- 1Vertebrates Division, Life Sciences Department, Natural History Museum, London, United Kingdom
- 2Center for Molecular Medicine, Umeå University, Umeå, Sweden
- 3Oceans Graduate School, University of Western Australia, Crawley, WA, Australia
- 4Oceans Institute, University of Western Australia, Crawley, WA, Australia
- 5School of Biological Sciences, University of Western Australia, Crawley, WA, Australia
- 6Center for Ophthalmology and Visual Science, Lions Eye Institute, University of Western Australia, Crawley, WA, Australia
There have been a growing number of studies into the visual evolution of vertebrates. However, there remain few detailed integrative studies on the visual system of amphibians using morphological, molecular and physiological methods outside of a few model species. There are many examples of amphibian species that are closely related phylogenetically, but occupy vastly different ecological niches and so provide a substantial resource for the study of adaptive evolution. This review will examine the published literature on the three living orders of amphibians, the Anurans, Caudata, and Gymnophiona.
Introduction
The amphibian ancestor that pioneered the vertebrate colonization of the terrestrial environment is considered to be one of the most critical periods in vertebrate evolution (Herrick, 1948). This event was mainly associated with the transformation of fins into five-digit appendages, thus transforming locomotion from swimming to walking and initiating the diversification of land-based vertebrates (Nilsson, 2017). It was suggested, however, that a dramatic change in sensory abilities preceded the evolution of walking (MacIver et al., 2017). The move from aquatic to terrestrial life changed general eye morphology and a shift in eye position dorsally during the evolution of the first amphibious tetrapods from lobe-finned fishes. The Anura (frogs), the Caudata (salamanders) and the Gymnophiona (caecilians) are the only three remaining living orders of Amphibia (Frost et al., 2006). However, amphibians as a group display substantial morphological diversity and a wide variety of parental care, as well as reproductive modes (Duellman and Trueb, 1994; Wake and Dicke, 1998; San Mauro et al., 2014).
This review will discuss the physiological, morphological and molecular adaptations associated with eye evolution in Amphibia.
General Morphology of a Visually Orientated Amphibian Eye
Visually orientated amphibians have an eye with features typically found in the vertebrate eye: for example, they possess a multi-layered retina, a crystalline lens, vitreous and aqueous humor, a tough sclera for support and a choroid which contains blood vessels (Walls, 1942).
Despite these common features, the amphibians show a wide diversity of eye morphologies that reflects their diverse life histories and habitats.
Disregarding the size of the eye, the frog (Anura) eye and the newt (Caudata) eye are similar in structure (Reyer, 1977). Both eye types have been extensively described (Walls, 1942; Rochon-Duvigneaud, 1943; Duke-Elder, 1958; Reyer, 1977) and are generally thought to have well-developed eyes capable of high-resolution, with vision being a dominant sense in these species (Dawley, 1998). Conversely, chemosensory and tactile sensory systems are mainly used in caecilians (Himstedt and Simon, 1995). Frogs are typically visually oriented predators; with a prey capture mechanism (i.e., projectile tongues) that depends on visual cues. Although some salamanders have independently evolved a projectile tongue, prey capture is more commonly through jaw movement, with both vision and olfaction being important when locating prey. Several salamanders are cave dwelling (e.g., Proteus sp.) and some are aquatic throughout their lives (e.g., Cryptobranchus sp.), often as neotenic forms with relatively small eyes (Griisser-Cornehls and Himstedt, 1976).
When amphibians left the water for dry land and acquired aerial vision they were met by two potential challenges: eye dryness and corneal, rather than lens, focusing. Specifically, an optical reorientation of the aquatic eye to suit the new medium occurred, with the provision of lids that were equipped with elaborate glandular structures as a protection against drying (Reyer, 1977). Accommodation in amphibians differs somewhat from fish due to the transition from water to air. Terrestrial features are apparent in the flattened shape of the lens and a more spherical shape of the eye. The cornea is the major refractive structure, especially in terrestrial animals, due to the high difference in density between the cornea and the surrounding air (Roth et al., 1998). Accommodation occurs by moving the lens as a whole rather than the shape of the lens being changed by ciliary muscles (Walls, 1942). The lens is subsequently displaced in terrestrial adult amphibians that allows for a deep anterior cavity and becoming rather flattened in the antero-posterior direction (Walls, 1942; Keller and Shilton, 2002); however, there are exceptions. For example, adult Triturus sp. exhibit considerable aquatic behaviors and possess a spherical lens (Himstedt, 1995).
The majority of amphibians that live their adult life on land develop a short upper and lower lid during metamorphosis (Walls, 1942). The retina and lens, structures related to light detection, appear early in larval life, while terrestrial elements do not develop until after metamorphosis (Volonteri et al., 2017), with axolotls only having a dorsal eyelid rudiment. Nictitating membranes, while present in frogs, are absent in urodeles, but the reasons underlying this loss are unclear (Cuny and Malacinski, 1986).
Eyelids and glands have adapted to protect eyes that are exposed to air rather than water. The eyelids are folds of skin that function in the cleaning and moistening of the cornea, whereas glands lubricate the eye. The lachrymal gland produces tear fluid and lies against the superior temporal quadrant of the eye in the anterior part of the orbit. These tears are mixed with mucus secreted by scattered cells in the conjunctiva. The Harderian glands are hypertrophied and occupy the nasal half of the orbit in caecilians (Walls, 1942).
The ciliary apparatus, used for accommodation in order to focus on an object, show differences between different amphibian orders, with a more complex mechanism found in visually orientated frogs and salamanders. Dorsally and ventrally, in both frogs and newts, smaller bundles of smooth muscle, the tensor choroideae, extend from the sclera and insert onto the choroid close to the ora serrata. In frogs, there is typically a dorsal and ventral protractor lentis muscle. In salamanders, there is only a ventral protractor lentis muscle and a dorsal suspensory ligament (Walls, 1942). Non-derived caecilians, such as Ichthyophis sp. and Rhinatrema sp., seem to have rudimentary elements of a ciliary body (e.g., underdeveloped zonule fibers and a potential ventral musculus protractor lentis) that is functionally associated with the presence of aqueous humor (Himstedt, 1995; Mohun and Wilkinson, 2015). An absence of iris muscles and accommodation muscles was reported in caecilians, so accommodation was presumed not to occur (Himstedt, 1995). However, zonule fibers connecting the iris to the lens and musculus protractor lentis are present in Rhinatrema sp. continuing toward the cornea and the iris that may suggest that some accommodation might occur (Mohun et al., 2010). Compared with other amphibians, the structure of the iris ciliary body complex appears to be weakly developed. There are no ciliary processes on the iris and no pupillary nodule to raise the iris from the lens and permit aqueous fluid to move to the back of the lens during accommodation (Mohun et al., 2010).
In addition to the six extrinsic muscles that occur in the vertebrate eye, most salamanders and frogs possess retractor bulbi and levator bulbi muscles that move the eye as a whole. The retractor bulbi muscle is probably derived from the external rectus muscle, as well as the levator bulbi muscle from the jaw musculature (Duke-Elder, 1958). If the eye is touched, the retractor muscle retracts the eyeball while simultaneously pulling the nictitating membrane over the cornea. The levator bulbi muscle pulls the eyeball forward again and the lower lid falls back to its normal position. Eye retraction accompanies feeding and is likely an aid to swallowing food (Levine et al., 2004).
The sensory tentacle found in caecilians is believed to have co-opted numerous elements of a typical vertebrate eye. For example, the Harderian gland, the eyelids, the retractor and levator bulbi muscles and their nerves are present in caecilians (Billo and Wake, 1987). The presence of a tentacle is associated with the vomeronasal organ and its glands is a diagnostic characteristic of caecilians. Indeed, it is believed that the tentacle is involved in tactile and chemoreceptive functional tasks (Himstedt and Simon, 1995). In most caecilians, the tentacle migrates anteriorly from the eye in ontogeny, whereas in scolecomorphids, the eye remains attached to the tentacle and migrates with it out of the orbital chamber (Nussbaum, 1977).
Amphibian retinae are unusual in having two types of rod photoreceptor (red and green), in addition to single and double cones. The outer segment of the red rod is unusually large and the nucleus is in contact with the outer limiting membrane, a level generally occupied by cone nuclei (Walls, 1942). The green rod differs from the red rod in having a shorter outer segment and an elongated inner segment. The single cone has a much smaller tapering outer segment compared to the red rod, as well as a plump inner segment containing both an ellipsoid of mitochondria (less densely packed than in the rod) and a darkly staining oil or lipid droplet. The single cone possesses an oil droplet, while it is missing in the red rod (Mariani, 1986). Differences have been noted between newt and frog retinas with respect to the presence of oil droplets, cytoplasmic extensions from the accessory member which clasp the principal member of the double cone to that of a rod, and more extensive areas of cell-to-cell contact among the different photoreceptors in frogs (Keefe, 1971). The proportions of the rods, cones and double cones are correlated with the relative degree of diurnal and nocturnal activity (Griisser-Cornehls and Himstedt, 1976).
