- 1Laboratory of Tree-Ring Research and School of Natural Resources and the Environment, University of Arizona, Tucson, AZ, United States
- 2Division of Atmospheric Sciences, Desert Research Institute, Reno, NV, United States
- 3School of Forestry, Northern Arizona University, Flagstaff, AZ, United States
Rapid climate change and altered disturbance regimes represent increasing stressors to the stability of existing ecosystems. Resilience is a widely used framework for post-disturbance response, but resilient responses are emergent properties resulting from component processes of persistence, recovery, and reorganization, with different mechanisms at work in each mode. We present a model of scaled resilience, which allows resilience to be decomposed across scales of space, time, and levels of biological organization. Using case examples of post-fire resilience in dry conifer forests of interior western North America, we illustrate the increased clarity gained by separating scale-dependent mechanisms of persistence, recovery, and reorganization. We conclude by describing how the scaled resilience framework can be applied in land and fire management by distinguishing relevant management actions before, during, and after wildfire.
Introduction
Traditionally, the objectives of land management, conservation biology, and restoration ecology have focused on maintaining or re-establishing historical conditions (Egan and Howell, 2005; Palmer et al., 2016). The underlying premise of these approaches is that species, communities, and ecosystems will be best prepared to cope with new or variable conditions if their environment is within the historical range of variability to which they are adapted.
In today's world, many of these assumptions about how ecosystems will respond to emerging conditions are being tested and challenged. Concentrations of atmospheric CO2 now fall outside of the documented range for the past 800,000 years, setting the stage for multiple centuries of altered climate (IPCC, 2014, 2018). As a consequence, in many areas, key climate variables such as annual and seasonal precipitation, temperature, and moisture stress are moving rapidly outside the known historical range of variability (USGCRP, 2017). Seasonal patterns of precipitation, snowpack soil water content and persistence, seasonal timing of streamflow, and humidity are changing in ways that are demonstrably unprecedented for thousands of years. In many regions of the world, widespread drought-related stress driven by rapidly rising seasonal temperatures is already causing elevated tree mortality, indicating the potential for substantial contraction of forested area, or replacement of current forested communities with species better adapted to persistent drought conditions (van Mantgem et al., 2009, 2018; Williams et al., 2013).
Other impacts in addition to the direct effects of altered climate are having equally profound consequences for ecosystem structure and function. Biogeochemical and hydrologic cycles are increasingly outside the ecologically relevant range of variability. Partially as a consequence, invasive non-native grasses have altered desert and shrubland communities throughout western North America, through competition with native species and facilitation of altered fire regimes (Chambers et al., 2013). These mechanisms tend to reinforce the success of the invader, making autogenic return of the community to its pre-invasion state unlikely without substantial human intervention (Stevens and Falk, 2009; Chambers et al., 2013).
These relatively slow (decadal to centennial) processes can be accelerated and multiplied substantially by severe disturbance. Ecological disturbances such as fires and insect outbreaks—all naturally occurring processes—may stress ecosystems beyond their ability to recover if they become elevated in severity, frequency, or extent. Following severe large-scale disturbances, in a context of altered climate, ecosystems may follow altered recovery trajectories in which resilient capacity is tested to its limits, or exceeded (Allen, 2007; Falk, 2013). Such “no-return” outcomes are evident in post-fire recovery pathways following large high-severity fire impacts in recent years (Figure 1). Chronic climate stress may impair the ability of individuals, populations, and communities to survive and recover from disturbance, in effect increasing its expressed severity and impact.
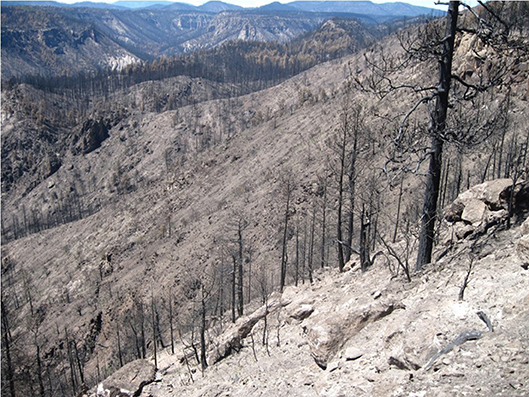
Figure 1. Profoundly altered landscapes affected by large and severe fires may take decades or centuries to return to pre-disturbance conditions, or may shift into alternative metastable states as changing climate inhibits return to the re-disturbance community. Cochiti Canyon, Jemez Mountains, New Mexico USA following the 2011 Las Conchas Fire.
Against this background, resilience—defined broadly, the ability of a system to recover following stress or disturbance—is a key emergent property of individuals, populations, and communities. While the expression of resilience to time-varying environments and episodic disturbance have always been important, this property is gaining significance in today's rapidly changing world as a key pathway to adaptive capacity, as the biosphere and its components are increasingly exposed to a world exceeding the millennial historical range of variation. Resilience in this context implies that some degree of ecosystem change may reflect processes of adaptation to altered environmental conditions; change is not necessarily an indicator of ecosystem failure.
We argue here that for models of resilience to be useful, both scientifically and to managers, the dimensionalities of both disturbance and post-disturbance response need to be incorporated into the framework. We outline a model of scaled resilience that takes these dimensions into account explicitly, allowing ecologists and managers to apply appropriate spatial, temporal, and biological scaling to their assessments of ecosystem resilience (Petraitis and Latham, 1999). Although the concept of scaled resilience is general, we illustrate the use of the framework with reference to the ecology of wildland fire in forests of interior western North America, with a focus on plant populations and communities.
Concepts of Resilience in the Ecological Literature
Lewontin (1969), Holling (1973), Sutherland (Sutherland, 1974, 1990), and May (1977) were among the first ecologists to describe the mathematics of non-linear ecological responses to perturbations, leading to a theoretical framework that demonstrated how ecosystems display resistance, thresholds and multiple alternative stable states. These models defined resilience space implicitly as the domain of response prior to an irreversible threshold change. Over time, concepts of ecological resilience have become prominent in the scientific and management literature as more ecosystems are pushed to or beyond their limits of recovery by multiple interacting stressors characterized by both positive and negative feedback relationships and cascading effects (Beisner et al., 2003; Andersen et al., 2009;Rocha et al., 2018).
Resilience is not a unitary response to disturbance. Rather, the response of ecological systems following a perturbation can be summarized in sequential stages operating at differing levels of biological organization across scales of space and time Figure 2) (Millar et al., 2007; Millar and Stephenson, 2015; Falk, 2017). The more general emergent response of resilience is comprised of component processes of resistance, recovery, and reorganization. The first stage, resistance, is the ability of individuals or structures to tolerate or persist through disturbance, allowing the system to return to its pre-disturbance state relatively unchanged (Halpern, 1988; Hershkovitz and Gasith, 2013; Lake, 2013). This property, also referred to as persistence (Sutherland, 1990; Carpenter et al., 1992), is characterized by high demographic survivorship (per capita survival over a time increment) or, conversely, low mortality rate. In physical terms, persistence generally favors demographic and environmental stability, because processes of growth and reproduction continue, and essential resources (e.g., intact soil profiles) are retained. Grassland fire regimes of perennial grasses exemplify ecological responses based on survivorship: the cured foliage burns in a surface fire, but the meristem is positioned near ground level where most individuals survive and refoliate the following season.
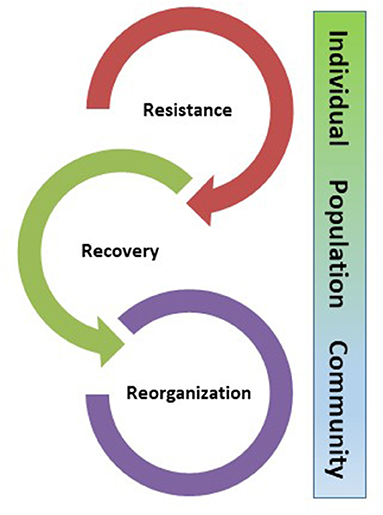
Figure 2. Processes of resistance, recovery, and reorganization are sequential components of the aggregate property of resilience, operating at progressively higher levels of biological organization.
When mechanisms of resistance (persistence) have been overcome, resilience shifts from individuals to population-level processes of recovery, which in an ecological context is the re-establishment of the pre-disturbance population following mortality of the original individuals, through recruitment or colonization. Recover processes can be relatively rapid, as in the case of populations of pines with serotinous cones (e.g., Lodgepole pine, Pinus contorta ssp. contorta), which depend on intense fires to open their cones and allow seed dispersal onto cleared ground, usually leading to abundant post-fire establishment within 1–2 years of fire. Other populations may recover more slowly following adult mortality, depending on the spatial and temporal patterns of seed production and dispersal, seed germination and establishment, and post-establishment competition. In many forested ecosystems, the initial phase of recovery is dominated by suites of regionally adapted early-successional species; in western North America, these include aspen (Populus tremuloides), Gambel oak (Quercus gambellii), New Mexico locust (Robinia neomexicana), Ceanothus spp., and others. These processes can extend over years and decades, illustrating the widely varying temporal dimension to the recovery phase.
Ecological systems reflect substantial inertia, or resistance to change, expressed as physical and informational legacies (Johnstone et al., 2016). However, ecosystems are not homeostatic over broader scales of space and time; community reorganization is a fundamental property in the paleoecological literature (Falk and Millar, 2016). As the magnitude of disturbance and interacting chronic stressors increase, both persistence and recovery processes can fail, and the system re-organizes into an alternative state (Beisner et al., 2003). These alternative states may be ephemeral, or disturbance and biotic interactions may cause the new state to be self-reinforcing, and the system may settle into a new alternative metastable state. Notably, the system is now resilient in every sense of the term, but in a new configuration (Hobbs and Norton, 1996; Cortina et al., 2006). Biome or vegetation type conversions are characteristic outcomes of reorganization. An ecological example of an alternative state is Great Basin sagebrush steppe that has been invaded heavily by cheatgrass (Bromus tectorum) and other invasive non-native grasses. These species alter not only competitive relationships and nutrient pools but also the fire regime, so that the system becomes resilient in its new state, and resists return to the original vegetation (Rowland et al., 2010; Suding et al., 2016). Similar persistent transitions are observed in Sonoran Desert uplands invaded by buffelgrass (Cenchrus ciliaris), where a formerly sparse understory comprised of shrubs, cacti, and herbaceous winter annuals converts to an annual grassland with increased fuel loads and continuity, creating an invasive fire regime that is lethal to many long-lived desert species with little adaptation to fire (Stevens and Falk, 2009).
A Model of Scaled Resilience
Current conceptual models of resilience and restoration provide a useful set of emergent variables, but as typically interpreted, they overlook scaling dimensionalities that are critical for understanding how ecosystems respond to major perturbations, especially in rapidly changing environments. Although scale is recognized widely as a fundamental property in ecological theory (Levin, 1992; Brown and West, 2000; Turner, 2010), models of resilience have for the most part not addressed critical dimensionalities. For example, in the threshold response to disturbance, the resilience response may be dependent on the extent of the overall disturbance in comparison to characteristic events, and its landscape spatial structure (e.g., whether the spatial mosaic of patches of differing burn severity comprised of many small patches, or whole watersheds). Temporal considerations are equally important: How long ago did the disturbance occur, and did the footprint of disturbance intersect or overlap with previous disturbances? Did all disturbance effects occur immediately, or were there significant lagging effects (delayed mortality, indirect or second order effects such as post-fire floods)? What biological entities were affected initially: individuals within a population, populations, entire communities? What was the pre- and post-disturbance climate, and how did this influence disturbance susceptibility and post-disturbance recovery processes? These scale-dependent processes influence ecological resilience as an emergent property of multiple mechanisms that govern the response to disturbance, operating along axes of space, time, and biological hierarchy (Figure 3).
