. Ecol. 15, 341–353. doi: 10.1017/S0266467499000863
Lee, D. E., Kissui, B. M., Kiwango, Y. A., and Bond, M. L. (2016). Migratory herds of wildebeests and zebras indirectly affect calf survival of giraffes. Ecol. Evol. 6, 8402–8411. doi: 10.1002/ece3.2561
Lendrum, P. E., Anderson, C. R. Jr., Monteith, K. L., Jenks, J. A., and Bowyer, R. T. (2013). Migrating mule deer: effects of anthropogenically altered landscapes. PLoS ONE 8:e64548. doi: 10.1371/journal.pone.0064548
Liedvogel, M., Åkesson, S., and Bensch, S. (2011). The genetics of migration on the move. Trends Ecol. Evol. 26, 561–569. doi: 10.1016/j.tree.2011.07.009
Lundberg, P. (1987). Partial bird migration and evolutionarily stable strategies. J. Theor. Biol. 125, 351–360. doi: 10.1016/S0022-5193(87)80067-X
Lundberg, P. (1988). The evolution of partial migration in birds. Trends Ecol. Evol. 3, 172–175. doi: 10.1016/0169-5347(88)90035-3
Lundberg, P. (2013). On the evolutionary stability of partial migration. J. Theor. Biol. 321, 36–39. doi: 10.1016/j.jtbi.2012.12.017
Matthysen, E. (2005). Density-dependent dispersal in birds and mammals. Ecography 28, 403–416. doi: 10.1111/j.0906-7590.2005.04073.x
McDevitt, A. D., Mariani, S., Hebblewhite, M., DeCesare, N. J., Morgantini, L. E., Seip, D. R., et al. (2009). Survival in the Rockies of an endangered hybrid swarm from diverged caribou (Rangifer tarandus) lineages. Mol. Ecol. 18, 665–679. doi: 10.1111/j.1365-294X.2008.04050.x
McNaughton, S. J., Ruess, R. W., and Seagle, S. W. (1988). Large mammals and process dynamics in African ecosystems. Bioscience 38, 794–800. doi: 10.2307/1310789
McPeek, M. A., and Holt, R. D. (1992). The evolution of dispersal in spatially and temporally varying environments. Am. Nat. 140, 1010–1027. doi: 10.1086/285453
Meland, M. (2014). Partial migration as a response to ground icing events in a high arctic ungulate (MSc thesis). Norwegian University of Life Sciences, As, Norway.
Merkle, J. A., Monteith, K. L., Aikens, E. O., Hayes, M. M., Hersey, K. R., Middleton, A. D., et al. (2016). Large herbivores surf waves of green-up during spring. Proc. R. Soc. B Biol. Sci. 283:20160456. doi: 10.1098/rspb.2016.0456
Middleton, A. D., Kauffman, M. J., McWhirter, D. E., Cook, J. G., Cook, R. C., Nelson, A. A., et al. (2013a). Animal migration amid shifting patterns of phenology and predation: lessons from a Yellowstone elk herd. Ecology 94, 1245–1256. doi: 10.1890/11-2298.1
Middleton, A. D., Kauffman, M. J., McWhirter, D. E., Jimenez, M. D., Cook, R. C., Cook, J. G., et al. (2013b). Linking anti-predator behaviour to prey demography reveals limited risk effects of an actively hunting large carnivore. Ecol. Lett. 16, 1023–1030. doi: 10.1111/ele.12133
Middleton, A. D., Merkle, J. A., McWhirter, D. E., Cook, J. G., Cook, R. C., White, P. J., et al. (2018). Green-wave surfing increases fat gain in a migratory ungulate. Oikos 127, 1060–1068. doi: 10.1111/oik.05227
Milner-Gulland, E. J., Kholodova, M. V., Bekenov, A., Bukreeva, O. M., Grachev, I. A., Amgalan, L., et al. (2001). Dramatic declines in saiga antelope populations. Oryx 35, 340–345. doi: 10.1046/j.1365-3008.2001.00202.x
Monteith, K. L., Bleich, V. C., Stephenson, T. R., Pierce, B. M., Conner, M. M., Kie, J. G., et al. (2014). Life-history characteristics of mule deer: effects of nutrition in a variable environment. Wildl. Monogr. 186, 1–62. doi: 10.1002/wmon.1011
Monteith, K. L., Bleich, V. C., Stephenson, T. R., Pierce, B. M., Conner, M. M., Klaver, R. W., et al. (2011). Timing of seasonal migration in mule deer: effects of climate, plant phenology, and life-history characteristics. Ecosphere 2, 1–34. doi: 10.1890/ES10-00096.1