Morphological Adaptations of Amphibians to the Dark
Amphibian visual systems has been observed in caecilians and salamanders to adapt in cave dwelling and fossorial habitats (Himstedt, 1995). This can be seen by a reduction in absolute size, disorganization of the retina, an absence of the lens and co-option of structures to other sensory systems (i.e., tactile/chemosensory tentacle).
Many authors have noted the reduction of the gymnophionian visual system (Norris and Hughes, 1918; Walls, 1942; Storch and Welsh, 1973). The eyes of adult caecilians are comparatively small, covered with skin and occasionally bone, with retinal cells containing only one type of opsin (rod) and structures now utilized for chemosensory and tactile functions (Billo and Wake, 1987). Some researchers have been prompted by the fossorial and possible nocturnal habits to suggest that the caecilian eye is non-functional (Norris and Hughes, 1918; Walls, 1942). Storch and Welsh (1973) examined the choroid layer ultrastructure and photoreceptor cells of Ichthyophis cf. kohtoensis, where they found reduced organelle counts in photoreceptor cells, a choroid layer with a smooth basal plasma membrane, as well as few apical cellular processes that only allow loose contact with the outer segments of photoreceptor cells. They interpreted these data as indicating “markedly decreased functional activity” (Storch and Welsh, 1973). There are further differences between caecilian and other amphibian eyes, including a lack of the development of components for accommodation and the fusion of the cornea to the dermis, all of which correlate with reduced function (Himstedt, 1995). Although caecilian eyes are considerably smaller compared to those of most other amphibians, both absolutely and in relation to head and body size (Himstedt, 1995), more recent research has provided anatomical, behavioral, physiological, neurological and molecular biological evidence that show that while caecilian eyes are reduced they persist to be functional in many respects (Wake, 1980, 1985; Himstedt, 1995; Dünker, 1997, 1998; Mohun, 2008; Mohun et al., 2010; Mohun and Wilkinson, 2015).
Wake (1985) examined 18 caecilian species, including seven of the ten families presently recognized (Wilkinson et al., 2011; Kamei et al., 2012). Morphological confirmation of a functioning eye, such as an optic nerve and a complex retina, was identified in all species. Wake (1985) also found that the eyes were relatively well-developed in caecilians with less heavily solidified skulls, such as Ichthyophis spp. Rhinatrema bivittatum appears to be more plesiomorphic than the ichthyophiids, with eyes projecting further out from the skull, with an incompletely adherent cornea less tightly attached to the surrounding skin, and possessing aqueous humor (Mohun and Wilkinson, 2015). Those with substantial, stegokrotaphic skulls (e.g., Gegeneophis sp.), thought to be a derived adaptation to a more obligate burrowing lifestyle (e.g., Ducey et al., 1993; Gower et al., 2004), sometimes had only a mass of relatively undifferentiated cells with more rudimentary eyes. The more rudimentary eye in Gegeneophis sp. is covered by opaque bone and glandular skin, while the Ichthyophis sp. eye is covered by transparent skin (Wake, 1985).
An atrophied visual system is an adaptively beneficial response to the unique underground (or cave dwelling) environment where light in greatly reduced or absent altogether (Nevo, 2007). The eye morphology of caecilians and cave dwelling salamanders have features that are associated with nocturnality, such as a rod-based retina, large lenses and a thick and dark pigment epithelium. These changes suggest that the eye has adapted to reflect as much light on to the retina with a reduction of obscuring light signals that could potentially reflect within the eyeball (Walls, 1942). Some commonalities are found with nocturnal vertebrates, such as some geckos and deep-sea fishes that have rod-based visual systems (Kojima et al., 1992). The primary benefit of sensory atrophy is the metabolic economy achieved by reducing visual structures that do not significantly contribute to the fitness of the animal (Nevo, 1999, 2007). However, the retention of a rudimentary visual system in those caecilians that have a heavily ossified skull and appear to be the most dedicated burrowers, as well as cave dwelling salamanders (e.g., Proteus anguinus) shows a loss of cone photoreceptors and their associated opsins while retaining the rod photopigment (Kos et al., 2001). Together, these aspects suggest that the rudimentary eye serves some function, perhaps with respect to photoperiodic perception and circadian photoentrainment.
Vertebrate Opsins and Photoreceptors
The photoreceptor cells of vertebrates are of two general types, namely the rods and cones (Walls, 1942). The rods are allied with dim light (scotopic) vision, while the cones are used for daylight and colored vision. This is due to specific visual photopigments (consisting of opsin proteins conjugated to light-sensitive chromophores) (Figure 1) that are associated with the rod and cone photoreceptors as well as the morphological properties of the photoreceptor cells themselves. However, these photoreceptor cells have essentially the same principal parts, the outer and inner segments, nucleus, and foot piece (Walls, 1942). The outer segment is a long membrane-enclosed lamellae of disks, with about 80% of the disk membrane protein complement consisting of a specific visual photopigment.
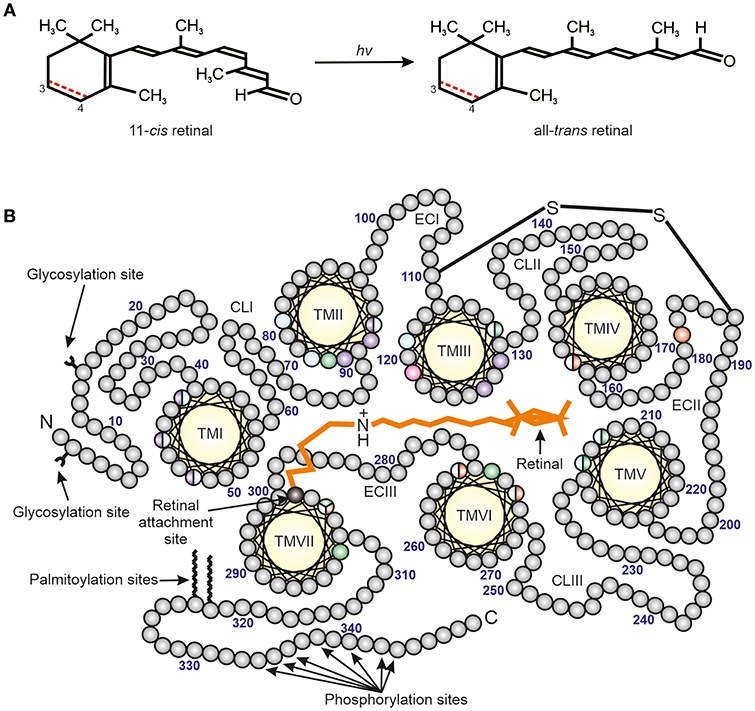
Figure 1. The structure of vertebrate photopigments. (A) The initial step in phototransduction consists of photon (hv) absorption by 11-cis retinal, which photoconverts to all-trans retinal. Vertebrate photopigments are broadly divided into rhodopsins that utilize a vitamin A1-derived chromophore (black line) or porphyropsins that contain a vitamin A2-derived chromophore (3,4-didehydroretinal). In the latter case, the presence of a double (C=C) bond between C3 and C4 is shown as a dotted red line. (B) A mid-membrane section of a typical (opsin) photopigment, showing the presence of seven transmembrane domains (yellow), archetypal of the GPCR superfamily, and their arrangement around the retinal chromophore (orange) (modified from Davies et al., 2012). The retinal attachment site (Lys296, black) and counterion (Glu113, pink) to the Schiff base are shown. Opsin residues that cluster either around the Schiff base or ionone ring of the retinal chromophore are colored to highlight the amino acids involved in the spectral tuning of LWS (red), SWS1 (violet), SWS2 (blue) and RH2/RH1 (green) photopigments. Residues important for stabilizing the tertiary structure (e.g., disulphide bridge, amino-terminal (N) glycosylation sites) and the activation/deactivation of photopigments (e.g., carboxyl-terminal (C) phosphorylation sites), as well as membrane anchorage (e.g., palmitoylation sites), are also shown. TM, transmembrane; CL, cytoplasmic loop; EC, extracellular loop. The numbering is based on the bovine rod opsin (RH1) sequence.
