- 1Department of Anthropology, Trent University, Peterborough, ON, Canada
- 2Department of Anthropology, University of British Columbia, Vancouver, BC, Canada
Stable carbon and nitrogen isotope analyses of human and animal tissues have become an important means of studying both anthropogenic and natural food webs in aquatic ecosystems. Within the rapidly expanding field of human and animal paleodietary analyses, archaeologists routinely incorporate isotopic data from fish, birds, and aquatic mammals into their interpretations of ancient freshwater resources use; however, these studies rarely consider the complex and dynamic nature of the carbon and nitrogen cycles that give structure to nutrient regimes and their isotopic compositions in freshwater ecosystems. This review outlines two thematic areas in which this surge in stable isotope applications to the study of ancient human societies could be enhanced by incorporating concepts from limnology, ecology, and biology. First, building on studies conducted in modern ecosystems, this paper outlines key aspects of the stable isotope ecology of freshwater environments, highlighting the importance of considering physical and biological processes associated with ancient biogeochemical cycles when conducting human paleodietary reconstructions. Second, this paper discusses areas where isotopic analyses of archaeological freshwater animal remains could contribute to broader research fields including climate change and cultural eutrophication research, human impacts on long-term food web dynamics and animal behavior, and by providing novel approaches to reconstructing ancient fish management practices.
Introduction
Drawing on research from ecology, biology, and limnology, this paper outlines some of the complexities of freshwater ecosystem biogeochemistry in order to address key areas of investigation in archaeology, including prehistoric human aquatic resource use and anthropogenic and natural changes in past aquatic ecosystems. Typically, stable carbon (δ13C) and nitrogen (δ15N) isotopic compositions of human, plant, and animal tissues are expected to reflect broadly predictable patterns according to factors such as trophic level, photosynthetic pathway, and environmental conditions (for review see Lee-Thorp, 2008). However, variation in other biogeochemical processes can also have a strong influence over patterning of the natural abundances of 13C and 15N in aquatic, and especially freshwater, environments. Because these biogeochemical processes often play an important role in structuring the isotopic composition of aquatic food webs at a range of spatial and temporal scales, it is important that they are adequately considered in isotopic studies of past human and animal populations.
Despite substantial growth in the use of isotopic techniques over the past 40, and especially the last 10, years (Figure 1), relatively little archaeological attention has been directed at understanding processes that give rise to isotopic variation in past aquatic ecosystems. While archaeological studies routinely consider the potential importance of aquatic resources in ancient human diets, most base interpretations on a narrow set of fundamental principles, established in the 1980s (e.g., Tauber, 1981; Chisholm et al., 1982; Schoeninger et al., 1983; Schoeninger and DeNiro, 1984), when framing interpretations of isotopic variation within aquatic ecosystems. In this context, archaeologists can overlook the considerable recent progress and insights that have been gained from studies of modern ecosystems, which provide important understanding of how biogeochemical processes structure isotopic variation in aquatic food webs. These advances demonstrate how the physical and biological conditions that are responsible for creating isotopic patterning in an average aquatic ecosystem are more complex and varied than is typically acknowledged. Therefore, wider appreciation of the biogeochemical processes operating on freshwater environments has substantial potential to: (1) improve the accuracy of archaeological interpretations of ancient human diets; and (2) facilitate new research into past subsistence technologies, seasonality, and long-term human impacts on aquatic environments.
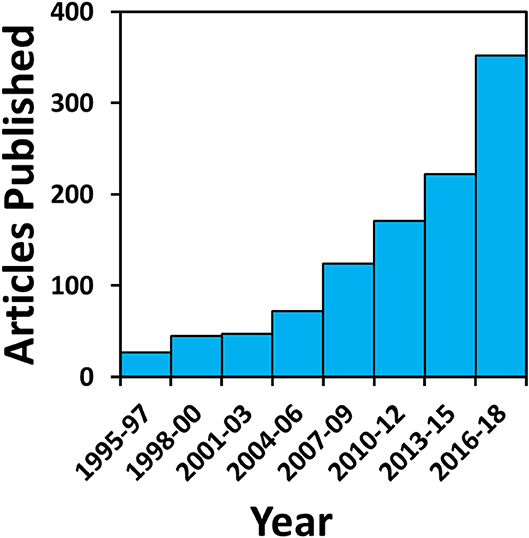
Figure 1. Growth in archaeology publications with isotope analyses. Data generated using key word searches in the Journal of Archaeological Science (JAS) and Journal of Archaeological Science Reports.
This paper synthesizes some of the advances from recent field and experimental research on freshwater environments, focusing on several areas that have direct relevance to understanding past diets, subsistence economies, and paleoenvironmental change. The goal of this paper is not to provide a review of aquatic isotope ecology, but rather to highlight some of the value that is offered by a more comprehensive consideration of the complexities of freshwater biogeochemical processes—especially those factors that can impact the isotopic compositions of aquatic resources that are commonly analyzed by archaeologists and paleoecologists (e.g., fish, bird, and mammal bone collagen). More broadly, it is hoped that this discussion of potentially productive areas of future study may help to encourage more focused research on the dynamics of the relationship between humans and freshwater environments in the past, and serve as a guide for the prioritizing of this kind of work.
Freshwater Ecosystem Isotope Ecology for Archaeological Diet Reconstructions
In recent years, some of the most dramatic growth in the archaeological sciences has been in applications of stable carbon and nitrogen isotope analyses to collagen from human and animal bones and teeth for the purpose of assessing past human diet (Figure 1). Such applications have revealed significant insights into the evolution of hominine diets, the shift from reliance on hunting and gathering to agriculture, as well as previously unknowable details about the social and economic importance of certain kinds of foods. The distinctive stable carbon isotopic compositions of C3 and C4 plants (O'Leary, 1981), for instance, have allowed researchers to track the spread and impact of maize in the Americas (Vogel and Van Der Merwe, 1977; Schwarcz, 2006) and millet in Asia (Lightfoot et al., 2013). A stepwise enrichment of 15N between trophic levels (DeNiro and Epstein, 1981; Minagawa and Wada, 1984) has enabled researchers to explore ancient weaning practices at a global scale (Fogel et al., 1989; Fuller et al., 2006; Tsutaya and Yoneda, 2015) and to ascertain the relative importance of meat in early human and Neanderthal diets (Bocherens et al., 1991; Bocherens, 2009). In coastal areas of Europe, differences in both δ13C and δ15N between broadly marine and terrestrial diets (Schoeninger et al., 1983) has provided a framework for tracking the nature and pace of the switch from Mesolithic to Neolithic subsistence practices (Tauber, 1981; Schulting, 2011).
A perennial issue shared by many of these studies has been determining the presence and relative importance of aquatic, and particularly freshwater, resources (e.g., Dufour et al., 1999; Katzenberg and Weber, 1999; Hedges and Reynard, 2007; Naito et al., 2016). Archaeologists often attribute higher δ15N values in human bone collagen to consumption of aquatic resources (Schoeninger et al., 1983) because fish are generally assumed to be a higher trophic level dietary item relative to terrestrial foods. When these higher δ15N values are associated with higher and lower δ13C values, a marine or freshwater origin for fish, respectively, is typically assumed (e.g., Müldner and Richards, 2005; Richards et al., 2015; Ottalagano and Loponte, 2017; Eriksson et al., 2018). Recent advances in the fields of aquatic ecology, biology, and limnology suggest this framework for interpreting aquatic resource use in the ancient past may, in some cases, be too general and does not account for new complexities. For example, freshwater resources—fish and the basal food web that support them—can have a wider range of isotopic compositions than is typically anticipated (Figure 2). Much of this research has been driven by improved understandings of the biogeochemical processes that operate when carbon and nitrogen are routed to and cycled through aquatic ecosystems. This section will review factors relevant to understanding stable isotope variation in freshwater aquatic food webs for the purpose of reconstructing ancient human diets, as schematized in Figure 3. While the carbon and nitrogen cycles in aquatic ecosystems are linked in many ways, as integral elements of a broader set of nutrient dynamics, for our purposes they will be treated separately in order to more clearly outline the factors relevant to interpreting human and animal bone collagen δ13C and δ15N.
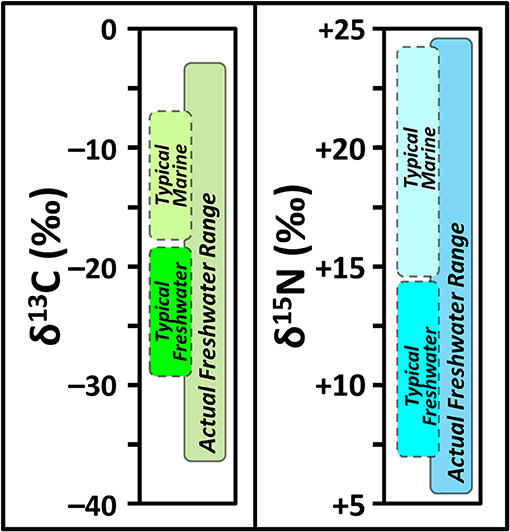
Figure 2. Generalized schematic showing the range of δ13C (left) and δ15N (right) that is typically anticipated for fish bone collagen during archaeological paleodietary interpretations compared to the ranges observed more broadly for fish collagen across the ecological literature (e.g., Dufour et al., 1999; Electronic Supplementary Information 1; Miller et al., 2010; Guiry et al., submitted).
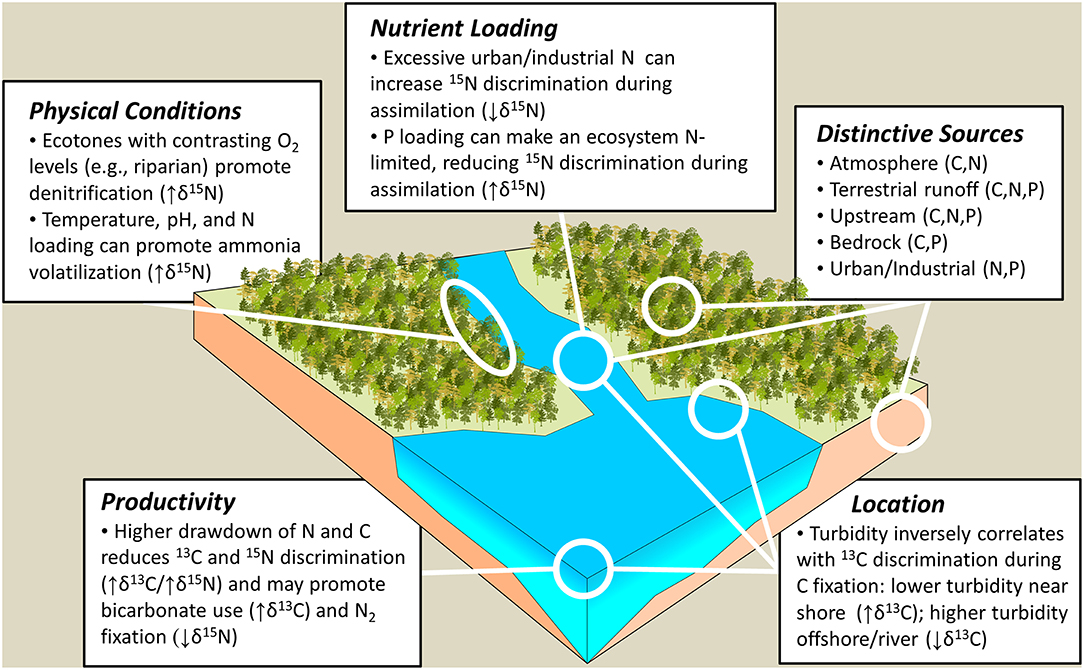
Figure 3. Schematized landscape overview highlighting areas and processes where stable carbon and nitrogen isotope fractionations relevant to archaeological research occur.
Bone Collagen Considerations
Differences Between Bone Collagen and Other Tissues
Collagen from bones and teeth is typically the only protein that is abundantly available for archaeological analyses and, for this reason, its compositional and diagenetic properties have received considerable attention (DeNiro, 1985; Brown et al., 1988; Szpak, 2011; Guiry et al., 2016c; Szpak et al., 2017). Considering the unique growth properties of bone collagen, it may seem unreasonable that some of the following sections focus discussion on factors that cause isotopic shifts in freshwater resources on a sub annual or seasonal basis. This is because bone collagen undergoes a very slow remodeling1 process over the life of an individual (Stenhouse and Baxter, 1979; Hobson and Clark, 1992; Hedges et al., 2007). Bone collagen isotopic compositions, therefore, typically reflect a longer-term, multiyear average perspective on an organism's dietary intake and will not provide a sensitive record of sub annual isotopic shifts. In contrast, other tissues like muscle, skin, blood, and liver typically remodel at much faster rate and have isotopic compositions reflecting an animal's diet over of period of the last few of weeks or months. It is essential to bear in mind that it is these soft tissues, and not bone collagen, which form the basis of human diet. In this context, short term fluctuations in the isotopic compositions of nutrients at the base of a freshwater food web can have a strong impact on the isotopic compositions of the edible portion of an animal that will not necessarily be detected in that animal's bone collagen (e.g., Hobson and Clark, 1992; Dalerum and Angerbjörn, 2005; Guiry et al., 2016a). In other words, seasonal shifts in the isotopic compositions of nutrient pools in aquatic ecosystems can generate more pronounced oscillations in human diet than would be expected based on faunal bone collagen isotopic baselines. For this reason, while the importance of shorter term seasonal variation in the isotopic composition of a food web may seem muted or less significant from the perspective of isotopic variation in archaeological bone collagen, it could play a very important role in altering the isotopic composition of human diets.
Analytical Approaches
Although a broad range of isotopic techniques are now applied in archaeology, the vast majority of this research focuses on stable carbon and nitrogen isotope analyses of bulk bone protein (“collagen”). However, techniques for compound-specific isotope analysis of amino acids (CSIA-AA) can offer significant advantages over the traditional analyses of bulk collagen (McMahon and McCarthy, 2016; Ohkouchi et al., 2017; Ishikawa, 2018). Amino acids are the building blocks from which collagen is formed and, because some AAs are hardley metabolized (“source” AAs), while others may be fully or partly metabolized (“trophic” AAs), comparing isotopic compositions of different AAs can allow for more robust and straightforward assessment of trophic position (McClelland and Montoya, 2002; Chikaraishi et al., 2009; O'Connell and Collins, 2018; although, for recent methodological considerations see Naito et al., 2018). Moreover, because AAs from different environmental sources can have distinctive isotopic compositions, CSIA-AA can help distinguish between potential source pools for carbon and nitrogen in aquatic environments (McMahon et al., 2010; Larsen et al., 2013; Ishikawa, 2018). While most archaeological research continues to focus on isotopic analyses of bulk collagen, this review also highlights some of the ways in which CSIA-AA analyses can help to improve paleoecological and paleodietary reconstructions.
δ13C Variation in Freshwater Environments
Freshwater carbon cycling is complex and can give rise to a much wider range of isotopic compositions in freshwater food webs (Finlay and Kendall, 2007; Ishikawa et al., 2012) than is acknowledged in the archaeological literature (Figure 3). With respect to aquatic foods, archaeologists generally consider the stable carbon isotopic compositions of archaeological bone collagen as an indicator for the extent to which human or animal diets focused on marine (higher δ13C) or freshwater and terrestrial (lower δ13C) food sources (Tauber, 1981; Chisholm et al., 1982). It is clear from ecological research, however, that freshwater species relevant to the interpretation of prehistoric subsistence regimes can have δ13C values spanning an enormous range (e.g., ~30‰; see Figure 2) and are not only extremely variable but also highly context dependent (Finlay and Kendall, 2007). This hyper-variability could be viewed as a major challenge for archaeologists grappling with stable isotope-based dietary interpretations in contexts where freshwater resources are potentially relevant to ancient subsistence practices (Dufour et al., 1999); or, armed with a clearer understanding of the primary axes along which δ13C varies within freshwater environments, they could be seen as an opportunity for providing a richer understanding of paleoenvironmental conditions and the ways in which human subsistence practices have been adapted to them (e.g., Brugam et al., 2016; Guiry et al., 2016b; Häberle et al., 2016). This section will outline some of the key factors underlying δ13C variation in freshwater resources including: resource origin (offshore-nearshore), carbon source (atmospheric, biological, and geological), and the environmental conditions for, and biology of, primary producers and fish.
Nearshore and Offshore Environments
A key axis along which δ13C varies in freshwater food webs is whether the relevant species have predominantly lived in offshore (pelagic) or nearshore (littoral) environments (Vander Zanden et al., 2011; Figure 4). This is because the stable carbon isotopic compositions of primary producers (e.g., phytoplankton, periphyton, marcophytes) at the base of aquatic food webs are sensitive to water movement (France, 1995). Although photosynthetic processes carried out by primary producers follow a C3 pathway (and therefore can discriminate strongly against 13C), there are large systematic differences in the stable carbon isotope fractionation exhibited by phytoplankton and periphyton associated with different levels of water turbidity (France, 1995; Finlay and Kendall, 2007). Larger isotopic fractionations discriminating against 13C (approaching that of terrestrial C3 plants) occurs during photosynthesis in faster moving water, when the fixable dissolved inorganic carbon (DIC; usually CO2 or ) in the boundary layer of water around cells is replenished more rapidly than in stiller or slower moving (lentic) waters (Hecky and Hesslein, 1995). For this reason, primary production, and the food webs this supports, in lentic waters of littoral environments, can have much higher δ13C values than in faster moving open pelagic water areas. Likewise in river settings, the faster moving (lotic) waters of riverine ecosystems can give rise to a food web with especially low δ13C values (Finlay et al., 1999; Finlay, 2004). A key distinction to bear in mind is that the variation in freshwater resource δ13C arising from differences in water turbidity originates in the photic zone, where primary producers are fixing carbon. The association between benthic (bottom) environments and slower moving waters has sometimes led to an incorrect anticipation of higher δ13C values for deep water ground dwelling fish taxa (Drucker et al., 2016). In contrast to freshwater resources from benthic areas of nearshore environments, which can have characteristically high δ13C values, freshwater fish inhabiting deeper offshore benthic (profundal) regions, where carbon must be sourced from materials settling out from overlying pelagic photic zone will have lower δ13C values.
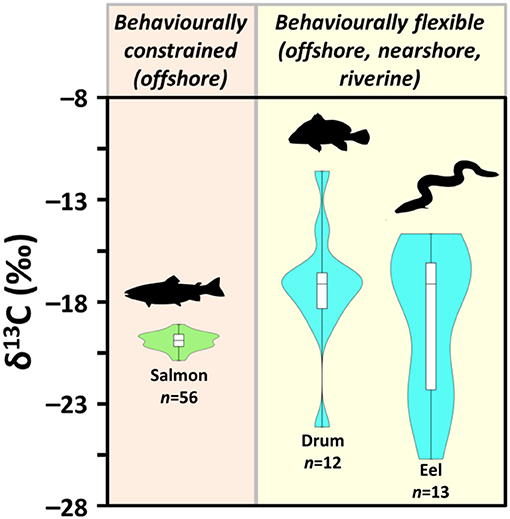
Figure 4. Violin and box plot of δ13C for fish : freshwater resident Atlantic salmon (Salmo salar), freshwater drum (Aplodinotus grunniens), and American eel (Anguilla rostrata)—from archaeological sites (1000–1800 CE) near Lake Ontario (data from van der Merwe et al., 2003; Guiry et al., 2016b; Hammersley, 2016; see Electronic Supplementary Information 1).
It is important for archaeologists to adequately consider the scale of δ13C variation in freshwater food webs related to the offshore-nearshore continuum. For example, human dietary contributions from freshwater resources that come from littoral benthic areas could contribute protein sources that have highly elevated δ13C values, overlapping with what archaeologists would typically associate with marine food webs (see Figure 2). Moreover, given the behavioral plasticity of many archaeologically prevalent fish species (e.g., Catostomidae, Esocidae, Ictaluridae, Percidae, Salmonidae), it is not uncommon for a large range of δ13C values to occur within a single species across a given catchment (e.g., Zambrano et al., 2010; Zhang et al., 2012) (certainly within the range of hunter-fisher-gatherer groups' mobility) based on differing habitat preferences and other factors such as age-related behavioral changes and seasonal movements. Figure 4, for example, compares archaeological bone collagen δ13C for taxa in a single catchment and shows how, relative to more behaviorally constrained species (freshwater resident Atlantic salmon), fish that can utilize a wider variety of environments (freshwater drum and American eels) exhibit more extreme isotopic variation. While this level of complexity may complicate paleodietary reconstructions, particularly in contexts where humans could have exploited littoral fish taxa, it could also provide a more nuanced understanding of fishing practices and paleoecology (see section Food Web Dynamics and Trophic Structure and Prehistoric Trade and Fishing Technology below).
