- 1College of the Environment and Life Sciences, University of Rhode Island, Kingston, RI, United States
- 2Biological Sciences/Environmental Studies, Central Washington University, Ellensburg, WA, United States
- 3Advanced Science Research Center at the Graduate Center, City University of New York, New York, NY, United States
Non-perennial streams dominate the extent of stream networks worldwide. Intermittent streams can provide ecosystem services to the entire network—including nitrate uptake to alleviate eutrophication of coastal waters—and are threatened by lack of legal protection. We examined 12 intermittent streams in the temperate, humid climate of the Northeast USA. Over 3 years of monitoring, continuous flow was observed a median of 277 d yr−1, with no-flow conditions from early summer into fall. Estimated median discharge was 2.9 L s−1 or 0.36 mm d−1. All intermittent streams originated from source wetlands (median area: 0.27 ha) and the median length of the intermittent stream from the source wetland to the downstream perennial stream was 344 m. Through regional geospatial analysis with high resolution orthophotography, we estimated that widely available, “high resolution” (1:24,000) hydrography databases (e.g., NHDPlus HR) only displayed 43% of the total number of intermittent streams. Whole-stream gross nitrate-N uptake rates were estimated at six intermittent streams during continuous flow conditions using pulse additions of nitrate and a conservative tracer. These rates displayed high temporal variability (range: no detect to over 6,000 mg N m−1 d−1); hot moments were noted in nine of the 65 pulse additions. Whole-stream gross nitrate-N uptake rates were significantly inversely related to discharge, with no measurable rates above 7 L s−1. Temperature was significantly positively correlated with whole-stream gross nitrate-N uptake rates, with more hot moments in the spring. Microbial assays demonstrated that nitrate cycling in intermittent streams are consistent with results from low order, perennial forested streams and highlighted the importance of debris dams and pools—potential locations for transient storage. Our assessment suggests that intermittent streams in our region may annually contribute 24–47% of the flow to perennial streams and potentially remove 4.1 to 80.4 kg nitrate-N km−2 annually. If development in these areas continues, perennial streams are in danger of losing a portion of their headwaters and potential nitrate uptake areas may become nitrate sources to downstream areas. These results argue to manage fluvial systems with a holistic approach that couples intermittent and perennial components.
Introduction
Non-perennial streams contribute over half of the discharge in the global river network (McDonagh et al., 2011; Datry et al., 2014; Costigan et al., 2016; Skoulikidis et al., 2017). However, they are often neglected in water legislation worldwide (Fritz et al., 2017). In the European Union, the Water Framework Directive (European Commission, 2000) incorporates hydrological and ecological criteria for stream protection, but most member states have not defined intermittent waterways (Fritz et al., 2017). In 2015 in the United States, a draft Clean Water Rule (U.S. Environmental Protection Agency, 2015) proposed to extend protection to non-perennial streams, but the rule was suspended (Fritz et al., 2017). Non-perennial streams are at risk of being buried, channelized or degraded by land-use changes associated with urbanization, mining, agriculture and other human activities (Acuña et al., 2014).
Many non-perennial streams are in the headwaters. Their protection is critical to the integrity of river networks and provides important ecosystem services to downstream users (Acuña et al., 2014; Leigh et al., 2015). The scientific community has identified these non-perennial streams as essential habitat and refugia for a high diversity of aquatic organisms as well as critical to the flux of water, sediment, carbon, nutrients, and organisms to higher order perennial streams (Freeman et al., 2007; Meyer et al., 2007; Elmore and Kaushal, 2008; Wohl, 2017). However, the value of these non-perennial waterways for protection has been undermined by the historical gap in identifying the extent and connectivity of these intermittent and ephemeral streams on maps of common scale (i.e., 1:24,000; Leibowitz et al., 2008; Benstead and Leigh, 2012; Datry et al., 2014; Ryan, 2017; Wohl, 2017). With the advancement of improved mapping, modeling, and field work in recent years, the extent and functional importance of intermittent streams (Benstead and Leigh, 2012; Fritz et al., 2013; Leigh et al., 2015; Nadeau et al., 2015; Williamson et al., 2015) and their connections to wetlands (Tiner, 2003; Marton et al., 2015; Lane and D'Amico, 2016; Rains et al., 2016) can be refined.