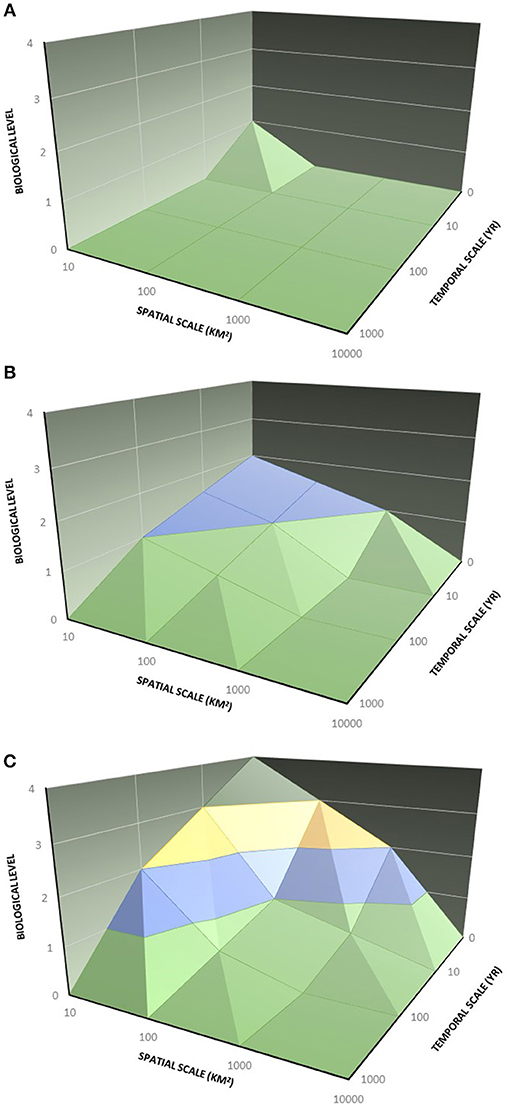
Figure 3. A graphical model of scaled resilience. Disturbances, and the ecological responses that follow, are scaled on primary axes: spatial scale of disturbance (x), recovery time (y), and level of biological organization in flux (z). Localized, low-severity disturbances causing minimal mortality are associated primarily with mechanisms of individual persistence (green volume A). As disturbances become larger and more severe, population-level recovery processes dominate, requiring longer temporal extent (blue region B). Very large and severe disturbances can trigger community-level reorganization and alternative states (yellow region, C).
We use forest fire regimes to illustrate the importance of scale in resilience ecology. From the perspective of ecological resilience theory, the spatial and temporal patterns of occurrence of fire—the fire regime—constitute one of the fundamental properties of the evolutionary environment of fire-adapted ecosystems. Like resilience itself, fire regimes can be defined along axes of space, time, and magnitude (Sugihara et al., 2006). Temporal attributes include mean and variance in fire seasonality, frequency, and event duration. Spatial attributes include the characteristic size, spatial complexity, and landscape severity distribution. Magnitude attributes include physical measures (fire intensity, behavior), severity, and fuel strata involved. Understanding how these fire regime attributes function in different vegetation types is critical for understanding the role of fire in a given system, and what phase of resilience will occur in any particular context. Fire events that fall within the normal range of variability along each of these axes are considered characteristic in that they constitute the evolutionary environment for the constituent species and communities of the system. From this perspective, the alteration of the natural fire regime, rather than fire itself, may be thought of as the disruption to the dynamics of the ecosystem (Falk, 2006).
Spatial Scale of Disturbance and Resilience
Disturbances vary widely in their spatial properties. Many disturbances are small in extent: Lightning can strike a single tree and burn a few square meters before being extinguished (Figure 4); defoliating insects can attack a small group of trees; slope failure may destabilize part of a hillslope; herds of bison can overturn soil in ha-sized patches over large landscapes. At the other extreme, large landscape fires throughout western North America now commonly burn hundreds of thousands of hectares, and total area burned per year has increased over the last two decades to more than 7 million ha yr−1 1. Insect outbreaks cover even more area; outbreaks of mountain pine beetle (Dendroctonus ponderosae Hopkins) in conifer forests of British Columbia have cumulatively affected in excess of 146 ha (Axelson et al., 2009).
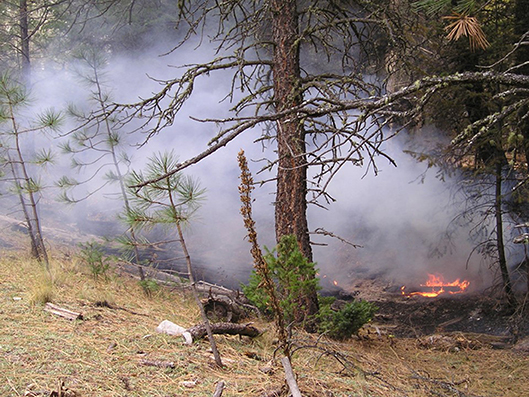
Figure 4. Small-scale fire disturbance in a New Mexico ponderosa pine community. Resistance processes among fire-adapted species dominate, resulting in low mortality rates among overstory trees.
In addition to overall extent, spatial scale of disturbance also includes heterogeneity in patch size and diversity. For example, the wildland fire burn severity mosaic (or spatial complexity) is a fundamental landscape property of wildland fires (Haire and McGarigal, 2010). Historically, evidence suggests that, in dry and mixed forests, these mosaics were often fine-grained, consisting of hundreds of smaller (e.g., < 1–100 ha) patches. Margolis et al. (2011) found that historical mean high severity patch sizes in higher elevations (>2600 m) in southwestern US mountains ranged from 286 ha to 521 ha in mixed conifer-aspen and spruce-fir forests, respectively. In southern Arizona ponderosa pine forests at elevations ranging from 2,450 to 2,750 m, high severity patches ranged historically from 0.1 ha to 100 ha (Yocom-Kent et al., 2015); high severity patch sizes in mixed conifer forests were as large as 100 ha, although these were qualified to occur under specific climate conditions and in dissected landscapes, as patch sizes in this forest type are generally inferred to have been small (Iniguez et al., 2009). In Oregon, larger patches of high severity fire were related to drought and annual fire extent, depending on the vegetation type (Reilly et al., 2017). Overall, they found that high severity patches were > 100 ha in vegetation types with historically high severity fire regimes, whereas vegetation with historically low and mixed severity fire regimes had almost half of the patches of high severity greater than or equal to 100 ha. Heyerdahl et al. (2014, 2019) found evidence of predominantly fine-grain spatial mosaics in multi-century studies in lodgepole-ponderosa pine and dry mixed conifer forests in eastern and central Oregon. In the northern Washington Cascade Range, Cansler and McKenzie (2014) found a positive relationship between fire size and amount of burn severity, high severity patch size and the spatial pattern of severity.
Large contemporary wildfires have departed significantly from these historical patterns (Figure 5). Especially since the onset ~ 2000 C.E. of persistent widespread drought conditions in much of North America, many wildfires are generating coarse-grained mosaics consisting of a smaller number of uncharacteristically large contiguous high-severity patches, some of which are tens of thousands of hectares in extent (Figure 6). Of particular importance to post-fire resilience is evidence of changes in spatial properties of the landscape burn severity mosaic, such as increasing aggregation of high-severity patches, as well as larger overall burned area and proportion of high-severity burn in some forest types (Cansler and McKenzie, 2014; Singleton et al., 2019).
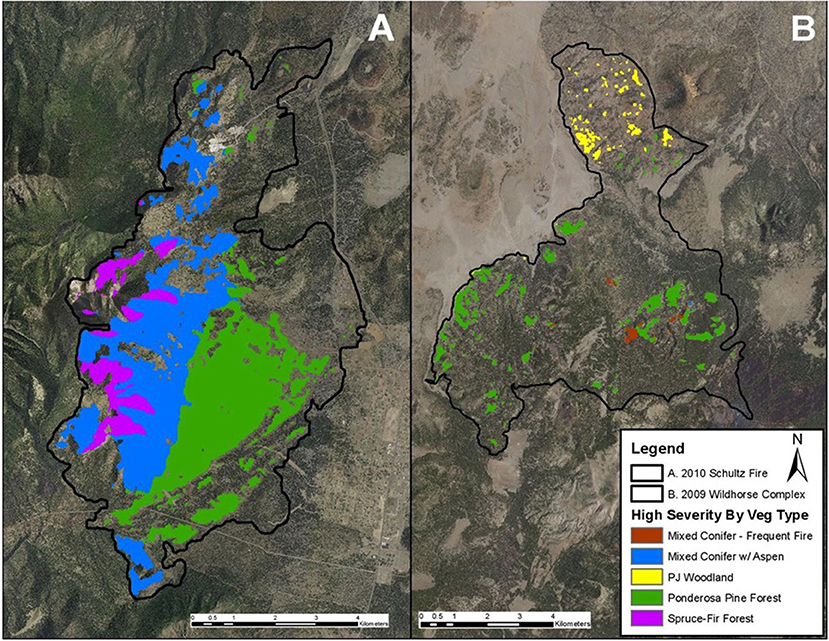
Figure 5. Patch size contrast in two large landscape wildfires in Arizona, USA with contrasting patch structure. A large proportion of the 2010 Schultz Fire (A) burned with high severity effects (high rates of tree mortality and soil damage) in large contiguous patches. This outcome contrasts with the fine-grain severity mosaic from the 2009 Wildhorse Complex (B), with a larger number of smaller, dispersed patches. Scaled resilience theory predicts more rapid recovery from the Wildhorse Complex compared to the Schultz Fire based on spatially limited processes such as seed dispersal.
