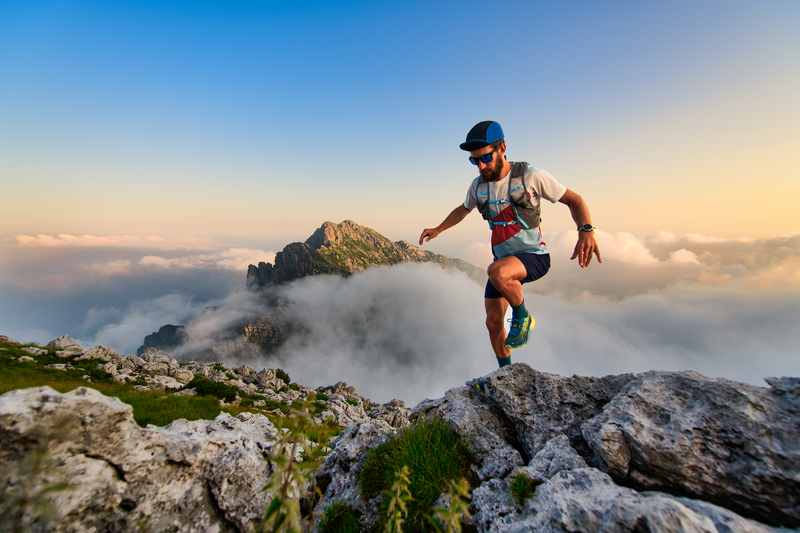
95% of researchers rate our articles as excellent or good
Learn more about the work of our research integrity team to safeguard the quality of each article we publish.
Find out more
HYPOTHESIS AND THEORY article
Front. Ecol. Evol. , 07 August 2019
Sec. Behavioral and Evolutionary Ecology
Volume 7 - 2019 | https://doi.org/10.3389/fevo.2019.00292
This article is part of the Research Topic How Enemies Shape Communication Systems: Sensory Strategies of Prey to Avoid Eavesdropping Predators and Parasites View all 13 articles
The study of tradeoffs between the attraction of mates and the attraction of eavesdropping predators and parasites has generally focused on a single species of prey, signaling in isolation. In nature, however, animals often signal from mixed-species aggregations, where interactions with heterospecific group members may be an important mechanism modulating tradeoffs between sexual and natural selection, and thus driving signal evolution. Although studies have shown that conspecific signalers can influence eavesdropper pressure on mating signals, the effects of signaling heterospecifics on eavesdropper pressure, and on the balance between natural and sexual selection, are likely to be different. Here, we review the role of neighboring signalers in mediating changes in eavesdropper pressure, and present a simple model that explores how selection imposed by eavesdropping enemies varies as a function of a signaling aggregation's species composition, the attractiveness of aggregation members to eavesdroppers, and the eavesdroppers' preferences for different member types. This approach can be used to model mixed-species signaling aggregations, as well as same-species aggregations, including those with non-signaling individuals, such as satellites or females. We discuss the implications of our model for the evolution of signal structure, signaling behavior, mixed-species aggregations, and community dynamics.
While the use of conspicuous sexual signals to attract mates is pervasive across animal taxa, these signals come with a cost (Olson and Owens, 1998; Andersson et al., 2002; Andersson and Simmons, 2006; Bradbury and Vehrencamp, 2011). In addition to attracting females, conspicuous signals are used by eavesdropping predators and parasites to home in on their prey (Cade, 1975; Soper et al., 1976; Endler, 1980; Ryan et al., 1982; Slagsvold et al., 1995; Zuk and Kolluru, 1998; Haynes and Yeargan, 1999; Bernal et al., 2006; Siemers et al., 2012; Steinberg et al., 2014). For sexual displays that attract females and eavesdroppers, the interaction between these two selective forces is profoundly important in shaping the evolution of signal structure and of signaling behavior (Endler, 1983; Zuk and Kolluru, 1998; Kotiaho, 2001). Moreover, the influence of female choice on signal evolution (typically for more conspicuous signaling) will often be at odds with that of pressures resulting from the attraction of eavesdroppers (typically for less conspicuous signaling; Endler, 1980; Goodale et al., 2019). In such cases, the locally adapted signal is the result of a balance between sexual selection imposed by females and natural selection imposed by eavesdropping enemies (Tuttle and Ryan, 1981; Endler, 1983, 1995a; Beckers and Wagner, 2012; Trillo et al., 2013).
Changes in eavesdropper pressure can significantly shift the balance between sexual and natural selection, and thus drive signal evolution. A common response to increased eavesdropper pressure is a plastic or evolutionary decrease in signal conspicuousness (Endler, 1980, 1983; Tuttle and Ryan, 1981; Ryan et al., 1982; Ruell et al., 2013; but see Beckers and Wagner, 2012). Decreased conspicuousness can be achieved through multiple means. Signalers can, for example, change their signaling behavior by timing their displays to moments of low eavesdropper abundance or activity (Endler, 1987; Gerhardt, 1994; Bertram et al., 2004; Velez and Brockmann, 2006), by reducing signaling activity (Tuttle et al., 1982; Cade and Wyatt, 1984; Cade, 1991; Jennions and Backwell, 1992; Gerhardt, 1994), or by switching to an alternative sensory modality (Morris, 1980; Morris and Beier, 1982; Belwood and Morris, 1987). They can also alter the signal itself by decreasing signal rate or duty cycle (Morris and Beier, 1982; Belwood and Morris, 1987; Halfwerk et al., 2018), by adding signal components (Stoddard, 1999), by subtracting signal components (Endler, 1980; Ryan et al., 1982; Trillo et al., 2013), or by ceasing to signal altogether (Zuk et al., 2006). In contrast, reduced eavesdropper pressure is expected to result in increasingly conspicuous and attractive signaling systems. A decrease in eavesdropper pressure due to urbanization, for example, significantly increased call complexity and calling rate in the Túngara frog, Physalaemus pustulosus (Halfwerk et al., 2018). Finally, the eavesdroppers' ability to shift the cost-benefit tradeoffs in mating signals can have longstanding consequences for lineage diversification if geographic variation in eavesdropper pressure influences signal divergence (Verrel, 1991; Hoskin and Higgie, 2010; Trillo et al., 2013).
Several factors can alter the pressures posed by eavesdroppers. Here we focus on nearby signalers as a key source of variation. While the effect that signaling conspecifics have on an individuals' eavesdropper risk has been well-studied (Cade, 1979; Ryan et al., 1981; Jennions and Backwell, 1992; Bernal et al., 2007; Alem et al., 2011), less is known about the role of signaling heterospecifics in modulating eavesdropper pressure, and thereby affecting the balance between natural and sexual selection (Trillo et al., 2016). Signaling aggregations in nature, however, are rarely homogeneous, and animals often signal from mixed-species groups (Morse, 1970; Kacelnik and Krebs, 1983; Sueur, 2002; Stensland et al., 2003; Phelps et al., 2006; Wells, 2010; Römer, 2013). Our aim in this article is to explore how signaling heterospecifics influence eavesdropper pressure in mixed-species mating aggregations. We first review studies that look at the influence of signaling conspecific and heterospecific neighbors on the risks posed by eavesdropping enemies. Next, we present a model that can be used to predict how changes in an individual's predation and parasitism risks depend on the relative attractiveness, identity, and density of its neighbors. In the final section, we discuss the influence of heterospecific signalers on the evolution of signal structure, signaling behavior, mixed-species aggregations, and community dynamics.