Moraga, C., Funes, M. C., Pizarro, J. C., Briceño, C., and Novaro, A. J. (2015). Effects of livestock on guanaco density, movements and habitat selection in a forest-grassland mosaic in Tierra del Fuego, Chile. Oryx 49, 30–41. doi: 10.1017/S0030605312001238
Morrison, T. A., and Bolger, D. T. (2012). Wet season range fidelity in a tropical migratory ungulate. J. Anim. Ecol. 81, 543–552. doi: 10.1111/j.1365-2656.2011.01941.x
Most, L., Hothorn, T., Muller, J., and Heurich, M. (2015). Creating a landscape of management: unintended effects on the variation of browsing pressure in a national park. For. Ecol. Manage. 338, 46–56. doi: 10.1016/j.foreco.2014.11.015
Mueller, T., Olson, K. A., Dressler, G., Leimgruber, P., Fuller, T. K., Nicolson, C., et al. (2011). How landscape dynamics link individual- to population-level movement patterns: a multispecies comparison of ungulate relocation data. Glob. Ecol. Biogeogr. 20, 683–694. doi: 10.1111/j.1466-8238.2010.00638.x
Mysterud, A. (1999). Seasonal migration pattern and home range of roe deer (Capreolus capreolus) in an altitudinal gradient in southern Norway. J. Zool. 247, 479–486. doi: 10.1111/j.1469-7998.1999.tb01011.x
Mysterud, A., Langvatn, R., Yoccoz, N. G., and Stenseth, N. C. (2001). Plant phenology, migration and geographical variation in body weight of a large herbivore: the effect of a variable topography. J. Anim. Ecol. 70, 915–923. doi: 10.1046/j.0021-8790.2001.00559.x
Mysterud, A., Loe, L. E., Zimmermann, B., Bischof, R., Veiberg, V., and Meisingset, E. L. (2011). Partial migration in expanding red deer populations at northern latitudes – a role for density dependence? Oikos 120, 1817–1825. doi: 10.1111/j.1600-0706.2011.19439.x
Mysterud, A., Qviller, L., Meisingset, E. L., and Viljugrein, H. (2016). Parasite load and seasonal migration in red deer. Oecologia 180, 401–407. doi: 10.1007/s00442-015-3465-5
Naidoo, R., Du Preez, P., Stuart-Hill, G., Jago, M., and Wegmann, M. (2012). Home on the range: factors explaining partial migration of African buffalo in a tropical environment. PLoS ONE 7:e36527. doi: 10.1371/journal.pone.0036527
Nelson, M. E. (1995). Winter range arrival and departure of white-tailed deer in northeastern Minnesota. Can. J. Zool. 73, 1069–1076. doi: 10.1139/z95-127
Nelson, M. E. (1998). Development of migratory behavior in northern white-tailed deer. Can. J. Zool. 76, 426–432. doi: 10.1139/z97-207
Nicholson, M. C., Bowyer, R. T., and Kie, J. G. (1997). Habitat selection and survival of mule deer: tradeoffs associated with migration. J. Mammal. 78, 483–504. doi: 10.2307/1382900
Northrup, J. M., Shafer, A. B. A., Anderson, C. R., Coltman, D. W., and Wittemyer, G. (2014). Fine-scale genetic correlates to condition and migration in a wild cervid. Evol. Appl. 7, 937–948. doi: 10.1111/eva.12189
Nouvellet, P., Bacon, J. P., and Waxman, D. (2009). Fundamental insights into the random movement of animals from a single distance-related statistic. Am. Nat. 174, 506–514. doi: 10.1086/605404
Olson, K. A., Fuller, T. K., Mueller, T., Murray, M. G., Nicolson, C., Odonkhuu, D., et al. (2010). Annual movements of Mongolian gazelles: nomads in the Eastern Steppe. J. Arid Environ. 74, 1435–1442. doi: 10.1016/j.jaridenv.2010.05.022
Packer, C., Ikanda, D., Kissui, B. M., and Kushnir, H. (2005). Conservation biology: Lion attacks on humans in Tanzania. Nature 436, 927–928. doi: 10.1038/436927a