In this study, we continue to explore the value of non-perennial streams. We examine intermittent streams (streams that cease to flow seasonally or occasionally for weeks to months) in the temperate, humid climate of the glaciated Northeast of the United States—a region likely to have a high density of intermittent streams that have been not been examined (Larned et al., 2010; Datry et al., 2014, 2017; von Schiller et al., 2017). We gathered information on hydroperiod (i.e., the extent of time that the streams had flowing water precluding the formation of fragmented pools; Boulton, 2003), stream structure and source wetland characteristics on 12 intermittent streams. Flow duration has been difficult to determine historically (Hanson, 2001; Biggs et al., 2017); our extensive monitoring contributes to the understanding of flow dynamics in intermittent streams in temperate, humid climates. We also expand our results with a mapping exercise to assess the density of intermittent streams in this region. We expect that the hydroperiod of these intermittent streams have been underestimated. In addition, if the density of intermittent streams have been underestimated, many wetlands may be found to discharge directly to stream channels and thus provide more rapid connections to fluvial networks than previously estimated (Rains et al., 2016). Many regulations and policies for wetland protection have been linked to whether they are “geographically isolated wetlands” or directly connected to stream channels or navigable waters (Leibowitz et al., 2008; Rains et al., 2016), lending considerable importance to this question. In the humid climate of the Northeast USA, these geographically isolated wetlands are connected hydrologically to stream networks via groundwater.
Nitrate-Nitrogen () has been examined within intermittent streams over the last decade, often focused on sites in Mediterranean climates (von Schiller et al., 2017). Many studies have provided insight into the processes associated with uptake and release that can vary during the shifting mosaic of wet-dry-rewetting (Schade et al., 2005; von Schiller et al., 2011, 2017; Bernal and Sabater, 2012), but information is lacking on nitrate uptake during continuous flow. We considered different approaches (in-situ and microcosm) to explore different aspects of N cycling at different scales. We focused on the time of continuous flow to examine environmental factors driving whole-reach gross uptake. We hypothesize that whole-reach gross uptake will be mostly associated with the presence of pools and debris dams with the presence of both organic matter and longer residence times. We expect that whole-stream gross uptake rates will increase with lower levels of water in the channel (e.g., lower depth, velocity, and discharge—Q). Precipitation events can contribute to higher water levels and may also stimulate changes in the configuration in the stream. This research will help improve the scientific understanding of the timing, contribution of flow and whole-reach gross uptake of intermittent streams within larger stream networks. Our methods of exploring hydrological connectivity can contribute to the local and state-level conversations among land managers deciding on how to identify and protect intermittent streams, regardless of the Federal policies in place at the time.
Materials and Methods
Sites
We selected 12 intermittent streams—all located in forested settings in Washington and Kent Counties in Rhode Island, USA (Figure 1). We identified potential unmapped stream locations with the following geospatial attributes: (1) depicted as sets of tight, uphill pointing crenulations on 1:24,000 scale USGS contour maps but with no identified stream lines, (2) located on forested, public land without recent disturbance, and (3) situated upstream from all culverts and road crossings. Once we field verified the presence of these streams, we randomly selected the streams to include in the study. All sites were located on glacial till with slopes > 3%. We established a 30-m study reach (Frissell et al., 1986) within each stream that was representative of the stream features found between the source (which was consistently found to be a hillslope wetland seep) and the downstream perennial stream. A fixed staff gauge was installed in the channel at each study reach, and we recorded the stage above the thalweg during weekly or biweekly visits.