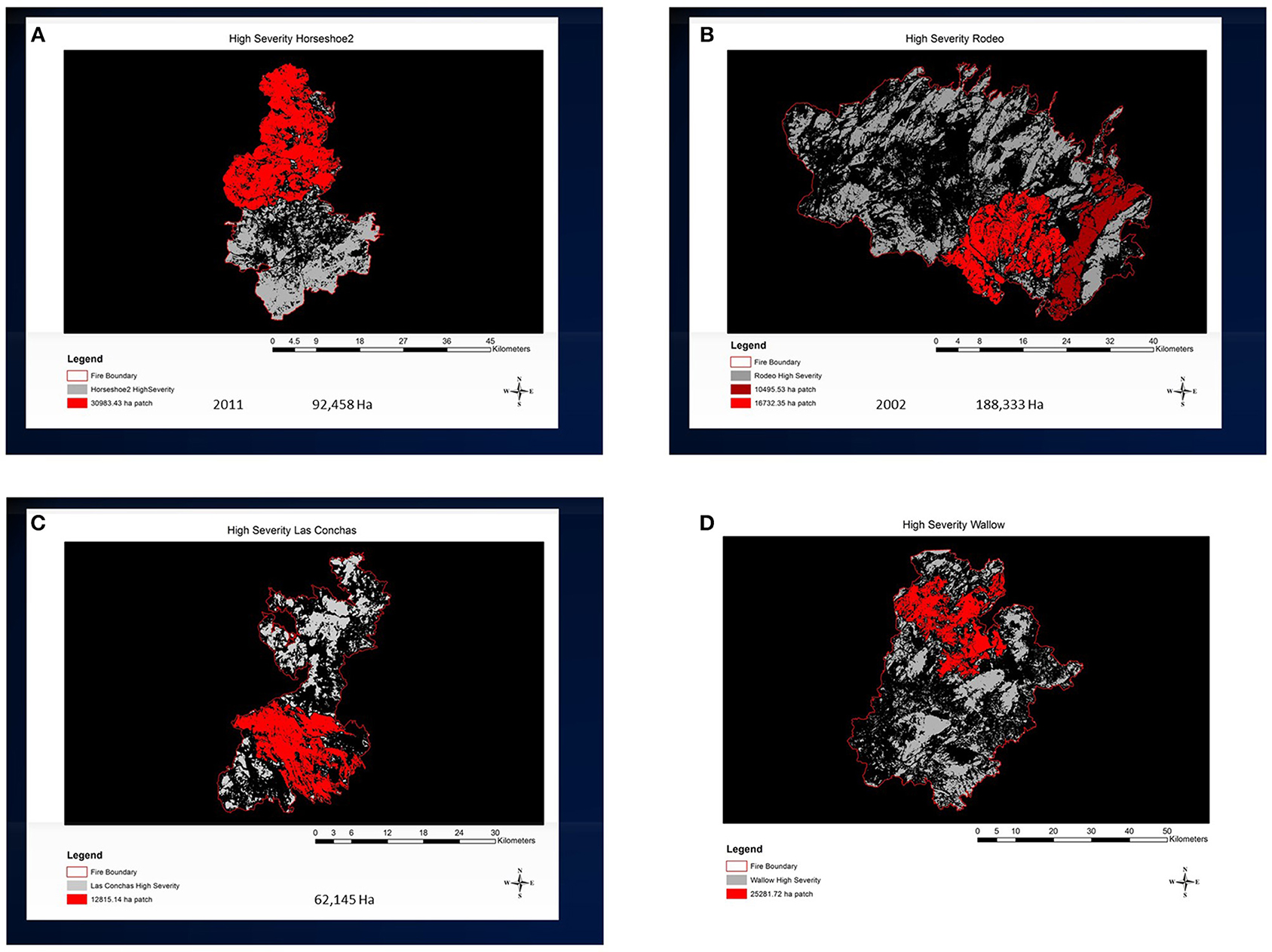
Figure 6. Large high-severity patches in recent fires in the southwestern USA determined from satellite imagery (www.mtbs.gov) for four recent fires. Red areas indicate the largest contiguous high-severity polygons compiled by connecting high severity pixels using a four-neighbor rule for the (A) 2011 Horseshoe 2 Fire, AZ (30,983 ha); (B) 2002 Rodeo-Chediski Fire, AZ (16,732 ha); (C) 2011 Las Conchas Fire, NM (12,815 ha); (D) 2011 Wallow Fire, AZ and NM (25,282 ha). High-severity delineated by RdNBR according to Singleton et al. (2019).
The spatial scale of disturbance has profound consequences for numerous ecological processes, including regeneration, soil and erosion, hydrologic processes and wildlife. In many cases, these spatial effects can determine the form of the resilience response. For example, large high-severity forest patches with few or no surviving trees may completely lack seed sources for post-fire tree recolonization; in these cases, seeds must be dispersed into the patch (Owen et al., 2017). Dispersal distances vary widely, from a few meters (in the case of some gravity-dispersed seeds) to tens of kilometers or more for some bird-dispersed species (Bonnet et al., 2005; Haire and McGarigal, 2010). Occasional long-distance dispersal mediated by mammals or birds can cover substantially more distance (Keane et al., 2012), and long-tailed dispersal kernels are an important mechanism of rapid dispersion (Clark, 1998), but successful seedling establishment is far from certain in any given year even when seeds are dispersed toward the patch interior, especially given the effect of climate variation on regeneration success (Law et al., 2019).
Higher severity fire can result in substantial physical and chemical changes to soil aggregation, porosity, water retention and hydrophobicity (DeBano, 2000); when these effects are extended over larger contiguous patches, there can be significant effects on soil erosion and hydrologic functioning due to positive feedbacks in runoff velocity and sediment load, leading to decreases in ecosystem sustainability and recovery potential (Neary et al., 1999). The burn severity mosaic and total fire size can create a range of vegetation and habitat mosaics from large homogenous patches of vegetation and habitat to more fine-grained complex vegetation mosaics with multiple plant species and habitat elements (Shaffer and Laudenslayer, 2006). These burn severity patches and mosaics variably affect wildlife species in contrasting ways (Dudley et al., 2012; Buchalski et al., 2013; Rockweit et al., 2017), through changing temporal and spatial attributes of habitat including patch size, juxtaposition and connectivity. These effects underscore the powerful influence of the spatial properties of a disturbance, particularly its overall extent and the pattern of patches, on the post-fire progression of an ecosystem. Ecosystem resilience cannot be understood fully without reference to the spatial scale of disturbance and recovery processes.
Temporal Scale of Disturbance and Resilience
How quickly a population or community or ecosystem recovers following disturbance is one of the most significant properties of resilience. We are more likely to judge a system that recovers rapidly to its pre-disturbance state as “resilient,” whereas one that recovers more slowly—according to our criteria—may be considered less resilient. However, these judgments are not always ecologically justified; ecological succession does not necessarily proceed at a pace to which humans can relate (i.e., decades to centuries). The pace and trajectory of ecosystem response to a disturbance is determined by the biology of its major constituents, geochemical and other environmental factors, and characteristics of the disturbance itself such as spatial scale.
The temporal properties of individual disturbance events include duration above critical thresholds. For wildland fires, a common metric of disturbance magnitude is fire severity, which can apply to both aboveground vegetation and soils. Fire effects on biota and soils are a function of reaction intensity (energetic heat output, kJ m−2 min−1) and fireline intensity (kJ m−1 sec−1), expressed over residence time, represented in the time-temperature function. The period during which a plant or area of soil experiences heat from a fire can determine whether plant roots experience lethal heating, for example, or the degree to which soil characteristics such as hydrophobicity, loss of organic matter, or sterilization occur (Neary et al., 2005). Both plants and animals can tolerate brief exposure to high temperatures that become lethal with extended duration (Engstrom, 2010).
The temporal aspects of a fire regime include the characteristic interval between events, including the central tendency and higher moments of the interval distribution. The historical range of variation in fire return intervals represents the evolutionary environment of the species that comprise that system, to which they are adapted through life history and anatomical features (Keeley et al., 2011; Keeley, 2012). The individual fire return interval—the time since a fire at a specific site—influences fuel properties (mass per area, horizontal and vertical continuity, size distribution), which in turn have important influences on the behavior and effects of the subsequent fire. This is illustrated clearly in the exclusion of surface fires throughout many western US forests during the majority of the twentieth century, driven by land use and not synchronous with climate variation (O'Connor et al., 2011; Fulé et al., 2012; Figure 7). This temporal departure in fire intervals is widely understood to have led to ahistorical fuel loads and continuity that are a key contributor to the era of megafires today (Parks et al., 2018). Thus, consistency (or departure) from the characteristic return interval distribution is a key temporal parameter regulating the form of post-fire responses.
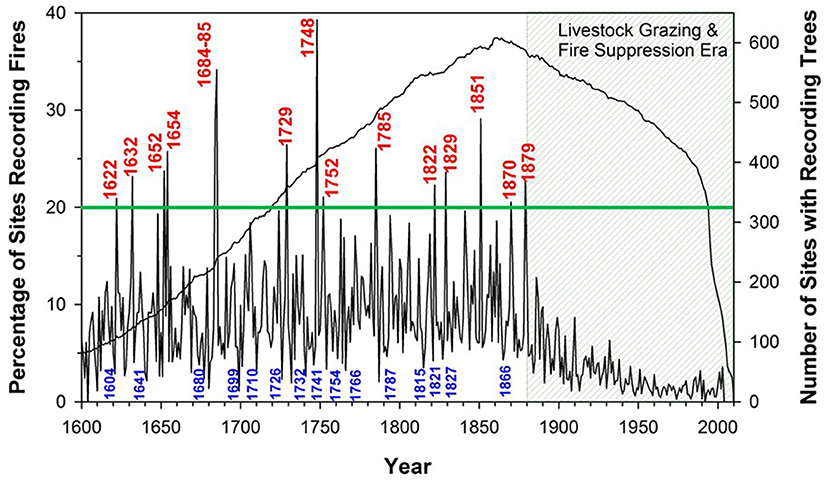
Figure 7. Regional fire records in the western USA, southwestern Canada, and northern Mexico 1600-2000 CE from a network of fire-scar based tree-ring sites. The dark blue line indicates the number of study sites active by year (right axis); black line shows the percentage of these sites recording fire each year (left axis). Years in which fire occurred in more than 20% of recording sites (horizontal green line) are identified in red; the smallest fire years are identified in blue. The abrupt termination of the fire regime in the early 1900s was due to effects of massively increased livestock grazing and subsequent fire suppression, not a change in climate. From Swetnam et al. (2016), used with permission.
Like changes in fire severity, or changes to its timing, the exclusion of fire for long periods in ecological systems adapted to its periodic occurrence can cause dramatic shifts in vegetation. In some fire-adapted systems, competitor species sensitive to fire are held in check by recurrent fire. In its absence, these species may out-compete the existing species or increase in dominance, leading to a shift in dominant plant functional types (O'Connor et al., 2014).
Another disruption in temporal properties of the fire regime include changes in the season of fire occurrence. Such changes have been observed in recent decades, as ignitions shift from periods of high lightning activity to late in the dry season, due to enhanced human-caused ignitions. Throughout western North America, fire seasons (meaning the sub-annual period during which meteorological conditions of temperature, precipitation, relative humidity, winds, and atmospheric stability are favorable for fire spread) are changing in response to climate forcing (Dale et al., 2001). Fire seasons have lengthened by 2–10 weeks in many areas, effectively allowing fires to start earlier and burn longer (Jolly et al., 2015; Kitzberger et al., 2017). These temporal changes can result in cascading ecological effects through asynchronous phenological effects on plants and animals.
Once disturbance has occurred, the time scale of recovery is a central response variable indicating the rate at which biophysical processes progress. For example, the post-fire growth response of aspen (Populus tremuloides) will be almost instantaneous, with sprouting stems appearing aboveground within weeks of fire exposure. In this instance (and for many other fire-adapted species) clonal resprouting is a dominant mode of post-fire reproduction, with the result that a post-fire community is re-established rapidly, even though its species composition differs from the immediate pre-fire community. Seeds of Interior Lodgepole pine (P. contorta var. latifolia), a serotinous fire-adapted conifer of the intermountain region of North America, typically germinate within a year of the fire event. In contrast, shade-tolerant conifers may take decades to re-establish a population after fire, via recruitment from seeds in seedbanks or by wind dispersal from nearby individuals (Savage et al., 2013). While these examples from forests illustrate relatively subtle differences in pace of response, ecosystems dominated by organisms with more widely differing reproductive rates and strategies (annual grasses vs. trees, for example) may display a greater range of rates of response to disturbance. Thus, the time definition for a “resilient” response is a system-specific property.
Some communities may take decades to centuries to resemble the original pre-disturbance condition. These time scales reflect the wide range of processes (seed dispersal, development of microclimates and habitat for pollinators and seed-dispersing animals, seedling establishment, presence of symbionts, growth, competition, age to first reproduction) necessary for post-disturbance community development, and do not necessarily indicate a resilience failure. In the interior western US, a number of species of shrubs and early successional trees in the genera Ceanothus, Quercus, Robinia, and others, may capture and dominate a post-fire site for decades following a stand-replacing event (Figure 8). Such type conversions are widely observed following high-severity fires, especially during the contemporary period of intensive management and climate-change effects in many western forests. However, whether they are permanent or transitory requires decades of controlled observation (Guiterman et al., 2018). Distinguishing slow processes of ecological successional from threshold-type conversions is a major obstacle to resilience ecology.