Spectral sensitivities can be modified by variations in the structure of the opsin protein by substitution of amino acids (so called “tuning sites”) and/or by the use of either vitamin A1 (rhodopsins) or vitamin A2 (porphyropsins) based chromophores (Crescitelli, 1972; Yokoyama, 2000; Bowmaker and Hunt, 2006; Palczewski, 2006; Hart and Hunt, 2007; Davies et al., 2012) (Figure 1). Rods typically express RH1 photopigments and rod-specific components of the phototransduction cascade. By contrast, vertebrate color vision utilzes up to four classes of cone opsin genes (Hunt et al., 2001; Bowmaker and Hunt, 2006; Davies et al., 2012). In addition to a rod photopigment that is maximally sensitive to around 500 nm, the λmax values of the cone opsin-based photopigments (based on a vitamin A1 chromophore) are between about 500–570 nm for the “red” (long-wavelength-sensitive; encoded by the LWS gene), 470–520 nm for the “green” (middle-wavelength-sensitive; encoded by the RH2 gene), 440–470 nm for the “blue” (short-wavelength-sensitive 2; encoded by the SWS2 gene) and 360–440 nm for the “ultraviolet” or “violet” (short-wavelength-sensitive 1; encoded by the SWS1 gene) (Yokoyama, 2000; Shichida and Matsuyama, 2009; Davies et al., 2012).
Amphibian Opsins and Photoreceptors
As mentioned earlier, amphibians have four types of photoreceptor. A common class (90–95%) of photoreceptors (i.e., the “red” rods) expressing a rod RH1 pigment with an average spectral maximum of 500 nm is found in all amphibian orders (Table 1). In addition, a marginal rod photoreceptor class expressing an SWS2 cone pigment gene in “green” rods is found in salamanders and frogs (Hisatomi et al., 1998; Ma et al., 2001a; Darden et al., 2003). Frogs and salamanders also have a double-cone LWS photopigment (Sherry et al., 1998; Makino et al., 1999; Röhlich and Szél, 2000; Korenyak and Govardovskii, 2013), coupled with either a UV-sensitive (SWS1) photopigment or a blue-sensitive (SWS2) photopigment expressed in single cones. Note that the latter photopigment is identical to the one expressed in the “green” rods of some species (Hárosi, 1975; Deutschlander and Phillips, 1995; Hisatomi et al., 1998; Ma et al., 2001a,b; Takahashi et al., 2001; Korenyak and Govardovskii, 2013), where it interacts with the rod-specific G-protein transducin isoform instead of the cone isoform found in blue short-wavelength-sensitive (SWS2) cones (Hisatomi et al., 1998).
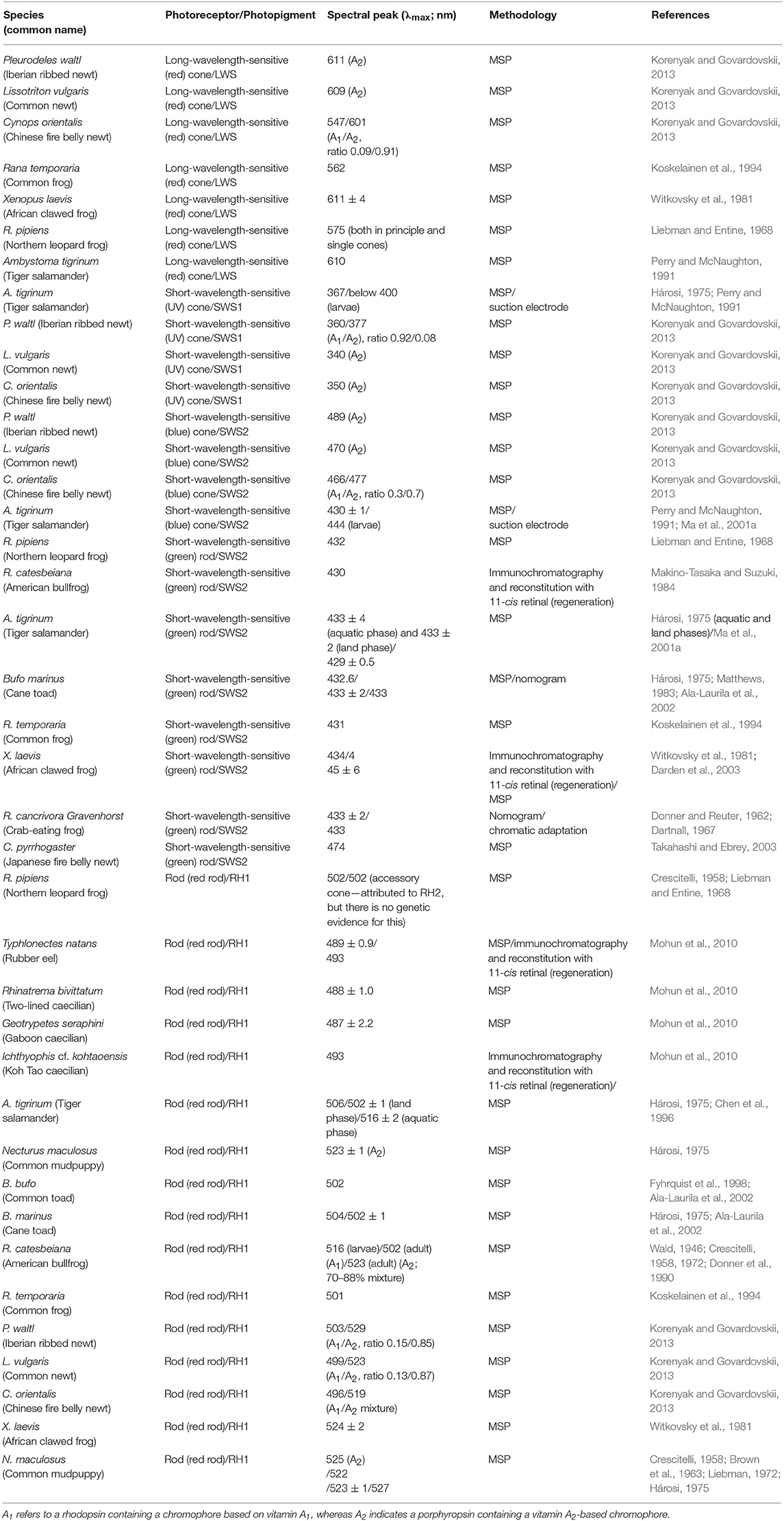
Table 1. Spectral absorbance peaks (λmax) and methodologies used for an array of different amphibian rods (red- and green-sensitive) and cones (blue- and UV-sensitive), with expressed photopigments and their chromophore usage indicated).
Opsin sequences have been isolated from every amphibian order. Full-length cone opsin sequences from the SWS2, SWS1 and LWS opsin classes (Figure 2) have been isolated from ranid frogs (Liebman and Entine, 1968), Xenopus laevis (Hárosi and Hashimoto, 1983; Batni et al., 1996), and the salamanders Ambystoma tigrinum (Hárosi, 1975) and Cynops pyrrhogaster (Takahashi et al., 2001; Sakakibara et al., 2002). The middle-wavelength-sensitive opsin (encoded by the RH2 gene) appears to have been missing in all amphibians studied thus far (Figure 2), but a visual pigment was identified by microspectrophotometry (MSP) with a maximal absorbance value at 502 nm in a cone (Liebman and Entine, 1968) (Table 1); however, the underlying photopigment gene has not been identified. It should be noted that no further evidence of the presence of RH2 in subsequent research on amphibian visual pigments has been found. In most cases, the rod opsin is found within “red” rods, which are also known as “principal” rods. The SWS2 opsin is associated with smaller “green” rods, whereas SWS1 and LWS are contained within small and large single cones, respectively (Röhlich and Szél, 2000). Nonetheless, exceptions do occur. No “green” rods are apparent in the Japanese common newt, Cynops pyrrhogaster, but the SWS2 gene has been identified in this species (Takahashi et al., 2001). Also, a violet-sensitive SWS1 photopigment has been identified in Xenopus laevis (Hunt et al., 2007). The proportions of each class of opsin and their photoreceptors vary in the retinas as measured in X. laevis (Röhlich and Szél, 2000). The greatest percentage (up to 53% in X. laevis.) of the retina is made up of rods, with 97–98% being “red” rods and a much lower proportion of “green” rods. The proportion of LWS single and double cone photoreceptors is greater than SWS2 “green” rods and individual SWS1 single cones being the fewest found (Röhlich and Szél, 2000).