Proximity to conspecifics can alter the selective pressures acting on a signaling individual (Jennions and Backwell, 1992; Bernal et al., 2007). On one hand, the presence of signaling conspecifics can enhance eavesdropper attraction if they increase encounter rates (Shelly, 2018). On the other hand, the presence of signaling conspecifics can reduce predation or parasitism risk and alleviate eavesdropper pressure via increased detection abilities (Pulliam, 1973; Van Schaik et al., 1983; Dehn, 1990), the dilution effect (Hamilton, 1971; Williams et al., 1993), the confusion effect (Landeau and Terborgh, 1986; Goodale et al., 2019), or any combination of these mechanisms. Studies on chorusing frogs and lekking moths, found that while an increase in group size did not change the number of eavesdroppers attracted to an aggregation, it did decrease per capita predation risk. In these cases, group size also influences male mating probability because females are disproportionally more attracted to larger groups (i.e., female-to-male ratio increases; Ryan et al., 1981; Alem et al., 2011). Thus, both studies argue that decreased predation costs paired with increased mating benefits might explain the evolution of communal displays in frogs and insects.
Prey foraging in mixed-species groups have been widely documented to enjoy reductions in predation risk (Grand and Dill, 1999; Stensland et al., 2003; Sridhar et al., 2009), but less is known about how inter-species dynamics influence risks to prey in signaling aggregations. In fact, until recently, studies of eavesdropping enemies and their effects on mating signals, have focused on single species of prey, signaling in isolation (Trillo et al., 2016). In nature, however, males advertising for mates often signal from mixed-species aggregations. Such aggregations are common in birds, frogs, and insects (Sueur, 2002; Marler and Slabbekoorn, 2004; Phelps et al., 2006; Wells, 2010; Römer, 2013). Mixed signaling aggregations may occur as a byproduct of habitat heterogeneity, if signaling species share similar requirements for mating and larval habitats and these habitats are patchily distributed (Downes, 1969; Davies, 1977; Macedo and DuVal, 2018). Additionally, selection pressure from eavesdropping enemies might also promote the evolution of mixed-species signaling aggregations in the same way that it promotes the evolution of single-species aggregations (Goodale et al., 2019).
The roles of eavesdroppers in modulating trade-offs between sexual and natural selection are likely to be amplified in mixed-species aggregations. Females are under strong selection to detect and choose males of their own species, and pairings with heterospecific males are likely to be restricted to discrimination or localization errors (Pfennig, 2000; Bonachea and Ryan, 2011). Eavesdroppers, however, are usually not as restrictive in their prey selection, and can often benefit from attacking a broad range of prey. As a result, predator and parasite attention attracted by a given signal can spill over to neighboring prey irrespective of species. Through this mechanism, predation and parasitism risks associated with eavesdropping are especially likely to transfer between neighbors in mixed-species aggregations, shifting the balance between natural and sexual selection.
While some eavesdroppers can be relatively permissive with their choice of prey, it is important to note that not all individuals within an aggregation are necessarily equal in the eyes, or ears, of predators and parasites homing in on their signals. We consider asymmetries in neighbor-mediated eavesdropper risk at two levels. First, eavesdroppers searching for an aggregation may be more or less attracted to different kinds of signaling prey. Then, after an eavesdropper arrives at an aggregation, it may preferentially attack one prey species over another. We label these two phases of choice as “attraction” and “preference,” respectively. Both definitions are sufficiently broad to include not only cognitive decisions, but also other components of choice such as the localizability of signals. Variation in the relative attractiveness of prey types may result in changes to the overall number of eavesdroppers attracted to the aggregation. Moreover, when eavesdropper preferences are asymmetrical, there may be a net transfer of eavesdropper attention between adjacent signalers. While these inequalities in attractiveness and preference can occur in conspecific aggregations, they are likely to be more important in mixed-species groups, where signals will differ substantially between prey species (Sueur, 2002; Schmidt et al., 2013; but see Tobias et al., 2014).
Signalers in an aggregation can alter eavesdropper risks faced by their neighbors in one of two ways. Signaling neighbors can increase the predator or parasitism risks of a focal individual, thus conferring “collateral damage” upon the focal. Alternatively, signaling neighbors can decrease the risks for a focal individual, thus providing a “shadow of safety” (Trillo et al., 2016). These terms have previously been applied to situations in which both the focal individual and the neighbor are signaling, and those in which the neighbor is substantially more attractive to eavesdroppers. For the purpose of this paper, we will consider the definitions of “collateral damage” and “shadow of safety” as sufficiently broad to also encompass situations in which the neighbor is not signaling, and in which the neighbor is less attractive to, or less preferred by, eavesdroppers than is the focal individual. In a study of P. pustulosus (túngara frogs) and Dendropsophus ebraccatus (hourglass treefrogs), Trillo et al. (2016) found a three-fold increase in the number of blood-sucking midges attracted to playbacks of hourglass treefrog calls when these calls were played near those of túngara frogs, demonstrating that proximity to heterospecific signalers can drastically alter the risks of signaling (in this case, through collateral damage). Similarly, Segami et al. (2016) found an increase in predation risk to cryptic female strawberry poison frogs that associated with conspicuous, aposematically colored males.
Given that mixed-species aggregations are common, we expect the previously described risk transfer mechanisms to be prevalent, and to thus be an important source of variation in the balance between natural and sexual selection acting on mating signals. Below, we describe a model that predicts the eavesdropper pressures faced by a focal signaler in an aggregation consisting of two types of prey. The model permits variation in the number of signalers of each prey type, the attractiveness of each type to mutual enemies, and the enemies' relative preference for the two types of signalers.
Our simple mathematical model estimates the number of enemies that are expected to attack a focal individual in an aggregation that contains two types of signalers: type 1 and type 2 (Table 1). We model the number of enemies that attack an individual of type 1 (EF) as the product of the total number of enemies that are attracted to the aggregation (EC) and the proportion of those enemies that attack the focal individual (PF, Equation 1). This can be considered an extension of earlier attempts to model conspecific aggregations by breaking down the costs and benefits of group living into the product of the rate of enemy encounters with an aggregation and the dilution of the resulting risk amongst group members (Wrona and Dixon, 1991). As with earlier efforts, the number of attacks on a focal signaler described in our model will be strongly influenced by the number of eavesdroppers attracted to an aggregation, and the dilution of risks they pose to prey. Our model also enables users to examine the effects of asymmetries on the attractiveness of, and eavesdropper preferences for, neighboring signalers.
We model the number of eavesdropping enemies attracted to the aggregation (EC) as a function of the number of prey individuals of each type in the aggregation (N1 and N2), their attractiveness to distant eavesdroppers (Q and R), and the relationship (S) between the weighted number of prey individuals and the number of attracted eavesdroppers (Equation 2). The term “R” scales type 2's attractiveness relative to type 1's. For example, R = 2 if one individual of type 2 attracts twice as many eavesdroppers as does one individual of type 1. Then “Q”, which is the number of enemies attracted to a single type 1 individual, scales the attractiveness of both types, weighted by R. For example, Q might be <1 when modeling the attraction of a rare enemy (e.g., a predatory mammal), because the probability of even a single attack is low. By contrast, when modeling the attraction of a common enemy (e.g., a blood-sucking parasite), Q would be high, because an individual prey can expect multiple attacks. The exponent “S” is discussed in more detail below (see section “Assumptions”).
We estimate the proportion of eavesdropping enemies at the aggregation that attack an individual of type 1 (PF) as a function of the number of type 1 and type 2 individuals and the enemies' relative preference for prey of type 1 vs. type 2 (Equation 3). The term “P1” describes eavesdroppers' expressed preference for type 1 relative to type 2 prey (see section “Assumptions”).