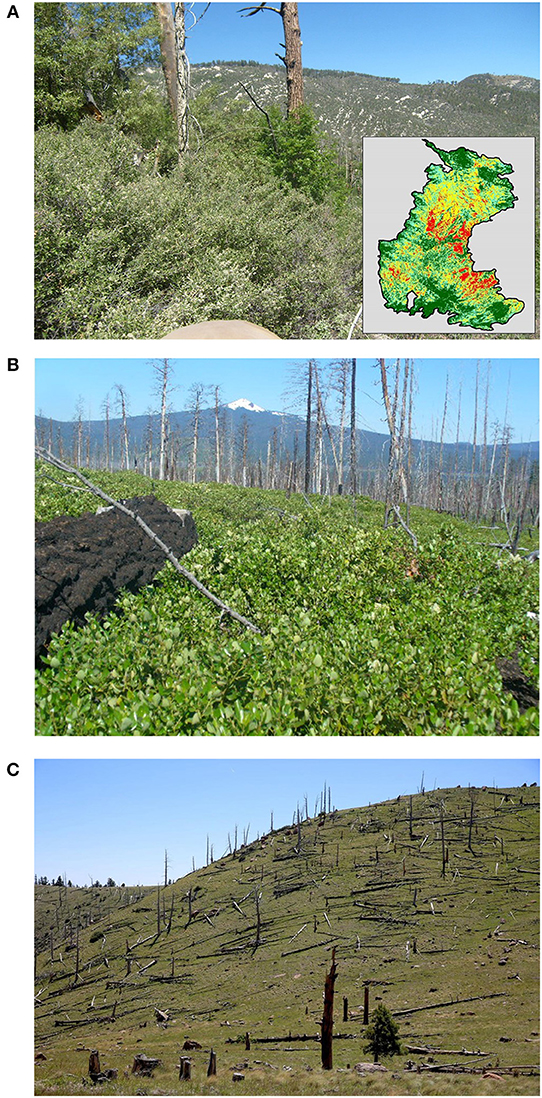
Figure 8. Examples of persistent post-fire community conversions: (A) Post-fire native shrubfield in former pine/mixed-conifer forest, Santa Catalina Mountains, AZ following the 2003 Aspen fire (photo: L. Maghran, University of Arizona). Inset: burn severity map. (B) Ceanothus velutinus dominates post-fire succession following the 2003 Davis Lake fire in lodgepole pine, west-central Oregon southeast of Maiden Peak. (C) Persistent type conversion at Mt Elden, AZ in 2017, 40 years after the 1977 Radio Fire (photo: J Bacon, Arizona Daily Sun).
The temporal and spatial axes in this framework are not completely independent. Other things being equal—for example, the percent of overstory trees killed in a disturbance event—large high-severity patches will take longer to recover if seed dispersal distance is limiting, whereas small high-severity patches can be colonized relatively quickly, representing a gap-dynamic mode of recruitment.
Biological Level of Organization
Ecosystems are complex, structured systems. A variety of organizational frameworks are used in their description, including evolutionary relationships, energetic and trophic levels, and levels of biological organization or hierarchy (Allen and Starr, 2017). The concept of ecological resilience intersects with levels of biological organization, in the same sense that resilience is expressed differentially along axes of space and time.
Individuals
The first layer of resilient response of an ecosystem to disturbance is at the level of individual organisms. Here we use the individual as the base of the ecological hierarchy, acknowledging from the outset that the concept of the unitary individual is problematic for many organisms (such as many fungi and clonal plants). The effects of disturbance or environmental variation on individuals are often a primary response variable in disturbance ecology. The most obvious effects are demographic: survival or mortality, changes in growth rate, and reproductive rate (Lloret et al., 2011; Keeling and Sala, 2012). Physiological and anatomical effects at the level of the individual, such as hydraulic failure (e.g., via tracheid cavitation), lethal leaf dehydration, changes in tracheid, ray, and resin duct anatomy in fire-exposed conifers, and formation of fire scars, are also demonstrable evidence of disturbance effects on individuals (Skov et al., 2004; McDowell et al., 2008; Arbellay et al., 2014a,b; Smith et al., 2016). Individual responses at this level of organization contributing to survival are the mechanism of the resistance phase of Millar et al. (2007).
The response of individuals to disturbance indicates life history evolution in the most direct sense. Many conifers in fire-prone ecosystems have multiple adaptations to survive low-intensity surface fires, including thick bark, lifted crowns, and physiological mechanisms for compartmentalizing wounds (Keeley, 2012). Other species in fire-prone environments such as Mediterranean chaparral communities have evolved the ability to re-sprout basally or epicormically to regrow damaged stems or crowns following moderate to high intensity heat exposure. The morphology and functional anatomy and history of grasses, particularly perennial bunchgrasses, reflect the joint evolutionary influence of grazing and fire favoring individual persistence.
Communities in which a large proportion of individuals persist through a disturbance event (fire, windstorm, drought, insect outbreak) can recover relatively quickly following cessation of the disturbance. Individual survival tends to stabilize ecological communities and promote return to the pre-disturbance community. Evidence that individuals may be reaching the limits of their persistence include reduced growth rates and physiological function, which may in turn predispose individuals to mortality from stressors (such as heat output and tissue damage during wildfire) that they might otherwise be able to tolerate (van Mantgem et al., 2018).
Populations
Where many individuals do not survive disturbance (e.g., higher-severity fire, drought, or insect outbreak), the resilient response occurs at the next higher hierarchical level of population-level recovery processes. Here the principle response is the establishment of new cohorts of individuals, which may be progeny of either survivors or killed plants. Population-level recruitment may create relatively even-aged cohorts, or episodic individual recruitment in gap-phase dynamics (Heyerdahl et al., 2012, 2014, 2019).
A different set of mechanisms governs population-level recovery processes compared to individual persistence. Life history adaptations that promote recruitment in post-disturbance environments include serotinous cones, seed masting and dispersal, seed scarification, rapid seedling establishment and growth; in animals, adaptations include variable habitat selection and spatial migration. These adaptations allow species with varying functional traits to recover following fire, promoting their persistence into the post-fire community (Enright et al., 1998; Barton and Poulos, 2018). Some post-disturbance communities (e.g., Rocky Mountain lodgepole pine communities) are highly similar to in recovery pre-fire compositions, whereas others are characterized by turnover in species composition in space and time, reflecting interactions among species including competition, facilitation, and the influence of initial conditions, which can govern how communities reassemble following disturbance (Temperton et al., 2004). Successional processes lead ecological communities to change over space and time, sometimes in predictable ways that revert toward the pre-disturbance condition, while at other times leading to novel combinations (Falk, 2013;Standish et al., 2014).
The time progression of population-level recovery can be influenced strongly by spatial properties of disturbance (Cansler and McKenzie, 2014). As noted previously, large high-severity patches with few or no surviving trees lack internal seed sources, so recolonization must be supported by propagules dispersing into the burned area. Where seed dispersal distances are small in relation to patch size, multiple generations requiring decades to centuries may be required before the first generation of seeds reaches the most interior areas. For example, the center of a 795 ha (1,964 ac) isodiametric patch in area is 1,591 m from the nearest seed trees on the margin of the patch if it lacks internal seed sources (Haire and McGarigal, 2010). Assuming that the majority of conifer seed dispersal distances are 125–250 m (Owen et al., 2017; Haffey et al., 2018), and age to first reproduction of 20–25 year, seeds from successive generations of trees might not reach the center of the patch for 127–318 year.
Community and Plant Functional Type Transitions
Once the thresholds for both persistence (individual survival) and recovery (population re-establishment) have been exceeded, the system must reorganize at a higher organizational level. Multiple individuals of interacting species comprise an ecological community; if disturbance alters biophysical conditions, different species may be favored in the post-fire environment than previously. This change is commonly observed as a shift in dominant species in the period immediately following disturbance (Coop et al., 2016; Haffey et al., 2018). How long that shift persists, and the trajectory of return to the pre-disturbance community, depends on community composition, trait diversity among species, properties of the disturbance, retention of soils and other components crucial to vegetation recovery, and the climate of the post-disturbance period (Figure 8).
Alternative post-disturbance successional pathways may be more widespread in forest ecosystems than previously appreciated (Weber et al., 2014), as are hysteretic trajectories in which return toward the pre-disturbance state is not simply a reversal of degradation pathways, especially in the vicinity of critical transition states (Scheffer et al., 2001; Adams, 2013; Suding et al., 2016). Whether these are transient or lead to alternative metastable states remains poorly constrained in complex real ecosystems during periods governed by community disassembly and assembly processes (Temperton et al., 2004; Bascompte and Stouffer, 2009). Overly-simplified univariate conceptual models, such as the ubiquitous ball-and-cup model, fail to account for multivariate community responses, heterogeneous adaptive landscapes, spatial and temporal variation in environmental conditions, and feedbacks between target species environmental conditions, and ecosystem processes (Beisner et al., 2003; Kitzberger et al., 2012). Models that link functional traits to post-fire environments have greater predictive power, but these have not been widely adopted in resilience ecology (Laughlin and Messier, 2015).
Effects of disturbance magnitude are recognized in traditional resilience heuristics as the likelihood of a system transitioning to an alternative basin of attraction (Scheffer et al., 2001). Severe disturbance may eliminate previously dominant species from a community, resulting in altered composition or structure, or trigger conversion in dominant plant functional types (Laughlin et al., 2018). When the effects of disturbance include severe alteration of the soil environment and hydrological function, a tipping point response may follow in which the system is so profoundly altered as to be unlikely to return to its pre-disturbance state.
Scaled Resilience and Ecosystem Management
The scaled resilience model can guide ecosystem management in significant ways. In this section, we explore potential utility of resilience theory, including the application of scaled resilience to management of wildland fire and the systems in which it occurs, under likely climatic regimes of the mid-twenty-first century, using wildland fire as an exemplar.
A number of studies have focused on applications of resilience theory to ecosystem management (Janssen et al., 1999; Gunderson, 2000) and the complex social-ecological systems that natural-resource agencies are charged with managing (Berkes et al., 2000; Carpenter et al., 2001; Walker et al., 2002). The focus of a management regime may include maintaining or restoring resilience of identified species populations (or in some cases, individuals) of plants or animals, pre-disturbance community types, habitat resources of wildlife species of interest, stable and intact soil environment, surface or groundwater quantity and quality, or riparian and hydrological structure and function. These elements would be the object of conservation or restoration focus, for which resilience consists of maintaining a range of values within the estimated envelope of natural variability (Keane et al., 2018). Managing for resilience itself, instead of specified ecosystem states, exemplifies the novelty of this approach and the importance of a sound theory of ecological resilience (Benson and Garmestani, 2011; Bowker et al., 2013; North et al., 2019).
So stated, management objectives ultimately aim to increase the resilience of an ecosystem, landscape, or community to a range of stressors. In the context of the scaled resilience framework, this goal can be re-stated as reducing the probability that an uncharacteristically severe disturbance will exceed a critical scaled threshold, leading to the development of an alternative ecosystem state or an uncharacteristically long recovery time (Standish et al., 2014; Lucash et al., 2017). Land managers can implement a range of measures prior to, during, and after disturbance (such as wildfire) that may influence the scaled resilience of the system in the post-fire recovery period. Resilience-focused management is system specific and draws on its evolved resistance, recovery, and reorganization potential (Larson et al., 2013; Thomas and Waring, 2014).