Peters, W. E., Hebblewhite, M., Mysterud, A., Eacker, D. R., Hewison, A. J. M., Linnell, J. D. C., et al. (2019). Large herbivore migration plasticity along environmental gradients in Europe: Life-history traits modulate forage effects. Oikos 128, 416–429. doi: 10.1111/oik.05588
Peters, W. E., Hebblewhite, M., Mysterud, A., Spitz, D. B., Focardi, S., Urbano, F., et al. (2017). Migration in geographic and ecological space by a large herbivore. Ecol. Monogr. 87, 297–320. doi: 10.1002/ecm.1250
Piersma, T., and Drent, J. (2003). Phenotypic flexibility and the evolution of organismal design. Trends Ecol. Evol. 18, 228–233. doi: 10.1016/S0169-5347(03)00036-3
Plumb, G. E., White, P. J., Coughenour, M. B., and Wallen, R. L. (2009). Carrying capacity, migration, and dispersal in Yellowstone bison. Biol. Conserv. 142, 2377–2387. doi: 10.1016/j.biocon.2009.05.019
Poor, E. E., Loucks, C., Jakes, A., and Urban, D. L. (2012). Comparing habitat suitability and connectivity modeling methods for conserving pronghorn migrations. PLoS ONE 7:e49390. doi: 10.1371/journal.pone.0049390
Pruvot, M., Lejeune, M., Kutz, S., Hutchins, W., Musiani, M., Massolo, A., et al. (2016). Better alone or in ill company? The effect of migration and interspecies comingling on Fascioloides magna infection in elk. PLoS ONE 11:e0159319. doi: 10.1371/journal.pone.0159319
Pulido, F. (2011). Evolutionary genetics of partial migration - the threshold model of migration revis(it)ed. Oikos 120, 1776–1783. doi: 10.1111/j.1600-0706.2011.19844.x
Pulido, F., Berthold, P., and van Noordwijk, A. J. (1996). Frequency of migrants and migratory activity are genetically correlated in a bird population: evolutionary implications. Proc. Natl. Acad. Sci. U. S. A. 93, 14642–14647. doi: 10.1073/pnas.93.25.14642
Purdon, A., Mole, M. A., Chase, M. J., and van Aarde, R. J. (2018). Partial migration in savanna elephant populations distributed across southern Africa. Sci. Rep. 8, 1–11. doi: 10.1038/s41598-018-29724-9
Qviller, L., Risnes-Olsen, N., Bærum, K. M., Meisingset, E. L., Loe, L. E., Ytrehus, B., et al. (2013). Landscape level variation in tick abundance relative to seasonal migration in red deer. PLoS ONE 8:e71299. doi: 10.1371/journal.pone.0071299
Raithel, J. D., Kauffman, M. J., and Pletscher, D. H. (2007). Impact of spatial and temporal variation in calf survival on the growth of elk populations. J. Wildl. Manage. 71, 795–803. doi: 10.2193/2005-608
Ramanzin, M., Sturaro, E., and Zanon, D. (2007). Seasonal migration and home range of roe deer (Capreolus capreolus) in the Italian eastern Alps. Can. J. Zool. 85, 280–289. doi: 10.1139/Z06-210
Ramenofsky, M., Cornelius, J. M., and Helm, B. (2012). Physiological and behavioral responses of migrants to environmental cues. J. Ornithol. 153, 181–191. doi: 10.1007/s10336-012-0817-3
Réale, D., Dingemanse, N. J., Kazem, A. J. N., and Wright, J. (2010). Evolutionary and ecological approaches to the study of personality. Philos. Trans. R. Soc. B Biol. Sci. 365, 3937–3946. doi: 10.1098/rstb.2010.0222
Rice, C. G. (2008). Seasonal altitudinal movements of mountain goats. J. Wildl. Manage. 72, 1706–1716. doi: 10.2193/2007-584
Rivrud, I. M., Heurich, M., Krupczynski, P., Müller, J., and Mysterud, A. (2016). Green wave tracking by large herbivores: an experimental approach. Ecology 97, 3547–3553. doi: 10.1002/ecy.1596
Rolandsen, C. M., Solberg, E. J., Saether, B.-E., Van Moorter, B., Herfindal, I., and Bjorneraas, K. (2017). On fitness and partial migration in a large herbivore – migratory moose have higher reproductive performance than residents. Oikos 126, 547–555. doi: 10.1111/oik.02996