Which reduces to the following:
We substitute Equations (2, 3) into Equation (1) to arrive at the full model (Equation 4).
Which reduces to the following:
For this model, we assume that each eavesdropper attracted to the aggregation attacks exactly one group member. Alternatively, we can consider the attraction phase to apply only to those enemies that eventually attack, as long as no enemies attack more than one prey. This assumption is likely to be valid for parasites, including parasitoids, and smaller predators that only consume one prey before moving on. The model would need to be modified to accommodate larger predators that consume more than one prey at a time, or to include multiple attacks by a single eavesdropper resulting from failure to capture prey on the first attack. One way to do this is to conceptualize EC as the number of “attacks” attracted to the aggregation and EF as the number of attacks per individual.
We also assume that eavesdropping enemy attraction follows an exponential function, permitting a range of response curves from flat (S = 0), through positive decelerating (0 < S < 1), linear (S = 1), and positive accelerating (S > 1). Values of S < 1 may be used, for example, to indicate environmental constraints on the numbers of eavesdropping enemies that can be attracted to the aggregation of signaling prey. In this case, the number of eavesdroppers attracted to the signaling aggregation increases with the size of the aggregation, but the marginal increase in the number of eavesdroppers attracted diminishes as the pool of potential enemies is exhausted. Attraction curves that cannot be represented by an exponential function, such as asymptotic or sigmoidal curves, could be accommodated with a slight modification to the model.
Importantly, our model assumes that both types of signalers have similarly-shaped enemy attraction functions that can be combined into a single function. This assumption does not hold in cases where signals produced by one species may directly interfere with eavesdropper perception of a second species (Simmons et al., 1971), or where the signaling behavior of one species may be influenced by that of another (Schwartz and Wells, 1984). The complexities of these interactions are beyond the scope of our simple model. Finally, we assume that the probability of attacking any one signaler is random, implying that signalers of types 1 and 2 are homogenously mixed in space. This assumption, of course, is often violated in nature (e.g., Given, 1990). This model considers only one kind of enemy at a time. However, multiple models could be used to assess multiple enemies.
It is important to note that although P1 is defined as a preference, it encompasses the overall relative probability of an eavesdropper attacking a type 1 individual vs. a type 2 individual. This is because P1 is not simply equivalent to the eavesdropping enemy's performance in an unconstrained two-choice test. P1 is also influenced by any constraints on the enemy's ability to attack one or both types of prey. For example, an enemy may “prefer” type 1 in a two-choice test, but if type 1 individuals are difficult to locate or difficult to attack because of their microhabitat, P1 could be < 1, indicating a higher probability of attacking type 2 than type 1.
Our model can be used to make predictions about EF, the number of attacks on an individual of type 1. We summarize the main results of the model in Table 2. Here and in the following sections, we set Q = 1. Q is useful for scaling real data, but it does not affect the relationships between the variables and predictions (this can be inferred from Equation 4). We begin by letting R = 1, so that type 1 and type 2 prey are equally attractive, P1 = 1, so that they are equally preferred, and S = 1, so that enemies scale linearly with the number of prey in the aggregation. With these parameters, EF is independent of N1 and N2 (Equation 4), meaning that attacks on an individual of type 1 are independent of the numbers of type 1 and type 2 individuals. If we substitute S = 0.5 (or any non-negative value < 1), so that marginal attraction decreases with the number of chorus members, EF becomes negatively related to both N1 and N2, demonstrating a dilution effect on eavesdropper pressure (Figure 1A; Hamilton, 1971). In this scenario, which assumes equal attraction and preference between prey types, the presence of other signalers, regardless of type, confers a shadow of safety effect on type 1 signalers.
Figure 1. Representative output from a model that predicts the number of eavesdroppers that will attack a focal individual (EF) of prey type 1, in an aggregation containing varying numbers of type 1 and type 2 signaling prey. Each frame presents predictions based on different values of the relative attractiveness of the two prey types (R), the eavesdroppers' expressed preference for type 1 prey (P1), and the relationship between the weighted number of prey and the number of eavesdroppers attracted to the aggregation (S). (A–E) represent EF on a scale from 0 to 2 (scale bar on upper right), and (F) represents EF on a scale from 0 to 10 (scale bar on lower right). See text for details.
When we allow R, the relative attractiveness of types 1 and 2, to differ and set all other constants to one, the relationships between the variables N1 and N2, and the prediction EF can be interpreted as the consequences of variation in the per capita attractiveness of the chorus. We begin by simulating a situation in which type 2 is less attractive to enemies than type 1 by lowering R to 0.5, while the other constants are 1. Under these conditions, the model predicts a positive, decelerating relationship between N1 and EF, because the per capita attractiveness of the chorus increases with N1 (Figure 1B). The relationship between N2 and EF is negative because increasing numbers of type 2 individuals decreases the per capita attractiveness of the chorus. Thus, lowering the attractiveness of type 2 prey promotes a shadow of safety effect: Type 1 prey that associate with type 2 prey can expect fewer attacks than those that associate with an equal number of type 1s or display alone. If we set R = 2, type 2 prey are more attractive than type 1 prey, and the opposite pattern emerges: EF decreases to an asymptote with increasing N1, and increases to an asymptote with increasing N2, tracking the per capita attractiveness of the chorus, such that the presence of type 2 prey promotes collateral damage on type 1 prey (not shown). Holding Q and S constant, the overall magnitude of EF tracks R (compare Figures 1B,C). In real-world applications Q could be used to correctly scale eavesdropper attraction.
Taking a more extreme approach, we can set R = 0, to simulate a system in which type 2 individuals do not attract enemies to the aggregation but may still be attacked. Parameterizing the model this way estimates risks to a signaling individual in an aggregation that includes non-signaling “satellite” males (Rowell and Cade, 1993), females (Segami et al., 2016), or callers of a second species whose calls do not attract eavesdroppers at a distance (e.g., because they are imperceptible to the eavesdroppers). The predictions are similar, but more extreme than the R = 0.5 example described above: a steeply positive, decelerating relationship between N1 and EF, and a steeply negative, decelerating relationship between N2 and EF (Figure 1C). In this scenario, type 2 prey cast a powerful shadow of safety on type 1 prey, because they do not attract additional enemies, but they absorb some of the attacks from enemies attracted by type 1 prey.
Setting P1, the eavesdropper's expressed preference to attack individuals of type 1 vs. 2, to values less than one promotes a shadow of safety effect on type 1, whereas P1 > 1 promotes collateral damage to prey of type 1 if type 2 signalers also attract eavesdroppers. We begin with all constants set to one, except for P1, which we set to 2 to represent a system in which predators' preference for type 1 individuals is double their preference for type 2s (Figure 1D). For any given combination of N1, N2, and R, EF is higher than in the condition P1 = 1, because enemies attracted to the chorus preferentially attack type 1 individuals. In this case we see that EF decreases with N1 and increases with N2, meaning that type 2s inflict collateral damage on type 1s, by attracting eavesdroppers that disproportionally attack type 1s. If we instead decrease P1 to 0.5, we simulate eavesdroppers that prefer to attack type 2s, and observe the opposite patterns: EF increases with N1 and decreases with N2, because the shadow of safety effect on type 1 increases with the proportional representation of type 2 individuals (figure not shown).