Variation in Carbon Sources
Shifts in the carbon sources that are used by primary producers can also cause large changes in the stable carbon isotopic composition of a food web at a variety of temporal and spatial scales, especially in river environments where conditions can change rapidly (Finlay, 2004). For this reason, changes in the relative importance of different carbon sources can lead to considerable variation in δ13C values for freshwater resources from the same region depending on season and local environmental conditions (Hodell and Schelske, 1998). For example, Figure 5 illustrates how δ13C of collagen from the same species, at precisely the same life stage (Atlantic salmon smolts), can vary significantly between different rivers due in part to differing δ13C for carbon sources between catchments.
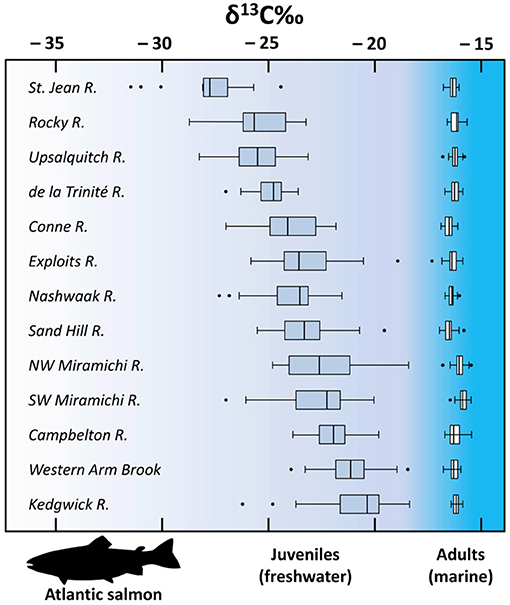
Figure 5. Box and dot plots showing mean δ13C for Atlantic salmon scale collagen from freshwater juveniles (left) and adults (right; after first winter at sea) that are departing and returning to their natal rivers, respectively, across eastern Canada between 2009 and 2010. For all samples n = 30, except for de la Trinité (22 juveniles; 19 adults), Exploits (21 adults), NW Miramichi (29 adults). Note the contrast between the extreme range of variation for smolts and how, after 1 year at sea, adults of the same population become comparatively homogenous. Data from Dixon et al. (2012).
There are three major carbon sources for freshwater primary producers. The first two are atmospheric and respired CO22, which have isotopic compositions similar to the atmosphere (δ13C = ~−8‰) and local organic matter, respectively. With respect to local organic matter, if the respired CO2 is derived from the metabolism of detritus originating from within the local aquatic ecosystem (autochthonous), the δ13C of new CO2 will be similar to that of the local food web. If the respired CO2 derives from metabolism of organic materials that have been washed in from adjacent terrestrial environments (allochthonous), the δ13C of newly respired CO2 may be highly 13C depleted (widely ranging but δ13C = ~−28‰ on average), particularly in regions where terrestrial photosynthesis is C3 dominated (Finlay, 2001). The relative importance of carbon from allochthonous sources may be particularly important for smaller lakes (Post, 2002; Bade et al., 2004). The stable carbon isotopic compositions of freshwater food webs, especially in rivers, may also be affected by CO2 contributions coming directly from terrestrial sources, which can be rich in DIC derived from degraded organic carbon (Jones and Mulholland, 1998; Jones et al., 1998; Cole et al., 2007). In general, broadly distinctive isotopic compositions for autochthonous vs. allochthonous carbon sources has provided ecologists with an important means of tracing dominant energy pathways in freshwater environments (Carpenter et al., 2005; Finlay and Kendall, 2007). Recent advances in CSIA-AA of modern fish have also demonstrated a capacity for assessing the relative importance of these two sources (McMahon et al., 2010; Larsen et al., 2013) and may therefore provide an means of assessing this type of δ13C variation in archaeological contexts. The third source of carbon in aquatic food webs is bicarbonate () (Maberly et al., 1992; Raven et al., 2002), which has δ13C values that are usually 7–10‰ higher than most CO2 sources (δ13C typically between −15 and −5‰) (Mook et al., 1974), reflecting a mixing of carbon sources during its formation through dissolution of carbonate minerals (δ13C = 0‰) (Craig, 1953) by carbonic acid (H2CO3) derived from CO2 (Mook and Tan, 1991; Kendall et al., 1992; Finlay and Kendall, 2007).
The dominant carbon source for primary producers depends on a range of factors but one of the most impactful of these are pH changes (Finlay and Kendall, 2007), which can promote carbonate dissolution, particularly in limestone-rich topographies. Decreasing pH, which may be driven by a variety of other factors (see section Alkaline Conditions), can release greater quantities of an alternate isotopically distinctive DIC source that is readily utilized by a variety of primary producers, especially if CO2 is in short supply and/or the primary producer growth rates are high (Fry and Sherr, 1989; Fogel and Cifuentes, 1993; Laws et al., 1995). Figure 6 illustrates how this can produce large positive shifts in the δ13C of freshwater primary producers, during summer months when CO2 supply is low for Lake Ontario, Canada, which is situated in a limestone-rich region and experiences substantial seasonal carbonate flux (Hodell and Schelske, 1998).
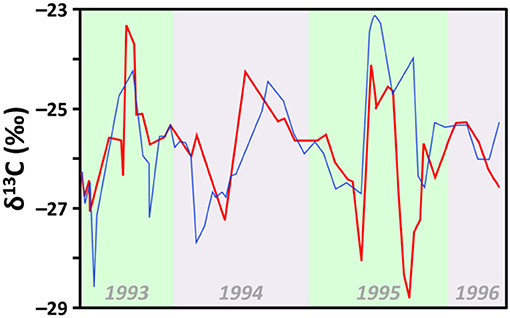
Figure 6. Seasonal fluctuation of organic matter δ13C from two locations (red and blue lines) collected biweekly during a 3 year period in Lake Ontario, Canada. Seasonal fluctuations reflect annual shifts in productivity that influence δ13C by: (1) drawing down CO2, which (2) promotes use of HCO−3 during C fixation. Data from Hodell and Schelske (1998).
For archaeologists, considering the relative importance of different potential DIC sources for the aquatic cycling of carbon in a local study region could help to anticipate whether, in general, freshwater food web stable carbon isotopic compositions are likely to fall at the higher or lower end of the δ13C continuum (Figures 2, 5). Moreover, the magnitude and intensity of carbon source shifts can vary at the multiannual scale and can change through time (Roussel et al., 2014). For this reason, greater isotopic variation that may otherwise be interpreted as shifts between freshwater, marine, and terrestrial resource use in the past, could be common in more chemically complex aquatic environments. Indeed, where carbonate sources are abundant such as the limestone regions of Europe, North America, and Asia, food web-wide increases in δ13C are possible (Zohary et al., 1994; Hodell and Schelske, 1998; Wang et al., 2013). This should be particularly important for archaeological interpretations in regions that are more geologically and ecologically diverse, such as those where prehistoric diets could incorporate freshwater resources from multiple watersheds with different carbon sources. It is therefore important to pay close attention when generating faunal dietary isotopic baselines and to be sure, where possible, to include a geographically representative sample of potential variation in freshwater resource origins. Shifting carbon sources can also have an impact on radiocarbon dating of freshwater fauna and their human consumers (i.e., by introducing old DIC into a food web; Cook et al., 2001) and therefore it could be possible to consider δ13C as an indicator for freshwater reservoir corrections when calibrating 14C dates.
Environment and Biology of Primary Production
The biology of primary producers as well as the nutritional and physical characteristics of their environment can also drive significant variation in the stable carbon isotopic composition of freshwater food webs. Unlike in terrestrial environments, which uses atmospheric CO2, the carbon pool (i.e., DIC) that is available to freshwater primary producers is limited (Talling, 1976). For this reason, factors that impact how primary producers use up (or “draw down”) the available DIC pool, relative to how quickly it is replenished (through atmospheric diffusion, cellular respiration, carbonate dissolution, and allochthonous DIC contributions) can significantly alter the extent to which discrimination against 13C occurs during carbon fixation (McKenzie, 1985; Hollander and McKenzie, 1991; Finlay and Kendall, 2007).
The two main sources of stable carbon isotope fractionation of CO2 for many freshwater primary producers are the aqueous diffusion of CO2 and the enzymatic carboxylation reaction during carbon fixation by ribulose 1,5-bisphosphate carboxylase/oxygenase (RuBisCO) (Barth et al., 1998). When there is ample CO2 available to support photosynthesis, the carboxylation of CO2 via RuBisCO strongly discriminates against heavier 13C (up to −20 to −29‰) (Wong et al., 1979; Roeske and O'Leary, 1984; Finlay, 2004), resulting in lower δ13C for primary producers and the food webs they support. However, if the CO2 pool becomes limited (e.g., as can happen seasonally during summer highs in productivity or eutrophication—see section Anthropogenic Nutrient Loading), primary producers must incorporate more of the remaining 13C-enriched CO2 during carbon fixation, significantly reducing the extent to which fractionation associated with carboxylation via RuBisCO can occur. In this context, where CO2 is limited, primary producer δ13C can become elevated because the relative importance of fractionation associated with CO2 diffusion (<1‰) (O'Leary, 1984) will increase and some primary producers may shift to using bicarbonate (Figure 6). For this reason, a variety of factors that have bearing on the efficiency of carbon fixation such as phytoplankton/periphyton community species composition (Vuori et al., 2012), size/geometry (Popp et al., 1998), and growth rate (Laws et al., 1995) can become important determinants of the δ13C of freshwater food webs. Factors that have an impact on the admixture of different carbon pools, such as the thermal and hydrological properties of a water body are also very important (see section Climate Change). For instance, the development of thermal stratification during warmer months, can effectively render large portions of the DIC pool (lower depths) inaccessible to primary producers during warmer months when growth is strongest, altering the carbon cycle on a seasonal basis and leading to changes in the isotopic composition of a food web (Hodell and Schelske, 1998).
The highly contextual nature of primary production means that the influence that factors related to the environment and biology of primary producers could have on the interpretation of archaeological δ13C values will also be context dependent and may be difficult to assess. When available, it may be possible to consider other paleoenvironmental proxy indicators from local paleolimonological studies, such as diatom species and elemental compositions as well as sedimentation rate data to help reconstruct the extent to which past productivity varied in a given research area. In any case, it is worth keeping in mind the range of biological and physical processes associated with primary producers and their environmental conditions that can help shape the stable carbon isotopic compositions of aquatic resources while interpreting isotopic values from past food webs.
Behavioral Biology of Fish
Studies of modern ecosystem relationships can inform a range of archaeological interpretations of isotopic variability associated with past freshwater resource use. Knowledge of fish behavior and growth physiology, in particular, can significantly improve interpretations by constraining the timeframes and range of environments that a species is likely to have used and thus the isotopic compositions they will have assumed. In archaeological studies, the isotopic compositions of bone collagen from migratory fishes, for example, are typically thought to reflect a mix of habitation in marine and freshwater environments and are therefore sometimes interpreted with the caveat that they represent a potential marine dietary source (e.g., Vika and Theodoropoulou, 2012; Pfeiffer et al., 2016). However, for species that spend a proportionally small period of their lives in their natal environment, studies show that it is the secondary environment (i.e., where the fish underwent most of its growth) that overwhelmingly bears out in its adult isotopic composition (Dixon et al., 2012). When these details about fish mobility and growth are coupled with information about the environment and behavior preferences for a particular taxon, some broader scale rules of interpretation may be possible.
Atlantic salmon (Salmo salar) provide a good example of how considering species' biology and behavior can improve the utility of archaeological faunal data for interpreting human diets. Anadromous Atlantic salmon, whether from Europe or North America, undergo their early growth in freshwater ecosystems before migrating to spend their adult lives feeding and growing in a handful of regions of the North Atlantic Ocean. Young salmon (smolts) departing from their natal rivers for the first time, across the North Atlantic coastal region, exhibit a high degree of variation in δ13C values due to differing local carbon sources and carbon cycling processes in their natal streams (Dixon et al., 2012). However, as highlighted in Figure 5, within 1 year of feeding at sea, the variation in δ13C of adult Atlantic salmon populations becomes significantly reduced and comparatively homogenous (Dixon et al., 2012, 2015; MacKenzie et al., 2012). In this context, it may be possible to use a relatively small sample of available archaeological Atlantic salmon bone collagen δ13C values from various sites from a particular time period as a baseline for human diet at other, more widely distributed sites. This would be useful at archaeological sites that, for one reason or another, do not have suitable faunal specimens for analyses. This could be especially useful for a species like Atlantic salmon, and possibly other salmon species, which were likely a ubiquitous and valuable subsistence resource across a large area of the world, but that may be less commonly recovered archaeologically due to taphonomic issues.
Careful consideration of species biology and behavior can also help clarify interpretations of behaviorally complex species. For instance, on the other end of the spectrum, catadromous3 fish such as eels that, whether European or North American (Anguilla rostrata or A. angilla; hereafter eel), begin their lives together in a specific area of the Atlantic Ocean (Avise, 2003), and eventually migrate back to marine, estuary, or inland freshwater rivers and lakes around the North Atlantic rim to feed and grow into adults (Tesch and White, 2008). Higher δ13C values for archaeological eel bone collagen at inland sites have been attributed to a mixed signal from diets in natal marine and adult freshwater habitats and have led to the categorization of eels as a possible marine human dietary contribution at inland sites (e.g., Pfeiffer et al., 2016). However, given that much of the growth eels undergo occurs after entering their adult environment (Tesch and White, 2008), the isotopic signal from their natal, marine ecosystem will be lost among eels that migrate back to freshwater ecosystems, as they take on the isotopic composition of their new environment during adult growth. Moreover, considering again (see section Differences Between Bone Collagen and Other Tissues) that it is primarily muscle and organ tissue that is contributing to human diet, which will more rapidly reflect a fish's migration-related dietary shifts (relative to bone collagen), there is little reason to anticipate that consumption of adult eels will provide a marine-derived carbon source for human diet. This is supported by a number of isotopic studies demonstrating that, despite the high degree of plasticity observed in eel mobility and dietary behavior, their isotopic compositions often reflect the local environment in which they were harvested (Bardonnet and Riera, 2005; Harrod et al., 2005; Cucherousset et al., 2011; Barry, 2015; Eberts et al., 2015). The behavioral flexibility and longevity of eels, particularly their capacity to use river, near-shore, and offshore environments, means that, even in freshwater environments at far inland locations, they could have δ13C values almost anywhere along the δ13C continuum for their local water body, including very high δ13C values (e.g., if specializing in littoral benthic environments). The small sample of eels (n = 13) shown in Figure 3, for example, are from archaeological sites well over 1,000 km inland, yet they exhibit a remarkable range of δ13C values spanning more than 11‰. In other words, rather than reflecting their natal marine origins, broad scale variation in the δ13C values of catadromous fishes like eels likely reflects the relative importance or availability of different kinds of freshwater habitat. While this adds a source of complexity to human dietary interpretations, it may also represent an opportunity for paleoecology or biology studies seeking to understand long term patterns in ecosystems and in species' behaviors (Robson et al., 2012) (see section Food Web Dynamics and Trophic Structure and Prehistoric Trade and Fishing Technology).
δ15N Variation in Freshwater Environments
Relative to the carbon cycle, nitrogen cycling and δ15N in freshwater ecosystems is more complex (see Electronic Supplementary Information 2; Figure 3). This is because the nitrogen cycle in freshwater environments can involve a wider range of biological and physical processes that cause isotopic fractionation (Kendall, 1998; Finlay and Kendall, 2007; Kendall et al., 2007). For most studies the δ15N of archaeological remains is primarily (often solely) used as an indicator of trophic position and is based on a well-established stepwise 3–5‰ enrichment in 15N observed between trophic levels (Post, 2002; Hedges and Reynard, 2007; Caut et al., 2009). In this context, higher δ15N values in human remains are commonly attributed to elevated reliance on aquatic resources (Hedges and Reynard, 2007) because food chains in aquatic environments can have more trophic levels than their terrestrial counterparts (up to 5 as opposed to 3, respectively). With respect to freshwater resource consumption, a common pattern for archaeological interpretations is that lower δ13C and higher δ15N values in human or animal remains is evidence for a reliance on freshwater resources such as higher trophic level fish (Bonsall et al., 1997; Cook et al., 2001; Hedges and Reynard, 2007). However, as has been outlined for δ13C values in section δ13C Variation in Freshwater Environments, freshwater resource use could incorporate foods with a wide range of isotopic compositions and it is therefore also critical to pay close attention to how δ15N varies within lake and river ecosystems. Figure 7, for example, illustrates this by showing how mean δ15N of scale collagen from adults of a single freshwater salmon species, kokanee (Oncorhynchus nerka) can vary by over 10‰ between different lakes along the western edge of North America. With respect trophic position, CSIA-AA (McClelland et al., 2003; Chikaraishi et al., 2009) can provide a powerful tool for helping to circumvent interpretive issues (by providing a baseline and trophic assessment in the same analysis), but an in-depth understanding of factors underlying variation in freshwater N cycling and δ15N may help to further enhance interpretations of archaeological human diet. Part of the reason that δ15N in freshwater ecosystems can be more variable is that there are more potential isotopically-distinct source pools of dissolved inorganic nitrogen (DIN; mainly ammonium [], nitrate [], and nitrite []) (for review see Kendall, 1998) and that aquatic nitrogen cycles are controlled by a series of bacterial processes that not only create large isotopic fractionations between steps but that are also highly sensitive to changes in local environmental conditions (for review see Electronic Supplementary Information 2). This section will review some of the primary axes along which δ15N varies in freshwater resources including: nitrogen sources, productivity, oxygenation, alkalinity, and depth.
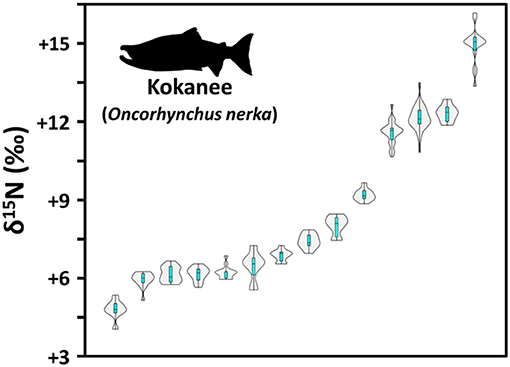
Figure 7. Violin and boxplot showing variation in δ15N between modern (1975–2015) kokanee (Oncorhynchus nerka) populations from lakes across western North America. Because data are from adults, this variation should broadly reflect environmental conditions rather than trophic level differences. From left to right: Fallen Leaf Lake, CA (n = 8); Wahleach Lake, BC (n = 15); Comox, BC (n = 20); Revelstoke, BC (n = 15); Alouette Reservoir, BC (n = 15); Kinbasket Lake, BC (n = 15); Cowichan Lake, BC (n = 9); Arrow Lake, BC (n = 16); Shuswap Lake, BC (n = 8); Tezzeron Lake, BC (n = 21); Okanagan Lake, BC (n = 25); Jo-Jo Lake, AK (n = 61); Kalamalka Lake, BC (n = 6); Lake Sammamish, WA (n = 17) (data from Guiry et al., in preparation; Overman et al., 2009; Shedd et al., 2015; Meeuwig and Peacock, 2017; see Electronic Supplementary Information 3).