Because of nearly a century of fire exclusion and fuel accumulation, and exacerbated by rapid climate change, landscape fire management is becoming increasingly focused on ecosystem resilience rather than a strict return to historical conditions (Drever et al., 2006; Reynolds et al., 2013; Stephens et al., 2016). Managers have a number of choices for pre-fire management actions, which include mechanical fuel reduction treatments, such as thinning or mastication, or prescribed burning, to reduce the potential behavior and severity of a subsequent wildfire. These treatments can improve forest resilience by reducing tree density and spatial fuel connectivity, which can modify subsequent fire behavior and improve understory plant diversity by allowing increased light penetration to the forest floor. Thinning treatments may also help avert threshold ecosystem change by ameliorating some climate-related stresses, such as shortages of available soil moisture (Kolb et al., 2007). In scaled resilience terms, fuels treatments aim to maintain the natural spatial and temporal properties of the fire regime, and to prevent higher-severity disturbances that will alter the community at higher levels of organization (Yocom, 2013).
Management actions during wildfires also influence ecosystem resilience significantly. With an average of over 6 million ha burned in wildfire per year in the continental US and Canada, management actions taken during wildfire incidents can have substantial positive and negative effects on resilience. If high severity fire in low-severity fire regimes can push ecosystems past tipping points into new configurations of composition and structure, then wildfire management is likewise an opportunity to affect subsequent ecosystem resilience and work toward having more fire that is the appropriate severity for the ecosystem. While fire operations attempt to contain uncharacteristic fire behavior, some measures used commonly in firefighting (such as cutting wide firebreaks using bulldozers and other heavy equipment, and high-severity burnout operations) can have persistent effects that can persist after the fire is controlled (Figure 9).
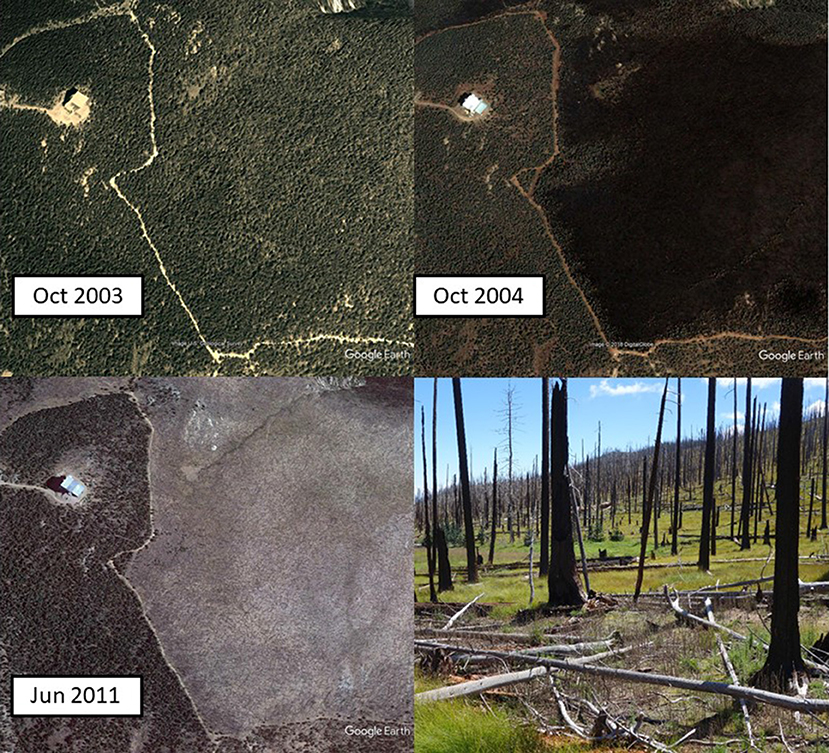
Figure 9. High-severity fire effects resulted from a tactical burnout conducted during the 2004 Nuttall-Gibson complex, Pinaleño Mountains, AZ to protect a high-value astronomical installation. Google Earth images from October 2003 (pre-fire, upper left), October 2004 (immediate post-fire, upper right), and June 2011 (seven years post fire, lower left). Lower right: stand condition as of 2010 (photo: Ann Lynch, US Forest Service).
Lastly, post-wildfire management can shape the future trajectories of a landscape. Post-fire management can enhance the capacity of populations and communities to recover from severe disturbance, and moderate the potential for reorganization (Stevens-Rumann et al., 2012). In many parts of the world, post-fire management actions start almost immediately with rehabilitation measures. These measures are designed to focus on mitigating short-term post-fire effects and stabilizing the burned area (Robichaud et al., 2009) to manage water runoff and quality, and minimize loss of soil productivity (Beyers, 2004), as well as restoring native vegetation and controlling non-native invasive species (Beyers, 2004; Robichaud et al., 2009). In some cases there are no active post-fire treatments, allowing post-fire recovery to progress naturally provided there is no risk to life and property (Robichaud et al., 2000). When high-severity patches are large, managers may try to overcome the limitations of spatial scale by dispersing propagules or rooted plants to bypass the uncertainties in natural dispersal and establishment.
Future Prospects: Resilience Ecology in a Changing World
The principle of maintaining or re-establishing historical evolutionary conditions has been a fundamental pillar of restoration ecology (Palmer et al., 2016). Restoration ecologists define these conditions by the use of reference sites, which represent environments that are as close to the historical condition as can be attained in today's world (Egan and Howell, 2005). Protected areas, parks, and wilderness reserves are frequently used to represent historical references, under the assumption that they are closer to historical conditions than more extensively modified environments in less rigorously protected areas (White and Walker, 1997). Reference conditions are guides to restoring communities and ecosystems, although generally not interpreted literally as the desired future condition (Jackson and Hobbs, 2009; Higgs et al., 2014).
Even so, reference conditions are increasingly problematic for at least two reasons. First, truly unmodified and non-degraded systems are increasingly difficult to find, because human influence has become so pervasive. Even individual sites that are remote from human activity are altered by changes in regional species (including both extinctions and new arrivals), hydrological and biogeochemical fluxes, landscape connectivity influences on migration and dispersal (Wilcove, 2008), and the ubiquitous effects of altered climate.
Second, it cannot be assumed in every case that historical communities or species distributions are optimally adaptive in a rapidly changing world. Abundant paleoecological evidence documents extensive changes in species geographic distributions and the composition of ecological communities through time, exemplified by the response of species distributions to millennial shifts in climate regime (Jackson et al., 2009). While mobile, short-lived species may be able to adapt their ranges to accommodate predicted climate velocity in the twenty-first century, longer-lived sessile species such as trees may lag climate substantially (IPCC, 2014). Many extant North American forests originated largely during the a climatic episode of prolonged cooler temperatures extending from the late 1500s to late 1800s (Mann et al., 2009), significantly cooler and wetter than conditions predicted for the middle twenty-first century and beyond. Thus, current tree species distributions may not match the future climatic envelope, requiring a more adaptive definition of reference conditions that is not tied to a specific geography. The fact of a rapidly changing world means that resilience, especially the phases of recovery and reorganization, must be understood as an adaptive response to changing conditions, not simply a return to a past state.
Landscape-scale wildfire represents an increasing stressor on the stability of ecosystems, especially interacting with changing climate (Schoennagel et al., 2017). Kitzberger et al. (2017) projected increases of 200–>500% in annual area burned by mid-century based on seasonal climate drivers projected under a mid-range GHG emissions scenario. Total annual area burned in high severity fire, measured using the Monitoring Trends in Burn Severity (MTBS) dataset, has increased since 1984 in some of the western U.S. and much of the Southwest (Miller et al., 2009; Dillon et al., 2011; Picotte et al., 2016). A changing climate creates a large potential for changes in the extent, severity and spatial pattern of fire regimes (Holden et al., 2018; Singleton et al., 2019). As fire sizes increase, the potential increases in burn severity, high severity patch size and the spatial pattern of severity may increase the proportion of the landscape in more homogeneous patches of post-fire vegetation recovery (Cansler and McKenzie, 2014). Future climate may also not provide conditions favorable for seedling establishment in existing locations, reducing the probability of post-fire recovery (Davis et al., 2019; Law et al., 2019). Collectively, these trends point to an increasing probability of massive reorganization of forest ecosystems on a scale that has not been previously observed for thousands of years. Significant effects of wildfire-climate change interactions are already documented in boreal (Johnstone et al., 2010; Mann et al., 2012), Mediterranean (Mitchell et al., 2009; Guiot and Cramer, 2016) ecosystems.
Ecological resilience requires cross-scale thinking, from individual safe sites to entire landscapes, including forests and fire regimes in their full spatial and temporal extent. Similarly, resilience requires taking a long view, because ecological time is often longer than our typical narrow temporal frame of reference. What may appear to be novel post-disturbance trajectories may actually be slow recovery arcs beyond our ability to estimate. Finally, some cases of reorganization may be not only unavoidable but also adaptive to future conditions. We cannot assume that all types, or even biome conversions, are adverse outcomes; there may be cases in which ecosystem adaptation will take forms that do not align with our limited perception of ecological change. Applying a scaling framework to post-disturbance ecological response can identify key mechanisms that will help ecosystems adapt to a rapidly changing and stressful world.
Author Contributions
DF originated the concept and led overall manuscript preparation. DF, AW, and AT wrote sections of the paper and co-edited the final manuscript.
Conflict of Interest Statement
The authors declare that the research was conducted in the absence of any commercial or financial relationships that could be construed as a potential conflict of interest.
Acknowledgments
This paper originated during a workshop on fire and ecological resilience organized in 2014 by the Southwest Fire Science Consortium in Tucson, AZ (Grant #09-S-04-13 from the Joint Fire Science Program); additional support was provided by the University of Arizona Water, Environmental, and Energy (WEES) Initiative and the Arizona Technology and Research Initiative Fund (TRIF). Thanks to Connie Millar and Dave Peterson (USDA Forest Service), and Nate Stephenson (US Geological Survey) for valuable suggestions during concept development. Thanks to Emily Heyerdahl and Ann Lynch (USDA Forest Service), Andrew Merschel (Oregon State University), and Lauren Maghran and James Malusa (University of Arizona) for assistance with figures. The authors gratefully acknowledge the commentary and suggestions by three reviewers, whose suggestions help improve an earlier version of the manuscript. DF was supported by a Faculty Fellowship from the University of Arizona Udall Center in Public Policy.
Footnotes
1. ^US data from National Interagency Fire Center (https://www.nifc.gov/fireInfo/fireInfo_statistics.html). Data for Canada from http://cwfis.cfs.nrcan.gc.ca.
References
Adams, M. A. (2013). Mega-fires, tipping points and ecosystem services: Managing forests and woodlands in an uncertain future. Forest Ecol. Manage. 294, 250–261. doi: 10.1016/j.foreco.2012.11.039
Allen, C. D. (2007). Interactions across spatial scales among forest dieback, fire, and erosion in northern New Mexico landscapes. Ecosystems 10, 797–808. doi: 10.1007/s10021-007-9057-4
Allen, T. F. H., and Starr, T. B. (2017). Hierarchy: Perspectives for Ecological Complexity. Chicago, IL: University of Chicago Press.