Altering S changes the relationship between the scaled number of members in the aggregation, and the enemies attracted to the aggregation. Lower values of S tend to favor shadow of safety effects on type 1 prey. Setting S = 0 results in flat relationships between the number of enemies attracted to the chorus (EC) and the variables N1 and N2, simulating a system in which the number of signalers does not influence the number of enemies attracted to the chorus. This configuration could represent situations where eavesdropping enemies are territorial, are limited by an external ecological factor, or follow a fixed foraging route. When we set S = 0 and all other constants to one, we find negative decelerating relationships between EF and both variables N1 and N2 (Figure 1E). Under these conditions, a fixed number of enemies attend the aggregation, so type 1 prey benefit from the dilution effect generated by adding group members of either type. Similarly, values of S between zero and one result in negative decelerating relationships between EF and both N1 and N2, because each additional chorus member results in diminishing marginal gains of enemies (EC is positive decelerating; Figure 1A). Setting S > 1 results in positive relationships between EF and N1 and N2 (Figure 1F). The shape of these relationships depend on the magnitude of S: positive decelerating when 1 < S < 2, positive linear when S = 2, and positive accelerating when S > 2.
In the previous section, we manipulated one parameter at a time to show that type 2 prey tend to cast a shadow of safety on type 1 prey when R, P1, and S are low. Here, we use the model to visualize how variation in R and P1 affect the consequences of signaling near one other individual (for this example Q = 1 and S = 1). We begin with a focal type 1 individual signaling alone, and examine the fitness consequences to that individual of adding one type 2 signaler. This can be achieved by calculating the difference in EF for N1 = 1 and N2 = 0 vs. N1 = 1 and N2 = 1, and plotting that difference (ΔEF) over the parameters R and P1 (Figure 2). Compared to signaling alone, signaling near a heterospecific can confer either a shadow of safety or collateral damage, depending both on the difference in attractiveness between the two species (R) and the difference in the enemies' expressed preferences once they arrive at the aggregation (P1).
Figure 2. The predicted change in eavesdropper pressure (ΔEF) on an individual signaler resulting from the introduction of a neighboring individual. The change in eavesdropper pressure is predicted to vary with (A) the relative attractiveness of the two types of prey to distant enemies and (B) the enemy's relative preference for the two types of prey. Values of ΔEF > 0 indicate that the presence of the neighbor inflicts collateral damage on the focal individual (pink shading), and ΔEF < 0 indicate that the neighbor casts a shadow of safety on the focal signaler (blue shading).
Animals displaying to attract mates commonly seek out or find themselves in aggregations with other signalers. In many cases, these displays also attract enemies in the forms of predators or parasites, and the evolution of signal structure and signaling behavior will be shaped by the balance between attraction of mates and these dangerous eavesdroppers. Here, we have provided a framework for understanding how differences between neighboring signalers can alter eavesdropper risks faced by signalers of a given type. Our model breaks eavesdropper pressure down into two components: the attraction of enemies to an aggregation, and the preferences of enemies for certain prey within that aggregation. We show that, although they have rarely been considered, asymmetries within these attraction and preference phases are vital for understanding the risks faced by prey signaling in mixed-species groups.
When members of a signaling aggregation are similarly attractive to eavesdroppers, and these eavesdroppers show no preference to attack one prey type over another, increasing group size will equally affect the risks faced by all group members. Thus, when the per capita number of eavesdroppers attracted scales negatively with group size (e.g., when S < 1), all group members will enjoy an equal reduction in eavesdropper risk due to dilution. When, instead, the per capita number of eavesdroppers attracted scales positively with group size (e.g., when S > 1), for example when large groups lead to higher encounter rates with eavesdroppers, all group members will suffer an equal increase in risk. In nature, however, group members might not be equally attractive to, or equally preferred by, eavesdroppers. In these cases, when prey attractiveness or preference are asymmetrical, individual prey may experience additional reductions or increases in eavesdropper pressure (shadow of safety or collateral damage, respectively) that can counteract or overwhelm group-wide dilution and encounter rate effects (Figure 2).
Collateral damage and shadow of safety effects resulting from asymmetries in eavesdropper attraction and preference have the potential to shape the evolution of signaling species in several interrelated ways. These include the emergence and composition of mixed-species signaling aggregations, the spatial distribution of signalers within an aggregation, the timing of signaling activity relative to neighbors, the structure of signals themselves, and even the geographic ranges of signaling species.
Each of the well-established adaptive explanations for the origin and maintenance of animal aggregations are also likely relevant to mixed-species groups of signalers. Larger, more complex groups may reduce risk faced by their members through predator dilution, enhanced detection abilities, and the confusion effect (Hamilton, 1971; Pulliam, 1973; Landeau and Terborgh, 1986; Gibson et al., 2002; Goodale et al., 2019). Our model suggests, however, that in order to understand eavesdropper risk faced by an individual in such an aggregation, asymmetries in the attraction to, and preference for, prey signals must also be considered.
Shadow of safety benefits resulting from asymmetries in eavesdropper attention (attraction and preference) are likely to combine with those due to dilution effects, and influence some prey to seek out aggregations with other species. In many cases, signalers displaying in proximity to relatively unattractive or highly preferred heterospecific neighbors will enjoy reduced eavesdropper pressure (Figures 2A,B). Thus, associations with unattractive or highly preferred heterospecific neighbors should encourage the evolution of mixed-species signaling groups. It should be noted, however, that the formation of such aggregations may not be evolutionarily stable if the shadow of safety benefits enjoyed by signalers of one species result in significant collateral damage to individuals of the other species.
Similarly, asymmetries in eavesdropper attraction and preferences are likely to modulate the benefits resulting from the confusion effect. Often considered for visually-oriented predators, the confusion effect describes situations in which the perceptual challenges faced by predators select for larger groups of prey (Pavlov and Kasumyan, 2000; Goodale et al., 2019). In many ways analogous to the “cocktail party” problem discussed for receivers in conspecific signaling contexts (Bee and Micheyl, 2008), these challenges are likely to be increased when additional signals produced by heterospecifics are added to the foraging tasks confronting eavesdroppers (see Goodale et al., 2019 for a review of this). This should result in reduced risks faced by prey, on average, within a group. However, as eavesdroppers attempt to integrate information from a wider range of sources in order to mitigate perceptual challenges (Schmidt et al., 2010), they are more likely to attend to characteristic differences between prey types. Túngara frogs, for example pair calls with conspicuous alternating inflations of their vocal sacs and flanks, while simultaneously producing ripples at the surface of the puddles from which they signal. Bat predators of these frogs are known to increasingly rely on echolocation cues rather than passive eavesdropping in contexts of high heterospecific chorus noise (Rhebergen et al., 2015). In this context, we might expect exaggerated preferences on the part of eavesdropping enemies for túngara frogs over heterospecific neighbors who produce more subtle modulations of their vocal sacs. Any apparent confusion benefits resulting from signaling within a mixed group, should thus, be weighed against collateral damage suffered by more conspicuous prey.
From the perspective of an individual deciding when and where to display within a mixed-species aggregation, neighbor-mediated eavesdropper risks are an important consideration. Whether it is more adaptive to signal in close proximity to, or distant from a heterospecific signaler will depend, in part, on whether eavesdropper risks will be transferred on net to (shadow of safety) or from (collateral damage) this potential neighbor. Similarly, neighbor-mediated eavesdropper risk may refine the timing of signaling behavior relative to that of signaling neighbors.
Signalers have been shown to adjust the timing or structure of their displays in response to those of heterospecific neighbors, but the role of eavesdroppers in the evolution of these aspects of signaling has generally not been addressed. Hourglass treefrogs (D. ebraccatus), for example, call less frequently and with fewer notes when neighboring small-headed treefrogs (D. microcephalus) are chorusing loudly (Schwartz and Wells, 1983). Modulations of signal structure and signaling behavior such as this are undoubtedly influenced by competition among males to maximally attract females. That said, this type of modulation is also consistent with the responses to changing neighbor-mediated eavesdropper pressures.