Variation in Nitrogen Sources
At a basal food web level, one of the most important factors for determining δ15N in an aquatic environment is the isotopic composition of DIN sources (Gu, 2009). The δ15N values of the DIN pools available in an aquatic ecosystem will determine the starting point from which all subsequent major (bacterially mediated or from trophic enrichment) nitrogen isotope fractionations occur. The DIN pool utilized by primary producers is replenished by several sources (Kendall, 1998): (1) autochthonous sources including ammonification of local organic matter and (2) allochthonous contributions including those from terrestrial and atmospheric sources of organic or mineralized nitrogen. Ammonification is a bacterial process where organic matter (which cannot be assimilated by primary producers) is transformed into ammonium, one of a range of mineralized forms of DIN which is a vital nutrient for the growth of aquatic primary producers. Because ammonification does not strongly discriminate against 15N, this process has little impact on the broader stable nitrogen isotopic composition of aquatic food webs (Kendall, 1998) (although see section Depth). While the organic matter available for ammonification may primarily be derived from autochthonous sources (detritus of local autotrophic and heterotrophic origins), it may also originate from allochthonous sources such as forest or agricultural soils in adjacent or upstream terrestrial environments.
The second major DIN source is allochthonous nitrogen contributions—either in organic or mineralized form—derived from other environments, especially terrestrial soil nitrogen. The movement of nitrogen from agricultural and forest soils into aquatic ecosystems (see section Anthropogenic Nutrient Loading), for instance, can result in significant upward shifts in the stable nitrogen isotopic composition of entire aquatic food webs (McClelland et al., 1997) not only due to differences in the initial δ15N of transported soil nutrients (Koerner et al., 1999) but also due to bacterial and physical processing acting on soil nitrogen during transportation that causes nitrogen isotope fractionation (usually leading to 15N enrichments) (Diebel and Vander Zanden, 2009; Botrel et al., 2014; Choi et al., 2017).
Another allochthonous nitrogen source important in coastal watersheds is marine subsidies from migratory anadromous fish, which can effect a large-scale upstream importation and deposition of nitrogen from marine environments (Kline et al., 1990; Bilby et al., 1996; Chaloner et al., 2002) (this is true of carbon also Garman and Macko, 1998). The impact of this imported marine-derived nitrogen on the δ15N of the affected freshwater systems will vary based on a variety of factors including the migratory species involved and the environmental conditions under which they grew. A further important, albeit poorly understood, allochthonous nitrogen source is atmospheric deposition (Kendall, 1998; Holtgrieve et al., 2011). While most nitrogen in the atmosphere is unreactive N2, a small part consists of reactive nitrogen oxides and, especially in nitrogen-limited environments, can provide an important contribution to aquatic DIN pools (Ostrom et al., 1998). The isotopic composition of atmospheric nitrogen depends partly on meteorological conditions and can therefore be highly variable (Kendall, 1998).
Relative to carbon source variation, a larger range of nitrogen sources as well as their more variable isotopic composition means that shifting nitrogen sources can have a less predictable effect on the δ15N of freshwater resources. This complexity has led to difficulties in interpreting isotopic variation even in modern freshwater food webs where key environmental parameters are known. From an archaeological perspective, particularly where data are derived from smaller sample sets and where paleoenvironmental data for past nutrient dynamics are unknown, it is important to approach interpretations of δ15N values in the context of use of freshwater resources with caution. In this context, ongoing advances in CSIA-AA could provide additional information to help distinguish between different nitrogen sources in ancient ecosystems (Jarman et al., 2017). For instance, studies comparing AA δ15N values can help assess the relative importance allochthonous vs. autochthonous inputs for aquatic consumers' diets (Ishikawa, 2018).
Biological Productivity
The biological productivity of an ecosystem (that is, how active its primary producers are relative to available nutrient sources) can also have a large impact on the isotopic compositions of freshwater food webs, with potential to both significantly increase or decrease the δ15N values of freshwater resources. It is important to bear in mind that it is the isotopic compositions of primary producers at the base of the food web that ultimately determines the δ15N values of consumers at higher trophic levels (Casey and Post, 2011). As primary producers assimilate nitrogen, they can discriminate strongly against 15N, which can leave the remaining DIN pool and primary producers with higher and lower δ15N values, respectively—this is true whether the DIN used is ammonium or nitrate (Fogel and Cifuentes, 1993; Needoba et al., 2004; Sigman et al., 2009). However, as primary producers begin to draw down available DIN sources, the remaining DIN, and by extension the entire food web, becomes progressively enriched in 15N (Pennock et al., 1996; Finlay and Kendall, 2007; Gu, 2009). This can have a large impact on the lake food web δ15N where environmental conditions favoring primary production fluctuate on a seasonal basis. As shown in Figure 8, the amplitude of seasonal change in δ15N of lake nutrients can be very large and is positively correlated with trophic state (oligotrophic, mesotrophic, and eutrophic lakes). Because climate plays an important role in this productivity-driven oscillation in DIN δ15N, this pattern follows a latitudinal gradient, with more northerly lakes being more strongly affected (Gu, 2009).
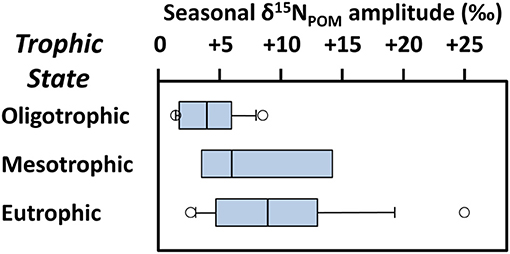
Figure 8. Lake trophic state vs. amplitude of annual shifts in δ15N of organic matter associated with seasonal change in 36 lakes: oligotrophic (n = 13), mesotrophic (n = 5), eutrophic (n = 18). Data are collated by Gu (2009).
As an ecosystem becomes increasingly nitrogen limited, bacteria capable of fixing unreactive N2 (a metabolically costly process) become proportionately more important to the ecosystem's nitrogen budget. The N2 used by nitrogen fixers will usually be atmospheric in origin4 and, because this has a δ15N of around 0‰ (Fogel and Cifuentes, 1993), nitrogen-limited freshwater food webs, such as those in eutrophic lakes (Figure 9) where nitrogen fixers have become an important nitrogen contributor can have much lower overall δ15N values (Gu et al., 1994, 1996; Gu, 2009). Across its full range, the effect of increasing productivity on the isotopic composition of freshwater resources is parabolic—from low, to high, and back to low δ15N values—and can therefore introduce significant complexities to interpretation of δ15N values in freshwater food webs where there are strong annual or inter annual shifts in productivity, such as where seasonal cycles or eutrophic conditions are present (see section Climate Change for discussion).
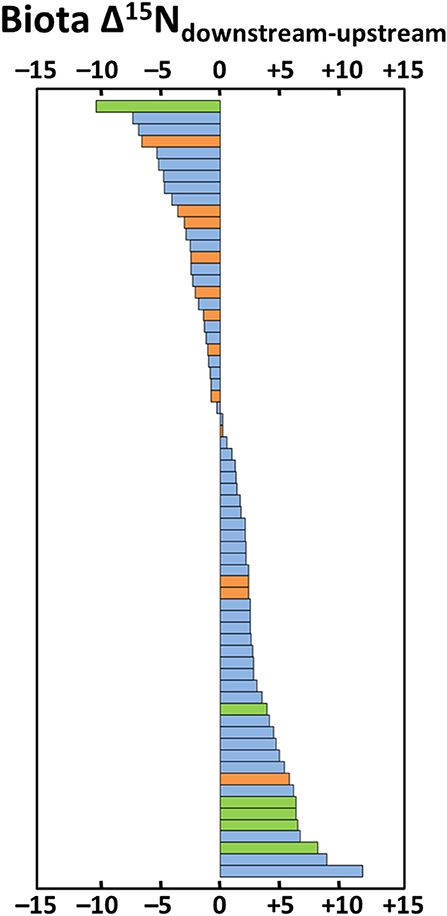
Figure 9. Effect of anthropogenic nitrogen loading on aquatic flora and fauna δ15N in rivers, including seston (green), invertebrates (blue), and fish (orange). Bars represent the difference between mean δ15N for a given biota upstream and downstream of an anthropogenic nitrogen source (e.g., urban, industrial, or agricultural effluent). Negative shifts in δ15N can occur when over-abundant DIN favors greater discrimination against 15N during assimilation by primary producers (see Electronic Supplementary Information 2; data from Harrington et al., 1998; Wayland and Hobson, 2001; DeBruyn and Rasmussen, 2002; Gustin et al., 2005; Ulseth and Hershey, 2005; Finlay and Kendall, 2007; Brown et al., 2011; di Lascio et al., 2013; Morrissey et al., 2013; Atkinson et al., 2014; Loomer et al., 2015; Robinson et al., 2016; data in Electronic Supplementary Information 4).
Oxygenation
The relative availability of oxygen (O2), a major control lever governing shifts between aerobic and anaerobic respiration, can also have a considerable impact on the prevailing nitrogen isotopic compositions of freshwater food webs (Sebilo et al., 2006; Kendall et al., 2007). This is because the presence of oxygen plays an important role in chemical transformations between most key forms of nitrogen (ammonium and nitrate) in the DIN pool, each of which can be associated with very large isotopic fractionations (Mariotti et al., 1984; Hübner, 1986; Fogel and Cifuentes, 1993; Sebilo et al., 2006). In well-oxygenated environments, through the process of nitrification, aerobic bacteria metabolize ammonium turning it into nitrate. Discrimination against heavier 15N during metabolism of ammonium can lead to increases in δ15N values of the remaining ammonium pool (Hübner, 1986; Casciotti et al., 2003). In ecosystems where there is a significant amount of nitrification and where there is an oversupply of ammonium, the δ15N of aquatic food webs can become significantly elevated (Sebilo et al., 2006) (for more on the complexities of isotopic variation in contexts where there is an oversupply of DIN see section Anthropogenic Nutrient Loading). However, nitrification typically will not have an impact on food web δ15N (Casciotti et al., 2003; Kendall et al., 2007) because in most natural environments available ammonium is usually rapidly used up5 through assimilation by primary producers, outside of special circumstance such as runoff from sewage and fertilizers.
A second process, denitrification, is often credited with causing significant changes in food web δ15N (Sebilo et al., 2006). Denitrification occurs under oxygen-poor circumstances where bacteria use oxygen from nitrate and nitrite () for cellular respiration and generate N2 in the process (Knowles, 1982). Denitrification discriminates very strongly against 15N leaving its substrate (nitrate) and product (N2) highly 15N enriched and depleted, respectively. This means that in aquatic ecosystems where nitrate forms an important part of the DIN pool for primary producers, denitrification has the potential to cause significant elevations in freshwater resource δ15N (Ogawa et al., 2001; Sebilo et al., 2003).
Because these two processes, nitrification and denitrification, are favored by opposing oxic and anoxic conditions, some of the most significant oxygenation-related impacts on δ15N in freshwater ecosystems occur along ecotones where different environmental conditions meet (Brandes and Devol, 1997; Sebilo et al., 2003; Lehmann et al., 2004; Sigman et al., 2005). This is especially the case in wetland and riparian zones where nitrate-rich surface waters can be fed into anoxic environments leading to extensive denitrification (Sebilo et al., 2003, 2006). From the perspective of prehistoric human subsistence, it is often exactly these kinds of ecotonal environments, where two ecosystems meet, that provide the some of the richest resource opportunities (Epp, 1985). It is therefore important for archaeologists to exercise caution when interpreting δ15N as evidence for freshwater or marine resource use in areas that may have been denitrification hotspots (McClain et al., 2003) in the past. The potential influence of denitrification on the isotopic composition of archaeological fauna could also provide significant opportunities for understanding paleoenvironmental change (see section Climate Change and Anthropogenic Nutrient Loading for further discussion).
Alkaline Conditions
Although rarely considered in ecology, research from limnology and geology has highlighted the potential importance of pH for influencing δ15N in some freshwater environments (Stüeken et al., 2015). The process of ammonia volatilization, where ammonium is lost from a system through degassing, is associated with extreme fractionation against 15N (Hübner, 1986; Kendall, 1998). In freshwater environments, ammonia volatilization can occur where pH is above 8.5 and can therefore influence the isotopic composition of a key portion of the DIN pool in some alkaline lakes (Talbot, 2001; Leng et al., 2006). The very large fractionation associated with volatilization should mean that even when ammonium supply is limited (as is common in many freshwater ecosystems), relatively minor occurrences of volatilization could have larger impacts on the nitrogen isotopic composition of the DIN pool and food web (Collister and Hayes, 1991; Talbot and Johannessen, 1992). Aquatic pH is, in turn, controlled by a variety of factors including temperature, biological productivity (as the main CO2 user), and local geology and therefore can vary temporally within the same aquatic system and between different water bodies in the same area. While the combination of conditions that facilitate ammonia volatilization in freshwater environments may be relatively rare, their overlap with some habitat preferences for humans (karst and high productivity area) (Lace and Mylroie, 2013) means that they may be worth considering when interpreting freshwater resource δ15N.
Depth
A positive correlation between the δ15N of DIN and depth is well-established in marine environments (Altabet, 1988) and has recently been considered as a possible explanation for δ15N patterns observed in deeper freshwater ecosystems (e.g., Kumar et al., 2011). In ocean environments, detritus settling downward over great distances in the water column provides a raw material—organic matter—for the nitrogen cycle in deeper waters via ammonification. Although ammonification is associated with only a small 15N fractionation, repeated ammonification through a downward journey of thousands of meters is thought to explain why DIN produced through ammonification of organic matter becomes successively enriched in 15N with depth (Altabet, 1988; Sigman et al., 2009). While relatively few freshwater environments reach the depths over which this kind of 15N enrichment has been observed in oceans, some limnological research has suggested that similar processes may also occur in freshwater environments (Sierszen et al., 2006). If depth related 15N enrichment does occur in deeper freshwater systems, then it is important for archaeologists to consider upwelling events (bringing 15N-enriched DIN up from below) and use of deeper water taxa as sources of δ15N variation when interpreting freshwater resource δ15N. From an archaeological perspective, this variable would apply in narrow range regions with very deep water bodies such as Lake Baikal (Yoshii, 1999) or the African Rift Lakes.
Beyond Diet
The isotopic composition of freshwater ecosystems is highly complex and can vary based on large number of interrelated factors that would make it difficult to apply universal principals or rules of interpretation during isotopic studies of the aquatic dimensions of any ancient human ecosystem. While this higher degree of potential for isotopic variability in freshwater environments often goes unrecognized in the archaeological literature, and may seem to be a drawback, especially for stable isotope approaches to understanding the role of freshwater resources in ancient human diets, it is at the same time a potential opportunity. Specifically, because this isotopic variation is directly linked with and often highly sensitive to past biological and physical conditions, the isotopic compositions of archaeological fauna and humans could provide an important indicator for changes in ancient environments—both anthropogenic and natural in origin—at a variety of spatiotemporal scales (Hebert et al., 1999; Hobson et al., 2015; Braje et al., 2017; Szpak et al., 2018; Guiry et al., submitted). In addition, by using isotopic analyses of fauna from multiple time periods to generate long-term retrospective time series for key archaeological taxa, there could be potential to use this variation as a marker not only for environmental change but also for previously invisible patterns in the development of prehistoric economies and technologies (e.g., Guiry and Gaulton, 2016). This section will review the potential of isotopic variation in prehistoric freshwater food webs to provide markers for past cultural practices and environmental conditions with an emphasis on areas with significant promise for future research.
Climate Change
Lakes and other freshwater environments are particularly sensitive to the effects of climate change (Adrian et al., 2009). Temperature plays an important role in most physical and biological processes that cause isotopic fractionation in both the carbon and nitrogen cycles. Climate change can therefore leave detectable traces in the isotopic composition of past freshwater food webs (Leng and Marshall, 2004; Leng et al., 2006). The complex and often highly interconnected responses exhibited by ecosystems to climatic variation means that resulting isotopic patterns can differ between regions and time periods. For this reason, assessing the utility of archaeological faunal isotopic data as an indicator for past climate should be context specific. Many of the processes and environmental conditions that play an important role in determining the stable carbon and nitrogen isotopic composition of a freshwater food web (such as pH, oxygenation, and nutrient sources) are impacted by climate either directly through temperature controls (e.g., diffusion rates for CO2 and O2), or indirectly through precipitation regimes, which control the supply (via transportation) and concentration (via water volume) of key nutrients (Smith and Walker, 1980; Beardall et al., 1982; Lucas, 1983). In addition to these, some of the most important climate-related factors include the effects of temperature on freshwater thermo-hydrological structure and the isotopic composition of allochthonous nutrients from terrestrial and upstream sources.
Climate can influence the isotopic composition of freshwater food webs by altering water temperature, which, in turn, plays a major role in determining the spatiotemporal distribution of nutrient pools (Schindler, 1997; Leng and Marshall, 2004). In many lakes, higher summer temperatures heat the upper water column (epilimnion), leaving the lower water column (hypolimnion) cooler. This creates a thermal boundary (thermocline) that reduces mixing of the water column, and nutrient pools, between the hypolimnion and epilimnion. Thermal barriers that isolate upper and lower strata of a water body from one another can decouple the physical and biological processes in their respective carbon and nitrogen cycles that are responsible for isotopic fractionation, allowing them to operate as semi-autonomous systems. Divergence between these systems can lead to increased heterogeneity in the isotopic compositions of nutrients available across different areas of the same water body (e.g., Hodell and Schelske, 1998) (see section Freshwater Ecosystem Isotope Ecology for Archaeological Diet Reconstructions). For instance, productivity differences can influence the relative rates of drawdown of available DIN and DIC in the separated strata, altering the net discrimination against heavier isotopes of 13C and 15N during assimilation by primary producers. DIC drawdown may have additional impacts on both δ13C and δ15N by: (1) influencing the relative availability and importance of isotopically distinctive carbon sources (e.g., switching between CO2 and ; see section Variation in Carbon Sources) for primary producers in one stratum relative to another (Schelske and Hodell, 1995; Hodell et al., 1998); a shift that (2) in turn, can also induce pH changes that can create environmental conditions fostering other processes such as ammonia volatilization (Collister and Hayes, 1991) (see section Alkaline Conditions). Likewise, reduced DIN concentration in one stratum could increase the relative importance of N-fixing cyanobacteria, thereby introducing another isotopically distinctive nitrogen source (Gu, 2009) (see section Biological Productivity). Differing rates of productivity can also cause divergence in oxygen concentrations between epilimnion and hypolimnion. When the boundary between adjacent strata becomes an interface between oxygen rich and poor systems, denitrification can have a significant effect on the isotopic composition of their respective DIN pools (Talbot and Johannessen, 1992; Ogawa et al., 2001) (see section Oxygenation). In dimitic lakes, with the onset of autumn and cooling water temperatures in the epilimnion, the thermocline breaks down causing significant turn over in the water column—mixing lower and upper strata and also stirring up bottom sediments—leading to greater overall homogeneity in the isotopic composition of DIC and DIN sources across the water body (Gu, 1993, 2009). Because the duration and intensity of this seasonal cycling depends on temperature, climate change can be associated with alternating levels of heterogeneity in the isotopic composition of freshwater food webs (e.g., Coletta et al., 2001). Although these examples occur on a seasonal basis, and the amplitude of potential oscillations in food web isotopic composition (e.g., Figures 6, 8) may be dampened when considering the stable isotopic compositions of longer lived taxa, there are still potential patterns to be found in the archaeological record. Indeed, slight variations in these parameters, for instance, shifting the onset of the breakdown of thermoclines, can have significant net impacts on prevailing isotopic compositions of whole food webs (Hodell et al., 1998) and, if repeated over a number of years as part of a climate change trend, could become visible in isotopic time series assembled from archaeological fauna (e.g., Szpak et al., 2018).