Andersen, T., Carstensen, J., Hernández-García, E., and Duarte, C. M. (2009). Ecological thresholds and regime shifts: approaches to identification. Trends Ecol. Evol. 24, 49–57. doi: 10.1016/j.tree.2008.07.014
Arbellay, E., Stoffel, M., Sutherland, E. K., Smith, K. T., and Falk, D. A. (2014a). Changes in tracheid and ray traits in fire scars of North American conifers and their ecophysiological implications. Ann. Bot. 114, 223–232. doi: 10.1093/aob/mcu112
Arbellay, E., Stoffel, M., Sutherland, E. K., Smith, K. T., and Falk, D. A. (2014b). Resin duct size and density as ecophysiological traits in fire scars of Pseudotsuga menziesii and Larix occidentalis. Ann. Bot. 114, 973–980. doi: 10.1093/aob/mcu168
Axelson, J. N., Alfaro, R. I., and Hawkes, B. C. (2009). Influence of fire and mountain pine beetle on the dynamics of lodgepole pine stands in British Columbia, Canada. Forest Ecol. Manage. 257, 1874–1882. doi: 10.1016/j.foreco.2009.01.047
Barton, A. M., and Poulos, H. M. (2018). Pine vs. oaks revisited: Conversion of Madrean pine-oak forest to oak shrubland after high-severity wildfire in the Sky Islands of Arizona. Forest Ecol. Manage. 414, 28–40. doi: 10.1016/j.foreco.2018.02.011
Bascompte, J., and Stouffer, D. B. (2009). The assembly and disassembly of ecological networks. Philos. Trans. R.Soc. B Biol. Sci. 364, 1781–1787. doi: 10.1098/rstb.2008.0226
Beisner, B. E., Haydon, D. T., and Cuddington, K. (2003). Alternative stable states in ecology. Front. Ecol. Environ. 1, 376–382. doi: 10.1890/1540-9295(2003)001[0376:ASSIE]2.0.CO;2
Benson, M. H., and Garmestani, A. S. (2011). Can we manage for resilience? The integration of resilience thinking into natural resource management in the United States. Environ. Manage. 48, 392–399. doi: 10.1007/s00267-011-9693-5
Berkes, F., Folke, C., and Colding, J. (2000). Linking Social and Ecological Systems: Management Practices and Social Mechanisms for Building Resilience. Cambridge, UK: Cambridge University Press.
Beyers, J. L. (2004). Postfire seeding for erosion control: effectiveness and impacts on native plant communities. Conserv. Biol. 18, 947–956. doi: 10.1111/j.1523-1739.2004.00523.x
Bonnet, V. H., Schoettle, A. W., and Shepperd, W. D. (2005). Postfire environmental conditions influence the spatial pattern of regeneration for Pinus ponderosa. Can. J. Forest Res. Revue Can. Recherche Forestiere 35, 37–47. doi: 10.1139/x04-157
Bowker, M. A., Miller, M. E., Belote, R. T., and Garman, S. L. (2013). Ecological Thresholds as a Basis for Defining Management Triggers for National Park Service Vital Signs—Case Studies for Dryland Ecosystems. Reston, VA: US Department of the Interior, US Geological Survey.
Brown, J. H., and West, G. B. (eds.) (2000). Scaling in Biology. New York, NY: Oxford University Press.
Buchalski, M. R., Fontaine, J. B., Heady, P. A., Hayes, J. P., and Frick, W. F. (2013). Bat response to differing fire severity in mixed-conifer forest USA, California. PLoS ONE 8:0057884. doi: 10.1371/journal.pone.0057884
Cansler, C. A., and McKenzie, D. (2014). Climate, fire size, and biophysical setting control fire severity and spatial pattern in the northern Cascade U. S. A. Range. Ecol. Appl. 24, 1037–1056. doi: 10.1890/13-1077.1
Carpenter, S., Walker, B., Anderies, J. M., and Abel, N. (2001). From metaphor to measurement: resilience of what to what? Ecosystems 4, 765–781. doi: 10.1007/s10021-001-0045-9
Carpenter, S. R., Kraft, C. E., Wright, R., He, X., Soranno, P. A., and Hodgson, J. R. (1992). Resilience and resistance of a lake phosphorus cycle before and after food web manipulation. Am. Natural. 140, 781–798. doi: 10.1086/285440
Chambers, J. C., Bethany Bradley, A., Cynthia Brown, S., DAntonio, C., Matthew Germino, J., James Grace, B., et al. (2013). Resilience to Stress and Disturbance, and Resistance to Bromus tectorum L. Invasion in Cold Desert Shrublands of Western North America. Ecosystems 17, 360–375. doi: 10.1007/s10021-013-9725-5
Clark, J. S. (1998). Why trees migrate so fast: Confronting theory with dispersal biology and the paleorecord. Am. Natural. 152, 204–224. doi: 10.1086/286162
Coop, J. D., Parks, S. A., McClernan, S. R., and Holsinger, L. M. (2016). Influences of prior wildfires on vegetation response to subsequent fire in a reburned southwestern landscape. Ecol. Appl. 26, 346–354. doi: 10.1890/15-0775
Cortina, J., Tomás Maestre, F., Vallejo, R., Baeza, M. J., Valdecantos, A., and Pérez-Devesa, M. (2006). Ecosystem structure, function, and restoration success: are they related? J. Nat. Conserv. 14, 152–160. doi: 10.1016/j.jnc.2006.04.004
Dale, V. H., Joyce, L. A., McNulty, S., Ayres, M. P., Flannigan, M. D., Hanson, P. J., et al. (2001). Climate change and forest disturbances. BioScience 51, 723–734. doi: 10.1641/0006-3568(2001)051[0723:CCAFD]2.0.CO;2
Davis, K. T., Dobrowski, S. Z., Higuera, P. E., Holden, Z. A., Veblen, T. T., Rother, M. T., et al. (2019). Wildfires and climate change push low-elevation forests across a critical climate threshold for tree regeneration. Proc. Natl. Acad. Sci. Maneta. 116, 6193–6198. doi: 10.1073/pnas.1815107116
DeBano, L. F. (2000). The role of fire and soil heating on water repellency in wildland environments: a review. J. Hydrol. 231, 195–206. doi: 10.1016/S0022-1694(00)00194-3
Dillon, G., Morgan, P., and Holden, Z. (2011). Mapping the potential for high severity wildfire in the western United States. Fire Manage. Today 71, 25–28.
Drever, R. C., Peterson, G., Messier, C., Bergeron, Y., and Flannigan, M. (2006). Can forest management based on natural disturbances maintain ecological resilience? Can. J. Forest Res. 36, 2285–2299. doi: 10.1139/x06-132
Dudley, J. G., Saab, V. A., and Hollenbeck, J. P. (2012). Foraging-habitat selection of Black-backed Woodpeckers in forest burns of southwestern Idaho. Condor 114, 348–357. doi: 10.1525/cond.2012.110020
Egan, D., and Howell, E. A. (eds.). (2005). The Historical Ecology Handbook: A Restorationists Guide to Reference Ecosystems. Washington, DC: Island Press.
Engstrom, R. T. (2010). First-order fire effects on animals: review and recommendations. Fire Ecol. 6, 115–130. doi: 10.4996/fireecology.0601115
Enright, N. J., Marsula, R., Lamont, B. B., and Wissel, C. (1998). The ecological significance of canopy seed storage in fire-prone environments: A model for non-sprouting shrubs. J. Ecol. 86, 946–959. doi: 10.1046/j.1365-2745.1998.00312.x
Falk, D. A. (2006). Process-centered restoration and reference dynamics. J. Nat. Conserv. 14, 140–151. doi: 10.1016/j.jnc.2006.04.005
Falk, D. A. (2013). “Are Madrean ecosystems approaching tipping points? Anticipating interactions of landscape disturbance and climate change.” in Merging Science and Management in a Rapidly Changing World: Biodiversity and Management of the Madrean Archipelago, III, eds G. J. Gottfried, P. F. Ffolliott, B. S. Gebow, L. G. Eskew, and L.C. Collins (Tucson, AZ: U.S. Department of Agriculture, Forest Service, Rocky Mountain Research Station. Fort Collins CO).
Falk, D. A. (2017). Restoration Ecology, Resilience, and the Axes of Change. Ann. Missouri Botan. Garden 102, 201–217. doi: 10.3417/2017006
Falk, D. A., and Millar, C. I. (2016). “The influence of climate variability and change on the science and practice of restoration ecology,” in Foundations of Restoration Ecology, Second Edition, eds A. M. Palmer, B. J. Zedler, and A. D. Falk (Washington, DC: Island Press).
Fulé, P. Z., Yocom, L. L., Montaño, C. C., Falk, D. A., and Cerano, J., Villanueva-Díaz, J. (2012). Testing a pyroclimatic hypothesis on the Mexico–United States border. J. Ecol. Villanueva-Díaz. 93, 1830–1840. doi: 10.1890/11-1991.1
Guiot, J., and Cramer, W. (2016). Climate change: the 2015 Paris Agreement thresholds and Mediterranean basin ecosystems. Science 354, 465–468. doi: 10.1126/science.aah5015
Guiterman, C. H., Ellis Margolis, Q., Craig Allen, D., Donald Falk, A., and Thomas Swetnam, W. (2018). Long-term persistence and fire resilience of oak shrubfields in dry conifer forests of northern New Mexico. Ecosystems 21, 943–959. doi: 10.1007/s10021-017-0192-2
Gunderson, L. H. (2000). Ecological resilience—in theory and application. Ann. Rev. Ecol. System. 31, 425–439. doi: 10.1146/annurev.ecolsys.31.1.425
Haffey, C., Sisk, T. D., Allen, C. D., Thode, A. E., and Margolis, E. Q. (2018). Limits to ponderosa pine regeneration following large high-severity forest fires in the United States Southwest. Fire Ecol. 14, 143–163. doi: 10.4996/fireecology.140114316
Haire, S. L., and McGarigal, K. (2010). Effects of landscape patterns of fire severity on regenerating ponderosa pine forests (Pinus ponderosa) in New Mexico and Arizona, U. S. A. Landscape Ecol. 25, 1055–1069. doi: 10.1007/s10980-010-9480-3
Halpern, C. B. (1988). Early successional pathways and the resistance and resilience of forest communities. Ecology 69, 1703–1715. doi: 10.2307/1941148
Hershkovitz, Y., and Gasith, A. (2013). Resistance, resilience, and community dynamics in mediterranean-climate streams. Hydrobiologia 719, 59–75. doi: 10.1007/s10750-012-1387-3
Heyerdahl, E. K., Lertzman, K., and Wong, C. M. (2012). Mixed-severity fire regimes in dry forests of southern interior British Columbia, Canada. Can. J. Forest Res. 42, 88–98. doi: 10.1139/x11-160
Heyerdahl, E. K., Loehman, R., and Falk, D., A. (2019). A multi-century history of fire regimes along a transect of mixed-conifer forests in central Oregon, U. S. A. Can. J. Forest Res. 49, 76–86. doi: 10.1139/cjfr-2018-0193
Heyerdahl, E. K., Loehman, R. A., and Falk, D., A. (2014). Mixed-severity fire in lodgepole pine dominated forests: are historical regimes sustainable on Oregons Pumice Plateau, USA? Can. J. Forest Res. 44, 593–603. doi: 10.1139/cjfr-2013-0413
Higgs, E., Falk, D. A., Guerrini, A., Hall, M., Harris, J., Richard Hobbs, J., et al. (2014). The changing role of history in restoration ecology. Front. Ecol. Environ. 12, 499–506. doi: 10.1890/110267
Hobbs, R. J., and Norton, D. A. (1996). Towards a conceptual framework for restoration ecology. Restoration Ecol. 4, 93–110. doi: 10.1111/j.1526-100X.1996.tb00112.x
Holden, Z. A., Swanson, A., Luce, C. H., Jolly, W. M., Maneta, M., Oyler, J. W., et al. (2018). Decreasing fire season precipitation increased recent western US forest wildfire activity. Proc. Natl. Acad. Sci. U.S.A. 115, E8349–E57. doi: 10.1073/pnas.1802316115
Holling, C. S. (1973). Resilience and stability of ecological systems. Ann. Rev. Ecol. Sys. 4, 1–23. doi: 10.1146/annurev.es.04.110173.000245
Iniguez, J., Swetnam, T., and Baisan, C. H. (2009). Spatially and temporally variable fire regime on rincon peak, Arizona, U. S. A. Fire Ecol. 5, 3–21. doi: 10.4996/fireecology.0501003
IPCC (2014). “Climate Change 2014: Synthesis Report,” in Contribution of Working Groups, I., II and III to the Fifth Assessment Report of the Intergovernmental Panel on Climate Change, eds R. K. Pachauri, and L.A. Meyer (Geneva).