While the literature addressing eavesdropper effects on the structure of sexual signals is growing steadily, the role of signaling neighbors in shaping the evolution of signal structure is relatively unexplored. When focal signalers experience collateral damage, we can expect the balance between mate-choice and eavesdropper selection to shift in the direction of less conspicuous or less localizable signals. We expect the opposite shift when signalers experience shadow of safety benefits.
In some cases, collateral damage induced by the presence of nearby signalers may affect a species' geographic distribution. If one species of prey attracts sufficient numbers of enemies to an area, it may lead to reductions in the abundance or even extirpation of other, more vulnerable, species. This possibility is well-explored in the apparent competition literature (Holt, 1984; Holt and Bonsall, 2017). Our model suggests that asymmetries in the vulnerabilities of prey to eavesdroppers, specifically resulting from their proximity at signaling aggregations could function as one important mechanism by which apparent competition influences the composition of prey communities. While there already exists empirical support for the idea that geographic heterogeneity in eavesdropper risk can shape the distribution of species or promote signal divergence (Endler, 1995b; Trillo et al., 2013), the possibility that heterospecific neighbors mediate these effects remains unexplored.
Signalers that attract greater numbers of eavesdroppers to an aggregation may also be preferred by enemies choosing among prey/hosts within that aggregation. The contrasting situation, in which one type of signaler is more attractive to eavesdroppers at a distance but the other type is preferred at closer range is also plausible. The active spaces of signals produced by prey species may differ, and eavesdroppers attracted to a widely broadcast signal may prefer another signal with a more restricted active space once in range to assess both. Marbled newts (Triturus marmoratus) eavesdrop on the calls of natterjack toads (Epidalea calamita) to locate breeding sites, but short-range visual, and likely chemical, signals are used by the newts during courtship (Diego-Rasilla and Luengo, 2004). Moreover, some predators and parasites also switch to alternative sensory modalities when assessing signalers at close distance (Hendrichs et al., 1994; Page et al., 2012). Frog-eating bats (Trachops cirrhosus) use passive auditory cues at relatively long distances, but may switch to echolocation cues at intermediate distances, and ultimately base prey acceptance or rejection decisions on chemical cues (Page and Jones, 2016). As these bats can also switch their foraging decisions to nearby prey in fractions of a second before an attack (MSC, in prep), it seems plausible to conclude that, for this species, long distance attraction and short distance preferences may not always be in line with each other.
The focus of our analysis has been on eavesdropper-mediated relationships between heterospecific signalers, but the general framework we set out can be readily extended to conspecifics displaying with differing signal variants, or to non-signaling conspecifics, such as females or satellite males. Individuals of types 1 and 2 in our model can represent distinct conspecific signal variants within an aggregation without any changes to the model parameters. Eavesdropper risks suffered by these signal variants can then be understood not only in the isolated two-choice contexts under which they are often studied (Ryan et al., 1982; Bernal et al., 2006; Trillo et al., 2013), but as interacting elements within a greater landscape of risk.
Our model also predicts the effects that non-signaling individuals have on the risks faced by signalers. The relative attractiveness of these individuals (R) is likely to be close to zero, but eavesdropper risk may still spill over to them at close ranges (i.e., P1 ≠ ∞). Risk to non-callers is expected to be greatest when enemies switch to alternative sensory modalities for close-range prey detection. Overall, satellite males and females are likely to result in some degree of shadow of safety for signaling males, as they do not attract additional enemies to the aggregations, and therefore reduce eavesdropper risks through dilution. We expect this shadow of safety to be augmented under conditions in which enemies face substantial perceptual challenges, such as loud chorus noise, and therefore suffer from reduced localization acuity (Caldwell and Bee, 2014; Rhebergen et al., 2015).
Although we have limited our discussion to one side of the trade-off between mate-choice and eavesdropper pressure, the model we present here should be equally adept at predicting the effects of neighboring heterospecific and conspecific signalers on female attention garnered by a focal signaler. Just like predators and parasites, females must first navigate to a signaling aggregation, and then choose between individuals at that aggregation. While the terms “collateral damage” and “shadow of safety” may not seem as relevant to a mate-choice context, the concepts are equally applicable. When signaling next to an attractive neighbor who is not highly preferred, a focal signaler can expect to receive “collateral damage” in the form of an increased number of female encounters. This appears to be the case for some frogs. Male Rhacophorus prasinatus associate with highly attractive males while signaling, presumably to benefit from “collateral damage” resulting from the increased local attraction of females (Chang et al., 2018). Ultimately, one could simultaneously model both female choice and eavesdropper risk, given asymmetries in attraction and preference for signalers within an aggregation.
We have chosen to keep our model somewhat simple to highlight its key aspects—namely that the number of eavesdroppers drawn to an aggregation will vary with the composition of the aggregation, that eavesdroppers attracted to an individual signaler may ultimately attack nearby guild members, and that eavesdropper risks faced by individual signalers are a function of both of these. There are many ways in which the model could be elaborated to more closely match the complexities of communication in nature. One such elaboration would be to include probabilities of prey capture success. While Ef predicts the number of eavesdropper attacks on a focal signaler, not all of these attacks will result in predation or parasitism (or copulation if modeling mate-choice). Furthermore, due to physical, chemical, or behavioral defenses, prey will differ in their ability to evade attacks. The dynamic behavior of both signalers and eavesdroppers could also be fruitful to model. For some species, signaling behavior is likely not independent of group size or composition. Túngara frogs, for example, are known to modify their call structure depending on the abundance of conspecifics, and alter their calling behavior depending on the signaling activity of heterospecifics (Phelps et al., 2006). Finally, we model fixed attractiveness of, and eavesdropper preferences for each type of prey. Eavesdropper behavior may change, however, with the absolute or relative abundances of signalers. Depletion of prey within an aggregation and prey switching, for example, may strongly affect the nature of predator-mediated intraspecific interactions (Holt and Kotler, 1987).
Collateral damage and shadow of safety effects predicted by our model can be viewed as mechanisms resulting in the widely modeled phenomena of “apparent competition” and “apparent mutualism” (Holt, 1977; Holt and Bonsall, 2017). These broader concepts include nearly any interactions between species mediated by a common predator. While the literature on apparent competition has focused on instances where the presence of one species negatively affects the abundance of the other by increasing predation on the second species (Holt and Bonsall, 2017), apparent competition theory itself is sufficiently broad to include positive interactions between guild members and alterations in predation risk, rather than changes in prey abundance (Holt and Kotler, 1987; Holt and Bonsall, 2017). Some places where our model extends beyond the traditional bounds of apparent competition theory are in the cases of eavesdropper-mediated interactions between conspecifics, and in the attraction of females, rather than predators.
We have presented a framework and a simple model for understanding how asymmetries in the attraction of eavesdroppers to an aggregation, and in their preferences for certain signalers within this aggregation can result in either heightened or relaxed risks for signalers. We expect the resulting collateral damage and shadow of safety effects to influence the evolution of signal structure, the spatial and temporal distributions of species within signaling aggregations, the species composition of such aggregations, and potentially the geographic distributions of signaling species.
Moving forward, several exciting paths for future research appear particularly promising. First, thus far, there are few empirical demonstrations of collateral damage or shadow of safety effects stemming from asymmetries in predator responses to prey (Segami et al., 2016; Trillo et al., 2016). Considering the near ubiquity of mixed-species aggregations and of eavesdropping enemies, these phenomena could be explored for much a wider range of taxa. Furthermore, while our model was conceived as a conceptual exploration of eavesdropper risks mediated by heterospecific signalers, its predictive power can be tested with natural populations. Importantly, we designed the parameters to be either directly measurable or their calculation to be straightforward, facilitating quantitative predictions. Finally, the model can be applied to examine the influence of neighboring signalers on female mate choice or extended to simultaneously include both females and eavesdroppers.