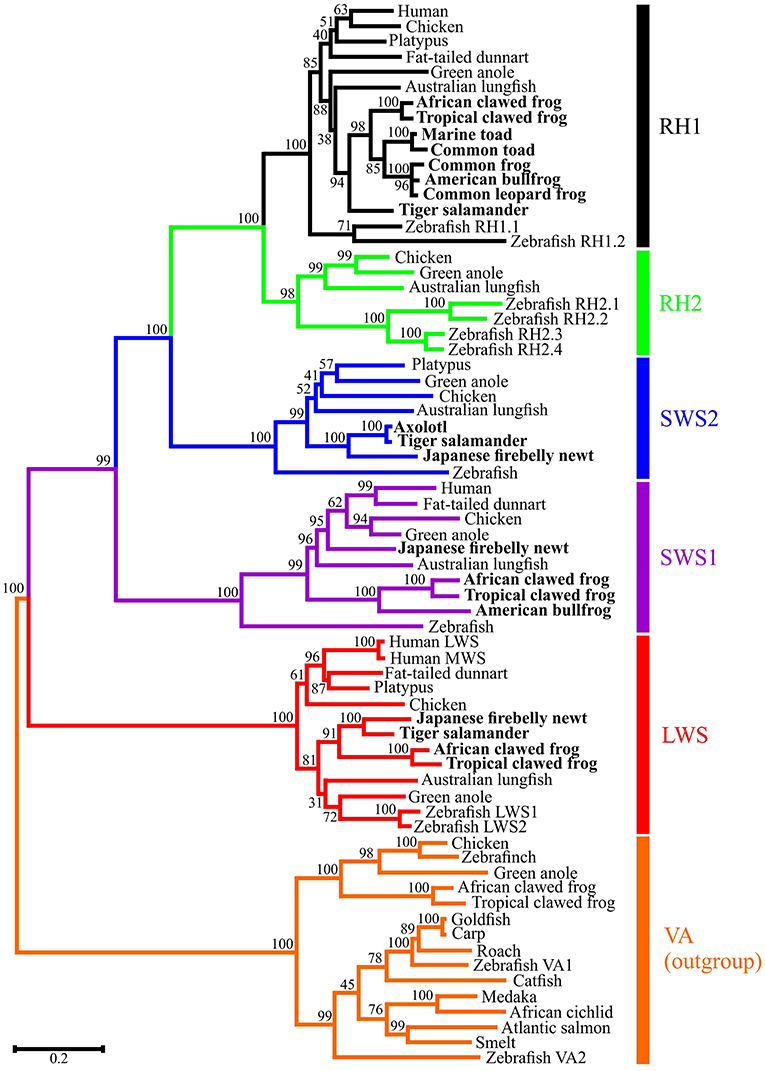
Figure 2. Molecular phylogenetic analysis by Maximum Likelihood (ML) method. The evolutionary history was inferred by using the ML method based on the General Time Reversible (GTR) model (Nei and Kumar, 2000). The tree with the highest log likelihood (-35193.70) is shown. The percentage of trees in which the associated taxa clustered together is shown next to the branches. Initial trees for the heuristic search were obtained automatically by applying Neighbor-Join and BioNJ algorithms to a matrix of pairwise distances estimated using the Maximum Composite Likelihood (MCL) approach, and then selecting the topology with superior log likelihood value. A discrete Gamma distribution was used to model evolutionary rate differences among sites [5 categories (+G, parameter = 0.9332)]. The rate variation model allowed for some sites to be evolutionarily invariable ([+I], 11.19% sites). The tree is drawn to scale, with branch lengths measured in the number of substitutions per site. The analysis involved 69 nucleotide sequences. Codon positions included were 1st+2nd+3rd+Noncoding. All positions with less than 95% site coverage were eliminated. That is, fewer than 5% alignment gaps, missing data, and ambiguous bases were allowed at any position. There were a total of 942 positions in the final dataset. Evolutionary analyses were conducted in MEGA7 (Kumar et al., 2016).
Rods are more sensitive to light compared to cones, but a higher visual acuity is achieved with cones (de Vries, 1943). Rods are thought to be younger in an evolutionary sense than cones (Collin et al., 2003; Pisani et al., 2006; Larhammar et al., 2009) with a possible intermediate type or a cone photoreceptor cell being the ancestral type (Collin et al., 2003). Thus, rods evolved later as a means of extending the daily activity of vertebrates into twilight and/or the night. This transition of a cone into a rod (the so-called “transmutation hypothesis”), first developed by Walls in 1943, is supported by studies on nocturnal geckoes with rod-based retinas that identified two types of opsin genes expressed (Kojima et al., 1992).
Only a single spectral class of photoreceptor, rods expressing the RH1 gene, have been found across the Gymnophiona, with no porphyropsins detected. Full-length RH1 sequences from three caecilian species, namely Geotrypetes seraphini, Typhlonectes natans, and Rhinatrema bivittatum, were isolated with mean maximum absorbances between 487 and 489 nm (Mohun et al., 2010) (Table 1). Typically, the spectral peak values of RH1-based visual photopigments from the rods of Amphibia ranges from 502 to 506 nm (Liebman and Entine, 1968; Hárosi, 1975; Partridge et al., 1992; Chen et al., 1996; Fyhrquist et al., 1998; Ala-Laurila et al., 2002) (Table 1). This obvious spectral shift to shorter wavelengths has been linked to shade in tropical forests and possibly twilight activity. To date, no cone opsin classes or cone photoreceptors have been found in caecilians (Mohun et al., 2010), thus is it proposed that these species have a rod-only retina, a conclusion that agrees with earlier work (Wake, 1985; Himstedt, 1995; Mohun et al., 2010; Mohun and Wilkinson, 2015). As such, the caecilian rod visual photopigment may be blue-shifted to match light levels during twilight (with a subsequent loss of cone types) and/or for the reduction of noise at low light levels.
Color Vision and Sensitivity
Kojima et al. (2017) and Yovanovich et al. (2017) recently hypothesized that the SWS2 cone opsin found in “green” rods may be used for scotopic vision. In the frogs investigated, the SWS2-expressing rods were likely to be used in scotopic vision, whereas in salamanders they exhibit a higher thermal isomerization similar to other vertebrate cone pigments, so were not likely to be used for scotopic vision (Kojima et al., 2017). Further elucidation of rod and cone associations has shown frogs to have an absence of improved color vision in dim light, but may help provide mesopic color vision in these species. Yovanovich et al. (2017) conducted behavioral experiments with two species of frog, Rana temporaria and Bufo bufo, from the Ranidae and Bufoidae families, respectively, at different light intensities to determine their color discrimination thresholds. The authors found that the ranid frogs could distinguish blue from green light down to the absolute visual threshold, whereas vision relied solely on signals from rod photoreceptors. The maximum sensitivities measured in B. bufo differed according to mate choice or prey-catching tasks, suggesting that in these behavioral contexts the differential sensitivities of spectrally distinct cone types, as well as task-specific factors, set limitations for the use of color. Thus in B. bufo there was no indication of rod-based color discrimination. This suggested the involvement of different pathways in the analysis of color vision for each task depending on ecological relevance (Yovanovich et al., 2017).
de Vries (1943) proposed that the shift in maximum sensitivity of the eye toward the shorter wavelengths of the visual spectrum and at low levels of illumination may have evolved as a means of obtaining a more favorable signal-to-noise ratio, thereby greatly increasing sensitivity of the eye to light. Such a shift should be accompanied by decreased thermal instability of the photochemical, thus producing the more favorable ratio. The noise hypothesis is based on the fact that, due to thermally induced isomerizations, visual pigments produce a “dark” noise signal (Archer, 1999). These random occurrences set a lower photon sensitivity limit below which the “light” signal cannot be distinguished from “dark” noise. Visual pigments that absorb at shorter wavelengths are predicted to be less disposed toward thermal noise and may form the basis for increasing sensitivity at lower light and higher temperatures (Shichida and Matsuyama, 2009).
Thermally induced noise was shown to decrease with shorter wavelengths, whilst porphyropsins were also noisier than rhodopsins (Donner et al., 1990). Porphyropsins, which have λmax values shifted toward longer wavelengths compared to rhodopsins for any given opsin class (Crescitelli, 1972), can occur in amphibians, which appears to vary in proportion depending on ontogeny. Larger proportions of porphyropsins are found in larval stages (Crescitelli, 1958; Wilt, 1959; Reuter, 1969). However, during metamorphosis in Rana temporaria the relative amount of porphyropsins decreases until the photoreceptors contained mostly rhodopsins (Reuter, 1969). By contrast, the paedomorphic mudpuppy, Necturus sp., which reaches sexual maturity whilst retaining larval characteristics, expresses mainly porphyropsins in the retina (Brown et al., 1963). There were contradicting reports over whether Xenopus laevis possess porphyropsins throughout the lifecycle or whether rhodopsins are present in the larval forms (Wald, 1960; Crescitelli, 1973); however, the consensus is that only porphyropsins are present (Bridges et al., 1977; Witkovsky, 2000). Bufo bufo, by contrast, expresses mostly rhodopsins in the larval tadpole phase, which are retained into adulthood (Muntz and Reuter, 1966).