Climate can also have a significant influence of the isotopic composition of freshwater food webs by altering the transport and sourcing of nutrients from adjacent terrestrial environments (Talbot, 2001; Leng and Marshall, 2004). Precipitation and temperature play a key role in structuring terrestrial ecosystems at all scales. One important impact on the isotopic composition of terrestrial food webs can come from the influence of climate on the biological composition of plant communities in terrestrial areas of a watershed. Warmer, drier climates can favor a shift in the relative abundance of C3 and C4 plants, a change that could have significant impacts on the extent to which carbon fixation discriminates against 13C (Still et al., 2003). This may ultimately lead to increased δ13C values for respired CO2 (coming from metabolized terrestrially-derived nutrients; see section Variation in Carbon Sources) that are passed up the food web (Nordt et al., 1994; Street-Perrott et al., 2004). Likewise, similar climatic trends that cause a transition from forests to open grasslands could promote a regime shift in prevalence of different kinds of mycorrhizal-plant relationships, which play an important role in the fractionation of nitrogen isotopes in nutrients taken up by terrestrial plants, potentially resulting in higher δ15N for nitrogenous nutrients (Szpak et al., 2014; Guiry et al., 2018) that are transported to adjacent aquatic ecosystems. Another important impact can come more directly from the influence of temperature and aridity on terrestrial nitrogen-cycle openness with warmer conditions potentially favoring higher levels of denitrification and ammonia volatilization (Austin and Vitousek, 1998; Handley et al., 1999; Szpak, 2014). Warmer conditions can also promote denitrification in aquatic ecosystems (Veraart et al., 2011) potentially directly leading to increases in the δ15N of aquatic DIN pools as well. In addition to altering the isotopic composition of terrestrial nutrient sources, climate can influence the relative amount and frequency of nutrient delivery. Climatic conditions, including the intensity and periodicity of major storms, droughts, and freeze/thaw events can cause large increases or decreases in the amount of nutrient loading to aquatic systems through erosion of terrestrial soils, thereby changing the relative balance of carbon and nitrogen sources for adjacent aquatic ecosystems between autochthonous and allochthonous origins (Jansson et al., 2000; Dunnette et al., 2014).
Climate change is widely acknowledged as an urgent and globally-relevant environmental issue and there is widespread interest in assessing how past fluctuations in climate have affected human culture and human ecosystems (e.g., Bellard et al., 2012; Wheeler and Von Braun, 2013). The many ways in which changing temperature and precipitation can affect the isotopic composition of aquatic nutrients, and the food webs that depend on them, can create opportunities for using archaeological materials to investigate past climate change (e.g., Szpak et al., 2018). This kind of research has been a staple in paleolimnological studies, which use isotopic analyses of sediments in concert with other proxies to investigate long-term patterns in climate change (Meyers and Lallier-Vergès, 1999; Leng and Marshall, 2004). In comparison with sediments, archaeological fauna record environmental change from a different ecological perspective and data interpretation are subject to a different set of strengths and weaknesses, making them potentially complementary (Guiry et al., submitted). For instance, variability associated with short term intra-annual oscillations in environmental conditions, sediment mixing, and diagenesis that may complicate interpretations of time series composed from sediment isotopic compositions (Lehmann et al., 2002; Lu et al., 2014) may not present a significant issue when analyzing bone collagen isotopic compositions (which provide an inter-annual average) from longer-lived fauna—a quality which can lend a degree of temporal stability to a data set (Guiry et al., 2018). In this context, the prospect for exploring past climate change through isotopic analyses of time series of aquatic fauna, such as fish and birds from archaeological sources, is worthy of closer consideration.
Anthropogenic Nutrient Loading
When an overabundance of nutrients are supplied to an aquatic environment, freshwater lakes, and rivers undergo eutrophication6, a process that dramatically alters an ecosystem's structure (Smith, 2003; Conley et al., 2009). The kinds of nutrient loading7 that cause eutrophication can also significantly alter the isotopic composition of aquatic food webs (Cabana and Rasmussen, 1996) in two important ways. First, the new nutrients can have a different isotopic composition relative to the previous nutrient regime (Vander Zanden et al., 2005). As shown in Figure 9, human activities can contribute DIN from diverse sources resulting in aquatic food webs with a very large range of isotopic compositions. One major source includes agricultural fertilizers, which are transported via run off to adjacent aquatic ecosystems (Lake et al., 2001; Cole et al., 2004; Anderson and Cabana, 2005, 2006; Diebel and Vander Zanden, 2009; Atkinson et al., 2014). Animal based fertilizers can have a wide range of δ15N values, depending on trophic position and digestive physiology of the animal, but will generally contribute nitrogen that has an elevated δ15N relative to DIN in an aquatic ecosystem (Simpson et al., 1997; Szpak, 2014; Choi et al., 2017). Modern synthetic fertilizers, on the other hand, are produced from atmospheric N2 and therefore contribute nitrogen with a δ15N that is often lower than aquatic DIN (Choi et al., 2017). Another major source relevant to the more ancient past, particularly in urban areas, is sewage and other human waste dumping (e.g., McClelland et al., 1997). The isotopic composition of human waste can be highly variable and studies of modern ecosystems have found that net decreases and increases in δ15N in different environments are possible (DeBruyn and Rasmussen, 2002; Botrel et al., 2014). In addition to the elevated initial isotopic compositions of anthropogenic nitrogen sources, the δ15N of primary producers, and foodwebs that depend on them, can be further altered through a wide range of physical and biological processes. The labile nature of important forms of nitrogenous nutrients (e.g., ammonium and nitrate) in fertilizers and sewage make them susceptible to denitrification and ammonia volatilization processes that act to further concentrate 15N in anthropogenic nitrogen sources (Szpak, 2014; Choi et al., 2017), driving foodweb δ15N higher (see sections Oxygenation and Alkaline Conditions). An overabundance of DIN can also have the opposite effect. Figure 9 highlights the potential for decreasing δ15N in biota affected by anthropogenic nitrogen loading. Under some circumstances (particularly where excessive supplies of nutrients pass through an ecosystem quickly and where the ecosystem is phosphorous-limited rather than nitrogen-limited) an oversupply of DIN can drive food web δ15N downward by favoring greater discrimination against 15N during assimilation by primary producers (DeBruyn and Rasmussen, 2002; di Lascio et al., 2013).
A less obvious but nonetheless important source of anthropogenic nutrient loading is through soil erosion. Human land management practices, especially deforestation and the intensive agricultural activities that can follow (such as stump removal and plowing) may destabilize soils and lead to large scale losses of soil nutrients through runoff (Sudduth et al., 2013) which can in turn alter the stable nitrogen isotopic composition of adjacent aquatic ecosystems (Vandermyde and Whitledge, 2008; Massa et al., 2012; Theissen et al., 2012; Yao and Xue, 2015). Prior to and during transport from terrestrial to aquatic ecosystems, soil nutrients could pass through a range of biological and physical processes that can cause isotopic fractionation (Hogberg, 1997; Hobbie and Ouimette, 2009; Nikolenko et al., 2018). For instance, intensive agricultural land use, especially where fertilizers are applied, can promote volatilization of ammonia (Ruess and McNaughton, 1988; Szpak, 2014; Choi et al., 2017). Movement through wetlands, riparian zones, and ground water can channel nitrate through oxic-to-anoxic transition interfaces that encourage denitrification (Knowles, 1982; Sebilo et al., 2003; Seitzinger et al., 2006) (see sections Oxygenation and Alkaline Conditions). It is not surprising, therefore, that numerous studies of aquatic ecosystems near agricultural land have observed a higher δ15N in local food webs (e.g., Harrington et al., 1998; Hebert and Wassenaar, 2001; Kendall et al., 2007; Diebel and Vander Zanden, 2009; Kohzu et al., 2009; Morrissey et al., 2013; Santoro et al., 2014; Loomer et al., 2015).
The second major way in which nutrient loading can alter the isotopic composition of aquatic food webs is by changing productivity. This is because elevated primary production, spurred by nutrient loading, can alter the physical and biological processes underlying key sources of isotopic fractionation within the carbon and nitrogen cycles (e.g., Hodell and Schelske, 1998; Hodell et al., 1998) (see sections Environment and Biology of Primary Production and Biological Productivity). The main nutrients limiting productivity in many freshwater environments are phosphorous and nitrogen (Elser et al., 1990, 2007). In phosphorous limited systems, nitrogen cannot be fully utilized (Downing and McCauley, 1992). This ensures that DIN assimilation by primary producers will be incomplete and will therefore lead to some degree of discrimination against 15N (Figure 9). However, many human activities generate phosphorous excesses and when this results in phosphorous overloading in a freshwater environment, the system shifts to being nitrogen limited, thereby encouraging the more intensive drawdown of available DIN. When DIN is more fully utilized, the discrimination against 15N associated with assimilation by primary producers will not occur, thereby driving up food web δ15N (Hodell and Schelske, 1998). However, in some environments, when nitrogen limitation is strong, N-fixing bacteria can become an important part of the nitrogen budget for freshwater ecosystems, driving δ15N back down (Gu, 2009) (see section Biological Productivity). Elevated productivity levels will also impact δ13C values, by increasing drawdown of DIC (reducing discrimination against 13C; see section Environment and Biology of Primary Production), which can also facilitate shifts between use of distinct carbon sources (from CO2 to ; see section Variation in Carbon Sources), and by shifting photic conditions in the littoral water column to favor pelagic instead of benthic primary production (Vadeboncoeur et al., 2003).
Over the last century it has become increasingly evident that the activities of modern societies are responsible for a global surge in eutrophication events (Smith, 2003; Conley et al., 2009; Ishimaru et al., 2011), yet relatively little is known about when and how humans became the primary drivers of eutrophication in the more distant past. The isotopic compositions of aquatic fauna provide an excellent indicator not only for the presence of excess anthropogenic nutrient loading but can also give information about the effects of these human impacts on the ecology of important species. What's more, archaeological fauna provide a unique window into past ecosystems prior to onset of the Anthropocene and the advent of industrial processes (Lyman, 1996, 2006, 2012; Lyman and Cannon, 2004; Humphries and Winemiller, 2009; Wolverton and Lyman, 2012; Rick and Lockwood, 2013). Isotopic baselines generated through retrospective analyses of historical collections as well as sediment cores are increasingly used to understand human impacts on environments (Solomon et al., 2008; Jeffers et al., 2015). However, because historical collections rarely predate the 19th C, when many human impacts were already well-established (Jeffers et al., 2015), these baselines often lack the kind of long-term hindsight needed to generate appropriate frames of reference for gauging the scale of change that humans have caused in the modern era. In this context, archaeological fauna offer a temporal depth that is usually not available in ecology (Barak et al., 2016). They also offer a valuable alternative to analyses of sediment records because they are not subject to the same limitations (e.g., robust diagenesis detection protocols and accurate data on species and trophic position).
Food Web Dynamics and Trophic Structure
The ecological consequences of human modifications to aquatic environments are a topic of broad significance in ecology and biology. Overfishing, alien species introductions, and hydrological controls, to name but a few factors, have caused drastic changes to food web interactions at a global scale (Halpern et al., 2008; Dodds et al., 2013). Following human population growth and industrial innovations, overexploitation of aquatic resources has occurred in many of the world's freshwater environments (Dudgeon et al., 2006), a process that can leave detectable traces in the isotopic composition of an aquatic food web (e.g., Wainright et al., 1993). For instance, when exploitation reduces the prevalence of a species, the niche that was occupied by that species may be fully or partly vacated, changing food web dynamics (Giller, 1984; Hamilton et al., 2014). Retrospective studies, for instance, using isotopic analyses of collagen from archived fish scales have shown how reduced niche breadth following overexploitation of fish species can result in more homogenous food web isotopic compositions (e.g., Blanke et al., 2018). Aquatic resource exploitation that targets a group of species at a specific trophic level can also have ecosystem-wide consequences. For instance, removal of lower trophic level species such as forage fish from an environment can effectively shorten the entire food chain. This has been observed in the Pacific Ocean using isotopic analyses of archaeological sea bird remains that show an inverse relationship between δ15N, as a measure of food chain length, and intensity of commercial fish harvesting through time (Wiley et al., 2013; Hobson et al., 2015; Ostrom et al., 2017). Alternatively, removal of apex predators that have played a key role in regulating prey population sizes at lower trophic levels can, in turn, lead to a trophic cascade, a chain reaction of shifting behavior throughout the rest of the food web (Pace et al., 1999). Szpak and colleagues provide an excellent example of this, using archaeological rockfish (Sebastes spp.) remains from the Pacific Ocean, demonstrating how the over hunting of otters (Enhydra lutris) allowed their urchin (Strongylocentrotus spp) prey to proliferate, eventually leading to the collapse of entire kelp forest ecosystems along North America's western coast (Szpak et al., 2013).
Adding new species to an ecosystem can have as much impact on the ecology and isotopic composition of native fauna as species' removals (Sagouis et al., 2015). The introduction of a new species to an ecosystem can force other taxa to vacate a niche for which they are no longer best adapted (Vander Zanden et al., 1999). Recent ecological studies (Rennie et al., 2013; Colborne et al., 2016; Fera et al., 2017) of fish scales from the Great Lakes and elsewhere have, for instance, effectively investigated the impacts of introduced species like the round goby (Neogobius melanostomus) and zebra mussels (Dreissena polymorpha) on major energy flow pathways, the decoupling of benthic and pelagic ecosystems, and lake-wide trophic state shifts. Human-mediated transplantation, intentional or unintentional, of aquatic species has a deep history dating back millennia (Nakajima et al., 2010; Haidvogl et al., 2015). Although this kind of research question has not yet been approached using isotopic analyses of archaeological remains, there could be substantial potential to reconstruct the impacts of ancient or early historical species' transplantations on relatively “pristine,” or pre-human, ecosystems.
Increasingly, retrospective stable isotope analyses of collagen from fish scales and keratin from bird feathers are being used to assess the impacts that human exploitation of aquatic environments have had on aquatic food web structures in the last several decades. These studies have been instrumental not only in identifying the effects of human freshwater resource exploitation but also for evaluation of the impacts of management policies which have been set in place to mitigate previous environmental deterioration (Zanden et al., 2003; Hebert et al., 2008; Nestler et al., 2011; Turner et al., 2015). Archaeological fish, bird, and mammal bones could provide an opportunity to assess the impacts of prehistoric freshwater resource exploitation on aquatic food webs as well as provide longer term baselines for present-day species behaviors (Barak et al., 2016). What's more, archaeological remains can provide a window on to the dietary and mobility behavior of extinct and extirpated populations (Humphries and Winemiller, 2009; Haidvogl et al., 2015; Naito et al., 2015) providing opportunities to address other questions relevant to species and ecosystem rehabilitation efforts.
Prehistoric Trade and Fishing Technology
Fishing technologies, and the accumulated ecological knowledge bases that have enabled effective exploitation of diverse aquatic resources, are integral to understanding the development and trajectory of human culture in many areas of the world (Erlandson, 2001). Fishing technologies are particularly important because they provide the platform that facilitates or constrains access to specific pools of freshwater resources (Cleland, 1982; Allen, 1992). From the perspective of ancient human diets, use of different fishing tools such as spears, tridents, weirs, gillnets, or seines, for instance, will each provide a distinctive freshwater resource profile (e.g., Colley, 1987; Needs-Howarth and Thomas, 1998; Losey et al., 2008; Hawkins and Caley, 2012). Likewise, knowledge of the habitat preferences and reproductive behaviors of key species enabled their more effective exploitation. The isotopic composition of fish consumed at a given archaeological site could provide details about the nature of the specific fish populations that people were accessing (Katzenberg and Weber, 1999; Barrett et al., 2008; Guiry et al., 2016b) and this information could, in turn, reveal clues about the fishing technologies and ecological knowledge employed in prehistoric subsistence practices (Figure 10). This section outlines some of the axes along which the isotopic composition of aquatic resources can vary in relation to the technologies and knowledge bases employed in fish catching and other aquatic resource exploitation.
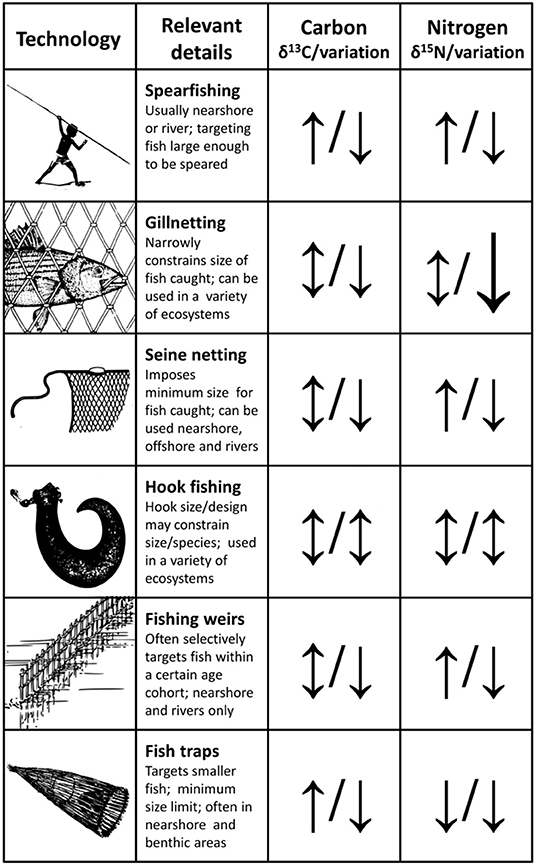
Figure 10. Examples of freshwater fishing technologies and implications of their use for the isotopic compositions of fish caught. These are generalizations and, in all cases, the direction and intensity of trends will vary based on a wide range of contextual variables including where, when, and how technologies were deployed as well as whether a particular species or age cohort was targeted.
Information about the location in which fish have been caught in the ancient past can help identify not only the technologies used but also the season of capture. Fish bone collagen δ13C can provide a useful indicator for the whether a fish had inhabited nearshore or offshore environments (see section Nearshore and Offshore Environments). For instance, depending on the species and context, lower fish δ13C values, pointing to offshore habitat use, could help provide details about the use of watercraft or specialized practices such as ice fishing. Alternatively, this may help build a case for targeting of spawning runs when fish annually return to the nearshore shallows or tributaries to spawn. While some species may be specialists occupying niches that are specific to either nearshore or offshore habitats, many are more flexible and have niches that can span the nearshore-to-offshore continuum (see Figure 4; Beaudoin et al., 1999; Vander Zanden and Vadeboncoeur, 2002). For these taxa, δ13C values could provide important clues for the specific kinds of fishing practices employed by prehistoric populations in a given environment. In this context, higher and lower fish δ13C values are indicative of catching fish that used nearshore and offshore habitats, respectively. There is also potential for identifying the importance of river-caught taxa. In rivers, faster moving waters, in addition to increased terrestrial carbon subsidies, can lead to freshwater riverine resources that have δ13C values that are significantly lower than even obligatorily offshore taxa.
Variation in fish δ15N may also be able to help reveal aspects about the manner in which they were caught. Some fishing technologies, such as gillnets and seines, are size selective. Gillnets, for instance, catch a narrow size range of fish, while seines impose a minimum size limit. By reducing the variation in catch size for fish, these technologies can influence the amount of isotopic variation that would be expected in a particular catch. While this could influence both carbon and nitrogen isotopic compositions of fish caught (e.g., Zhang et al., 2012), it could have a particularly strong impact on the amount of variation seen in fish δ15N. Because size plays an important role in limiting the types of foods that a fish can consume (especially mouth size) it is closely associated with trophic position (Werner and Gilliam, 1984; Scharf et al., 2000). For this reason, as gillnets target fish within a narrower size cohort, gillnetting should result in more homogenous fish δ15N values. In contrast, fish caught using seines would have more isotopic variation, reflecting less size-discrimination in catches. Traditional ecological knowledge could also influence variability in the isotopic composition of fish catches. Where fish with specific qualities have been selectively taken, either using technologies that enable targeting of individual fish (e.g., tridents and spearing), or where only choice fish from a catch have been kept, the isotopic composition of archaeological fish assemblages could be constrained. For instance, if the criteria by which fish have been selected gave preference to particular qualities such as size, sex, or weight, the isotopic composition of a group of archaeological fish should become more homogenous. While zooarchaeological analyses are also well-suited to reconstructing catch size profiles, particularly when larger quantities of fish head bones are preserved, there are many cases where this will not be possible using traditional osteological analyses, such as when most fish bones recovered archaeologically are from vertebrae. In these cases, particularly when combined with aDNA techniques (Royle et al., 2018), isotopic analyses of archaeological fish have significant potential to reveal ancient fisheries management practices such as whether fish of a particular sex and size cohort were targeted (Royle et al., in review).