PT contributed the original concept. DL developed the initial mathematical model. PT, CB, MC, and DL further revised and troubleshot the mathematical model. PT, CB, MC, TL, OP, and DL contributed sections of the manuscript. All authors contributed to manuscript revision, read, and approved the submitted version.
This research was funded in part by a Natural Sciences and Engineering Research Council of Canada Discovery Grant (2015-06553) to DL, and a Gettysburg College Research and Professional Development Grant to PT. OP was funded with a Gettysburg College Cross-Disciplinary Science Fellowship.
The authors declare that the research was conducted in the absence of any commercial or financial relationships that could be construed as a potential conflict of interest.
We thank Dr. Darren Glass, Dr. Robert Laird, Samantha Krause, and Peter Mower for their input and advice during the conceptual and mathematical development of the model. We thank the two referees, for their extensive and helpful comments. We also want to thank Lucy Marks, Juliet Aguilera, and Merlyn Maldonado for taking care of PT and MC's children for several weekends during the preparation of this manuscript.
Alem, S., Koselj, K., Siemers, B. M., and Greenfield, M. D. (2011). Bat predation and the evolution of leks in acoustic moths. Behav. Ecol. Sociobiol. 65, 2105–2116. doi: 10.1007/s00265-011-1219-x
Andersson, M., and Simmons, L. W. (2006). Sexual selection and mate choice. Trends Ecol. Evol. 21, 296–302. doi: 10.1016/j.tree.2006.03.015
Andersson, S., Pryke, S. R., Ornborg, J., Lawes, M. J., and Andersson, M. (2002). Multiple receivers, multiple ornaments, and a trade-off between agonistic and epigamic signaling in a widowbird. Am. Natural. 160, 683–691. doi: 10.1086/342817
Beckers, O. M., and Wagner, W. E. J. (2012). Eavesdropping parasitoids do not cause the evolution of less conspicuous signalling behavior in a field cricket. Anim. Behav. 84, 1457–1462. doi: 10.1016/j.anbehav.2012.09.016
Bee, M. A., and Micheyl, C. (2008). The cocktail party problem: what is it? How can it be solved? And why should animal behaviorists study it? J. Compar. Psychol. 122, 235. doi: 10.1037/0735-7036.122.3.235
Belwood, J. J., and Morris, G. K. (1987). Bat predation and its influence on calling behavior in neotropical katydids. Science 238, 64–67. doi: 10.1126/science.238.4823.64
Bernal, X. E., Page, R. A., Rand, A. S., and Ryan, M. J. (2007). Cues for eavesdroppers: do frog calls indicate prey density and quality? Am. Natural. 169, 409–415. doi: 10.1086/510729
Bernal, X. E., Rand, A. S., and Ryan, M. J. (2006). Acoustic preferences and localization performance of blood-sucking flies (Corethrella Coquillett) to tungara frog calls. Behav. Ecol. 17, 709–715. doi: 10.1093/beheco/arl003
Bertram, S. M., Xochitl Orozco, S., and Bellani, R. (2004). Temporal shifts in conspicuousness: mate attraction displays of the Texas field cricket, Gryllus texensis. Ethology 110, 963–975. doi: 10.1111/j.1439-0310.2004.01031.x
Bonachea, L. A., and Ryan, M. J. (2011). Predation risk increases permissiveness for heterospecific advertisement calls in tngara frogs, Physalaemus pustulosus. Anim. Behav. 82, 347–352. doi: 10.1016/j.anbehav.2011.05.009
Bradbury, J. W., and Vehrencamp, S. L. (2011). Principles of Animal Communication, 2nd Edn. Sunderland, MA: Sinauer Associates.
Cade, W. (1975). Acoustically orienting parasitoids: fly phonotaxis to cricket song. Science 190, 1312–1313. doi: 10.1126/science.190.4221.1312
Cade, W. (1979). “The evolution of alternative male strategies in field crickets,” in Sexual Selection and Reproductive Competition in Insects. eds N. Blum and M. Blum (New York, NY: Academic Press), 343–390.
Cade, W. (1991). Inter- and intraspecific variation in nightly calling duration in field crickets, Gryllus integer and G. rubens (Orthoptera: Gryllidae). J. Insect Behav. 4, 185–194. doi: 10.1007/BF01054611
Cade, W., and Wyatt, D. R. (1984). Factors affecting calling behaviour in field crickets, Teleogryllus and Gryllus (age, weight, density, and parasites). Behaviour 88, 61–75. doi: 10.1163/156853984X00489
Caldwell, M. S., and Bee, M. A. (2014). Spatial hearing in Cope's gray treefrog: I. Open and closed loop experiments on sound localization in the presence and absence of noise. J. Comp. Physiol. A Neuroethol. Sens. Neural. Behav. Physiol. 200, 265–284. doi: 10.1007/s00359-014-0882-6
Chang, C., Cheng, Y.-C., and Lin, S.-M. (2018). Influence of conspecific and heterospecific cues on phonotaxis behavior in a polyandrous treefrog. Behav. Ecol. Sociobiol. 72:179. doi: 10.1007/s00265-018-2593-4
Davies, N. B. (1977). Prey selection and social behaviour in wagtails (Aves: Motacillidae). J. Anim. Ecol. 46, 37–57. doi: 10.2307/3945
Dehn, M. M. (1990). Vigilance for predators: detection and dilution effects. Behav. Ecol. Sociobiol. 26, 337–342. doi: 10.1007/BF00171099
Diego-Rasilla, F. J., and Luengo, R. M. (2004). Heterospecific call recognition and phonotaxis in the orientation behavior of the marbled newt, Triturus marmoratus. Behav. Ecol. Sociobiol. 55, 556–560. doi: 10.1007/s00265-003-0740-y
Downes, J. A. (1969). The swarming and mating flight of diptera. Ann. Rev. Entomol. 14, 271–298. doi: 10.1146/annurev.en.14.010169.001415
Endler, J. A. (1980). Natural selection on color patterns in Poecilia reticulata. Evolution 34, 76–91. doi: 10.1111/j.1558-5646.1980.tb04790.x
Endler, J. A. (1983). Natural and sexual selection on color patterns in poeciliid fishes. Exp. Biol. Fishes 9, 173–190. doi: 10.1007/BF00690861
Endler, J. A. (1987). Predation, light intensity and courtship behaviour in Poecilia reticulata (Pisces: Poeciliidae). Anim. Behav. 35, 1376–1385. doi: 10.1016/S0003-3472(87)80010-6
Endler, J. A. (1995a). Multiple trait coevolution and environmental gradients in guppies. Trends Ecol. Evol. 10, 22–29. doi: 10.1016/S0169-5347(00)88956-9
Endler, J. A. (1995b). Multiple-trait coevolution and environmental gradients in guppies. Trends Ecol. Evol. 10, 22–29.