IPCC (2018). Global Warming of 1.5°C, an IPCC Special Report on the Impacts of Global Warming of 1.5°C Above pre-Industrial levels (SR1.5). Geneva: Intergovernmental Panel on Climate Change (IPCC).
Jackson, S. T., Betancourt, J. L., Booth, R. K., and Gray, S. T. (2009). Ecology and the ratchet of events: climate variability, niche dimensions, and species distributions. Proc. Natl. Acad. Sci. U.S.A.106, 19685–19692. doi: 10.1073/pnas.0901644106
Jackson, S. T., and Hobbs, R. J. (2009). Ecological restoration in the light of ecological history. Science 325, 567–569. doi: 10.1126/science.1172977
Janssen, M. A., and Carpenter, S., R. (1999). Managing the resilience of lakes: a multi–agent modeling approach. Conserv. Ecol. 3. doi: 10.5751/ES-00145-030215
Johnstone, J. F., Allen, C. D., Franklin, J. F., Frelich, L. E., Harvey, B. J., Higuera, P. E., et al. (2016). Changing disturbance regimes, ecological memory, and forest resilience. Front. Ecol. Environ. 14, 369–378. doi: 10.1002/fee.1311
Johnstone, J. F., Chapin, F., S, Hollingsworth, T. N., Mack, M. C., Romanovsky, V., and Turetsky, M. (2010). Fire, climate change, and forest resilience in interior Alaska. Can. J. Forest Res. 40, 1302–1312. doi: 10.1139/X10-061
Jolly, W. M., Cochrane, M. A., Freeborn, P. H., Holden, Z. A., Brown, T. J., Williamson, G. J., et al. (2015). Climate-induced variations in global wildfire danger from 1979 to 2013. J. Nat. Commun. Bowman. 6:7537. doi: 10.1038/ncomms8537
Keane, R. E., Loehman, R. A., Holsinger, L. M., Falk, D. A., Higuera, P., Hood, S. M., et al. (2018). Use of landscape simulation modeling to quantify resilience for ecological applications. Ecosphere 9:e02414. doi: 10.1002/ecs2.2414
Keane, R. E., Tomback, D. F., Aubry, C. A., Bower, A. D., and Campbell, E. M., Cripps, C. L., et al. (2012). RMRS-GTR-279. Fort Collins McKinney, CO: US Department of Agriculture, Forest Service, Rocky Mountain Research Station. 108 p., A range-wide restoration strategy for whitebark pine (Pinus albicaulis). J Gen. Tech. Rep. 279. doi: 10.2737/RMRS-GTR-279
Keeley, J. E. (2012). Ecology and evolution of pine life histories. J. Ann. Forest Sci. 69, 445–453. doi: 10.1007/s13595-012-0201-8
Keeley, J. E., Pausas, J. G., Rundel, P. W., Bond, W. J., and Bradstock, R. A. (2011). Fire as an evolutionary pressure shaping plant traits. Trends Plant Sci. 16, 406–411. doi: 10.1016/j.tplants.2011.04.002
Keeling, E. G., and Sala, A. (2012). Changing growth response to wildfire in old-growth ponderosa pine trees in montane forests of north central Idaho. Global Change Biol. 18, 1117–1126. doi: 10.1111/j.1365-2486.2011.02574.x
Kitzberger, T., Aráoz, E., Gowda, J. H., Mermoz, M., and Morales, J. M. (2012). Decreases in fire spread probability with forest age promotes alternative community states, reduced resilience to climate variability and large fire regime shifts. Ecosystems 15, 97–112. doi: 10.1007/s10021-011-9494-y
Kitzberger, T., Falk, D. A., Westerling, A. L., and Swetnam, T. W. (2017). Direct and indirect climate controls predict heterogeneous early-mid 21st century wildfire burned area across western and boreal North America. PLoS ONE. 12:e0188486. doi: 10.1371/journal.pone.0188486
Kolb, T. E., Agee, J. K., Fule, P. Z., McDowell, N. G., Pearson, K., Sala, A., et al. (2007). Perpetuating old ponderosa pine. Forest Ecol. Manage. 249, 141–157. doi: 10.1016/j.foreco.2007.06.002
Lake, P. S. (2013). Resistance, resilience and restoration. Ecol. Manage. Restoration 14, 20–24. doi: 10.1111/emr.12016
Larson, A. J., Belote, R, T., Cansler, C, A., Parks, S, A., and Dietz, M, S. (2013). Latent resilience in ponderosa pine forest: effects of resumed frequent fire. J. Ecol. Appl. Dietz. 23, 1243–1249. doi: 10.1890/13-0066.1
Laughlin, D. C., Chalmandrier, L., Joshi, C., Renton, M., Dwyer, J. M., and Funk, J., L. (2018). Generating species assemblages for restoration and experimentation: a new method that can simultaneously converge on average trait values and maximize functional diversity. Methods Ecol. Evol. 9, 1764–1771. doi: 10.1111/2041-210X.13023
Laughlin, D. C., and Messier, J. (2015). Fitness of multidimensional phenotypes in dynamic adaptive landscapes. Trends Ecol. Evol. 30, 487–496. doi: 10.1016/j.tree.2015.06.003
Law, D. J., Adams, H. D., Breshears, D. D., Cobb, N. S., Bradford, J. B., Zou, B. C., et al. (2019). Bioclimatic envelopes for individual demographic events driven by extremes: plant mortality from drought and warming. Int. J. Plant Sci. 180, 53–62. doi: 10.1086/700702
Levin, S. A. (1992). The problem of pattern and scale in ecology. Ecology 73, 1943–1967. doi: 10.2307/1941447
Lloret, F., Keeling, E. G., and Sala, A. (2011). Components of tree resilience: effects of successive low-growth episodes in old ponderosa pine forests. Oikos 120, 1909–1920. doi: 10.1111/j.1600-0706.2011.19372.x
Lucash, M. S., Scheller, R. M., Gustafson, E. J., and Brian,. R. (2017). Spatial resilience of forested landscapes under climate change and management. J. Landscape Ecol. Sturtevant. 32, 953–969. doi: 10.1007/s10980-017-0501-3
Mann, D. H., Rupp, T. S., Olson, M. A., and Duffy, P. A. (2012). Is Alaskas boreal forest now crossing a major ecological threshold? Arctic Antarctic Alpine Res. 44, 319–331. doi: 10.1657/1938-4246-44.3.319
Mann, M. E., Zhang, Z., Rutherford, S., Bradley, R. S., Hughes, M. K., Shindell, D., et al. (2009). Global signatures and dynamical origins of the Little Ice Age and Medieval Climate Anomaly. Science 326, 1256–1260. doi: 10.1126/science.1177303
Margolis, E. Q., Swetnam, T. W., and Allen, C. D. (2011). Historical stand-replacing fire in upper montane forests of the Madrean Sky Islands and Mogollon Plateau, Southwestern, U. S. A. Fire Ecol. 7, 88–107. doi: 10.4996/fireecology.0703088
May, R. H. (1977). Thresholds and breaking points in ecosystems with a multiplicity of stable states. Nature 269, 471–477. doi: 10.1038/269471a0
McDowell, N., Pockman, W. T., Allen, C. D., Breshears, D. D., Cobb, N., Kolb, T., et al. (2008). Mechanisms of plant survival and mortality during drought: why do some plants survive while others succumb to drought? N. Phytol. 178, 719–739. doi: 10.1111/j.1469-8137.2008.02436.x
Millar, C. I., and Stephenson, N. L. (2015). Temperate forest health in an era of emerging megadisturbance. Science 349, 823–826. doi: 10.1126/science.aaa9933
Millar, C. I., Stephenson, N. L., and Stephens, S. L. (2007). Climate change and forests of the future: Managing in the face of uncertainty. Ecol. Appl. 17, 2415–2151. doi: 10.1890/06-1715.1
Miller, J. D., Safford, H. D., Crimmins, M., and Thode, A. E. (2009). Quantitative evidence for increasing forest fire severity in the Sierra Nevada and southern Cascade Mountains, California and Nevada, U. S. A. Ecosystems 12, 16–32. doi: 10.1007/s10021-008-9201-9
Mitchell, R. J., Simonson, W., Flegg, L., A., Santos, P., and Hall, J. (2009). A comparison of the resilience of four habitats to fire, and the implications of changes in community composition for conservation: a case study from the Serra de Monchique, Portugal. Plant Ecol. Diversity 2, 45–56. doi: 10.1080/17550870902752421
Neary, D. G., Klopatek, C. C., DeBano, L. F., and Ffolliott, P. F. (1999). Fire effects on belowground sustainability: A review and synthesis. Forest Ecol. Manage. 122, 51–71. doi: 10.1016/S0378-1127(99)00032-8
Neary, D. G., Ryan, K. C., Landsberg, J. A., and DeBano, L. (eds.). (2005). Wildland Fire in Ecosystems: Effects of Fire on Soils and Water Rocky Mountain Research Station, RMRS-GTR-42, Vol. 4 (Ft. Collins, CO). doi: 10.2737/RMRS-GTR-42-V4
North, M. P., Stevens, J. T., Greene, D. F., Coppoletta, M., Knapp, E. E., Latimer, A. M., et al. (2019). Tamm review: reforestation for resilience in dry western US forests. J. Forest Ecol. York Manage. 432, 209–224. doi: 10.1016/j.foreco.2018.09.007
O'Connor, C. D., Falk, D. A., Lynch, A. M., and Swetnam, T. W. (2014). Fire severity, size, and climate associations diverge from historical precedent along an ecological gradient in the Pinaleno Mountains, Arizona, USA. Forest Ecol. Manage. 329, 264–278. doi: 10.1016/j.foreco.2014.06.032
O'Connor, C. D., Garfin, G. M., Falk, D. A., and Thomas, W. (2011). Human pyrogeography: A new synergy of fire, climate and people is reshaping ecosystems across the globe. J. Geogr. Compass Swetnam 5, 329–350. doi: 10.1111/j.1749-8198.2011.00428.x
Owen, S. M., Sieg, C. H., Sánchez Meador, A. J., Ful,é, P. Z., Iniguez, J. M., Baggett, L. S., et al. (2017). Spatial patterns of ponderosa pine regeneration in high-severity burn patches. Forest Ecol. Manage. 405, 134–149. doi: 10.1016/j.foreco.2017.09.005
Palmer, M. A., Zedler, J. B., and Falk, D. A. (2016). Foundations of Restoration Ecology. Washington, DC: Island Press.