It could also be possible to explore the ancient trade of fish products using isotopic analyses of archaeological fish remains. The isotopic composition of some economically important fish populations may be distinctive within a regional context. For instance, fish of the same species and behavioral type that live in separate lakes or rivers, with different factors dominating their respective carbon and nitrogen cycles, could have markedly different isotopic compositions (see Figures 5, 7). If these isotopic differences between populations can provide a regionally unique marker for a fish's lake of origin, they may be useful as an indicator of trade or transport of fish from their native context. However, the suitability of a species for this type of inference depends on their behavioral ecology (see Figure 4). Fish that can adapt their behavior to exploit food sources from a wide range of habitats would not be suitable because they also would have a wide range of potential isotopic compositions within the same lake ecosystem. Fish that are highly specialized feeders, on the other hand, are constrained by their behavioral ecology. Specialized feeding makes a species better suited for this kind of approach because isotopic differences between populations are more likely to reflect broad scale differences in environmental conditions rather than differences in trophic level or habitat preference between populations. This has been demonstrated in a recent study of archaeological Atlantic salmon (freshwater resident Salmo salar) bone collagen in North America's Great Lakes-St. Lawrence region, which identified the presence of multiple freshwater source populations for fish consumed at a groups of sites (Guiry et al., 2016b).
Despite a long standing concern for the presence of freshwater fish in human diets among stable isotope based paleodietary studies, the specific technologies and practices employed in the harvesting of aquatic resources has received relatively little attention. Recently, there has been growing number of studies specifically targeting fish remains for stable carbon and nitrogen isotope analyses to address questions not only about human diet but also human impacts on past aquatic environments (Robson et al., 2012, 2015; Guiry et al., 2016b; Häberle et al., 2016; Braje et al., 2017). As this section highlights, there remains significant untapped potential to use these same data for the purpose of better understanding human intentionality involved in exploiting aquatic resources, from both technological and traditional ecological knowledge perspectives.
Summary and Conclusion
Owing to the tremendously complex nature of freshwater biogeochemistry, a diverse range of factors can influence the isotopic composition of aquatic resources. Although the importance of freshwater resources in prehistoric human subsistence practices is widely acknowledged, few studies give sufficient consideration to these complexities during stable isotope based human paleodietary reconstructions. While the factors that influence δ13C and δ15N in freshwater food webs are often deeply interconnected, the biogeochemical processes governing isotopic fractionations in the carbon and nitrogen cycles differ and need to be carefully considered on their own terms and against local environmental conditions.
While factors influencing the isotopic composition of aquatic resources are context dependent, some useful generalizations can nonetheless be made. For stable carbon isotopic compositions, key variables to consider when interpreting aquatic resource use include whether offshore, nearshore, and riverine freshwater species were used, which should have widely divergent δ13C values (ranging 30‰ or more). Differing carbon sources between and even within freshwater systems can also give rise to large differences in the δ13C (~10–15‰) of aquatic resources at a range of spatial and temporal scales. For stable nitrogen isotopic compositions, a much wider range of potential nitrogen sources, as well as the more complex nature of aquatic nitrogen cycles means that fewer generalizations are possible. However, the importance of environmental parameters such oxygenation, pH, and nitrogen limitation should be kept in mind as these are some of the key levers governing major shifts in the isotopic composition of nitrogenous nutrients in aquatic ecosystems. In this context, the presence of ecotonal environments (e.g., wetlands, riparian zones) and potential allochthonous nitrogen sources (terrestrial runoff, upstream environments, and human activities) can be particularly important. Though many of these variables may be difficult to establish for ancient ecosystems, clues may be found in the form of other paleoenvironmental data for an area or by analogy through comparisons of archaeological contexts with modern ecosystems that may be similar in ecological structure and environmental conditions. In all cases, it is important to remember that these sources of variation, even on a seasonal basis, may have a stronger impact on the isotopic composition of the edible portions of a given freshwater food item (i.e., flesh) than might be apparent from isotopic baselines from corresponding archaeological bone collagen.
Faced with the extreme complexity of freshwater ecosystems, it is critical that archaeologists accurately and honestly convey the uncertainty associated with their interpretations of freshwater resources use when reconstructing ancient human diets. There are a number of practices that could help improve archaeological interpretations of freshwater resource use. These could include incorporating additional analyses from stable sulfur isotopic compositions of bone collagen (e.g., Privat et al., 2007) as well as from CSIA-AA (e.g., McMahon et al., 2010; Honch et al., 2012; Larsen et al., 2013; Naito et al., 2013; McMahon and McCarthy, 2016; Ohkouchi et al., 2017; Ishikawa, 2018), which may in some cases help to better distinguish between use of different types of aquatic resources. Increasing sample sizes of the aquatic fauna that are used as a baseline for interpreting human isotopic data can help to incorporate and more accurately represent the variation that is inherent to a past subsistence regime. In addition, mixing models that are becoming more widely embraced by archaeologists can offer a means of quantifying the uncertainty associated with interpretation of freshwater resource contributions to human diet. Both of these latter suggestions assume that faunal assemblages available for analyses from relevant archaeological sites are of sufficient size and include a broad range of taxa. While fish remains can be exceedingly abundant at some archaeological sites, larger scale analyses that are amenable to more sophisticated quantitative analyses may not be possible for many archaeological sites where poor preservation limits samples size. Especially for smaller datasets, organizing data from freshwater species according to their behavioral ecology (e.g., regrouping data based on affinity for offshore, nearshore, and riverine habitats) can provide a means of condensing data from many smaller groups of disparate taxa into a manageable number of larger, more analytically meaningful units.
While the complexities of freshwater ecosystems may present a challenge to interpretations of ancient human diets, they can also provide new opportunities for reconstructing variation in past environmental conditions and cultural practices for exploiting freshwater environments. Freshwater carbon and nitrogen cycles are sensitive to changes in climate, eutrophication, and other human interventions, all of which can leave detectable traces in the isotopic compositions of ancient fish, bird, and mammal bones. Likewise, many of the technologies and ecological knowledge frameworks that have enabled humans to effectively exploit freshwater resources throughout our evolutionary history may also leave behind characteristic patterns in the isotopic composition of archaeological fish remains. This paper has aimed to outline some of the ways in which carefully designed isotopic time series, with sufficient sample sizes, could utilize archaeological fauna to help address major questions of broader interdisciplinary significance.
Author Contributions
The author confirms being the sole contributor of this work and has approved it for publication.
Funding
Social Sciences and Humanities Research Council of Canada (SSHRC) Insight Development Grant, SSHRC Postdoctoral Research Fellowship, and a SSHRC Banting Postdoctoral Research Fellowship.
Conflict of Interest Statement
The author declares that the research was conducted in the absence of any commercial or financial relationships that could be construed as a potential conflict of interest.
Acknowledgments
This manuscript has benefited substantially from discussions with or technical assistance from: Kate Dougherty, Paul Szpak (Trent University), Thomas Royle (Simon Fraser University), Tyler Wire (British Columbia Fisheries Management), Dylan Hillis (University of Victoria), Trevor Orchard (University of Toronto Mississauga), Suzanne Needs-Howarth (Perca Zooarchaeology).
Supplementary Material
The Supplementary Material for this article can be found online at: https://www.frontiersin.org/articles/10.3389/fevo.2019.00313/full#supplementary-material
Footnotes
1. ^Remodeling is the rate at which older materials are replaced by newer materials, usually through routine cellular maintenance or replacement.
2. ^CO2 that is breathed out by organisms as a product of metabolic processes.
3. ^Fish that can live in freshwater environments but migrate to the sea to spawn.
4. ^It is also possible that the N2 could be supplied from denitrification processes, in which case this would drive the overall stable nitrogen isotopic compositions further downward.
5. ^Ammonium is the least metabolically expensive form of DIN for autotrophs to process and is therefore preferred by many primary producers.
6. ^Eutrophication is the excessive input of nutrients to a aquatic ecosystem.
7. ^Nutrient loading is the addition of excessive nutrients to an ecosystem.
References
Adrian, R., O'Reilly, C. M., Zagarese, H., Baines, S. B., Hessen, D. O., Keller, W., et al. (2009). Lakes as sentinels of climate change. Limnol. Oceanogr. 54, 2283–2297. doi: 10.4319/lo.2009.54.6_part_2.2283
Allen, M. S. (1992). Temporal variation in polynesian fishing strategies: the southern cook Islands in regional perspective. Asian Perspect. 31, 183–204.
Altabet, M. (1988). Variations in nitrogen isotopic composition between sinking and suspended particles: implications for nitrogen cycling and particle transformation in the open ocean. Deep Sea Res. A 35, 535–554. doi: 10.1016/0198-0149(88)90130-6
Anderson, C., and Cabana, G. (2005). δ15N in riverine food webs: effects of N inputs from agricultural watersheds. Can. J. Fish. Aqu. Sci. 62, 333–340. doi: 10.1139/f04-191
Anderson, C., and Cabana, G. (2006). Does δ 15 N in river food webs reflect the intensity and origin of N loads from the watershed? Sci. Total Environ. 367, 968–978. doi: 10.1016/j.scitotenv.2006.01.029
Atkinson, C. L., Christian, A. D., Spooner, D. E., and Vaughn, C. C. (2014). Long-lived organisms provide an integrative footprint of agricultural land use. Ecol. Appl. 24, 375–384. doi: 10.1890/13-0607.1
Austin, A. T., and Vitousek, P. (1998). Nutrient dynamics on a precipitation gradient in Hawai'i. Oecologia 113, 519–529. doi: 10.1007/s004420050405
Avise, J. C. (2003). “Catadromous eels of the north atlantic: a review of molecular genetic findings relevant to natural history, population structure, speciation, and phylogeny,” in Eel Biology, eds K. Aida, K. Tsukamoto, and K. Yamauchi (Tokyo: Springer), 31–48. doi: 10.1007/978-4-431-65907-5_3
Bade, D. L., Carpenter, S. R., Cole, J. J., Hanson, P. C., and Hesslein, R. H. (2004). Controls of δ13C-DIC in lakes: geochemistry, lake metabolism, and morphometry. Limnol. Oceanogr. 49, 1160–1172. doi: 10.4319/lo.2004.49.4.1160
Barak, R. S., Hipp, A. L., Cavender-Bares, J., Pearse, W. D., Hotchkiss, S. C., Lynch, E. A., et al. (2016). Taking the long view: integrating recorded, archeological, paleoecological, and evolutionary data into ecological restoration. Int. J. Plant Sci. 177, 90–102. doi: 10.1086/683394
Bardonnet, A., and Riera, P. (2005). Feeding of glass eels (Anguilla anguilla) in the course of their estuarine migration: new insights from stable isotope analysis. Estu. Coast. Shelf Sci. 63, 201–209. doi: 10.1016/j.ecss.2004.11.009
Barrett, J., Johnstone, C., Harland, J., Van Neer, W., Ervynck, A., Makowiecki, D., et al. (2008). Detecting the medieval cod trade: a new method and first results. J. Archaeol. Sci. 35, 850–861. doi: 10.1016/j.jas.2007.06.004
Barry, J. (2015). The Foraging Specialisms, Movement and Migratory Behaviour of the European Eel. Ph.D. thesis, University of Glasgow, Glasgow, United Kingdom.
Barth, J. A., Veizer, J., and Mayer, B. (1998). Origin of particulate organic carbon in the upper St. Lawrence: isotopic constraints. Earth Planet. Sci. Lett. 162, 111–121. doi: 10.1016/S0012-821X(98)00160-5
Beardall, J., Griffiths, H., and Raven, J. (1982). Carbon isotope discrimination and the CO2 accumulating mechanism in Chlorella emersonii. J. Exp. Bot. 33, 729–737. doi: 10.1093/jxb/33.4.729
Beaudoin, C. P., Tonn, W. M., Prepas, E. E., and Wassenaar, L. I. (1999). Individual specialization and trophic adaptability of northern pike (Esox lucius): an isotope and dietary analysis. Oecologia 120, 386–396. doi: 10.1007/s004420050871
Bellard, C., Bertelsmeier, C., Leadley, P., Thuiller, W., and Courchamp, F. (2012). Impacts of climate change on the future of biodiversity. Ecol. Lett. 15, 365–377. doi: 10.1111/j.1461-0248.2011.01736.x
Bilby, R. E., Fransen, B. R., and Bisson, P. A. (1996). Incorporation of nitrogen and carbon from spawning coho salmon into the trophic system of small streams: evidence from stable isotopes. Can. J. Fish. Aquat. Sci. 53, 164–173. doi: 10.1139/cjfas-53-1-164
Blanke, C., Chikaraishi, Y., and Vander Zanden, M. J. (2018). Historical niche partitioning and long-term trophic shifts in Laurentian Great Lakes deepwater coregonines. Ecosphere 9:e02080. doi: 10.1002/ecs2.2080
Bocherens, H. (2009). “Neanderthal dietary habits: review of the isotopic evidence,” in The Evolution of Hominin Diets: Integrating Approaches to the Study of Palaeolithic Subsistence, eds J. -J. Hublin and M. P. Richards (Dordrecht: Springer), 241–250. doi: 10.1007/978-1-4020-9699-0_19
Bocherens, H., Fizet, M., Mariotti, A., Lange-Badre, B., Vandermeersch, B., Borel, J. P., et al. (1991). Isotopic biogeochemistry (13C,15N) of fossil vertebrate collagen: application to the study of a past food web including Neandertal man. J. Hum. Evolu. 20, 481–492. doi: 10.1016/0047-2484(91)90021-M
Bonsall, C., Lennon, R., McSweeney, K., Stewart, C., Harkness, D., Boroneanţ, V., et al. (1997). Mesolithic and early neolithic in the iron gates: a palaeodietary perspective. J. Eur. Archaeol. 5, 50–92. doi: 10.1179/096576697800703575
Botrel, M., Gregory-Eaves, I., and Maranger, R. (2014). Defining drivers of nitrogen stable isotopes (δ15N) of surface sediments in temperate lakes. J. Paleolimnol. 52, 419–433. doi: 10.1007/s10933-014-9802-6
Braje, T. J., Rick, T. C., Szpak, P., Newsome, S. D., McCain, J. M., Smith, E. A., et al. (2017). Historical ecology and the conservation of large, hermaphroditic fishes in Pacific Coast kelp forest ecosystems. Sci. Adv. 3:e1601759. doi: 10.1126/sciadv.1601759
Brandes, J. A., and Devol, A. H. (1997). Isotopic fractionation of oxygen and nitrogen in coastal marine sediments. Geochim. Cosmochim. Acta 61, 1793–1801. doi: 10.1016/S0016-7037(97)00041-0
Brown, C. J., Knight, B. W., McMaster, M. E., Munkittrick, K. R., Oakes, K. D., Tetreault, G. R., et al. (2011). The effects of tertiary treated municipal wastewater on fish communities of a small river tributary in Southern Ontario, Canada. Environ. Pollut. 159, 1923–1931. doi: 10.1016/j.envpol.2011.03.014
Brown, T. A., Nelson, D. E., Vogel, J. S., and Southon, J. R. (1988). Improved collagen extraction by modified Longin method. Radiocarbon 30, 171–177. doi: 10.1017/S0033822200044118
Brugam, R., Little, K., Kohn, L., Brunkow, P., Vogel, G., and Martin, T. (2016). Tracking change in the Illinois river using stable isotopes in modern and ancient fishes. River Res. Appl. 33, 341–352. doi: 10.1002/rra.3099
Cabana, G., and Rasmussen, J. B. (1996). Comparison of aquatic food chains using nitrogen isotopes. Proc. Natl. Acad. Sci. U.S.A. 93, 10844–10847. doi: 10.1073/pnas.93.20.10844
Carpenter, S. R., Cole, J. J., Pace, M. L., Van de Bogert, M., Bade, D. L., Bastviken, D., et al. (2005). Ecosystem subsidies: terrestrial support of aquatic food webs from 13C addition to contrasting lakes. Ecology 86, 2737–2750. doi: 10.1890/04-1282
Casciotti, K. L., Sigman, D. M., and Ward, B. B. (2003). Linking diversity and stable isotope fractionation in ammonia-oxidizing bacteria. Geomicrobiol. J. 20, 335–353. doi: 10.1080/01490450303895
Casey, M. M., and Post, D. M. (2011). The problem of isotopic baseline: reconstructing the diet and trophic position of fossil animals. Earth Sci. Rev. 106, 131–148. doi: 10.1016/j.earscirev.2011.02.001
Caut, S., Angulo, E., and Courchamp, F. (2009). Variation in discrimination factors (Δ15N and Δ13C): the effect of diet isotopic values and applications for diet reconstruction. J. Appl. Ecol. 46, 443–453. doi: 10.1111/j.1365-2664.2009.01620.x
Chaloner, D. T., Martin, K. M., Wipfli, M. S., Ostrom, P. H., and Lamberti, G. A. (2002). Marine carbon and nitrogen in southeastern Alaska stream food webs: evidence from artificial and natural streams. Can. J. Fish. Aquat. Sci. 59, 1257–1265. doi: 10.1139/f02-084
Chikaraishi, Y., Ogawa, N. O., Kashiyama, Y., Takano, Y., Suga, H., Tomitani, A., et al. (2009). Determination of aquatic food-web structure based on compound-specific nitrogen isotopic composition of amino acids. Limnol. Oceanogr. 7, 740–750. doi: 10.4319/lom.2009.7.740
Chisholm, B. S., Nelson, D. E., and Schwarcz, H. P. (1982). Stable-carbon isotope ratios as a measure of marine versus terrestrial protein in ancient diets. Science 216, 1131–1132. doi: 10.1126/science.216.4550.1131
Choi, W.-J., Kwak, J.-H., Lim, S.-S., Park, H.-J., Chang, S. X., Lee, S.-M., et al. (2017). Synthetic fertilizer and livestock manure differently affect δ15N in the agricultural landscape: a review. Agri. Ecosyst. Environ. 237, 1–15. doi: 10.1016/j.agee.2016.12.020
Cleland, C. E. (1982). The inland shore fishery of the northern Great Lakes: its development and importance in prehistory. Am. Antiquity 47, 761–784. doi: 10.2307/280281
Colborne, S. F., Rush, S. A., Paterson, G., Johnson, T. B., Lantry, B. F., and Fisk, A. T. (2016). Estimates of lake trout (Salvelinus namaycush) diet in Lake Ontario using two and three isotope mixing models. J. Great Lakes Res. 42, 695–702. doi: 10.1016/j.jglr.2016.03.010
Cole, J. J., Prairie, Y. T., Caraco, N. F., McDowell, W. H., Tranvik, L. J., Striegl, R. G., et al. (2007). Plumbing the global carbon cycle: integrating inland waters into the terrestrial carbon budget. Ecosystems 10, 172–185. doi: 10.1007/s10021-006-9013-8
Cole, M. L., Valiela, I., Kroeger, K. D., Tomasky, G. L., Cebrian, J., Wigand, C., et al. (2004). Assessment of a δ15N isotopic method to indicate anthropogenic eutrophication in aquatic ecosystems. J. Environ. Q. 33, 124–132. doi: 10.2134/jeq2004.0124
Coletta, P., Pentecost, A., and Spiro, B. (2001). Stable isotopes in charophyte incrustations: relationships with climate and water chemistry. Palaeogeogr. Palaeoclimatol. Palaeoecol. 173, 9–19. doi: 10.1016/S0031-0182(01)00305-4
Colley, S. M. (1987). Fishing for facts. Can we reconstruct fishing methods from archaeological evidence? Aust. Archaeol. 24, 16–26.
Collister, J. W., and Hayes, J. M. (1991). “A preliminary study of the carbon and nitrogen isotope biogeochemistry of lacustrine sedimentary rocks from the Green River Formation,” in Recent Geochemical, Biogeochemical, and Sedimentological Studies of the Green River Formation, Edition: U. S. Geological Survey Bulletin 1973, ed M. L. Tuttle (U.S. G.P.O.), C1–C16.