Gerhardt, H. C. (1994). The evolution of vocalizations in frogs and toads. Ann. Rev. Ecol. Evol. Systemat. 25, 293–324. doi: 10.1146/annurev.ecolsys.25.1.293
Gibson, R. M., Aspbury, A. S., and McDaniel, L. L. (2002). Active formation of mixed-species grouse leks: a role for predation in lek evolution? Proc. Biol. Sci. 269, 2503–2507. doi: 10.1098/rspb.2002.2187
Given, M. F. (1990). Spatial distribution and vocal interaction in Rana clamitans and R. virgatipes. J. Herpetol. 24, 377–382. doi: 10.2307/1565053
Goodale, E., Ruxton, G. D., and Beauchamp, G. (2019). Predator eavesdropping in a mixed-species environment: How prey species may use grouping, confusion, and the cocktail party effect to reduce predator detection. Front. Ecol. Evol. 7:141. doi: 10.3389/fevo.2019.00141
Grand, T. C., and Dill, L. M. (1999). Predation risk, unequal competitors and the ideal free distribution. Evol. Ecol. Res. 1, 389–409.
Halfwerk, W., Blaas, M., Kramer, L., Hijner, N., Trillo, P. A., Bernal, X. E., et al. (2018). Adaptive changes in sexual signalling in response to urbanization. Nat. Ecol. Evol. 3, 374–380. doi: 10.1038/s41559-018-0751-8
Hamilton, W. D. (1971). Geometry for the selfish herd. J. Theoret. Biol. 31, 295–311. doi: 10.1016/0022-5193(71)90189-5
Haynes, K. F., and Yeargan, K. V. (1999). Exploitation of intraspecific communication systems: illicit signalers and receivers. Ann. Entomol. Soc. Am. 92, 960–967. doi: 10.1093/aesa/92.6.960
Hendrichs, J., Katsoyannos, B. I., Wornoayporn, V., and Hendrichs, M. A. (1994). Odour-mediated foraging by yellowjacket wasps (Hymenoptera: Vespidae): predation on leks of pheromone-calling Mediterranean fruit fly males (Diptera: Tephritidae). Oecologia 99, 88–94. doi: 10.1007/BF00317087
Holt, R. D. (1977). Predation, apparent competition, and the structure of prey communities. Theor. Popul. Biol. 12, 197–229. doi: 10.1016/0040-5809(77)90042-9
Holt, R. D. (1984). Spatial heterogeneity, indirect interactions, and the coexistence of prey species. Am. Nat. 124, 377–406. doi: 10.1086/284280
Holt, R. D., and Bonsall, M. B. (2017). Apparent competition. Ann. Rev. Ecol. Evol. System. 48, 447–471. doi: 10.1146/annurev-ecolsys-110316-022628
Holt, R. D., and Kotler, B. P. (1987). Short-term apparent competition. Am. Nat. 130, 412–430. doi: 10.1086/284718
Hoskin, C. J., and Higgie, M. (2010). Speciation via species interactions: the divergence of mating traits within species. Ecol. Lett. 13, 409–420. doi: 10.1111/j.1461-0248.2010.01448.x
Jennions, M. D., and Backwell, P. R. Y. (1992). Chorus size influences anti-predator response of a Neotropical frog. Anim. Behav. 44, 990–992. doi: 10.1016/S0003-3472(05)80596-2
Kacelnik, A., and Krebs, J. R. (1983). The dawn chorus in the great tit (Parus major): proximate and ultimate causes. Behaviour 83, 287–308. doi: 10.1163/156853983X00200
Kotiaho, J. S. (2001). Costs of sexual traits: a mismatch between theoretical considerations and empirical evidence. Biol. Rev. 76, 365–376. doi: 10.1017/S1464793101005711
Landeau, L., and Terborgh, J. (1986). Oddity and the ‘confusion effect’ in predation. Anim. Behav. 34, 1372–1380. doi: 10.1016/S0003-3472(86)80208-1
Macedo, R. H., and DuVal, E. H. (2018). Friend or foe? The dynamics of social life. Anim. Behav. 143, 139–143. doi: 10.1016/j.anbehav.2018.06.022
Marler, P., and Slabbekoorn, H. (2004). Nature's Music: The Science of Birdsong. London: Elsevier Academic Press.
Morris, G. K. (1980). Calling display and mating behavior of Copiphora rhinocerus Pictet (Orthoptera: Tettigoniidae). Anim. Behav. 28, 42–51. doi: 10.1016/S0003-3472(80)80006-6
Morris, G. K., and Beier, M. (1982). Song structure and description of some Costa Rican katydids (Orthoptera: Tettigoniidae). Transact. Am. Entomol. Soc. 108, 287–314.
Morse, D. H. (1970). Ecological aspects of some mixed-species foraging flocks of birds. Ecol. Monograph. Ecol. Monograph. 40, 119–168. doi: 10.2307/1942443
Olson, V. A., and Owens, I. P. (1998). Costly sexual signals: are carotenoids rare, risky or required? Trends Ecol. Evol. 13, 510–514. doi: 10.1016/S0169-5347(98)01484-0
Page, R. A., and Jones, P. L. (2016). “Overcoming sensory uncertainty: factors affecting foraging decisions in frog-eating bats,” in Psychological Mechanisms in Animal Communication. eds M. A. Bee and C. T. Miller (Cham: Springer International Publishing, 285–312.
Page, R. A., Schnelle, T., Kalko, E. K., Bunge, T., and Bernal, X. E. (2012). Sequential assessment of prey through the use of multiple sensory cues by an eavesdropping bat. Naturwissenschaften 99, 505–509. doi: 10.1007/s00114-012-0920-6
Pavlov, D., and Kasumyan, A. (2000). Patterns and mechanisms of schooling behavior in fish: a review. J. Ichthyol. 40, S163–S231.
Pfennig, K. S. (2000). Female spadefoot toads compromise on mate quality to ensure conspecific matings. Behav. Ecol. 11, 220–227. doi: 10.1093/beheco/11.2.220
Phelps, S. M., Rand, A. S., and Ryan, M. J. (2006). The mixed-species chorus as public information: túngara frogs eavesdrop on a heterospecific. Behav. Ecol. 18, 108–114. doi: 10.1093/beheco/arl063
Pulliam, H. R. (1973). On the advantages of flocking. J. Theoret. Biol. 38, 419–422. doi: 10.1016/0022-5193(73)90184-7
Rhebergen, F., Taylor, R. C., Ryan, M. J., Page, R. A., and Halfwerk, W. (2015). Multimodal cues improve prey localization under complex environmental conditions. Proc. Biol. Sci. 282:20151403. doi: 10.1098/rspb.2015.1403
Römer, H. (2013). “Masking by noise in acoustic insects: problems and solutions,” in Animal Communication and Noise, ed H. Brumm (Berlin: Springer, 33–63.