Non-visual Light Detection
Other structures than the visual apparatus of the eye are used for light detection. As well as the eye (e.g., in the retinal ganglion cells expressing melanopsin), the pineal complex, the deep brain, the Harderian gland and the skin participate in photoperiodic perception (Avivi et al., 2004). Indeed, melanopsin [one of the first non-visual pigments identified and known to mediate photoentrainment (Davies et al., 2010, 2014; Hankins et al., 2014)] was first identified in the melanophores of the skin in Xenopus laevis (Provencio et al., 1998). The pineal gland of adult caecilians is described to be similar to that of a juvenile salamander (Leclercq et al., 1996). Furthermore, there is some behavioral evidence of light detection in the skin of caecilians (Ross, 1959). Ichthyophis glutinosus show negative phototaxis when light is shone on the tail: in a typical photoresponse, after several seconds of latency, skin illumination is followed by locomotive action. In addition, early behavioral studies show that some lower vertebrates, including amphibians, respond to skin illumination (Steven, 1963; Ronan and Bodznick, 1991). For example, dermal melanophores of the frog Xenopus laevis, like retinal photoreceptors, are photosensitive (Provencio et al., 1998), where the melanosomes move in response to illumination to the cell margin in vitro (Provencio et al., 1998), a phenomenon reviewed recently in Kelley and Davies (2016).
The opsins associated with non-visual photoreception include melanopsin (encoded by the OPN4 gene), vertebrate ancient (VA) opsin, pineal or pinopsin, parapineal opsin, teleost multiple tissue (TMT) opsin, neuropsin (encoded by the OPN5 gene) and panopsin (encephalopsin) amongst others (Kusakabe et al., 2001; Terakita, 2005; Davies et al., 2015). These opsins play a role in non-spatial directional (non-image-forming) vision (Land and Nilsson, 2002) or in non-visual photoreception (e.g., circadian photoentrainment) (Davies et al., 2010, 2014; Hankins et al., 2014). As well as the discovery of OPN4 expression in the skin of frogs (Provencio et al., 1998), including two different isoforms (Xenopus-like melanopsin, OPN4X, and mammalian-like melanopsin, OPN4M, Bellingham et al., 2006), OPN5 has been found in the deep brain of the Xenopus laevis tadpole (Currie et al., 2016). More recently, a number of novel atypical non-visual opsins, namely OPN6, OPN7 and OPN8 (as well as the more typical visual and non-visual opsin genes) were also discovered in the genome of Xenopus tropicalis (Davies et al., 2015).
Recently, it has been suggested that the lateral line of amphibians (in Xenopus laevis) may be light sensitive as cells surrounding the central pore of the lateral line express melanopsin (Baker et al., 2015). As such, the authors suggest that the predominantly mechanoreceptive lateral line may also detect light. Lateral line systems have been observed in all three amphibian orders. For example, salamanders have electroreceptive ampullary organs and double or triple rows of mechanoreceptive neuromast organs; caecilians have ampullary and single rows of neuromasts; frogs have been described as having single rows of neuromasts dividing into transverse planes to form secondary neuromasts, but they do not possess ampullary organs; (Lannoo, 2009). However, there is no evidence of a lateral line system in viviparous caecilians within the Caeciliidae, Scolecomorphidae and Typhlonectidae (Himstedt and Simon, 1995). Thus far, only a single publication has linked light detection to the lateral line system in amphibians (Baker et al., 2015), but such associations have been discovered in lower vertebrates such as lampreys (Ronan and Bodznick, 1991; Deliagina et al., 1995). As such, this is an interesting area that requires far more scientific attention.
Concluding Remarks
In amphibians, diversity in the visual system appears to reflect the various habitats, behaviors, life-cycles and animal coloration (Rudh and Qvarnström, 2013). However, a possible limitation in these herpetological studies is the availability of diverse material, which if addressed could lead to an improvement in amphibian husbandry and the understanding of how light plays a critical role in survival.
To appreciate the evolution of eye degeneration in amphibians, and in particular the caecilians and cave salamanders, it is critical that the cellular and molecular mechanisms of the rudimentation process are studied in these species. It would be interesting to discover if visual, non-visual and developmental genes, as well as the mechanisms involved in vision degeneration as found in other organisms are broadly similar in all three amphibian orders. Such gene losses and rudimentation mechanisms have been previously studied extensively in Astyanax sp. (Yamamoto and Jeffery, 2000; Jeffery, 2001) and Phreatichthys andruzzii cavefishes (Friedrich, 2013; Rohner, 2018), and the blind mole rat Spalax ehlenbergi using behavioral, molecular and physiological methods (Peichl, 2005). As such, they pose as potential candidate genes to continue these studies in Amphibia.
Author Contributions
SM and WD have provided substantial contributions to the conception or design of this work and provided approval for publication of the content. SM composed the initial drafts and both WD and SM revised it critically for important intellectual content.
Funding
This work was supported by the Australian Research Council (ARC) via a Future Fellowship (FT110100176) and a Discovery Project grant (DP140102117) awarded to WD and a BBSRC studentship (BBS/S/K/2003/10085) to SM.
Conflict of Interest Statement
The authors declare that the research was conducted in the absence of any commercial or financial relationships that could be construed as a potential conflict of interest.
References
Ala-Laurila, P., Saarinen, P. I. A., Albert, R., Koskelainen, A. R. I., and Donner, K. (2002). Temperature effects on spectral properties of red and green rods in toad retina. Vis. Neurosci. 19, 781–792. doi: 10.1017/S0952523802196088
Archer, S. N. (1999). “Visual pigments and photoreception,” in Adaptive Mechanisms in the Ecology of Vision, eds S. N. Archer, M. B. A. Djamgoz, E. R. Loew, J. C. Partridge, and S. Vallerga (Dordrecht: Springer Netherlands). doi: 10.1007/978-94-017-0619-3
Avivi, A., Oster, H., Joel, A., Beiles, A., Albrecht, U., and Nevo, E. (2004). Circadian genes in a blind subterranean mammal III: molecular cloning and circadian regulation of cryptochrome genes in the blind subterranean mole rat, Spalax ehrenbergi superspecies. J. Biol. Rhythms 19, 22–34. doi: 10.1177/0748730403260622
Baker, G. E., de Grip, W. J., Turton, M., Wagner, H. J., Foster, R. G., and Douglas, R. H. (2015). Light sensitivity in a vertebrate mechanoreceptor? J. Exp. Biol. 218, 2826–2829. doi: 10.1242/jeb.125203
Batni, S., Scalzetti, L., Moody, S. A., and Knox, B. E. (1996). Characterization of the Xenopus rhodopsin gene. J. Biol. Chem. 271, 3179–3186. doi: 10.1074/jbc.271.6.3179
Bellingham, J., Chaurasia, S. S., Melyan, Z., Liu, C., Cameron, M. A., Tarttelin, E. E., et al. (2006). Evolution of melanopsin photoreceptors: discovery and characterization of a new melanopsin in nonmammalian vertebrates. PLoS Biol. 4:e254. doi: 10.1371/journal.pbio.0040254
Billo, R., and Wake, M. H. (1987). Tentacle development in Dermophis mexicanus (Amphibia, Gymnophiona) with an hypothesis of tentacle origin. J. Morphol. 192, 101–111. doi: 10.1002/jmor.1051920203
Bowmaker, J. K., and Hunt, D. M. (2006). Evolution of vertebrate visual pigments. Curr. Biol. 16, R484–R489. doi: 10.1016/j.cub.2006.06.016
Bridges, C. D., Hollyfield, J. G., Witkovsky, P., and Gallin, E. (1977). The visual pigment and vitamin A of Xenopus laevis embryos, larvae and adults. Exp. Eye Res. 24, 7–13. doi: 10.1016/0014-4835(77)90279-2
Brown, P. K., Gibbons, I. R., and Wald, G. (1963). The visual cells and visual pigment of the mudpuppy, necturus. J. Cell Biol. 19, 79–106. doi: 10.1083/jcb.19.1.79
Chen, N., Ma, J. X., Corson, D. W., Hazard, E. S., and Crouch, R. K. (1996). Molecular cloning of a rhodopsin gene from salamander rods. Invest. Ophthalmol. Vis. Sci. 37, 1907–1913.