Sabine, D. L., Morrison, S. F., Whitlaw, H. A., Ballard, W. B., Forbes, G. J., and Bowman, J. (2002). Migration behavior of white-tailed deer under varying winter climate regimes in New Brunswick. J. Wildl. Manage. 66, 718–728. doi: 10.2307/3803137
Sakuragi, M., Igota, H., Uno, H., Kaji, K., Kaneko, M., Akamatsu, R., et al. (2003). Benefit of migration in female sika deer population in eastern Hokkaido, Japan. Ecol. Res. 18, 347–354. doi: 10.1046/j.1440-1703.2003.00560.x
Sawyer, H., and Kauffman, M. J. (2011). Stopover ecology of a migratory ungulate. J. Anim. Ecol. 80, 1078–1087. doi: 10.1111/j.1365-2656.2011.01845.x
Sawyer, H., Merkle, J. A., Middleton, A. D., Dwinnell, S. P. H., and Monteith, K. L. (2018). Migratory plasticity is not ubiquitous among large herbivores. J. Anim. Ecol. 8, 450–460. doi: 10.1111/1365-2656.12926
Sawyer, H., Middleton, A. D., Hayes, M. M., Kauffman, M. J., and Monteith, K. L. (2016). The extra mile: ungulate migration distance alters the use of seasonal range and exposure to anthropogenic risk. Ecosphere 7, 1–11. doi: 10.1002/ecs2.1534
Schuyler, E. M., Dugger, K. M., and Jackson, D. H. (2019). Effects of distribution, behavior, and climate on mule deer survival. J. Wildl. Manage. 83, 89–99. doi: 10.1002/jwmg.21558
Seip, D. R., and Bunnell, F. L. (1985). Foraging behaviour and food habits of Stone's sheep. Can. J. Zool. 63, 1638–1646. doi: 10.1139/z85-243
Shaw, A. K., and Binning, S. A. (2016). Migratory recovering from infection as a selective pressure for the evolution of migration. Am. Nat. 187, 491–501. doi: 10.1086/685386
Sih, A., Bell, A., and Johnson, J. C. (2004). Behavioral syndromes: an ecological and evolutionary overview. Trends Ecol. Evol. 19, 372–378. doi: 10.1016/j.tree.2004.04.009
Sih, A., Cote, J., Evans, M., Fogarty, S., and Pruitt, J. (2012). Ecological implications of behavioural syndromes. Ecol. Lett. 15, 278–289. doi: 10.1111/j.1461-0248.2011.01731.x
Sinclair, A. R. E. (1983). “The function of distance movement in vertebrates,” in Ecology of Animal Movement, eds I. R. Swingland and P. J. Greenwood (Oxford: Clarendon Press, 248–258.
Singer, F. J., Otto, D. K., Tipton, A. R., and Hable, C. P. (1981). Home ranges, movements, and habitat use of European wild boar in Tennessee. J. Wildl. Manage. 45, 343–353. doi: 10.2307/3807917
Singh, N. J., Allen, A. M., and Ericsson, G. (2016). Quantifying migration behaviour using net squared displacement approach: clarifications and caveats. PLoS ONE 11:e0149594. doi: 10.1371/journal.pone.0149594
Singh, N. J., Börger, L., Dettki, H., Bunnefeld, N., Ericsson, G., Borger, L., et al. (2012). From migration to nomadism: movement variability in a northern ungulate across its latitudinal range. Ecol. Appl. 22, 2007–2020. doi: 10.1890/12-0245.1
Skov, C., Baktoft, H., Brodersen, J., Bronmark, C., Chapman, B. B., Hansson, L.-A., et al. (2011). Sizing up your enemy: individual predation vulnerability predicts migratory probability. Proc. R. Soc. B Biol. Sci. 278, 1414–1418. doi: 10.1098/rspb.2010.2035
Smith, H. G., and Nilsson, J.-Å. (1987). Intraspecific variation in migratory pattern of a partial migrant, the blue tit (Parus caeruleus): an evaluation of different hypotheses. Auk 104, 109–115. doi: 10.2307/4087239
Spitz, D. B. (2015). Does migration matter? Causes and consequences of migratory behavior in Sierra Nevada bighorn sheep (Ph.D. dissertation). University of Montana, Missoula, MT, USA.