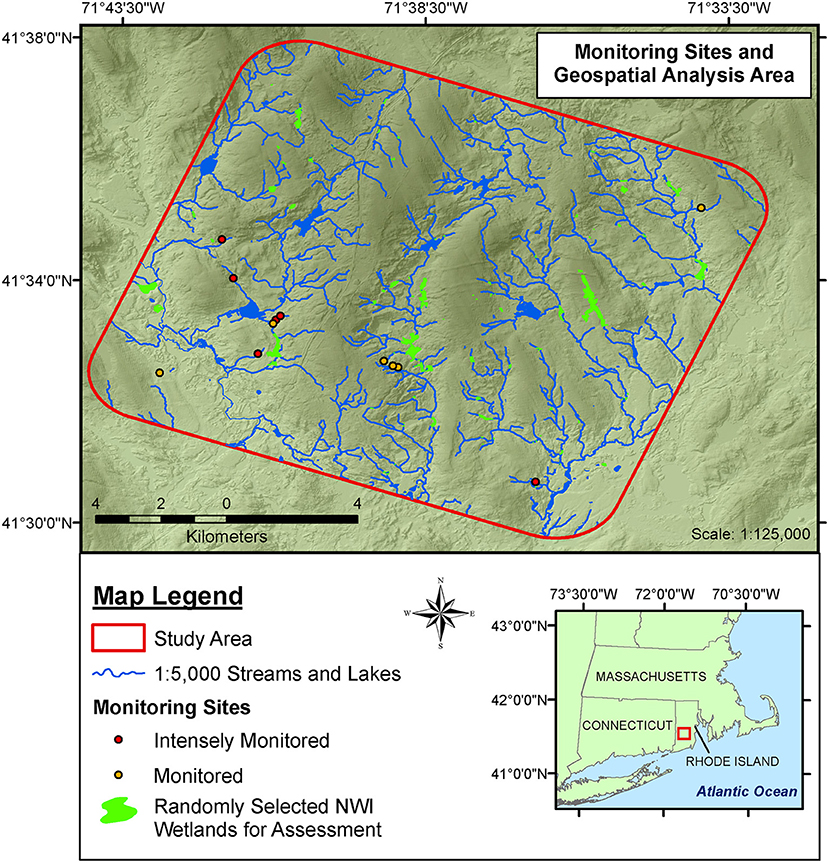
Figure 1. Intermittent stream study sites and the National Wetland Inventory (NWI) wetlands examined for hydrological connections in geospatial analysis. All 12 intermittent stream locations were monitored to determine hydroperiods and wetland attributes. Six of those intermittent streams were used for assessment of whole-reach gross nitrate-N uptake during baseflow conditions, transient storage modeling, and microbial assessment.
Hydroperiod and Wetland Attributes
We created waypoints on a Garmin hand held GPS (model GPSMAP® 64s) as we walked upstream from the perennial stream through the intermittent stream to the source wetland that according to USGS 1:24,000 scale maps did not connect to a stream channel outlet. Also, we mapped the outer margins of the wetland by walking around the wetland with the GPS. The data were converted into shapefiles manually via heads up digitizing, generating polygons for wetlands and lines for streams, using ArcGIS Version 10.3.1 (Environmental Systems Research Institute ESRI, 2011). Within ArcGIS, we calculated the length of stream reach hydrologically connecting the source wetland to the downstream perennial stream, area of the source wetland, and the watershed area of the study sites.
We defined the hydroperiod (i.e., the extent of time when the channel had flowing water) of the intermittent streams using two monitoring devices: (1) stacked Thermochron iButtons (model DS1921G, Embedded Data Systems, Lawrenceburg, KY) and (2) High frequency Odyssey Capacitance Water Level Probes (Dataflow Systems; Christchurch, New Zealand).
Themochron iButtons
In 2009 we installed two Thermochron iButtons in compartments at 2 and 32 cm above the stream bed within a vertical PVC pipe housed within a secondary slotted PVC pipe. These sensors were located within the thalweg at the midpoint of the study reach. These iButtons are widely used to measure, record and store temperature data from 1 to 255 min intervals. We collected temperature data six times per day at these two depths in the stream channels. The iButton installed at 32-cm measured ambient air temperature. The temperature readings from the 2-cm stage iButton served to indicate presence or absence of water based on comparison with the air temperature. When the two temperatures diverged by > ±1°C (level of iButton accuracy), we assumed that water presence was indicated. Based on our weekly or bi-weekly field observations, temperature divergence at the 2-cm depth generally indicated water flow in the stream channel; however, in some cases it could have indicated a stagnant pool in which case the hydroperiods may have been slightly overestimated.