Conley, D. J., Paerl, H. W., Howarth, R. W., Boesch, D. F., Seitzinger, S. P., Havens, K. E., et al. (2009). Controlling eutrophication: nitrogen and phosphorus. Science 323, 1014–1015. doi: 10.1126/science.1167755
Cook, G. T., Bonsall, C., Hedges, R. E., McSweeney, K., Boronean, V., and Pettitt, P. B. (2001). A freshwater diet-derived 14 C reservoir effect at the Stone Age sites in the Iron Gates gorge. Radiocarbon 43, 453–460. doi: 10.1017/S0033822200038327
Craig, H. (1953). The geochemistry of the stable carbon isotopes. Geochim. Cosmochim. Acta 3, 53–92. doi: 10.1016/0016-7037(53)90001-5
Cucherousset, J., Acou, A., Blanchet, S., Britton, J. R., Beaumont, W. R., and Gozlan, R. E. (2011). Fitness consequences of individual specialisation in resource use and trophic morphology in European eels. Oecologia 167, 75–84. doi: 10.1007/s00442-011-1974-4
Dalerum, F., and Angerbjörn, A. (2005). Resolving temporal variation in vertebrate diets using naturally occurring stable isotopes. Oecologia 144, 647–658. doi: 10.1007/s00442-005-0118-0
DeBruyn, A. M., and Rasmussen, J. B. (2002). Quantifying assimilation of sewage-derived organic matter by riverine benthos. Ecol. Appl. 12, 511–520. doi: 10.1890/1051-0761(2002)012[0511:QAOSDO]2.0.CO;2
DeNiro, M. J. (1985). Postmortem preservation and alteration of in vivo bone collagen isotope ratios in relation to palaeodietary reconstruction. Nature 317, 806–809. doi: 10.1038/317806a0
DeNiro, M. J., and Epstein, S. (1981). Influence of diet on the distribution of nitrogen isotopes in animals. Geochim. Cosmochim. Acta 45, 341–351. doi: 10.1016/0016-7037(81)90244-1
di Lascio, A., Rossi, L., Carlino, P., Calizza, E., Rossi, D., and Costantini, M. L. (2013). Stable isotope variation in macroinvertebrates indicates anthropogenic disturbance along an urban stretch of the river Tiber (Rome, Italy). Ecol. Indicat. 28, 107–114. doi: 10.1016/j.ecolind.2012.04.006
Diebel, M. W., and Vander Zanden, M. J. (2009). Nitrogen stable isotopes in streams: effects of agricultural sources and transformations. Ecol. Appl. 19, 1127–1134. doi: 10.1890/08-0327.1
Dixon, H. J., Dempson, J. B., and Power, M. (2015). Assessing the use of different marine growth zones of adult Atlantic salmon scales for studying marine trophic ecology with stable isotope analysis. Fish. Res. 164, 112–119. doi: 10.1016/j.fishres.2014.10.009
Dixon, H. J., Power, M., Dempson, J. B., Sheehan, T. F., and Chaput, G. (2012). Characterizing the trophic position and shift in Atlantic salmon (Salmo salar) from freshwater to marine life-cycle phases using stable isotopes. ICES J. Marine Sci. 69, 1646–1655. doi: 10.1093/icesjms/fss122
Dodds, W. K., Perkin, J. S., and Gerken, J. E. (2013). Human impact on freshwater ecosystem services: a global perspective. Environ. Sci. Technol. 47, 9061–9068. doi: 10.1021/es4021052
Downing, J. A., and McCauley, E. (1992). The nitrogen: phosphorus relationship in lakes. Limnol. Oceanogr. 37, 936–945. doi: 10.4319/lo.1992.37.5.0936
Drucker, D. G., Rosendahl, W., Van Neer, W., Weber, M.-J., Görner, I., and Bocherens, H. (2016). Environment and subsistence in north-western Europe during the Younger Dryas: an isotopic study of the human of Rhünda (Germany). J. Archaeol. Sci. 6, 690–699. doi: 10.1016/j.jasrep.2015.08.002
Dudgeon, D., Arthington, A. H., Gessner, M. O., Kawabata, Z.-I., Knowler, D. J., Lévêque, C., et al. (2006). Freshwater biodiversity: importance, threats, status and conservation challenges. Biol. Rev. 81, 163–182. doi: 10.1017/S1464793105006950
Dufour, E., Bocherens, H., and Mariotti, A. (1999). Palaeodietary implications of isotopic variability in Eurasian lacustrine fish. J. Archaeol. Sci. 26, 617–627. doi: 10.1006/jasc.1998.0379
Dunnette, P. V., Higuera, P. E., McLauchlan, K. K., Derr, K. M., Briles, C. E., and Keefe, M. H. (2014). Biogeochemical impacts of wildfires over four millennia in a Rocky Mountain subalpine watershed. N. Phytol. 203, 900–912. doi: 10.1111/nph.12828
Eberts, R. L., Wissel, B., Manzon, R. G., Wilson, J. Y., Boreham, D. R., and Somers, C. M. (2015). Consistent differential resource use by sympatric lake (Coregonus clupeaformis) and round (Prosopium cylindraceum) whitefish in Lake Huron: a multi-time scale isotopic niche analysis. Can. J. Fish. Aquat. Sci. 73, 1072–1080. doi: 10.1139/cjfas-2015-0324
Elser, J. J., Bracken, M. E., Cleland, E. E., Gruner, D. S., Harpole, W. S., Hillebrand, H., et al. (2007). Global analysis of nitrogen and phosphorus limitation of primary producers in freshwater, marine and terrestrial ecosystems. Ecol. Lett. 10, 1135–1142. doi: 10.1111/j.1461-0248.2007.01113.x
Elser, J. J., Marzolf, E. R., and Goldman, C. R. (1990). Phosphorus and nitrogen limitation of phytoplankton growth in the freshwaters of North America: a review and critique of experimental enrichments. Can. J. Fish. Aquat. Sci. 47, 1468–1477. doi: 10.1139/f90-165
Epp, H. T. (1985). Ecological edges and archaeological site location in Saskatchewan, Canada. North Am. Archaeol. 5, 323–336. doi: 10.2190/XR8C-T1T8-UHNH-87MN
Eriksson, G., Frei, K. M., Howcroft, R., Gummesson, S., Molin, F., Lidén, K., et al. (2018). Diet and mobility among Mesolithic hunter-gatherers in Motala (Sweden)-The isotope perspective. J. Archaeol. Sci. 17, 904–918. doi: 10.1016/j.jasrep.2016.05.052
Erlandson, J. M. (2001). The archaeology of aquatic adaptations: paradigms for a new millennium. J. Archaeol. Res. 9, 287–350. doi: 10.1023/A:1013062712695
Fera, S. A., Rennie, M. D., and Dunlop, E. S. (2017). Broad shifts in the resource use of a commercially harvested fish following the invasion of dreissenid mussels. Ecology 98, 1681–1692. doi: 10.1002/ecy.1836
Finlay, J. C. (2001). Stable-carbon-isotope ratios of river biota: implications for energy flow in lotic food webs. Ecology 82, 1052–1064. doi: 10.1890/0012-9658(2001)082[1052:SCIROR]2.0.CO;2
Finlay, J. C. (2004). Patterns and controls of lotic algal stable carbon isotope ratios. Limnol. Oceanogr. 49, 850–861. doi: 10.4319/lo.2004.49.3.0850
Finlay, J. C., and Kendall, C. (2007). Stable isotope tracing of temporal and spatial variability in organic matter sources to freshwater ecosystems. Stable Isotopes Ecol. Environ. Sci. 2, 283–333. doi: 10.1002/9780470691854.ch10
Finlay, J. C., Power, M. E., and Cabana, G. (1999). Effects of water velocity on algal carbon isotope ratios: implications for river food web studies. Limnol. Oceanogr. 44, 1198–1203. doi: 10.4319/lo.1999.44.5.1198
Fogel, M. L., and Cifuentes, L. A. (1993). “Isotope fractionation during primary production,” in Organic Geochemistry, eds M. H. Engel and S. A. Macko (Boston, MA: Springer), 73–98. doi: 10.1007/978-1-4615-2890-6_3
Fogel, M. L., Tuross, N., and Owsley, D. W. (1989). Nitrogen isotope tracers of human lactation in modern and archaeological populations. Carnegie Inst. Washington Yearbook 88, 111–117.
France, R. (1995). Carbon-13 enrichment in benthic compared to planktonic algae: foodweb implications. Mar. Ecol. Prog. Ser. 124, 307–312. doi: 10.3354/meps124307
Fry, B., and Sherr, E. B. (1989). “δ13C measurements as indicators of carbon flow in marine and freshwater ecosystems,” in Stable Isotopes in Ecological Research, eds P. W. Rundel, J. R. Ehleringr, and K. A. Nagy (New York, NY: Springer), 196–229. doi: 10.1007/978-1-4612-3498-2_12
Fuller, B. T., Fuller, J. L., Harris, D. A., and Hedges, R. E. (2006). Detection of breastfeeding and weaning in modern human infants with carbon and nitrogen stable isotope ratios. Am. J. Phys. Anthropol. 129, 279–293. doi: 10.1002/ajpa.20249
Garman, G. C., and Macko, S. A. (1998). Contribution of marine-derived organic matter to an Atlantic coast, freshwater, tidal stream by anadromous clupeid fishes. J. North Am. Benthol. Soc. 17, 277–285. doi: 10.2307/1468331
Gu, B. (1993). Natural Abundance of 15N in a Subarctic Lake and Biogeochemical Implications to Nitrogen Cycling. Fairbanks, AK: University of Alaska Fairbanks.
Gu, B. (2009). Variations and controls of nitrogen stable isotopes in particulate organic matter of lakes. Oecologia 160, 421–431. doi: 10.1007/s00442-009-1323-z
Gu, B., Schell, D. M., and Alexander, V. (1994). Stable carbon and nitrogen isotopic analysis of the plankton food web in a subarctic lake. Can. J. Fish. Aquat. Sci. 51, 1338–1344. doi: 10.1139/f94-133
Gu, B., Schelske, C. L., and Brenner, M. (1996). Relationship between sediment and plankton isotope ratios (δ13C and δ15N) and primary productivity in Florida lakes. Can. J. Fish. Aquatic Sci. 53, 875–883. doi: 10.1139/f95-248
Guiry, E., Beglane, F., Szpak, P., Schulting, R., McCormick, F., and Richards, M. P. (2018). Anthropogenic changes to the Holocene nitrogen cycle in Ireland. Sci. Adv. 4:eaas9383. doi: 10.1126/sciadv.aas9383
Guiry, E. J., and Gaulton, B. C. (2016). Inferring human behaviors from isotopic analyses of rat diet: a critical review and historical application. J. Archaeol. Method Theory 23, 399–426. doi: 10.1007/s10816-015-9248-9
Guiry, E. J., Hepburn, J. C., and Richards, M. P. (2016a). High-resolution serial sampling for nitrogen stable isotope analysis of archaeological mammal teeth. J. Archaeol. Sci. 69, 21–28. doi: 10.1016/j.jas.2016.03.005
Guiry, E. J., Needs-Howarth, S., Friedland, K. D., Hawkins, A. L., Szpak, P., Macdonald, R., et al. (2016b). Lake Ontario salmon (Salmo salar) were not migratory: a long-standing historical debate solved through stable isotope analysis. Sci. Rep. 6:36249. doi: 10.1038/srep36249
Guiry, E. J., Szpak, P., and Richards, M. P. (2016c). Effects of lipid extraction and ultrafiltration on stable carbon and nitrogen isotopic compositions of fish bone collagen. Rapid Commun. Mass Spectr. 30, 1591–1600. doi: 10.1002/rcm.7590
Gustin, M. S., Saito, L., and Peacock, M. (2005). Anthropogenic impacts on mercury concentrations and nitrogen and carbon isotope ratios in fish muscle tissue of the Truckee River watershed, Nevada, U.S.A. Sci. Total Environ. 347, 282–294. doi: 10.1016/j.scitotenv.2004.12.009
Häberle, S., Nehlich, O., Fuller, B. T., Schibler, J., Van Neer, W., and Plogmann, H. H. (2016). Carbon and nitrogen isotopic ratios in archaeological and modern Swiss fish as possible markers for diachronic anthropogenic activity in freshwater ecosystems. J. Archaeol. Sci. Rep. 10, 411–423. doi: 10.1016/j.jasrep.2016.10.012
Haidvogl, G., Hoffmann, R., Pont, D., Jungwirth, M., and Winiwarter, V. (2015). Historical ecology of riverine fish in Europe. Aquat. Sci. 77, 315–324. doi: 10.1007/s00027-015-0400-0
Halpern, B. S., Walbridge, S., Selkoe, K. A., Kappel, C. V., Micheli, F., D'agrosa, C., et al. (2008). A global map of human impact on marine ecosystems. Science 319, 948–952. doi: 10.1126/science.1149345
Hamilton, S. L., Newsome, S. D., and Caselle, J. E. (2014). Dietary niche expansion of a kelp forest predator recovering from intense commercial exploitation. Ecology 95, 164–172. doi: 10.1890/13-0014.1
Hammersley, P. (2016). Stable Isotope Analysis of Archaeological Faunal Remains From the Middle Trent Valley. Peterborough, ON: Trent University.
Handley, L., Austin, A., Stewart, G., Robinson, D., Scrimgeour, C., Raven, J., et al. (1999). The 15N natural abundance (δ15N) of ecosystem samples reflects measures of water availability. Funct. Plant Biol. 26, 185–199. doi: 10.1071/PP98146
Harrington, R. R., Kennedy, B. P., Chamberlain, C. P., Blum, J. D., and Folt, C. L. (1998). 15N enrichment in agricultural catchments: field patterns and applications to tracking Atlantic salmon (Salmo salar). Chem. Geol. 147, 281–294. doi: 10.1016/S0009-2541(98)00018-7
Harrod, C., Grey, J., McCarthy, T. K., and Morrissey, M. (2005). Stable isotope analyses provide new insights into ecological plasticity in a mixohaline population of European eel. Oecologia 144, 673–683. doi: 10.1007/s00442-005-0161-x
Hawkins, A. L., and Caley, E. (2012). Seasonality, mass capture, and exploitation of fish at the Steven Patrick site, a Uren period village near Kempenfelt Bay. Ontario Archaeol. 92, 95–122.
Hebert, C. E., Shutt, J. L., Hobson, K. A., and Weseloh, D. C. (1999). Spatial and temporal differences in the diet of Great Lakes herring gulls (Larus argentatus): evidence from stable isotope analysis. Can. J. Fish. Aquatic Sci. 56, 323–338. doi: 10.1139/f98-189
Hebert, C. E., and Wassenaar, L. I. (2001). Stable nitrogen isotopes in waterfowl feathers reflect agricultural land use in western Canada. Environ. Sci. Technol. 35, 3482–3487. doi: 10.1021/es001970p
Hebert, C. E., Weseloh, D. V., Idrissi, A., Arts, M. T., O'Gorman, R., Gorman, O. T., et al. (2008). Restoring piscivorous fish populations in the Laurentian Great Lakes causes seabird dietary change. Ecology 89, 891–897. doi: 10.1890/07-1603.1
Hecky, R., and Hesslein, R. (1995). Contributions of benthic algae to lake food webs as revealed by stable isotope analysis. J. North Am. Benthol. Soc. 14, 631–653. doi: 10.2307/1467546
Hedges, R. E., Clement, J. G., Thomas, C. L. D, and O'Connell, T. C. (2007). Collagen turnover in the adult femoral mid-shaft: Modeled from anthropogenic radiocarbon tracer measurements. Am. J. Phys. Anthropol. 133, 808–816. doi: 10.1002/ajpa.20598
Hedges, R. E., and Reynard, L. M. (2007). Nitrogen isotopes and the trophic level of humans in archaeology. J. Archaeol. Sci. 34, 1240–1251. doi: 10.1016/j.jas.2006.10.015
Hobbie, E. A., and Ouimette, A. P. (2009). Controls of nitrogen isotope patterns in soil profiles. Biogeochemistry 95, 355–371. doi: 10.1007/s10533-009-9328-6
Hobson, K. A., Blight, L. K., and Arcese, P. (2015). Human-induced long-term shifts in gull diet from marine to terrestrial sources in North America's coastal Pacific: More evidence from more isotopes (δ2H, δ34S). Environ. Sci. Technol. 49, 10834–10840. doi: 10.1021/acs.est.5b02053
Hobson, K. A., and Clark, R. G. (1992). Assessing avian diets using stable isotopes i: turnover of 13C in tissues. Condor 94, 181–188. doi: 10.2307/1368807
Hodell, D. A., and Schelske, C. L. (1998). Production, sedimentation, and isotopic composition of organic matter in Lake Ontario. Limnol. Oceanogr. 43, 200–214. doi: 10.4319/lo.1998.43.2.0200
Hodell, D. A., Schelske, C. L., Fahnenstiel, G. L., and Robbins, L. L. (1998). Biologically induced calcite and its isotopic composition in Lake Ontario. Limnol. Oceanogr. 43, 187–199. doi: 10.4319/lo.1998.43.2.0187
Hogberg, P. (1997). 15N natural abundance in soil–plant systems. Tansley Review No. 95. N. Phytol. 137, 179–203. doi: 10.1046/j.1469-8137.1997.00808.x
Hollander, D. J., and McKenzie, J. A. (1991). CO2 control on carbon-isotope fractionation during aqueous photosynthesis: a paleo-pCO2 barometer. Geology 19, 929–932. doi: 10.1130/0091-7613(1991)019<0929,CCOCIF>2.3.CO;2
Holtgrieve, G. W., Schindler, D. E., Hobbs, W. O., Leavitt, P. R., Ward, E. J., Bunting, L., et al. (2011). A coherent signature of anthropogenic nitrogen deposition to remote watersheds of the northern hemisphere. Science 334, 1545–1548. doi: 10.1126/science.1212267
Honch, N. V., McCullagh, J. S., and Hedges, R. E. (2012). Variation of bone collagen amino acid δ13C values in archaeological humans and fauna with different dietary regimes: developing frameworks of dietary discrimination. Am. J. Phys. Anthropol. 148, 495–511. doi: 10.1002/ajpa.22065
Hübner, H. (1986). “Chapter 9—isotope effects of nitrogen in the soil and biosphere,” in The Terrestrial Environment, B, eds P. Fritz and J. Fontes (Amsterdam: Elsevier), 361–425. doi: 10.1016/B978-0-444-42225-5.50014-0
Humphries, P., and Winemiller, K. O. (2009). Historical impacts on river fauna, shifting baselines, and challenges for restoration. BioScience 59, 673–684. doi: 10.1525/bio.2009.59.8.9
Ishikawa, N. F. (2018). Use of compound-specific nitrogen isotope analysis of amino acids in trophic ecology: assumptions, applications, and implications. Ecol. Res. 33, 825–837. doi: 10.1007/s11284-018-1616-y
Ishikawa, N. F., Doi, H., and Finlay, J. C. (2012). Global meta-analysis for controlling factors on carbon stable isotope ratios of lotic periphyton. Oecologia 170, 541–549. doi: 10.1007/s00442-012-2308-x
Ishimaru, E., Tayasu, I., Umino, T., and Yumoto, T. (2011). Reconstruction of ancient trade routes in the Japanese Archipelago using carbon and nitrogen stable isotope analysis: identification of the stock origins of marine fish found at the Inland Yokkaichi Site, Hiroshima Prefecture, Japan. J. Island Coast. Archaeol. 6, 160–163. doi: 10.1080/15564894.2010.541552
Jansson, M., Bergström, A.-K., Blomqvist, P., and Drakare, S. (2000). Allochthonous organic carbon and phytoplankton/bacterioplankton production relationships in lakes. Ecology 81, 3250–3255. doi: 10.1890/0012-9658(2000)081[3250:AOCAPB]2.0.CO;2
Jarman, C. L., Larsen, T., Hunt, T., Lipo, C., Solsvik, R., Wallsgrove, N., et al. (2017). Diet of the prehistoric population of Rapa Nui (Easter Island, Chile) shows environmental adaptation and resilience. Am. J. Phys. Anthropol. 164, 343–361. doi: 10.1002/ajpa.23273
Jeffers, E. S., Nogué, S., and Willis, K. J. (2015). The role of palaeoecological records in assessing ecosystem services. Quat. Sci. Rev. 112, 17–32. doi: 10.1016/j.quascirev.2014.12.018
Jones, J. J. B., and Mulholland, P. J. (1998). Carbon dioxide variation in a hardwood forest stream: an integrative measure of whole catchment soil respiration. Ecosystems 1, 183–196. doi: 10.1007/s100219900014
Jones, R. I., Grey, J., Sleep, D., and Quarmby, C. (1998). An assessment, using stable isotopes, of the importance of allochthonous organic carbon sources to the pelagic food web in Loch Ness. Proc. R. Soc. Lond. Ser. B 265, 105–110. doi: 10.1098/rspb.1998.0270
Katzenberg, M. A., and Weber, A. (1999). Stable isotope ecology and palaeodiet in the Lake Baikal region of Siberia. J. Archaeol. Sci. 26, 651–659. doi: 10.1006/jasc.1998.0382
Kendall, C. (1998). “Tracing nitrogen sources and cycling in catchments,” in Isotope tracers in Catchment Hydrology (Elsevier), 519–576. doi: 10.1016/B978-0-444-81546-0.50023-9
Kendall, C., Elliott, E. M., and Wankel, S. D. (2007). Tracing anthropogenic inputs of nitrogen to ecosystems. Stable Isotopes Ecol. Environ. Sci. 2, 375–449. doi: 10.1002/9780470691854.ch12
Kendall, C., Mast, M., and Rice, K. (1992). “Tracing watershed weathering reactions with δ13C,” in Proceedngs of the 7th nternatinoal Symposium of Water-rock interation, eds Y. Kharaka and A. Maest (Rotterdam: A.A. Balkema, 569–572.