Collin, S. P., Knight, M. A., Davies, W. L., Potter, I. C., Hunt, D. M., and Trezise, A. E. (2003). Ancient colour vision: multiple opsin genes in the ancestral vertebrates. Curr. Biol. 13:R864–R865. doi: 10.1016/j.cub.2003.10.044
Crescitelli, F. (1958). The natural history of visual pigments. Ann. N. Y. Acad. Sci. 74, 230–255. doi: 10.1111/j.1749-6632.1958.tb39548.x
Crescitelli, F. (1972). “The visual cells and visual pigments of the vertebrate eye,” in Photochemistry of Vision Handbook of Sensory Physiology, ed H. J. A. Dartnall (Berlin, Heidelberg: Springer Berlin Heidelberg), 245–363. doi: 10.1007/978-3-642-65066-6_8
Crescitelli, F. (1973). The visual pigment system of Xenopus laevis: tadpoles and adults. Vision Res. 13, 855–865. doi: 10.1016/0042-6989(73)90048-5
Cuny, R., and Malacinski, G. (1986). Axolotl retina and lens development: mutual tissue. J. Embryol. Exp. Morphol. 96, 151–170.
Currie, S. P., Doherty, G. H., and Sillar, K. T. (2016). Deep-brain photoreception links luminance detection to motor output in Xenopus frog tadpoles. Proc. Natl. Acad. Sci. U.S.A. 113, 6053–6058. doi: 10.1073/pnas.1515516113
Darden, A. G., Wu, B. X., Znoiko, S. L., Hazard, E. S. III., Kono, M., Crouch, R. K., et al. (2003). A novel Xenopus SWS2, P434 visual pigment: structure, cellular location, and spectral analyses. Mol. Vis. 9, 191–199.
Dartnall, H. J. (1967). The visual pigment of the green rods. Vis. Res. 7, 1–16. doi: 10.1016/0042-6989(67)90022-3
Davies, W. I., Collin, S. P., and Hunt, D. M. (2012). Molecular ecology and adaptation of visual photopigments in craniates. Mol. Ecol. 21, 3121–3158. doi: 10.1111/j.1365-294X.2012.05617.x
Davies, W. I., Tamai, T. K., Zheng, L., Fu, J. K., Rihel, J., Foster, R. G., et al. (2015). An extended family of novel vertebrate photopigments is widely expressed and displays a diversity of function. Genome Res. 25, 1666–1679. doi: 10.1101/gr.189886.115
Davies, W. I. L., Foster, R. G., and Hankins, M. W. (2014). “The evolution and function of melanopsin in craniates,” in Evolution of Visual and Non-Visual Pigments, eds D. M. Hunt, M. W. Hankins, S. P. Collin, and N. J. Marshall (Boston, MA: Springer), 23–63. doi: 10.1007/978-1-4614-4355-1_2
Davies, W. L., Hankins, M. W., and Foster, R. G. (2010). Vertebrate ancient opsin and melanopsin: divergent irradiance detectors. Photochem. Photobiol. Sci. 9, 1444–1457. doi: 10.1039/c0pp00203h
Dawley, E. M. (1998). “Olfaction” in Amphibian Biology, eds H. Heatwole and M. Davies (Chipping Norton, NSW: Surrey Beatty & Sons, 711–742.
de Vries, H. L. (1943). The quantum character of light and its bearing upon threshold of vision, the differential sensitivity and visual acuity of the eye. Physica 10, 553–564. doi: 10.1016/S0031-8914(43)90575-0
Deliagina, T., Ullén, F., Gonzalez, M., Ehrsson, H., Orlovsky, G., and Grillner, S. (1995). Initiation of locomotion by lateral line photoreceptors in lamprey: behavioural and neurophysiological studies. J. Exp. Biol. 198, 2581–2591.
Deutschlander, M. E., and Phillips, J. B. (1995). Characterization of an ultraviolet photoreception mechanism in the retina of an amphibian, the axolotl (Ambystoma mexicanum). Neurosci. Lett. 197, 93–96. doi: 10.1016/0304-3940(95)11905-C
Donner, K., Firsov, M. L., and Govardovskii, V. I. (1990). The frequency of isomerization-like “dark” events in rhodopsin and porphyropsin rods of the bull-frog retina. J. Physiol. (Lond). 428, 673–692. doi: 10.1113/jphysiol.1990.sp018234
Donner, K., and Reuter, T. (1962). The spectral sensitivity and photopigment of the green rods in the frog's retina. Vis. Res. 2, 357–372. doi: 10.1016/0042-6989(62)90003-2
Ducey, P. K., Formanowicz, D. F., Boyet, L., Mailloux, J., and Nussbaum, R. (1993). Experimental examination of burrowing behavior in caecilians (Amphibia: Gymnophiona): effects of soil compaction on burrowing ability of four species. Herpetologica 49, 450–457.
Dünker, N. (1997). Development and organization of the retinal dopaminergic system of Ichthyophis kohtaoensis (Amphibia; Gymnophiona). Cell Tissue Res. 289, 265–274. doi: 10.1007/s004410050873
Dünker, N. (1998). Colocalization of serotonin and GABA in retinal neurons of Ichthyophis kohtaoensis (amphibia; Gymnophiona). Anat. Embryol. 197, 69–75. doi: 10.1007/s004290050120
Friedrich, M. (2013). Biological clocks and visual systems in cave-adapted animals at the dawn of speleogenomics. Integr. Comp. Biol. 53, 50–67. doi: 10.1093/icb/ict058
Frost, D. R., Grant, T., Faivovich, J., Bain, R. H., Haas, A., Haddad, C. F. B., et al. (2006). The amphibian tree of life. Bull. Am. Mus. Nat. Hist. 297, 1–291. doi: 10.1206/0003-0090(2006)297[0001:TATOL]2.0.CO;2
Fyhrquist, N., Donner, K., Hargrave, P. A., McDowell, J. H., Popp, M. P., and Smith, W. C. (1998). Rhodopsins from three frog and toad species: sequences and functional comparisons. Exp. Eye Res. 66, 295–305. doi: 10.1006/exer.1997.0430
Gower, D. J., Loader, S. P., Wilkinson, M., and Moncreiff, C. B. (2004). Niche separation and comparative abundance of Boulengerula boulengeri and Scolecomorphus vittatus (Amphibia: Gymnophiona) in East Usambara forest, Tanzania. Afr. J. Herpetol. 53, 183–190. doi: 10.1080/21564574.2004.9635510
Griisser-Cornehls, U., and Himstedt, W. (1976). “The urodele visual system,” in The Amphibian Visual System. A Multidisciplinary Approach, ed K. Fite (London: Academic Press), 203–266. doi: 10.1016/B978-0-12-257450-4.50012-4
Hankins, M. W., Davies, W. I. L., and Foster, R. G. (2014). “The Evolution of non-visual photopigments in the central nervous system of vertebrates,” in Evolution of Visual and Non-Visual Pigments, eds D. M. Hunt, M. W. Hankins, S. P. Collin, and N. J. Marshall (Boston, MA: Springer), 65–103. doi: 10.1007/978-1-4614-4355-1_3
Hárosi, F. I. (1975). Absorption spectra and linear dichroism of some amphibian photoreceptors. J. Gen. Physiol. 66, 357–382. doi: 10.1085/jgp.66.3.357
Hárosi, F. I., and Hashimoto, Y. (1983). Ultraviolet visual pigment in a vertebrate: a tetrachromatic cone system in the dace. Science 222, 1021–1023. doi: 10.1126/science.6648514
Hart, N. S., and Hunt, D. M. (2007). Avian visual pigments: characteristics, spectral tuning, and evolution. Am. Nat. 169 (Suppl. 1), S7–S26. doi: 10.1086/510141
Herrick, C. J. (1948). The Brain of the Tiger Salamander, Ambystoma tigrinum. Chicago, IL: University of Chicago Press. doi: 10.5962/bhl.title.6375
Himstedt, W. (1995). Structure and function of the eyes in the caecilian Ichthyophis kohtaoensis (Amphibia, Gymnophiona). Zoology-Jena 99, 81–94.
Himstedt, W., and Simon, D. (1995). Sensory basis of foraging behaviour in caecilians(Amphibia, Gymnophiona). Herpetol. J. 5, 266–270.
Hisatomi, O., Kayada, S., Taniguchi, Y., Kobayashi, Y., Satoh, T., and Tokunaga, F. (1998). Primary structure and characterization of a bullfrog visual pigment contained in small single cones. Comp. Biochem. Physiol. Part B Biochem. Mol. Biol. 119, 585–591. doi: 10.1016/S0305-0491(98)00032-7
Hunt, D. M., Carvalho, L. S., Cowing, J. A., Parry, J. W. L., Wilkie, S. E., Davies, W. L., et al. (2007). Spectral tuning of shortwave-sensitive visual pigments in vertebrates. Photochem. Photobiol. 83, 303–310. doi: 10.1562/2006-06-27-IR-952
Hunt, D. M., Dulai, K. S., Partridge, J. C., Cottrill, P., and Bowmaker, J. K. (2001). The molecular basis for spectral tuning of rod visual pigments in deep-sea fish. J. Exp. Biol. 204, 3333–3344.