In the late fall and winter, the temperature of flowing water was expected to be warmer than the air temperature at 32-cm iButton. In the late spring and summer, the temperature of flowing water was expected to be cooler than the 32-cm iButton. When the 2-cm and 32-cm iButtons were within 1°C of each other, the air and water temperature were similar likely indicating no water in the channel at 2 cm. However, in late spring and early fall, water and air temperatures are often very similar in our area during part of the day (Example in Supplement 1). To confirm presence or absence of flow, we examined the weekly or biweekly field observations of stage and/or the daily rainfall data (weather data from Kingston, RI; within 15 km from all sites; U.S. Climate Data, 2018). After large rain storms, we visited the sites and found that the stream stage rarely rose above 32 cm.
Odyssey Capacitance Water Level Probe
The probes were calibrated to the nearest millimeter and programmed to record depth daily. To obtain insight into the flow regime via stage-discharge relationships at a subset of six sites, we began to replace the iButtons with water level probes in mid-2010. Continuous stage was not recorded at all six sites at the same time periods due to equipment shortages. From January 10, 2012 to January 9, 2013, four sites shared the same daily one-year data logger collection period. We determined the Q and the normalized Q (i.e., Q divided by watershed area). We assumed that the excess precipitation (annual precipitation minus evapotranspiration) per unit area was roughly equivalent across the study area, so the normalized Q was used to determine if there were differences between the groundwater recharge area to the wetlands vs. the watershed area derived from surficial topography. We used graphical methods to separate baseflow from storm flow (Viessman and Lewis, 2003) at these four sites. We excluded days when there was no flow in our analysis as the goal was to describe flow conditions.
We examined stream bank vegetation (Summer 2010) and riparian soils (Spring 2016) at the intermittent streams to determine if they contained wetland indicators that would meet the regulatory classification of wetlands, an important factor in many resource management decisions. We conducted vegetation surveys along the right and left banks along each study reach—assessing four zones extending out 6 m orthogonally from the defined stream channel. We identified the species of all plants in the overstory, understory, and ground cover and estimated their abundance to determine the dominant species. We used the updated National Wetland Plant List (standard reference for hydrophytic indicator rating of vascular plants in the USA; Lichvar et al., 2016) to determine the wetland indicator status (i.e., the likelihood the plant would be in a wetland) of each dominant plant. We determined the wetland indication for all sites based on the U.S. Army Corps of Engineers (2012) manual for the Northeastern USA Sites indicated wetland status if they passed the rapid test for hydrophytic vegetation (all dominant species are rated obligate or facultative wetland or a combination of both) or the dominance test (more than 50 percent of the dominant plant species are rated obligate, facultative wetland or facultative).
We created three transects perpendicular to the stream on both banks of the stream channel—up to 18 m from the channel—to examine the extent of hydric soils along each stream. Hydric soils are important indicators of wetland conditions as they are formed under conditions of saturation long enough during the growing season to develop anaerobic conditions in the upper portion of the profile. Along the transects, soil augers were used to identify the presence/absence and extent of hydric soil indicator A11 (U. S. Department of Agriculture, 2010), i.e., presence of a soil layer with a depleted or gleyed matrix starting at a depth ≤ 30 cm from the soil surface.
We also recorded additional information from the study intermittent streams to compare them to perennial streams. Stream sinuosity (ratio of channel length to valley length) and stream bed particle size (Wolman, 1954) were documented at each site. In every stream reach, we surveyed the stream bed at 1-m intervals noting geomorphic features present, i.e., pool, debris dam, run or riffle.
Transient Storage
We used the OTIS-P (One-Dimensional Transport with Inflow and Storage with automated parameter estimation; Runkel, 1998) to model hydraulic transport parameters in five of our intermittent streams (these five are part of the subset of six sites used to explore dynamics below). The field data were derived from constant-rate injections of Br− (Kilpatrick and Cobb, 1985) during Spring 2013. We collected stream water in a carboy, amended it with bromide (Br−), and pumped it into the stream over the course of an hour at a steady rate of 130 ml h−1 with a MasterFlex L/S portable peristaltic pump (Cole Parmer, Vernon Hills, IL). Water samples were collected every minute at multiple stations up to 30 m downgradient of the injection and stored at 4°C until analysis. We calculated the degree of entrainment (AsA−1; transient storage area divided by channel area; Harvey and Wagner, 2000) and F which represents how much transient storage affects the travel time over a standardized 200 m reach (Runkel, 2002). We chose to calculate these parameters to compare to other streams as they are widely reported in the literature.