Parks, S. A., Holsinger, L. M., Panunto, M. H., Jolly, W. M., Dobrowski, S. Z., and Dillon, G. K. (2018). High-severity fire: evaluating its key drivers and mapping its probability across western US forests. Environ. Res. Lett. 13:044037. doi: 10.1088/1748-9326/aab791
Petraitis, P. S., and Latham, R. E. (1999). The importance of scale in testing the origins of alternative community states. Ecology 80, 429–442. doi: 10.1890/0012-9658(1999)080[0429:TIOSIT]2.0.CO;2
Picotte, J. J., Peterson, B., Meier, G., and Howard, S. M. (2016). 1984-2010 trends in fire burn severity and area for the conterminous US. Int. J. Wildland Fire 25, 413–420. doi: 10.1071/WF15039
Reilly, M. J., Dunn, C. J., Garrett Meigs, W., Thomas Spies, A., Robert Kennedy, E., John Bailey, D., et al. (2017). Contemporary patterns of fire extent and severity in forests of the Pacific Northwest, USA (1985–2010). Ecosphere 8:e01695. doi: 10.1002/ecs2.1695
Reynolds, R. T., Andrew Sanchez Meador, J., James Youtz, A., Nicolet, T., Megan Matonis, S., et al. (2013). Restoring Composition and Structure in Southwestern Frequent-Fire Forests: A Science-Based Framework for Improving Ecosystem Resiliency. Ft. Collins, CO: USDA Forest Service, Rocky Mountain Research Station.
Robichaud, P. R., Beyers, J. L., and Neary, D. G. (2000). Evaluating the Effectiveness of Postfire Rehabilitation Treatments. Gen. Tech. Rep. RMRS-GTR-63. 85. Fort Collins, CO: US Department of Agriculture, Forest Service, Rocky Mountain Research Station.
Robichaud, P. R., Lewis, S. A., Brown, R. E., and Ashmun, L. E. (2009). Emergency post-fire rehabilitation treatment effects on burned area ecology and long-term restoration. Fire Ecol. 5, 115–128. doi: 10.4996/fireecology.0501115
Rocha, J. C., Peterson, G., Bodin, Ö., and Levin, S. (2018). Cascading regime shifts within and across scales. J. Sci. Levin 362, 1379–1383. doi: 10.1126/science.aat7850
Rockweit, J. T., Franklin, A. B., and Carlson, P. C. (2017). Differential impacts of wildfire on the population dynamics of an old-forest species. Ecology 98, 1574–1582. doi: 10.1002/ecy.1805
Rowland, M. M., Suring, L. H., and Michael, J. (2010). “Advances in threat assessment Wisdom, their application to forest, rangeland management, 673-685. Assessment of habitat threats to shrublands in the Great Basin: a case study,” in Advances in threat assessment. Gen. Tech. Rep. PNW-GTR-802, eds M. John Pye, H. Michael Rauscher, Y. Sands, Danny Lee, C., and S. Jerome Beatty (Portland, OR: US Department of Agriculture, Forest Service, Pacific Northwest and Southern Research Stations).
Savage, M., Joy Mast, N., and Feddema, J. J. (2013). Double whammy: high-severity fire and drought in ponderosa pine forests of the Southwest. Can. J. Forest Res. 43, 570–583. doi: 10.1139/cjfr-2012-0404
Scheffer, M., Carpenter, S., Foley, J. A., Folke, C., and Walker, B. (2001). Catastrophic shifts in ecosystems. Nature 413, 591–596. doi: 10.1038/35098000
Schoennagel, T., Balch, J. K., Brenkert-Smith, H., Dennison, P. E., Harvey, B. J., Krawchuk, M. A., et al. (2017). Adapt to more wildfire in western North American forests as climate changes. Proc. Natl. Acad. Sci. Rasker 114, 4582–4590. doi: 10.1073/pnas.1617464114
Shaffer, K. E., and Laudenslayer, W. F. Jr. (2006). “Fire and animal interactions,” in Fire in Californias Ecosystems, eds N. G. Sugihara, W. J. Van Wagtendonk, E. K. Shaffer, J. Fites-Kaufman and A. E. Thode (Berkeley, CA: University of California Press).
Singleton, M. P., Thode, A., E., and Sánchez Meador, A., J., Jose, M. (2019). Increasing trends in high-severity fire in the southwestern USA from 1984 to 2015. J Forest Ecol. Iniguez Manage. 433, 709–719. doi: 10.1016/j.foreco.2018.11.039
Skov, K. R., Kolb, T. E., and Wallin, K. F. (2004). Tree size and drought affect ponderosa pine physiological response to thinning and burning treatments. Forest Sci. 50, 81–91.
Smith, K. T., Arbellay, E., Falk, D. A., and Sutherland, E. K. (2016). Macroanatomy and compartmentalization of recent fire scars in three North American conifers. Can. J. Forest Res. 46, 535–542. doi: 10.1139/cjfr-2015-0377
Standish, R. J., Hobbs, R. J., Mayfield, M. M., Bestelmeyer, B. T., Suding, K. N., Battaglia, L. L., et al. (2014). Resilience in ecology: Abstraction, distraction, or where the action is? J. Biol. Conser. Cramer 177, 43–51. doi: 10.1016/j.biocon.2014.06.008
Stephens, S. L., Collins, B. M., Biber, E., and Fulé, P. Z. (2016). US federal fire and forest policy: emphasizing resilience in dry forests. Ecosphere 7:e01584. doi: 10.1002/ecs2.1584
Stevens, J., and Falk, D. A. (2009). Can buffelgrass invasions be controlled in the American Southwest? Using invasion ecology theory to understand buffelgrass success and develop comprehensive restoration and management. Ecol. Restor. 27, 417–427. doi: 10.3368/er.27.4.417
Stevens-Rumann, C. S., Sieg, C. H., and Hunter, M. E. (2012). Ten years after wildfires: how does varying tree mortality impact fire hazard and forest resiliency? Forest Ecol. Manage. 267, 199–208. doi: 10.1016/j.foreco.2011.12.003
Suding, K., Spotswood, E., Chapple, D., Beller, E., and Gross, K. (2016). “Ecological dynamics and ecological restoration,” in Foundations of Restoration Ecology eds M. A. Palmer, J. D. Zedler, and D. A. Falk (Washington, DC: Island Press).
Sugihara, N. G., Van Wagtendonk, J. W., and and, Fites-Kaufman, J. (2006). “Fire as an ecological process,” in Fire in Californias ecosystems, eds N. Sugihara, W. J. van Wagtendonk, Kevin Shaffer, E., J. Fites-Kaufman and E. A. Thode (Berkeley, CA: University of California Press), 38–74.
Sutherland, J. P. (1974). Multiple stable states in natural communities. Am. Natural. 108, 859–873. doi: 10.1086/282961
Sutherland, J. P. (1990). Perturbations, resistance, and alternative views of the existence of multiple stable points in nature. Am. Natural. 136, 270–275. doi: 10.1086/285097
Swetnam, T. W., Farella, J., Roos, C. I., Liebmann, M. J., Falk, D. A., and Allen, C. D. (2016). Multi-scale perspectives of fire, climate and humans in western north America and the Jemez Mountains, U.S.A. Philos. Trans. R. Soc. B 371:20150168. doi: 10.1098/rstb.2015.0168
Temperton, V. M., Hobbs, R., J., Nuttle, T., and Halle, S. (2004). (ed.)∧(eds.) Assembly Rules and Restoration Ecology. (Washington, DC: Island Press).
Thomas, Z., and Waring, K. M. (2014). Enhancing resiliency and restoring ecological attributes in second-growth ponderosa pine stands in northern New Mexico, U.S.A. Forest Sci. 61, 93–104. doi: 10.5849/forsci.13-085
Turner, M. G. (2010). Disturbance and landscape dynamics in a changing world. Ecology 91, 2833–2849. doi: 10.1890/10-0097.1
USGCRP (2017). “Climate Science Special Report: Fourth National Climate Assessment, Volume, I.” In eds D. J. Wuebbles, D. W. Fahey, K. A. Hibbard, D. J. Dokken, B. C. Stewart, and T. K. Maycock (Washington, DC: U.S. Global Change Research Program), 470.
van Mantgem, P. J., Donald Falk, A., Emma Williams, C., Adrian Das, J., and Nathan Stephenson, L. (2018). Pre-fire drought and competition mediate post-fire conifer mortality in western US National Parks. Ecol. Appl. 28, 1730–1739. doi: 10.1002/eap.1778
van Mantgem, P. J., Stephenson, N. L., Byrne, J. C., Daniels, L. D., Franklin, J. F., Ful,é, P. Z., et al. (2009). Widespread increase of tree mortality rates in the western United States. Science 323, 521–524. doi: 10.1126/science.1165000
Walker, B., Carpenter, S., Anderies, J., Abel, N., Cumming, G., Janssen, M., et al. (2002). Resilience management in social-ecological systems: a working hypothesis for a participatory approach. Conserv. Ecol. 6:14. doi: 10.5751/ES-00356-060114
Weber, A., Kimmins, J., P., Gilbert, B., Y.-H., Lo and Blanco, J., A. (2014). Multiple-pathway succession in coastal Tsuga heterophylla, Thuja plicata, and Abies amabilis forests on northeastern Vancouver Island, British Columbia. Can. J. Forest Res. 44, 1145–1155. doi: 10.1139/cjfr-2014-0060
White, P. S., and Walker, J. L. (1997). Approximating natures variation: selecting and using reference information in restoration ecology. Restor. Ecol. 5, 338–349. doi: 10.1046/j.1526-100X.1997.00547.x
Wilcove, D. S. (2008). No Way Home: The Decline of the Worlds Great Animal Migrations. Washington, DC: Island Press.
Williams, P. A., Allen, C. D., Macalady, A. K., Griffin, D., Woodhouse, C. A., Meko, D. M., et al. (2013). Temperature as a potent driver of regional forest drought stress and tree mortality. Nat. Clim. Change 3, 292–297 doi: 10.1038/nclimate1693
Yocom, L. (2013). “Working paper 27: Fuel treatment longevity,” in ERI Working Papers. Ecological Restoration Institute (Flagstaff, AZ: Northern Arizona University).
Keywords: dispersal, disturbance, ecosystem management, persistence, recovery, reorganization, tipping points, wildland fire
Citation: Falk DA, Watts AC and Thode AE (2019) Scaling Ecological Resilience. Front. Ecol. Evol. 7:275. doi: 10.3389/fevo.2019.00275
Received: 10 December 2018; Accepted: 02 July 2019;
Published: 24 July 2019.
Edited by:
Brenda C. McComb, Oregon State University, United StatesReviewed by:
John Morgan, La Trobe University, AustraliaLuis Zambrano, National Autonomous University of Mexico, Mexico
Carolyn Fonyo, Oregon State University, United States
Copyright © 2019 Falk, Watts and Thode. This is an open-access article distributed under the terms of the Creative Commons Attribution License (CC BY). The use, distribution or reproduction in other forums is permitted, provided the original author(s) and the copyright owner(s) are credited and that the original publication in this journal is cited, in accordance with accepted academic practice. No use, distribution or reproduction is permitted which does not comply with these terms.
*Correspondence: Donald A. Falk, ZGFmYWxrQGVtYWlsLmFyaXpvbmEuZWR1