Jeffery, W. R. (2001). Cavefish as a model system in evolutionary developmental biology. Dev. Biol. 231, 1–12. doi: 10.1006/dbio.2000.0121
Kamei, R. G., San Mauro, D., Gower, D. J., Van Bocxlaer, I., Sherratt, E., Thomas, A., et al. (2012). Discovery of a new family of amphibians from northeast India with ancient links to Africa. Proc. R. Soc. B Biol. Sci. 279, 2396–2401. doi: 10.1098/rspb.2012.0150
Keefe, J. R. (1971). The fine structure of the retina in the newt, Triturus viridescens. J. Exp. Zool. 177, 263–293. doi: 10.1002/jez.1401770302
Keller, C. B., and Shilton, C. M. (2002). The amphibian eye. Vet. Clin. North Am. Exot. Anim. Pract. 5, 261–274. doi: 10.1016/S1094-9194(01)00005-6
Kelley, J. L., and Davies, W. I. L. (2016). The biological mechanisms and behavioral functions of opsin-based light detection by the skin. Front. Ecol. Evol. 4:106. doi: 10.3389/fevo.2016.00106
Kojima, D., Okano, T., Fukada, Y., Shichida, Y., Yoshizawa, T., and Ebrey, T. G. (1992). Cone visual pigments are present in gecko rod cells. Proc. Natl. Acad. Sci. U.S.A. 89, 6841–6845. doi: 10.1073/pnas.89.15.6841
Kojima, K., Matsutani, Y., Yamashita, T., Yanagawa, M., Imamoto, Y., Yamano, Y., et al. (2017). Adaptation of cone pigments found in green rods for scotopic vision through a single amino acid mutation. Proc. Natl. Acad. Sci. U.S.A. 114, 5437–5442. doi: 10.1073/pnas.1620010114
Korenyak, D. A., and Govardovskii, V. I. (2013). Photoreceptors and visual pigments in three species of newts. J. Evol. Biochem. Physiol. 49, 399–407. doi: 10.1134/S0022093013040038
Kos, M., Bulog, B., Szel, A., and Rohlich, P. (2001). Immunocytochemical demonstration of visual pigments in the degenerate retinal and pineal photoreceptors of the blind cave salamander (Proteus anguinus). Cell Tissue Res. 303, 15–25. doi: 10.1007/s004410000298
Koskelainen, A., Hemil,ä, S., and Donner, K. (1994). Spectral sensitivities of short- and long-wavelength sensitive cone mechanisms in the frog retina. Acta Physiol. Scand. 152, 115–124. doi: 10.1111/j.1748-1716.1994.tb09790.x
Kumar, S., Stecher, G., and Tamura, K. (2016). MEGA7: molecular evolutionary genetics analysis version 7.0 for bigger datasets. Mol. Biol. Evol. 33,1870–1874. doi: 10.1093/molbev/msw054
Kusakabe, T., Kusakabe, R., Kawakami, I., Satou, Y., Satoh, N., and Tsuda, M. (2001). Ci-opsin1, a vertebrate-type opsin gene, expressed in the larval ocellus of the ascidian Ciona intestinalis. FEBS Lett. 506, 69–72. doi: 10.1016/S0014-5793(01)02877-0
Lannoo, M. J. (2009). The evolution of the amphibian lateral line system and its bearing on amphibian phylogeny. J. Zool. Syst. Evol. Res. 26, 128–134. doi: 10.1111/j.1439-0469.1988.tb00304.x
Larhammar, D., Nordström, K., and Larsson, T. A. (2009). Evolution of vertebrate rod and cone phototransduction genes. Philos. Trans. R. Soc. Lond. B Biol. Sci. 364, 2867–2880. doi: 10.1098/rstb.2009.0077
Leclercq, B., Parent, J. P., and Exbrayat, J. M. (1996). Embryonic development of pinealcomplex of Pleurodeles waltl (Amphibia, Urodela): presence of parapineal organ. Ann. Sci. Nat. Zool. Biol. Anim. 17:135.
Levine, R. P., Monroy, J. A., and Brainerd, E. L. (2004). Contribution of eye retraction to swallowing performance in the northern leopard frog, Rana pipiens. J. Exp. Biol. 207, 1361–1368. doi: 10.1242/jeb.00885
Liebman, P. A. (1972). “Microspectrophotometry of photoreceptors,” in Handbook of Sensory Physiology, Vol. VII/1. Photochemistry of Vision, ed H. J. A. Dartnall (New York, NY: Springer-Verlag), 481–528.
Liebman, P. A., and Entine, G. (1968). Visual pigments of frog and tadpole (Rana pipiens). Vision Res. 8, 761–767. doi: 10.1016/0042-6989(68)90128-4
Ma, J., Znoiko, S., Othersen, K. L., Ryan, J. C., Das, J., Isayama, T., et al. (2001b). A visual pigment expressed in both rod and cone photoreceptors. Neuron 32, 451–461. doi: 10.1016/S0896-6273(01)00482-2
Ma, J. X., Kono, M., Xu, L., Das, J., Ryan, J. C., Hazard, E. S., et al. (2001a). Salamander UV cone pigment: sequence, expression, and spectral properties. Vis. Neurosci. 18, 393–399. doi: 10.1017/S0952523801183057
MacIver, M. A., Schmitz, L., Mugan, U., Murphey, T. D., and Mobley, C. D. (2017). Massive increase in visual range preceded the origin of terrestrial vertebrates. Proc. Natl. Acad. Sci. U.S.A. 114, E2375–E2384. doi: 10.1073/pnas.1615563114
Makino, C. L., Groesbeek, M., Lugtenburg, J., and Baylor, D. A. (1999). Spectral tuning in salamander visual pigments studied with dihydroretinal chromophores. Biophys. J. 77, 1024–1035. doi: 10.1016/S0006-3495(99)76953-5
Makino-Tasaka, M., and Suzuki, T. (1984). The green rod pigment of the bullfrog, Rana catesbeiana. Vis. Res. 24, 309–322. doi: 10.1016/0042-6989(84)90056-7
Mariani, A. P. (1986). Photoreceptors of the larval tiger salamander retina. Proc. R. Soc. Lond. B Biol. Sci. 227, 483–492. doi: 10.1098/rspb.1986.0035
Matthews, G. (1983). Physiological characteristics of single green rod photoreceptors from toad retina. J. Physiol. (Lond). 342, 347–359. doi: 10.1113/jphysiol.1983.sp014855
Mohun, S. M. (2008). Rudimentary Organs and Genes: Evolution of the Visual System in Caecilians. (Dissertation/Ph.D. thesis). London: University College London.
Mohun, S. M., Davies, W. L., Bowmaker, J. K., Pisani, D., Himstedt, W., Gower, D. J., et al. (2010). Identification and characterization of visual pigments in caecilians (Amphibia: Gymnophiona), an order of limbless vertebrates with rudimentary eyes. J. Exp. Biol. 213, 3586–3592. doi: 10.1242/jeb.045914
Mohun, S. M., and Wilkinson, M. (2015). The eye of the caecilian Rhinatrema bivittatum (Amphibia:Gymnophiona: Rhinatrematidae). Acta Zool. 96, 147–153. doi: 10.1111/azo.12061
Muntz, W. R., and Reuter, T. (1966). Visual pigments and spectral sensitivity in Rana temporaria and other European tadpoles. Vis. Res. 12, 601–618. doi: 10.1016/0042-6989(66)90072-1
Nei, M., and Kumar, S. (2000). Molecular Evolution and Phylogenetics. New York, NY: Oxford University Press.
Nevo, E. (1999). Mosaic Evolution of Subterranean Mammals: Regression, Progression, and Global Convergence. New York, NY: Oxford University Press.
Nevo, E. (2007). “Mosaic evolution of subterranean mammals: tinkering, regression, progression, and global convergence,” in Subterranean Rodents, eds S. Begall, H. Burda, and C. E. Schleich (Berlin, Heidelberg: Springer), 375–388. doi: 10.1007/978-3-540-69276-8_28
Nilsson, D. E. (2017). Evolution: an irresistibly clear view of land. Curr. Biol. 27, R715–R717. doi: 10.1016/j.cub.2017.05.082
Norris, H. P., and Hughes, S. P. (1918). The cranial and anterior spinal nerves of the caecilian amphibians. J. Morphol. 31, 490–557. doi: 10.1002/jmor.1050310304
Nussbaum, R. A. (1977). “Rhinatrematidae: a new family of caecilians (Amphibia: Gymnophiona),” in Occasional Papers of the Museum of Zoology, University of Michigan, Vol. 682, 1–30.