Rowell, G. A., and Cade, W. H. (1993). Simulation of alternative male reproductive behavior: calling and satellite behavior in field crickets. Ecol. Model. 65, 265–280. doi: 10.1016/0304-3800(93)90083-5
Ruell, E. W., Handelsman, C. A., Hawkins, C. L., Sofaer, H. R., Ghalambor, C. K., and Angeloni, L. (2013). Fear, food and sexual ornamentation: plasticity of colour development in Trinidadian guppies. Proc. R. Soc. B 280:20122019. doi: 10.1098/rspb.2012.2019
Ryan, M. J., Tuttle, M. D., and Rand, A. S. (1982). Bat predation and sexual advertisement in a neotropical anuran. Am. Nat. 119, 136–139. doi: 10.1086/283899
Ryan, M. J., Tuttle, M. D., and Taft, L. K. (1981). The costs and benefits of frog chorusing behavior. Behav. Ecol. Sociobiol. 8, 273–278. doi: 10.1007/BF00299526
Schmidt, A. K. D., Römer, H., and Riede, K. (2013). Spectral niche segregation and community organization in a tropical cricket assemblage. Behav. Ecol. 24, 470–480. doi: 10.1093/beheco/ars187
Schmidt, K. A., Dall, S. R. X., and van Gils, J. A. (2010). The ecology of information: an overview on the ecological significance of making informed decisions. Oikos 119, 304–316. doi: 10.1111/j.1600-0706.2009.17573.x
Schwartz, J. J., and Wells, K. D. (1983). An experimental study of acoustic interference between two species of neotropical treefrogs. Anim. Behav. 31, 181–190. doi: 10.1016/S0003-3472(83)80187-0
Schwartz, J. J., and Wells, K. D. (1984). Interspecific acoustic interactions of the neotropical treefrog Hyla ebraccata. Behav. Ecol. Sociobiol. 14, 211–224. doi: 10.1007/BF00299621
Segami, J. C., Rudh, A., Rogell, B., Odeen, A., Lovlie, H., Rosher, C., et al. (2016). Cryptic female Strawberry poison frogs experience elevated predation risk when associating with an aposematic partner. Ecol. Evol. 7, 744–750. doi: 10.1002/ece3.2662
Shelly, T. E. (2018). Sexual selection on leks: A fruit fly primer. J. Insect Sci. 18, 1–16. doi: 10.1093/jisesa/iey048
Siemers, B. M., Kriner, E., Kaipf, I., Simon, M., and Greif, S. (2012). Bats eavesdrop on the sound of copulating flies. Curr. Biol. 22, R563–R564. doi: 10.1016/j.cub.2012.06.030
Simmons, J. A., Wever, E. G., and Pylka, J. M. (1971). Periodical cicada: sound production and hearing. Science 171, 212–213. doi: 10.1126/science.171.3967.212
Slagsvold, T., Dale, S., and Kruszewicz, A. (1995). Predation favours cryptic coloration in breeding male pied flycatchers. Anim. Behav. 50, 1109–1121. doi: 10.1016/0003-3472(95)80110-3
Soper, R. S., Delyzer, A. J., and Smith, L. F. (1976). The genus Massospora entomopathogenic for cicadas. Part. II. Biology of Massospora levispora and its host Okanagana rimosa, with notes on Massospora cicadina on the periodical cicadas. Ann. Entomol. Soc. Am. 69, 89–95. doi: 10.1093/aesa/69.1.89
Sridhar, H., Beauchamp, G., and Shanker, K. (2009). Why do birds participate in mixed-species foraging flocks? A large-scale synthesis. Anim. Behav. 78, 337–347. doi: 10.1016/j.anbehav.2009.05.008
Steinberg, D. S., Losos, J. B., Schoener, T. W., Spiller, D. A., Kolbe, J. J., and Leal, M. (2014). Predation-associated modulation of movement-based signals by a Bahamian lizard. Proc. Natl. Acad. Sci. U. S. A. 111, 9187–9192. doi: 10.1073/pnas.1407190111
Stensland, E. V. A., Angerbjörn, A., and Berggren, P. E. R. (2003). Mixed species groups in mammals. Mammal Rev. 33, 205–223. doi: 10.1046/j.1365-2907.2003.00022.x
Stoddard, P. K. (1999). Predation enhances complexity in the evolution of electric fish signals. Nature 400, 254–256. doi: 10.1038/22301
Sueur, J. (2002). Cicada acoustic communication: potential sound partitioning in a multispecies community from Mexico (Hemiptera: Cicadomorpha: Cicadidae). Biol. J. Linnean Soc. 75, 379–394. doi: 10.1111/j.1095-8312.2002.tb02079.x
Tobias, J. A., Planqué, R., Cram, D. L., and Seddon, N. (2014). Species interactions and the structure of complex communication networks. Proc. Natl. Acad. Sci. U. S. A. 111, 1020–1025. doi: 10.1073/pnas.1314337111
Trillo, P. A., Athanas, K. A., Goldhill, D. H., Hoke, K. L., and Funk, W. C. (2013). The influence of geographic heterogeneity in predation pressure on sexual signal divergence in an Amazonian frog species complex. J. Evol. Biol. 26, 216–222. doi: 10.1111/jeb.12041
Trillo, P. A., Bernal, X. E., Caldwell, M. S., Halfwerk, W., Wessel, M. O., and Page, R. A. (2016). Collateral damage or a shadow of safety? The effects of signalling heterospecific neighbours on the risks of parasitism and predation. Proc. R. Soc. 283:20160343. doi: 10.1098/rspb.2016.0343
Tuttle, M. D., and Ryan, M. J. (1981). Bat predation and the evolution of frog vocalizations in the neotropics. Science 214, 677–678. doi: 10.1126/science.214.4521.677
Tuttle, M. D., Taft, L. K., and Ryan, M. J. (1982). Evasive behavior of a frog in response to bat predation. Anim. Behav. 30, 393–397. doi: 10.1016/S0003-3472(82)80050-X
Van Schaik, C. P., Noordwijk, M. A., Warsono, B., and Sutriono, E. (1983). Party size and early detection of predators in Sumatran forest primates. Primates 24, 211–221. doi: 10.1007/BF02381083
Velez, M. J., and Brockmann, H. J. (2006). Seasonal variation in selection on male calling song in the field cricket, Gryllus rubens. Anim. Behav. 72, 439–448. doi: 10.1016/j.anbehav.2006.02.002
Verrel, P. A. (1991). Illegitimate exploitation of sexual signalling systems and the origin of species. Ethol. Ecol. Evol.3, 273–283. doi: 10.1080/08927014.1991.9525356
Wells, K. D. (2010). The Ecology and Behavior of Amphibians. Chicago, IL: University of Chicago Press.
Williams, K. S., Smith, K. G., and Stephen, F. M. (1993). Emergence of 13-yr periodical cicadas (Cicadidae: Magicicada): Phenology, mortality, and predators satiation. Ecology 74, 1143–1152. doi: 10.2307/1940484
Wrona, F. J., and Dixon, R. J. (1991). Group size and predation risk: a field analysis of encounter and dilution effects. Am. Nat. 137, 186–201. doi: 10.1086/285153
Zuk, M., and Kolluru, G. R. (1998). Exploitation of sexual signals by predators and parasitoids. Q. Rev. Biol. 73, 415–438. doi: 10.1086/420412
Keywords: mixed-species aggregations, eavesdroppers, mating signals, collateral damage, heterospecific neighbors, predation, parasitism
Citation: Trillo PA, Benson CS, Caldwell MS, Lam TL, Pickering OH and Logue DM (2019) The Influence of Signaling Conspecific and Heterospecific Neighbors on Eavesdropper Pressure. Front. Ecol. Evol. 7:292. doi: 10.3389/fevo.2019.00292
Received: 24 February 2019; Accepted: 22 July 2019;
Published: 07 August 2019.
Edited by:
Martin Stevens, University of Exeter, United KingdomReviewed by:
Ken Schmidt, Texas Tech University, United StatesCopyright © 2019 Trillo, Benson, Caldwell, Lam, Pickering and Logue. This is an open-access article distributed under the terms of the Creative Commons Attribution License (CC BY). The use, distribution or reproduction in other forums is permitted, provided the original author(s) and the copyright owner(s) are credited and that the original publication in this journal is cited, in accordance with accepted academic practice. No use, distribution or reproduction is permitted which does not comply with these terms.
*Correspondence: Paula A. Trillo, cHRyaWxsb0BnZXR0eXNidXJnLmVkdQ==
Disclaimer: All claims expressed in this article are solely those of the authors and do not necessarily represent those of their affiliated organizations, or those of the publisher, the editors and the reviewers. Any product that may be evaluated in this article or claim that may be made by its manufacturer is not guaranteed or endorsed by the publisher.
Research integrity at Frontiers
Learn more about the work of our research integrity team to safeguard the quality of each article we publish.