Kline, T. C. Jr., Goering, J. J., Mathisen, O. A., Poe, P. H., and Parker, P. L. (1990). Recycling of elements transported upstream by runs of Pacific salmon: I, δ15N and δ13C evidence in Sashin Creek, Southeastern Alaska. Can. J. Fish. Aquat. Sci. 47, 136–144. doi: 10.1139/f90-014
Koerner, W., Dambrine, E., Dupouey, J. L., and Benoît, M. (1999). δ15N of forest soil and understorey vegetation reflect the former agricultural land use. Oecologia 121, 421–425. doi: 10.1007/s004420050947
Kohzu, A., Tayasu, I., Yoshimizu, C., Maruyama, A., Kohmatsu, Y., Hyodo, F., et al. (2009). Nitrogen-stable isotopic signatures of basal food items, primary consumers and omnivores in rivers with different levels of human impact. Ecol. Res. 24, 127–136. doi: 10.1007/s11284-008-0489-x
Kumar, S., Finlay, J. C., and Sterner, R. W. (2011). Isotopic composition of nitrogen in suspended particulate matter of Lake Superior: implications for nutrient cycling and organic matter transformation. Biogeochemistry 103, 1–14. doi: 10.1007/s10533-010-9441-6
Lace, M. J., and Mylroie, J. E. (2013). “The biological and archaeological significance of coastal caves and karst features,” in Coastal Karst Landforms, eds M. J. Lace and J. E. Mylroie (Dordrecht: Springer), 111–126. doi: 10.1007/978-94-007-5016-6_5
Lake, J. L., McKinney, R. A., Osterman, F. A., Pruell, R. J., Kiddon, J., Ryba, S. A., et al. (2001). Stable nitrogen isotopes as indicators of anthropogenic activities in small freshwater systems. Can. J. Fish. Aquat. Sci. 58, 870–878. doi: 10.1139/f01-038
Larsen, T., Ventura, M., Andersen, N., O'Brien, D. M., Piatkowski, U., and McCarthy, M. D. (2013). Tracing carbon sources through aquatic and terrestrial food webs using amino acid stable isotope fingerprinting. PLoS ONE 8:e73441. doi: 10.1371/journal.pone.0073441
Laws, E. A., Popp, B. N., Bidigare, R. R., Kennicutt, M. C., and Macko, S. A. (1995). Dependence of phytoplankton carbon isotopic composition on growth rate and [CO2) aq: theoretical considerations and experimental results. Geochim. Cosmochim. Acta 59, 1131–1138. doi: 10.1016/0016-7037(95)00030-4
Lee-Thorp, J. A. (2008). On isotopes and old bones*. Archaeometry 50, 925–950. doi: 10.1111/j.1475-4754.2008.00441.x
Lehmann, M. F., Bernasconi, S. M., Barbieri, A., and McKenzie, J. A. (2002). Preservation of organic matter and alteration of its carbon and nitrogen isotope composition during simulated and in situ early sedimentary diagenesis. Geochim. Cosmochim. Acta 66, 3573–3584. doi: 10.1016/S0016-7037(02)00968-7
Lehmann, M. F., Sigman, D. M., and Berelson, W. M. (2004). Coupling the 15N/14N and 18O/16O of nitrate as a constraint on benthic nitrogen cycling. Mar. Chem. 88, 1–20. doi: 10.1016/j.marchem.2004.02.001
Leng, M. J., Lamb, A. L., Heaton, T. H. E., Marshall, J. D., Wolfe, B. B., Jones, M. D., et al. (2006). “Isotopes in lake sediments,” in Isotopes in Palaeoenvironmental Research ed M. J. Leng (Dordrecht: Springer), 147–184. doi: 10.1007/1-4020-2504-1_04
Leng, M. J., and Marshall, J. D. (2004). Palaeoclimate interpretation of stable isotope data from lake sediment archives. Quat. Sci. Rev. 23, 811–831. doi: 10.1016/j.quascirev.2003.06.012
Lightfoot, E., Liu, X., and Jones, M. K. (2013). Why move starchy cereals? A review of the isotopic evidence for prehistoric millet consumption across Eurasia. World Archaeol. 45, 574–623. doi: 10.1080/00438243.2013.852070
Loomer, H., Oakes, K., Schiff, S., Taylor, W., and Servos, M. (2015). Use of stable isotopes to trace municipal wastewater effluents into food webs within a highly developed river system. River Res. Appl. 31, 1093–1100. doi: 10.1002/rra.2826
Losey, R. J., Nomokonova, T., and Goriunova, O. I. (2008). Fishing ancient Lake Baikal, Siberia: inferences from the reconstruction of harvested perch (Perca fluviatilis) size. J. Archaeol. Sci. 35, 577–590. doi: 10.1016/j.jas.2007.05.005
Lu, Y. H., Meyers, P. A., Robbins, J. A., Eadie, B. J., Hawley, N., and Ji, K. H. (2014). Sensitivity of sediment geochemical proxies to coring location and corer type in a large lake: implications for paleolimnological reconstruction. Geochem. Geophys. Geosyst. 15, 1960–1976. doi: 10.1002/2013GC004989
Lucas, W. J. (1983). Photosynthetic assimilation of exogenous HCO3 by aquatic plants. Annu. Rev. Plant Physiol. 34, 71–104. doi: 10.1146/annurev.pp.34.060183.000443
Lyman, R. L. (1996). Applied zooarchaeology: the relevance of faunal analysis to wildlife management. World Archaeol. 28, 110–125. doi: 10.1080/00438243.1996.9980334
Lyman, R. L. (2006). Paleozoology in the service of conservation biology. Evolu. Anthropol. 15, 11–19. doi: 10.1002/evan.20083
Lyman, R. L. (2012). A warrant for applied palaeozoology. Biol. Rev. 87, 513–525. doi: 10.1111/j.1469-185X.2011.00207.x
Lyman, R. L., and Cannon, K. P. (2004). Zooarchaeology and Conservation Biology. Salt Lake City, UT: University of Utah Press.
Maberly, S., Raven, J., and Johnston, A. (1992). Discrimination between 12 C and 13 C by marine plants. Oecologia 91, 481–492. doi: 10.1007/BF00650320
MacKenzie, K. M., Trueman, C. N., Palmer, M. R., Moore, A., Ibbotson, A. T., Beaumont, W. R., et al. (2012). Stable isotopes reveal age-dependent trophic level and spatial segregation during adult marine feeding in populations of salmon. ICES J. Mar. Sci. 69, 1637–1645. doi: 10.1093/icesjms/fss074
Mariotti, A., Lancelot, C., and Billen, G. (1984). Natural isotopic composition of nitrogen as a tracer of origin for suspended organic matter in the Scheldt estuary. Geochim. Cosmochim. Acta 48, 549–555. doi: 10.1016/0016-7037(84)90283-7
Massa, C., Bichet, V., Gauthier, É., Perren, B. B., Mathieu, O., Petit, C., et al. (2012). A 2500 year record of natural and anthropogenic soil erosion in South Greenland. Quat. Sci. Rev. 32, 119–130. doi: 10.1016/j.quascirev.2011.11.014
McClain, M. E., Boyer, E. W., Dent, C. L., Gergel, S. E., Grimm, N. B., Groffman, P. M., et al. (2003). Biogeochemical hot spots and hot moments at the interface of terrestrial and aquatic ecosystems. Ecosystems 6, 301–312. doi: 10.1007/s10021-003-0161-9
McClelland, J. W., Holl, C., and Montoya, J. (2003). Relating low δ15N values of zooplankton to N2-fixation in the tropical North Atlantic: insights provided by stable isotope ratios of amino acids. Deep Sea Res. Part I. 50, 849–861. doi: 10.1016/S0967-0637(03)00073-6
McClelland, J. W., and Montoya, J. P. (2002). Trophic relationships and the nitrogen isotopic composition of amino acids in plankton. Ecology 83, 2173–2180. doi: 10.1890/0012-9658(2002)083[2173:TRATNI]2.0.CO;2
McClelland, J. W., Valiela, I., and Michener, R. H. (1997). Nitrogen-stable isotope signatures in estuarine food webs: a record of increasing urbanization in coastal watersheds. Limnol. Oceanogr. 42, 930–937. doi: 10.4319/lo.1997.42.5.0930
McKenzie, J. (1985). “Carbon isotopes and productivity in the lacustrine and marine environment,” in Chemical Processes in Lakes, ed W. Stumm (New York, NY: Wiley and Sons, 99–118.
McMahon, K. W., Fogel, M. L., Elsdon, T. S., and Thorrold, S. R. (2010). Carbon isotope fractionation of amino acids in fish muscle reflects biosynthesis and isotopic routing from dietary protein. J. Anim. Ecol. 79, 1132–1141. doi: 10.1111/j.1365-2656.2010.01722.x
McMahon, K. W., and McCarthy, M. D. (2016). Embracing variability in amino acid δ15N fractionation: mechanisms, implications, and applications for trophic ecology. Ecosphere 7:e01511. doi: 10.1002/ecs2.1511
Meeuwig, M. H., and Peacock, M. M. (2017). Food web interactions associated with a lahontan cutthroat trout reintroduction effort in an alpine lake. J. Fish Wildlife Manag. 8, 449–464. doi: 10.3996/092016-JFWM-073
Meyers, P. A., and Lallier-Vergès, E. (1999). Lacustrine sedimentary organic matter records of Late Quaternary paleoclimates. J. Paleolimnol. 21, 345–372. doi: 10.1023/A:1008073732192
Miller, M. J., Capriles, J. M., and Hastorf, C. A. (2010). The fish of Lake Titicaca: implications for archaeology and changing ecology through stable isotope analysis. J. Archaeol. Sci. 37, 317–327. doi: 10.1016/j.jas.2009.09.043
Minagawa, M., and Wada, E. (1984). Stepwise enrichment of 15N along food chains: further evidence and the relation between δ15N and animal age. Geochim. Cosmochim. Acta 48, 1135–1140. doi: 10.1016/0016-7037(84)90204-7
Mook, W., Bommerson, J., and Staverman, W. (1974). Carbon isotope fractionation between dissolved bicarbonate and gaseous carbon dioxide. Earth Planet. Sci. Lett. 22, 169–176. doi: 10.1016/0012-821X(74)90078-8
Mook, W., and Tan, F. (1991). Stable carbon isotopes in rivers and estuaries,”in Biogeochemistry of Major World Rivers, eds E. Degens, S. Kempe, and J. Richey (Chichester: John Wiley, 245–264.
Morrissey, C. A., Boldt, A., Mapstone, A., Newton, J., and Ormerod, S. J. (2013). Stable isotopes as indicators of wastewater effects on the macroinvertebrates of urban rivers. Hydrobiologia 700, 231–244. doi: 10.1007/s10750-012-1233-7
Müldner, G., and Richards, M. P. (2005). Fast or feast: reconstructing diet in later medieval England by stable isotope analysis. J. Archaeol. Sci. 32, 39–48. doi: 10.1016/j.jas.2004.05.007
Naito, Y. I., Bocherens, H., Chikaraishi, Y., Drucker, D. G., Wißing, C., Yoneda, M., et al. (2016). An overview of methods used for the detection of aquatic resource consumption by humans: compound-specific delta N-15 analysis of amino acids in archaeological materials. J. Archaeol. Sci. Rep. 6, 720–732. doi: 10.1016/j.jasrep.2015.11.025
Naito, Y. I., Chikaraishi, Y., Drucker, D. G., Ohkouchi, N., Semal, P., Wißing, C., et al. (2018). Reply to “Comment on” Ecological niche of Neanderthals from Spy Cave revealed by nitrogen isotopes of individual amino acids in collagen. J. Hum. Evol. 117, 53–55. doi: 10.1016/j.jhevol.2017.09.008
Naito, Y. I., Chikaraishi, Y., Ohkouchi, N., Drucker, D. G., and Bocherens, H. (2013). Nitrogen isotopic composition of collagen amino acids as an indicator of aquatic resource consumption: insights from Mesolithic and Epipalaeolithic archaeological sites in France. World Archaeol. 45, 338–359. doi: 10.1080/00438243.2013.820650
Naito, Y. I., Okuda, K., Koganezawa, M., and Tsutsumi, T. (2015). Stable carbon and nitrogen isotopic composition of a whisker and fur from a stuffed 19th century specimen of the extinct Japanese river otter collected from inland Honshu, Japan. Mammal Study 40, 265–270. doi: 10.3106/041.040.0407
Nakajima, T., Nakajima, M., and Yamazaki, T. (2010). Evidence for fish cultivation during the Yayoi Period in western Japan. Int. J. Osteoarchaeol. 20, 127–134.
Needoba, J. A., Sigman, D. M., and Harrison, P. J. (2004). The mechanism of isotope fractionation during algal nitrate assimilation as illuminated by the 15n/14n of intracellular nitrate1. J. Phycol. 40, 517–522. doi: 10.1111/j.1529-8817.2004.03172.x
Needs-Howarth, S., and Thomas, S. C. (1998). Seasonal variation in fishing strategies at two Iroquoian village sites near Lake Simcoe, Ontario. Environ. Archaeol. 3, 109–120. doi: 10.1179/env.1998.3.1.109
Nestler, A., Berglund, M., Accoe, F., Duta, S., Xue, D., Boeckx, P., et al. (2011). Isotopes for improved management of nitrate pollution in aqueous resources: review of surface water field studies. Environ. Sci. Pol. Res. 18, 519–533. doi: 10.1007/s11356-010-0422-z
Nikolenko, O., Jurado, A., Borges, A. V., Knöller, K., and Brouyére, S. (2018). Isotopic composition of nitrogen species in groundwater under agricultural areas: a review. Sci. Total Environ. 621, 1415–1432. doi: 10.1016/j.scitotenv.2017.10.086
Nordt, L. C., Boutton, T. W., Hallmark, C. T., and Waters, M. R. (1994). Late Quaternary vegetation and climate changes in central Texas based on the isotopic composition of organic carbon. Quat. Res. 41, 109–120. doi: 10.1006/qres.1994.1012
O'Connell, T. C., and Collins, M. J. (2018). Comment on “Ecological niche of Neanderthals from Spy Cave revealed by nitrogen isotopes of individual amino acids in collagen”[J. Hum. Evol. 93 (2016) 82-90]. Journal of human evolution:53-55. doi: 10.1016/j.jhevol.2017.05.006
Ogawa, N. O., Koitabashi, T., Oda, H., Nakamura, T., Ohkouchi, N., and Wada, E. (2001). Fluctuations of nitrogen isotope ratio of gobiid fish (Isaza) specimens and sediments in Lake Biwa, Japan, during the 20th century. Limnol. Oceanogr. 46, 1228–1236. doi: 10.4319/lo.2001.46.5.1228
Ohkouchi, N., Chikaraishi, Y., Close, H. G., Fry, B., Larsen, T., Madigan, D. J., et al. (2017). Advances in the application of amino acid nitrogen isotopic analysis in ecological and biogeochemical studies. Org. Geochem. 113, 150–174. doi: 10.1016/j.orggeochem.2017.07.009
O'Leary, M. H. (1981). Carbon isotope fractionation in plants. Phytochemistry 20, 553–567. doi: 10.1016/0031-9422(81)85134-5
O'Leary, M. H. (1984). Measurement of the isotope fractionation associated with diffusion of carbon dioxide in aqueous solution. J. Phys. Chem. 88, 823–825. doi: 10.1021/j150648a041
Ostrom, N. E., Long, D. T., Bell, E. M., and Beals, T. (1998). The origin and cycling of particulate and sedimentary organic matter and nitrate in Lake Superior. Chem. Geol. 152, 13–28. doi: 10.1016/S0009-2541(98)00093-X
Ostrom, P. H., Wiley, A. E., James, H. F., Rossman, S., Walker, W. A., Zipkin, E. F., et al. (2017) Broad-scale trophic shift in the pelagic North Pacific revealed by an oceanic seabird. R. Soc. 284. doi: 10.1098/rspb.2016.2436.