Geospatial Analysis of Wetland Connections to Intermittent Streams
We examined the extent of unmapped intermittent streams that may connect wetlands via channelized surface runoff to perennial streams in our area. We used ArcGIS to select a contiguous area of 150 km2 that serves as the headwaters for several river systems in Rhode Island and contained the 12 study sites. Within this area, we only included hilly, forested moraine landscapes that mirror the settings of the study sites. The final analysis zone was 117 km2.
We located 443 NWI palustrine wetlands within the study zone with the National Wetland Inventory (NWI) dataset (RIGIS, 2014, 1:24,000). We randomly selected 100 of these wetlands to determine the type of hydrological connection (channelized surface runoff vs. groundwater flow) of these wetlands to streams via two coverages: (1) National Hydrography Dataset High Resolution (NHDPlus HR) stream coverage (1:24,000; USGS, 2018) representing the highest resolution stream data available across the entire Northeast US and (2) the 2011 RIDEM Digital True Color Orthophotography (RI DEM 2011, resolution of 0.15 m spatial resolution or 1:300 scale). We considered the 0.15 m spatial resolution Color Orthophotography to represent “true” conditions. Wetlands within 17 m (digital tolerance) of NHDPlus HR streams were considered “connected” to surface stream channels based on this 1:24,000 coverage. To aid the interpretation of the orthophotography, two people both reviewed a set of 15 NWI wetlands to ensure they both agreed if there were a visually apparent stream leaving the wetland. The interpreter was conservative in the assessment; only clearly visible surface stream channels were considered “connected.” If NHDPlus HR coverage indicated that the wetland was not connected to a NHDPlus HR stream but the orthophotography depicted a stream outlet, we assumed that an intermittent stream, like our study sites, hydrologically connected the wetland via channel flow to the perennial stream. Within ArcGIS, we measured the straight distance from the NWI wetland to the origin of the nearest perennial stream (depicted on the NHDPlus HR coverage) as an approximation of the extent of the intermittent stream reach. We then applied a 1.1 sinuosity factor to the length estimate based on the sinuosity of the 12 intermittent streams to estimate stream lengths for this study.
Whole-Reach Gross Nitrate-N Uptake and Retention
We used a subset of six intermittent streams for assessment of whole-reach gross uptake rates during baseflow conditions from 2010 through 2012. To examine the whole-reach gross uptake of these intermittent streams, we amended the stream with and a conservative tracer, Br−, and examined concentrations downstream. We completed a total of 65 pulse additions (a.k.a., slug-injection; Kilpatrick and Cobb, 1985) on the six intermittent streams over 3 years. There were at least three replicate pulse additions in the spring, fall, and winter seasons on each stream. We tried several pulse additions during summer low flow conditions, but we were unable in a reasonable field day to recover a substantial portion of the pulse injections, so we discontinued summer additions.
Ten liters of stream water were enriched with and Br− in a carboy and poured into the stream quickly. We collected water samples 30 m downstream at one-min intervals until we expected the plume to have passed by, usually within 3 h. We targeted the pulse additions to raise stream concentrations by 10 mg Br− L−1 and 1–2 mg L−1; we used more Br− as the analytical resolution was lower compared to . All water samples collected were stored on ice in the field, filtered upon return to the laboratory, and stored at 4°C until analysis.
Using the time-concentration data of the Br− conservative tracer from the pulse additions, we calculated Q and mean velocity per Kilpatrick and Cobb (1985). Deviation from the known starting tracer : Br− ratio in downstream samples allowed us to calculate the whole-reach gross uptake as mass of lost per meter of stream per day (Williams et al., 2015). The data represent “potential” rates as levels in the stream during the pulse additions were elevated rather than at ambient stream concentrations.