Spitz, D. B., Hebblewhite, M., and Stephenson, T. R. (2017). ‘MigrateR': extending model-driven methods for classifying and quantifying animal movement behavior. Ecography 40, 788–799. doi: 10.1111/ecog.02587
Spitz, D. B., Hebblewhite, M., Stephenson, T. R., and German, D. W. (2018). How plastic is migratory behavior? Quantifying elevational movement in a partially-migratory alpine ungulate, the Sierra Nevada bighorn sheep (Ovis canadensis sierrae). Can. J. Zool. 96, 1385–1394. doi: 10.1139/cjz-2017-0367
Sutherland, W. J. (1998). Evidence for flexibility and constraint in migration systems. J. Avian Biol. 29, 441–446. doi: 10.2307/3677163
Sweanor, P. Y., and Sandegren, F. (1988). Migratory behavior of related moose. Holarct. Ecol. 11, 190–193. doi: 10.1111/j.1600-0587.1988.tb00800.x
Swingland, I. R. (1983). “Intraspecific differences in movement,” in The Ecology of Animal Movement, eds I. R. Swingland and P. J. Greenwood (Oxford: Clarendon Press, 102–115.
Swingland, I. R., and Lessells, C. M. (1979). The natural regulation of giant tortoise populations on Aldabra Atoll. Movement polymorphism, reproductive success and mortality. J. Anim. Ecol. 48, 639–654. doi: 10.2307/4184
Takii, A., Izumiyama, S., and Taguchi, M. (2012). Partial migration and effects of climate on migratory movements of sika deer in Kirigamine Highland, central Japan. Mammal Study 37, 331–340. doi: 10.3106/041.037.0407
Terrill, S. B. (1987). Social dominance and migratory restlessness in the dark-eyed junco (Junco hyemalis). Behav. Ecol. Sociobiol. 21, 1–11. doi: 10.1007/BF00324429
Tucker, M. A., Böhning-Gaese, K., Fagan, W. F., Fryxell, J. M., Moorter, B., Van Alberts, S. C., et al. (2018). Moving in the Anthropocene: global reductions in terrestrial mammalian movements. Science 359, 466–469. doi: 10.1126/science.aam9712
Valenzuela, L. O., Sironi, M., Rowntree, V. J., and Seger, J. (2009). Isotopic and genetic evidence for culturally inherited site fidelity to feeding grounds in southern right whales (Eubalaena australis). Mol. Ecol. 18, 782–791. doi: 10.1111/j.1365-294X.2008.04069.x
Van Deelen, T. R., Campa, H. III., Hamady, M., and Haufler, J. B. (1998). Migration and seasonal range dynamics of deer using adjacent deeryards in northern Michigan. J. Wildl. Manage. 62, 205–213. doi: 10.2307/3802280
Visscher, D. R., and Merrill, E. H. (2018). Functional connectivity in ruminants: a generalized state-dependent modelling approach. PLoS ONE 13:e0199671. doi: 10.1371/journal.pone.0199671
Wahlström, L. K., and Liberg, O. (1995). Patterns of dispersal and seasonal migration in roe deer (Capreolus capreolus). J. Zool. 235, 455–467. doi: 10.1111/j.1469-7998.1995.tb01762.x
Weber, B. J., Wolfe, M. L., White, G. C., and Rowland, M. M. (1984). Physiologic response of elk to differences in winter range quality. J. Wildl. Manage. 48, 248–253. doi: 10.2307/3808482
White, K. S., Barten, N. L., Crouse, S., and Crouse, J. (2014). Benefits of migration in relation to nutritional condition and predation risk in a partially migratory moose population. Ecology 95, 225–237. doi: 10.1890/13-0054.1
Keywords: ungulate, partial migration, density-dependence, frequency-dependence, condition, review
Citation: Berg JE, Hebblewhite M, St. Clair CC and Merrill EH (2019) Prevalence and Mechanisms of Partial Migration in Ungulates. Front. Ecol. Evol. 7:325. doi: 10.3389/fevo.2019.00325
Received: 29 March 2019; Accepted: 13 August 2019;
Published: 29 August 2019.
Edited by:
Brett K. Sandercock, Norwegian Institute for Nature Research (NINA), NorwayReviewed by:
Arne Hegemann, Lund University, SwedenInger Maren Rivrud, Norwegian Institute for Nature Research (NINA), Norway
Marco Festa-Bianchet, Université de Sherbrooke, Canada
Copyright © 2019 Berg, Hebblewhite, St. Clair and Merrill. This is an open-access article distributed under the terms of the Creative Commons Attribution License (CC BY). The use, distribution or reproduction in other forums is permitted, provided the original author(s) and the copyright owner(s) are credited and that the original publication in this journal is cited, in accordance with accepted academic practice. No use, distribution or reproduction is permitted which does not comply with these terms.
*Correspondence: Jodi E. Berg, amJlcmdAdWFsYmVydGEuY2E=