Ottalagano, F., and Loponte, D. (2017). Stable isotopes and diet in complex hunter-gatherers of Paraná River Basin, South America. Archaeol. Anthropol. Sci. 9, 865–877. doi: 10.1007/s12520-015-0308-6
Overman, N. C., Beauchamp, D. A., Berge, H. B., Mazur, M. M., and McIntyre, J. K. (2009). Differing forage fish assemblages influence trophic structure in neighboring urban lakes. Trans. Am. Fish. Soc. 138, 741–755. doi: 10.1577/T08-158.1
Pace, M. L., Cole, J. J., Carpenter, S. R., and Kitchell, J. F. (1999). Trophic cascades revealed in diverse ecosystems. Trends Ecol. Evolu. 14, 483–488. doi: 10.1016/S0169-5347(99)01723-1
Pennock, J. R., Velinsky, D. J., Ludlam, J. M., Sharp, J. H., and Fogel, M. L. (1996). Isotopic fractionation of ammonium and nitrate during uptake by Skeletonema costatum: implications for δ15N dynamics under bloom conditions. Limnol. Oceanogr. 41, 451–459. doi: 10.4319/lo.1996.41.3.0451
Pfeiffer, S., Sealey, J., Williamson, R., Needs-Howarth, S., and Lesage, L. (2016). Maize, fish, and deer: investigating dietary staples among ancestral Huron-Wendat villages, as documented from tooth samples. Amer Antiquity 81, 515–532. doi: 10.7183/0002-7316.81.3.515
Popp, B. N., Laws, E. A., Bidigare, R. R., Dore, J. E., Hanson, K. L., and Wakeham, S. G. (1998). Effect of phytoplankton cell geometry on carbon isotopic fractionation. Geochim. Cosmochim. Acta 62, 69–77. doi: 10.1016/S0016-7037(97)00333-5
Post, D. M. (2002). Using stable isotopes to estimate trophic position: models, methods, and assumptions. Ecology 83, 703–718. doi: 10.1890/0012-9658(2002)083[0703:USITET]2.0.CO;2
Privat, K. L., O'Connell, T. C., and Hedges, R. E. (2007). The distinction between freshwater-and terrestrial-based diets: methodological concerns and archaeological applications of sulphur stable isotope analysis. J. Archaeol. Sci. 34, 1197–1204. doi: 10.1016/j.jas.2006.10.008
Raven, J. A., Johnston, A. M., Kübler, J. E., Korb, R., McInroy, S. G., Handley, L. L., et al. (2002). Mechanistic interpretation of carbon isotope discrimination by marine macroalgae and seagrasses. Funct. Plant Biol. 29, 355–378. doi: 10.1071/PP01201
Rennie, M. D., Evans, D. O., and Young, J. D. (2013). Increased dependence on nearshore benthic resources in the Lake Simcoe ecosystem after dreissenid invasion. Inland Waters 3, 297–310. doi: 10.5268/IW-3.2.540
Richards, M. P., Karavanić, I., Pettitt, P., and Miracle, P. (2015). Isotope and faunal evidence for high levels of freshwater fish consumption by Late Glacial humans at the Late Upper Palaeolithic site of Šandalja, II, Istria, Croatia. J. Archaeol. Science 61, 204–212. doi: 10.1016/j.jas.2015.06.008
Rick, T. C., and Lockwood, R. (2013). Integrating paleobiology, archeology, and history to inform biological conservation. Conserv. Biol. 27, 45–54. doi: 10.1111/j.1523-1739.2012.01920.x
Robinson, C. S., Tetreault, G. R., McMaster, M. E., and Servos, M. R. (2016). Impacts of a tertiary treated municipal wastewater effluent on the carbon and nitrogen stable isotope signatures of two darter species (Etheostoma blennioides and E. caeruleum) in a small receiving environment. Ecol. Indicat. 60, 594–602. doi: 10.1016/j.ecolind.2015.06.041
Robson, H., Andersen, S., Craig, O., Fischer, A., Glykou, A., Hartz, S., et al. (2012). Carbon and nitrogen isotope signals in eel bone collagen from Mesolithic and Neolithic sites in northern Europe. J. Archaeol. Sci. 39, 2003–2011. doi: 10.1016/j.jas.2012.01.033
Robson, H. K., Andersen, S. H., Clarke, L., Craig, O. E., Gron, K. J., Jones, A. K., et al. (2015). Carbon and nitrogen stable isotope values in freshwater, brackish and marine fish bone collagen from Mesolithic and Neolithic sites in central and northern Europe. Environ. Archaeol. 21, 105–118. doi: 10.1179/1749631415Y.0000000014
Roeske, C., and O'Leary, M. H. (1984). Carbon isotope effects on enzyme-catalyzed carboxylation of ribulose bisphosphate. Biochemistry 23, 6275–6284. doi: 10.1021/bi00320a058
Roussel, J. M., Perrier, C., Erkinaro, J., Niemelä, E., Cunjak, R. A., Huteau, D., et al. (2014). Stable isotope analyses on archived fish scales reveal the long-term effect of nitrogen loads on carbon cycling in rivers. Glob. Change Biol. 20, 523–530. doi: 10.1111/gcb.12293
Royle, T. C. A., Sakhrani, D., Speller, C. F., Butler, V. L., Devlin, R. H., Cannon, A., et al. (2018). An efficient and reliable DNA-based sex identification method for archaeological Pacific salmonid (Oncorhynchus spp.) remains. PLoS ONE 13:e0193212. doi: 10.1371/journal.pone.0193212
Ruess, R. W., and McNaughton, S. J. (1988). Ammonia volatilization and the effects of large grazing mammals on nutrient loss from East African grasslands. Oecologia 77, 382–386. doi: 10.1007/BF00378047
Sagouis, A., Cucherousset, J., Villéger, S., Santoul, F., and Boulêtreau, S. (2015). Non-native species modify the isotopic structure of freshwater fish communities across the globe. Ecography 38, 979–985. doi: 10.1111/ecog.01348
Santoro, R., Bentivoglio, F., Carlino, P., Calizza, E., Costantini, M., and Rossi, L. (2014). Sensitivity of food webs to nitrogen pollution: a study of three transitional water ecosystems embedded in agricultural landscapes. Trans Waters Bull. 8, 84–97. doi: 10.1285/i1825229Xv8n1p84
Scharf, F. S., Juanes, F., and Rountree, R. A. (2000). Predator size-prey size relationships of marine fish predators: interspecific variation and effects of ontogeny and body size on trophic-niche breadth. Mar. Ecol. Prog. Ser. 208, 229–248. doi: 10.3354/meps208229
Schelske, C. L., and Hodell, D. A. (1995). Using carbon isotopes of bulk sedimentary organic matter to reconstruct the history of nutrient loading and eutrophication in Lake Erie. Limnol. Oceanogr. 40, 918–929. doi: 10.4319/lo.1995.40.5.0918
Schindler, D. W. (1997). Widespread effects of climatic warming on freshwater ecosystems in North America. Hydrol. Process. 11, 1043–1067. doi: 10.1002/(SICI)1099-1085(19970630)11:8<;1043::AID-HYP517>3.0.CO;2-5
Schoeninger, M. J., and DeNiro, M. J. (1984). Nitrogen and carbon isotopic composition of bone collagen from marine and terrestrial animals. Geochim. Cosmochim. Acta 48, 625–639. doi: 10.1016/0016-7037(84)90091-7
Schoeninger, M. J., DeNiro, M. J., and Tauber, H. (1983). Stable nitrogen isotope ratios of bone collagen reflect marine and terrestrial components of prehistoric human diet. Science 220, 1381–1383. doi: 10.1126/science.6344217
Schulting, R. (2011). “Mesolithic-neolithic transitions: an isotopic tour through Europe,” in Human Bioarchaeology of the Transition to Agriculture, eds R. Pinhasi and J. T. Stock (Chichester: Wiley-Blackwell), 15–41.
Schwarcz, H. P. (2006). “Stable carbon isotope analysis and human diet: a synthesis,” in Histories of Maize, eds J. Staller, R. Tykot, and B. Benz (New York, NY: Elsevier), 315–324. doi: 10.1016/B978-012369364-8/50274-6
Sebilo, M., Billen, G., Grably, M., and Mariotti, A. (2003). Isotopic composition of nitrate-nitrogen as a marker of riparian and benthic denitrification at the scale of the whole Seine River system. Biogeochemistry 63, 35–51. doi: 10.1023/A:1023362923881
Sebilo, M., Billen, G., Mayer, B., Billiou, D., Grably, M., Garnier, J., et al. (2006). Assessing nitrification and denitrification in the Seine River and estuary using chemical and isotopic techniques. Ecosystems 9, 564–577. doi: 10.1007/s10021-006-0151-9
Seitzinger, S., Harrison, J. A., Böhlke, J. K., Bouwman, A. F., Lowrance, R., Peterson, B., et al. (2006). Denitrification across landscapes and waterscapes: a synthesis. Ecol. Appl. 16, 2064–2090. doi: 10.1890/1051-0761(2006)016[2064:DALAWA]2.0.CO;2
Shedd, K. R., von Hippel, F. A., Willacker, J. J., Hamon, T. R., Schlei, O. L., Wenburg, J. K., et al. (2015). Ecological release leads to novel ontogenetic diet shift in kokanee (Oncorhynchus nerka). Can. J. Fish. Aquat. Sci. 72, 1718–1730. doi: 10.1139/cjfas-2015-0146
Sierszen, M. E., Peterson, G. S., and Scharold, J. V. (2006). Depth-specific patterns in benthic planktonic food web relationships in Lake Superior. Can. J. Fish. Aquatic Sci. 63, 1496–1503. doi: 10.1139/f06-057
Sigman, D., Karsh, K., and Casciotti, K. (2009). “Ocean process tracers: nitrogen isotopes in the ocean,” in Encyclopedia of Ocean Science, 2 Edn., eds J. H. Steele, K. K. Turekian, and S. A. Thorpe (San Diego: Academic Press), 4139–4152. doi: 10.1016/B978-012374473-9.00632-9
Sigman, D. M., Granger, J., DiFiore, P. J., Lehmann, M. M., Ho, R., Cane, G., et al. (2005). Coupled nitrogen and oxygen isotope measurements of nitrate along the eastern North Pacific margin. Glob. Biogeochem. Cycles 19. doi: 10.1029/2005GB002458
Simpson, I., Bol, R., Dockrill, S., Petzke, K. J., and Evershed, R. (1997). Compound-specific δ15N amino acid signals in palaeosols as indicators of early land use: a preliminary study. Archaeol. Prospect. 4, 147–152. doi: 10.1002/(SICI)1099-0763(199709)4:3<147::AID-ARP74>3.0.CO;2-5
Smith, F., and Walker, N. (1980). Photosynthesis by aquatic plants: effects of unstirred layers in relation to assimilation of CO2 and HCO3– and to carbon isotopic discrimination. N. Phytol. 86, 245–259. doi: 10.1111/j.1469-8137.1980.tb00785.x
Smith, V. H. (2003). Eutrophication of freshwater and coastal marine ecosystems a global problem. Environ. Sci. Pollut. Res. 10, 126–139. doi: 10.1065/espr2002.12.142
Solomon, C. T., Carpenter, S. R., Rusak, J. A., and Vander Zanden, M. J. (2008). Long-term variation in isotopic baselines and implications for estimating consumer trophic niches. Can. J. Fish. Aquatic Sci. 65, 2191–2200. doi: 10.1139/F08-125
Stenhouse, M., and Baxter, M. (1979). “The uptake of bomb 14C in humans,” in Radiocarbon Dating, eds R. Berger and H. Suess (Berkeley: University of California Press, 324–341.
Still, C. J., Berry, J. A., Collatz, G. J., and DeFries, R. S. (2003). Global distribution of C3 and C4 vegetation: carbon cycle implications. Glob. Biogeochem. Cycles 17. doi: 10.1029/2001GB001807
Street-Perrott, F. A., Ficken, K. J., Huang, Y., and Eglinton, G. (2004). Late Quaternary changes in carbon cycling on Mt. Kenya, East Africa: an overview of the δ13C record in lacustrine organic matter. Quat. Sci. Rev. 23, 861–879. doi: 10.1016/j.quascirev.2003.06.007
Stüeken, E., Buick, R., and Schauer, A. (2015). Nitrogen isotope evidence for alkaline lakes on late Archean continents. Earth Planet. Sci. Lett. 411, 1–10. doi: 10.1016/j.epsl.2014.11.037
Sudduth, E. B., Perakis, S. S., and Bernhardt, E. S. (2013). Nitrate in watersheds: Straight from soils to streams? J. Geophys. Res. Biogeosci. 118, 291–302. doi: 10.1002/jgrg.20030
Szpak, P. (2011). Fish bone chemistry and ultrastructure: implications for taphonomy and stable isotope analysis. J. Archaeol. Sci. 38, 3358–3372. doi: 10.1016/j.jas.2011.07.022
Szpak, P. (2014). Complexities of nitrogen isotope biogeochemistry in plant-soil systems: implications for the study of ancient agricultural and animal management practices. Front. Plant Sci. 5. doi: 10.3389/fpls.2014.00288
Szpak, P., Buckley, M., Darwent, C. M., and Richards, M. P. (2018). Long-term ecological changes in marine mammals driven by recent warming in northwestern Alaska. Glob. Change Biol. 24, 490–503. doi: 10.1111/gcb.13880
Szpak, P., Krippner, K., and Richards, M. (2017). Effects of sodium hydroxide treatment and ultrafiltration on the removal of humic contaminants from archaeological bone. Int. J. Osteoarchaeol. 27, 1070–1077. doi: 10.1002/oa.2630
Szpak, P., Longstaffe, F. J., Millaire, J.-F., and White, C. D. (2014). Large variation in nitrogen isotopic composition of a fertilized legume. J. Archaeol. Sci. 45, 72–79. doi: 10.1016/j.jas.2014.02.007
Szpak, P., Orchard, T. J., Salomon, A. K., and Gröcke, D. R. (2013). Regional ecological variability and impact of the maritime fur trade on nearshore ecosystems in southern Haida Gwaii (British Columbia, Canada): evidence from stable isotope analysis of rockfish (Sebastes spp.) bone collagen. Archaeol. Anthropol. Sci. 5, 159–182. doi: 10.1007/s12520-013-0122-y
Talbot, M. R. (2001). “Nitrogen isotopes in palaeolimnology,” in Tracking Environmental Change Using Lake Sediments: Physical and Geochemical Methods, eds W. M. Last and J. P. Smol (Dordrecht: Springer), 401–439. doi: 10.1007/0-306-47670-3_15
Talbot, M. R., and Johannessen, T. (1992). A high resolution palaeoclimatic record for the last 27,500 years in tropical West Africa from the carbon and nitrogen isotopic composition of lacustrine organic matter. Earth Planet. Sci. Lett. 110, 23–37. doi: 10.1016/0012-821X(92)90036-U
Talling, J. (1976). The depletion of carbon dioxide from lake water by phytoplankton. J. Ecol. 64, 79–121. doi: 10.2307/2258685
Tauber, H. (1981). 13C evidence for dietary habits of prehistoric man in Denmark. Nature 292, 332–333. doi: 10.1038/292332a0
Theissen, K. M., Hobbs, W. O., Hobbs, J. M., Zimmer, K. D., Domine, L. M., Cotner, J. B., et al. (2012). The altered ecology of Lake Christina: a record of regime shifts, land-use change, and management from a temperate shallow lake. Sci. Total Environ. 433, 336–346. doi: 10.1016/j.scitotenv.2012.06.068
Tsutaya, T., and Yoneda, M. (2015). Reconstruction of breastfeeding and weaning practices using stable isotope and trace element analyses: a review. Am. J. Phys. Anthropol. 156, 2–21. doi: 10.1002/ajpa.22657
Turner, T. F., Krabbenhoft, T. J., Collyer, M. I., Krabbenhoft, C. A., Edwards, M. S., and Sharp, Z. D. (2015). Retrospective stable isotope analysis reveals ecosystem responses to river regulation over the last century. Ecology 96, 3213–3226. doi: 10.1890/14-1666.1
Ulseth, A. J., and Hershey, A. E. (2005). Natural abundances of stable isotopes trace anthropogenic N and C in an urban stream. J. North Am. Benthol. Soc. 24, 270–289. doi: 10.1899/03-080.1
Vadeboncoeur, Y., Jeppesen, E., Zanden, M. J. V., Schierup, H.-H., Christoffersen, K., and Lodge, D. M. (2003). From Greenland to green lakes: cultural eutrophication and the loss of benthic pathways in lakes. Limnol. Oceanogr. 48, 1408–1418. doi: 10.4319/lo.2003.48.4.1408
van der Merwe, N. J., Williamson, R. F., Pfeiffer, S., Thomas, S. C., and Allegretto, K. O. (2003). The Moatfield ossuary: isotopic dietary analysis of an Iroquoian community, using dental tissue. J. Anthropol. Archaeol. 22, 245–261. doi: 10.1016/S0278-4165(03)00038-2
Vander Zanden, M. J., Casselman, J. M., and Rasmussen, J. B. (1999). Stable isotope evidence for the food web consequences of species invasions in lakes. Nature 401, 464–467. doi: 10.1038/46762
Vander Zanden, M. J., and Vadeboncoeur, Y. (2002). Fishes as integrators of benthic and pelagic food webs in lakes. Ecology 83, 2152–2161. doi: 10.1890/0012-9658(2002)083[2152:FAIOBA]2.0.CO;2
Vander Zanden, M. J., Vadeboncoeur, Y., and Chandra, S. (2011). Fish reliance on littoral-benthic resources and the distribution of primary production in lakes. Ecosystems 14, 894–903. doi: 10.1007/s10021-011-9454-6
Vander Zanden, M. J., Vadeboncoeur, Y., Diebel, M. W., and Jeppesen, E. (2005). Primary consumer stable nitrogen isotopes as indicators of nutrient source. Environ. Sci. Technol. 39, 7509–7515. doi: 10.1021/es050606t
Vandermyde, J. M., and Whitledge, G. W. (2008). Otolith δ15N distinguishes fish from forested and agricultural streams in southern Illinois. J. Freshwater Ecol. 23, 333–336. doi: 10.1080/02705060.2008.9664206
Veraart, A. J., De Klein, J. J., and Scheffer, M. (2011). Warming can boost denitrification disproportionately due to altered oxygen dynamics. PLoS ONE 6:e18508. doi: 10.1371/journal.pone.0018508
Vika, E., and Theodoropoulou, T. (2012). Re-investigating fish consumption in Greek antiquity: results from δ13C and δ15N analysis from fish bone collagen. J. Archaeol. Sci. 39, 1618–1627. doi: 10.1016/j.jas.2012.01.016
Vogel, J. C., and Van Der Merwe, N. J. (1977). Isotopic evidence for early maize cultivation in New York State. Am. Antiquity 42, 238–242. doi: 10.2307/278984
Vuori, K., Kiljunen, M., Kanerva, M., Koljonen, M. L., and Nikinmaa, M. (2012). Stock-specific variation of trophic position, diet and environmental stress markers in Atlantic salmon Salmo salar during feeding migrations in the Baltic Sea. J. Fish Biol. 81, 1815–1833. doi: 10.1111/j.1095-8649.2012.03386.x
Wainright, S. C., Fogarty, M., Greenfield, R., and Fry, B. (1993). Long-term changes in the Georges Bank food web: trends in stable isotopic compositions of fish scales. Mar. Biol. 115, 481–493. doi: 10.1007/BF00349847
Wang, B., Liu, C.-Q., Peng, X., and Wang, F. (2013). Mechanisms controlling the carbon stable isotope composition of phytoplankton in karst reservoirs. J. Limnol. 72:11. doi: 10.4081/jlimnol.2013.e11
Wayland, M., and Hobson, K. A. (2001). Stable carbon, nitrogen, and sulfur isotope ratios in riparian food webs on rivers receiving sewage and pulp-mill effluents. Can. J. Zool. 79, 5–15. doi: 10.1139/cjz-79-1-5
Werner, E. E., and Gilliam, J. F. (1984). The ontogenetic niche and species interactions in size-structured populations. Ann. Rev. Ecol. Syst. 15, 393–425. doi: 10.1146/annurev.es.15.110184.002141
Wheeler, T., and Von Braun, J. (2013). Climate change impacts on global food security. Science 341, 508–513. doi: 10.1126/science.1239402
Wiley, A. E., Ostrom, P. H., Welch, A. J., Fleischer, R. C., Gandhi, H., Southon, J. R., et al. (2013). Millennial-scale isotope records from a wide-ranging predator show evidence of recent human impact to oceanic food webs. Proc. Natl. Acad. Sci. 110, 8972–8977. doi: 10.1073/pnas.1300213110
Wolverton, S., and Lyman, R. L. (2012). Conservation Biology and Applied Zooarchaeology. Tucson, AZ: University of Arizona Press.
Wong, W. W., Benedict, C. R., and Kohel, R. J. (1979). Enzymic fractionation of the stable carbon isotopes of carbon dioxide by ribulose-1, 5-bisphosphate carboxylase. Plant Physiol. 63, 852–856. doi: 10.1104/pp.63.5.852
Yao, S., and Xue, B. (2015). Sedimentary geochemical record of human-induced environmental changes in Huanggaihu Lake in the middle reach of the Yangtze River, China. J. Limnol. 74, 31–39. doi: 10.4081/jlimnol.2014.957
Yoshii, K. (1999). Stable isotope analyses of benthic organisms in Lake Baikal. Hydrobiologia 411, 145–159. doi: 10.1023/A:1003810004451
Zambrano, L., Valiente, E., and Jake Vander Zanden, M. (2010). Stable isotope variation of a highly heterogeneous shallow freshwater system. Hydrobiologia 646, 327–336. doi: 10.1007/s10750-010-0182-2
Zanden, M. J. V., Chandra, S., Allen, B. C., Reuter, J. E., and Goldman, C. R. (2003). Historical food web structure and restoration of native aquatic communities in the lake tahoe (California-Nevada) basin. Ecosystems 6, 274–288. doi: 10.1007/s10021-002-0204-7
Zhang, L., Campbell, L. M., and Johnson, T. B. (2012). Seasonal variation in mercury and food web biomagnification in Lake Ontario, Canada. Environ. Pollut. 161, 178–184. doi: 10.1016/j.envpol.2011.10.023
Keywords: archaeology, palaeoecology, stable isotope, freshwater, paleodiet
Citation: Guiry E (2019) Complexities of Stable Carbon and Nitrogen Isotope Biogeochemistry in Ancient Freshwater Ecosystems: Implications for the Study of Past Subsistence and Environmental Change. Front. Ecol. Evol. 7:313. doi: 10.3389/fevo.2019.00313
Received: 10 June 2019; Accepted: 06 August 2019;
Published: 21 August 2019.
Edited by:
Florent Rivals, Catalan Institute for Research and Advance Studies (ICREA), SpainReviewed by:
Naoto F. Ishikawa, Japan Agency for Marine-Earth Science and Technology, JapanYuichi Naito, Nagoya University, Japan
Copyright © 2019 Guiry. This is an open-access article distributed under the terms of the Creative Commons Attribution License (CC BY). The use, distribution or reproduction in other forums is permitted, provided the original author(s) and the copyright owner(s) are credited and that the original publication in this journal is cited, in accordance with accepted academic practice. No use, distribution or reproduction is permitted which does not comply with these terms.
*Correspondence: Eric Guiry, ZWd1aXJ5JiN4MDAwNDA7bGFrZWhlYWR1LmNh