Microbial Assays
We used laboratory assays to obtain insights into denitrification potential and other microbial processes of different types of stream substrates (debris dam material—dominated by leaves mixed with some decomposed organic material, pool sediments, and riffle/run sediments). The denitrification enzyme activity assay (DEA) is designed to assay the maximum potential activity of denitrification enzymes present in the soil at the time of sampling. Although the production of these enzymes has been shown to be inducible by the onset of anaerobic conditions, with rapid destruction following the onset of aerobic conditions in the laboratory, they have been shown to persist for long periods under field conditions (Smith and Tiedje, 1979; Groffman, 1987; Martin et al., 1988). As a result, DEA has been found in multiple studies to vary much less in time and space than actual denitrification activity, make it a useful index of variation in the potential for denitrification in different places (Groffman and Tiedje, 1989; Groffman et al., 2006).
In the Fall of 2011, we collected stream material to assess DEA, potential net mineralization, potential net nitrification, and respiration from the six subset streams where we conducted pulse additions. Samples were collected into zip-lock bags, transported to the lab on ice, and stored at 4°C until assays commenced within 2 weeks. Following Groffman et al. (2005), we created a slurry of the debris dam materials by using a blender to shred the leaf material within added stream water for use in the assays. As described by Groffman et al. (1999, 2006), DEA samples were amended with , dextrose (as a carbon source), chloramphenicol (to limit protein synthesis), and acetylene (to halt denitrification process at N2O), incubated under anaerobic conditions for 90 min. Gas samples were removed from incubation flasks at 30 and 90 min and analyzed for nitrous oxide (N2O).
Rates of potential net N mineralization, nitrification and microbial respiration were measured in 10-d incubations at 25 °C (Groffman et al., 2006). Inorganic nitrogen ions (ammonium, , and ) were extracted with 2 M KCl at the beginning and end of the incubation and stored at 4 °C until analysis. The headspace was also sampled at the end of the incubation. Rates of potential net N mineralization were calculated from the accumulation of plus N. Rates of net nitrification were calculated from the accumulation of alone during the incubation. Rates of microbial respiration were calculated from the accumulation of carbon dioxide (CO2) during the incubation.
Analytical Analyses
Stream samples were analyzed for with the open tubular cadmium reduction method (4500-NO3−; Eaton et al., 1998) and for Br− with the phenol red colorimetric method (4500-Br−; Eaton et al., 1998) on an Astoria Pacific Model 303A Segmented Continuous Flow Autoanalyzer (Astoria-Pacific Inc.). Soil extracts were also analyzed for with the same method as stream samples. Soil extracts were analyzed for with the automated phenate method (4500-NH3; Eaton et al., 1998) on the Astoria Pacific Model 303A. We analyzed for dissolved organic carbon (DOC) on a Shimadzu Total Organic Carbon Analyzer. Gas samples were analyzed for N2O with an electron capture detector and for CO2 with a flame ionization detector on a gas chromatograph (GC-2014; Shimadzu Scientific Instruments).
Statistical Analyses
As the data were not normally distributed, the whole-reach gross uptake rates were compared by Kruskal Wallis ANOVA by ranks for seasons and sites. Pairwise Mann-Whitney U-Tests were used to tease out significant differences indicated by the Kruskal Wallis ANOVA. We used Spearman Rank Correlation statistics to examine relationships between whole-reach gross uptake rates and the following metrics: Q and velocity derived from the pulse addition data, depth and stream temperature measured before the pulse addition, DOC concentration from samples collected before the pulse addition. Spearman Rank Correlation statistics also examined the relationship between whole-reach gross uptake rates and the most recent rain event total before the pulse additions (weather data from Kingston, RI; within 15 km from all sites; U.S. Climate Data, 2018). Statistical significance was set at α < 0.05 for all analyses. There are varied approaches in the literature on how to define hot moments” (Bernhardt et al., 2017). We defined a hot moment as a mild outlier (e.g., values above Upper quartile + (1.5 x Interquartile range; Molodovskaya et al., 2012). These statistical analyses were performed with Statistica Academic (TIBCO Software 2017).