Palczewski, K. (2006). G protein–coupled receptor rhodopsin. Annu. Rev. Biochem. 75, 743–767. doi: 10.1146/annurev.biochem.75.103004.142743
Partridge, J. C., Archer, S. N., and Vanoostrum, J. (1992). Single and multiple visual pigments in deep-sea fishes. J. Mar. Biol. Ass. 72, 113–130. doi: 10.1017/S0025315400048827
Peichl, L. (2005). Diversity of mammalian photoreceptor properties: adaptations to habitat and lifestyle? Anat. Rec. A 287A, 1001–1012. doi: 10.1002/ar.a.20262
Perry, R. J., and McNaughton, P. A. (1991). Response properties of cones from the retina of the tiger salamander. J. Physiol. 433, 561–87. doi: 10.1113/jphysiol.1991.sp018444
Pisani, D., Mohun, S. M., Harris, S. R., McInerney, J. O., and Wilkinson, M. (2006). Molecular evidence for dim-light vision in the last common ancestor of the vertebrates. Curr. Biol. 16, R318–R319. author reply: R320. doi: 10.1016/j.cub.2006.03.090
Provencio, I., Jiang, G., De Grip, W. J., Hayes, W. P., and Rollag, M. D. (1998). Melanopsin: an opsin in melanophores, brain, and eye. Proc. Natl. Acad. Sci. U.S.A. 95, 340–345. doi: 10.1073/pnas.95.1.340
Reuter, T. (1969). Visual pigments and ganglion cell activity in the retinae of tadpoles and adult frogs (Rana temporaria L.). Acta Zool. 122, 5–59.
Reyer, R. W. (1977). Morphological evidence for lens differentiation from intra-ocular implants of lens epithelium in Ambystoma maculatum. Exp. Eye Res. 24, 511–522. doi: 10.1016/0014-4835(77)90272-X
Rochon-Duvigneaud, A. (1943). Les Yeux et la Vision des Vertebres. Paris: Libraries del'Academie de Medicine.
Röhlich, P., and Szél, A. (2000). Photoreceptor cells in the Xenopus retina. Microsc. Res. Tech. 50, 327–337. doi: 10.1002/1097-0029(20000901)50:5<327::AID-JEMT2>3.0.CO;2-P
Rohner, N. (2018). Evolution: a dark past. Curr. Biol. 28, R1190–R1192. doi: 10.1016/j.cub.2018.08.045
Ronan, M., and Bodznick, D. (1991). Behavioral and neurophysiological demonstration of a lateralis skin photosensitivity in larval sea lampreys. J. Exp. Biol. 161, 97–117.
Ross, D. M. (1959). The response to light in ichthyophis (amphibia-apoda) from ceylon. Proc. Zool. Soc. Lond. 132, 83–98. doi: 10.1111/j.1469-7998.1959.tb05515.x
Roth, G., Dicke, U., and Wiggers, W. (1998). Amphibian Biology: Sensory Perception. Chipping Norton, NSW: Surrey Beatty and Sons Pty Ltd.
Rudh, A., and Qvarnström, A. (2013). Adaptive colouration in amphibians. Semin. Cell Dev. Biol. 24, 553–561. doi: 10.1016/j.semcdb.2013.05.004
Sakakibara, S., Hiramatsu, H., Takahashi, Y., Hisatomi, O., Kobayashi, Y., Sakami, S., et al. (2002). Opsin expression in adult, developing, and regenerating newt retinas. Brain Res. Mol. Brain Res. 103, 28–35. doi: 10.1016/S0169-328X(02)00164-X
San Mauro, D., Gower, D. J., Müller, H., Loader, S. P., Zardoya, R., Nussbaum, R. A., et al. (2014). Life-history evolution and mitogenomic phylogeny of caecilian amphibians. Mol. Phylogenet. Evol. 73, 177–189. doi: 10.1016/j.ympev.2014.01.009
Sherry, D. M., Bui, D. D., and Degrip, W. J. (1998). Identification and distribution of photoreceptor subtypes in the neotenic tiger salamander retina. Vis. Neurosci. 15, 1175–1187. doi: 10.1017/S0952523898156201
Shichida, Y., and Matsuyama, T. (2009). Evolution of opsins and phototransduction. Philos. Trans. R. Soc. Lond. B. Biol. Sci. 364, 2881–2895. doi: 10.1098/rstb.2009.0051
Steven, D. M. (1963). The dermal light sense. Biol. Rev. Camb. Philos. Soc. 38, 204–240. doi: 10.1111/j.1469-185X.1963.tb00783.x
Storch, V., and Welsh, U. (1973). Zur Ultrastruktur von Pigmentepithel und Photoreceptoren der Seitenaugen von Ichthyophis kohtoaensis (Gymnophiona, Amphibia). Zool. Jahrb. Abt. Anat. 90, 160–173.
Takahashi, Y., and Ebrey, T. G. (2003). Molecular basis of spectral tuning in the newt short wavelength sensitive visual pigment. Biochemistry 42, 6025–6034. doi: 10.1021/bi020629+
Takahashi, Y., Hisatomi, O., Sakakibara, S., Tsukahara, Y., Ebrey, T. G., and Tokunaga, F. (2001). Molecular cloning of the blue-sensitive opsin expressed in the Japanese commonnewt (Cynops pyrrhogaster). Invest. Ophthalmol. Vis. Sci. 42:984.
Volonteri, C., Barrasso, D. A., Cotichelli, L., Basso, N. G., and Hermida, G. N. (2017). Eye ontogeny in Pleurodema bufoninum: a comparison with Pleurodema somuncurense (Anura, Leptodactylidae). J. Morphol. 278, 896–906. doi: 10.1002/jmor.20682
Wake, M. H. (1985). The comparative morphology and evolution of the eyes of caecilians (Amphibia, Gymnophiona). Zoomorphology 105, 277–295. doi: 10.1007/BF00312059
Wake, M. H., and Dicke, R. (1998). Oviduct structure and function and reproductive modes in amphibians. J. Exp. Zool. 282, 477–506. doi: 10.1002/(SICI)1097-010X(199811/12)282:4/5<477::AID-JEZ6>3.0.CO;2
Wald, G. (1960). The significance of vertebrate metamorphosis. Circulation 21, 916–938. doi: 10.1161/01.CIR.21.5.916
Walls, G. (1942). The vertebrate eye and its adaptive radiation. Bloomfield Hills, MI: Cranbrook Institute of Science. doi: 10.5962/bhl.title.7369
Wilkinson, M., Mauro, D. S., Sherratt, E., and Gower, D. J. (2011). A nine-family classification of caecilians (Amphibia: Gymnophiona). Zootaxa. 2874. doi: 10.11646/zootaxa.2874.1.3
Wilt, F. H. (1959). The differentiation of visual pigments in metamorphosing larvae of Rana catesbeiana. Dev. Biol. 1, 199–233. doi: 10.1016/0012-1606(59)90026-0
Witkovsky, P. (2000). Photoreceptor classes and transmission at the photoreceptor synapse in the retina of the clawed frog, Xenopus laevis. Microsc. Res. Tech. 50, 338–346. doi: 10.1002/1097-0029(20000901)50:5<338::AID-JEMT3>3.0.CO;2-I
Witkovsky, P., Levine, J. S., Engbretson, G. A., Hassin, G., and MacNichol, E. F. Jr. (1981). A microspectrophotometric study of normal and artificial visual pigments in the photoreceptors of Xenopus laevis. Vis. Res. 21, 867–873. doi: 10.1016/0042-6989(81)90187-5
Yamamoto, Y., and Jeffery, W. R. (2000). Central role for the lens in cave fish eye degeneration. Science 289, 631–633. doi: 10.1126/science.289.5479.631
Yokoyama, S. (2000). Molecular evolution of vertebrate visual pigments. Prog. Retin. Eye Res. 19, 385–419. doi: 10.1016/S1350-9462(00)00002-1
Keywords: evolution, amphibian, visual ecology, eyes, visual pigment, Lissamphibia
Citation: Mohun SM and Davies WIL (2019) The Evolution of Amphibian Photoreception. Front. Ecol. Evol. 7:321. doi: 10.3389/fevo.2019.00321
Received: 24 February 2019; Accepted: 08 August 2019;
Published: 27 August 2019.
Edited by:
Chuan-Chin Chiao, National Tsing Hua University, TaiwanReviewed by:
Karen Carleton, University of Maryland, College Park, United StatesCristiano Bertolucci, University of Ferrara, Italy
Copyright © 2019 Mohun and Davies. This is an open-access article distributed under the terms of the Creative Commons Attribution License (CC BY). The use, distribution or reproduction in other forums is permitted, provided the original author(s) and the copyright owner(s) are credited and that the original publication in this journal is cited, in accordance with accepted academic practice. No use, distribution or reproduction is permitted which does not comply with these terms.
*Correspondence: Samantha Mila Mohun, sammohun@hotmail.com