Results
Stream Attributes & Hydroperiod
Over a 3-year period, all 12 field-identified stream channels were confirmed to be intermittent streams. Based on continuous monitoring of stage from 2010 to 2012, flow generally ceased in all streams for periods in summer; only one stream maintained continuous flow throughout the year in 2012. The intermittent streams all originated at field-verified, hillslope wetlands (Table 1) where groundwater seeped onto the surface and created a surface water channel. Based on surface topography the median watershed area of the intermittent stream study reaches was 61 ha (IQR: 35–69), but we recognize that the surface topography may not capture groundwater flow in hummocky terrains. The source wetlands of our monitored streams were relatively small in area (median: 0.27 ha; IQR 0.12–0.69). These source wetlands were comparable to the source wetland areas in the geospatial analysis (median: 0.19 ha; IQR: 0.08–0.47; Table 1). The intermittent stream length from the source wetland to the downstream perennial stream at the field sites (median 344 m; IQR: 263–469) were comparable to similar intermittent streams identified using geospatial analysis (median: 317 m; IQR: 201–648; Table 1).
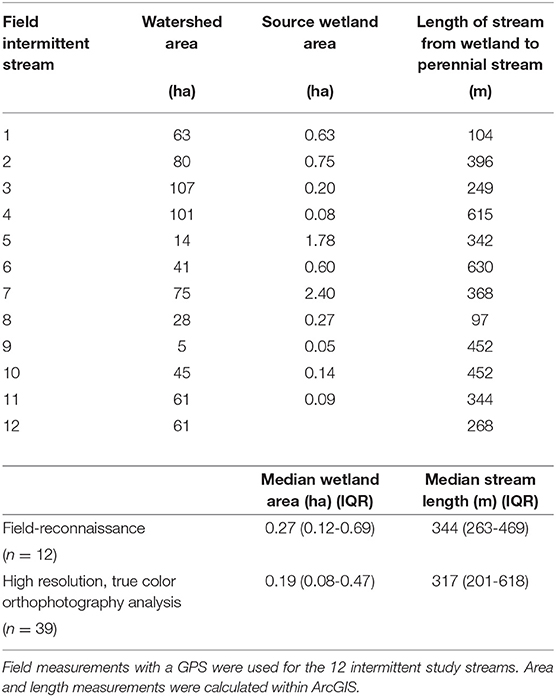
Table 1. Intermittent stream watershed area, source wetland area, and the length of intermittent stream from its source wetland to the perennial stream.
The hydroperiod of the intermittent streams showed a clear seasonal pattern and varied among sites. For much of the year, the intermittent streams hydrologically connected the upstream source wetlands to downstream perennial streams. All sites flowed throughout winters when not frozen. Continuous flow of these streams began by day 60 of the Julian calendar. Continuous flow generally ceased in the summer (median Julian date: 184, IQR: 174–201; n = 25; number of streams with data varied over the 3 years of data) and resumed in the fall (median Julian date: 272; IQR: 248–309; n = 27) (Figure 2A). The stream channels began to contract in late spring and summer until flow fragmented into standing water in isolated pools (Figure 2B). Once continuous flow ceased, these intermittent streams no longer had visible surface hydrologic connectivity of the headwater wetlands to downstream perennial waters. This period of disconnected flow lasted for a median of 88 days (IQR: 74–108; n: 23). During the intermittent period, after rainfall we noted periodic, patchy flow for a few hours to a few days due to runoff gathering into the intermittent stream channels or brief elevation of the water table. As with the initial drawdown, this brief intermittent flow was often followed by shallow standing pools for a few hours to days until the stream bed dried.
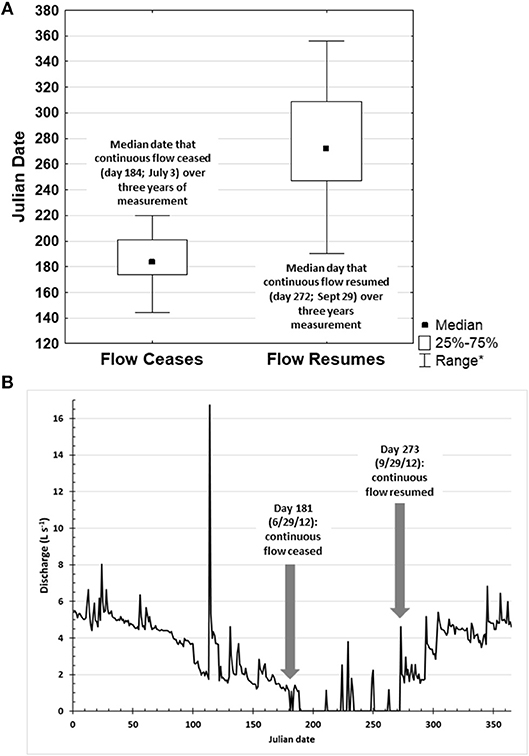
Figure 2. Hydroperiods of intermittent streams. (A) Julian dates when continuous flow ceased and resumed in 12 intermittent streams in the Northeast USA over 3 years. (B) Example of the discharge (L s−1) over Julian dates in 2012 derived from stage data at Site 4 and the site-specific stage-discharge relationship. Arrows indicate when continuous flow ceases (Julian date 181) and resumes (Julian date 273). *The range excludes outliers (point which falls more than 1.5 times the interquartile range above the third quartile or below the first quartile) and extremes (point which falls more than 3.0 times the interquartile range above the third quartile or below the first quartile).
When intermittent streams were flowing, the median Q at the four sites with common logger data was 2.9 L s−1 (IQR: 1.7–4.0). When flow was normalized for watershed area, median Q was 0.36 mm d−1 (IQR: 0.19–0.56; Figure 3).
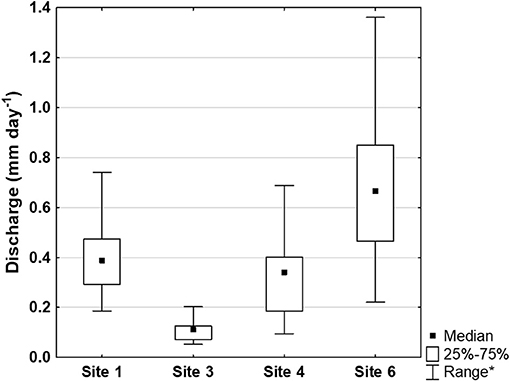
Figure 3. Normalized discharge (mm d-1) from January 10, 2012 to January 9, 2013 at Sites 1, 3, 4, and 6, based on stage-discharge curves developed during the pulse additions and stage collected with dataloggers. *The range excludes outliers (point which falls more than 1.5 times the interquartile range above the third quartile or below the first quartile) and extremes (point which falls more than 3.0 times the interquartile range above the third quartile or below the first quartile).
Eleven of the 12 sites had hydrophytic vegetation that indicate wetland conditions extending beyond 2 m of the stream channel on at least one bank. All riparian zones were found to have hydric soils. The channel structure of these intermittent streams resembled perennial, hillslope headwater streams throughout the glaciated Northeast USA with a mix of riffles, runs, and pools (Leopold, 1994). Tree roots, large woody debris and small woody debris dams primarily controlled the structure of pools and likely helped to retain organic matter in the system (Bilby and Likens, 1980). Mean sinuosity of all sites was 1.1, indicating few meanders. Stream sediments were generally composed of medium gravel to small cobbles. From the OTIS -P analysis of five intermittent streams, As:A had a median of 0.19 (IQR: 0.06–0.2 and F was 16.2% (IQR: 5.5-17.3%).
Geospatial Analysis of Connectivity
Within a 117 km2 area of hilly, forested terrain, we assessed the classification of 100 of the 443 palustrine wetlands (Table 2) identified in the NWI (RIGIS, 2014 1:24,000). Using the NHDPlus HR data (and verified with the orthophotography), 29/100 wetlands were found to be directly connected to stream channels (Table 2)—which we assume were perennial streams. This yields a density of 1.1 wetlands connected to stream outlets km−2 over the entire 117 km2. However, we found that 39/100 of NWI wetlands classified as groundwater discharge wetlands (Table 2) in the NHDPlus HR data actually showed clear stream connections in the orthophotos. While we don't know if all of these streams are intermittent (rather than perennial), the fine scale of these stream features is comparable to the 12 intermittent streams we assessed in this study (Table 1). For purposes of evaluating the role of these small streams at the watershed scale, we assumed that streams that show connections to wetland outlets only at very high resolutions have intermittent flow regimes, yielding a density of an additional 1.5 wetlands connected to stream outlets km−2. Another 29 of the NWI wetlands evaluated had no apparent streams in neither NHDPlus HR nor orthophotography—we suggest that these are groundwater discharge wetlands.
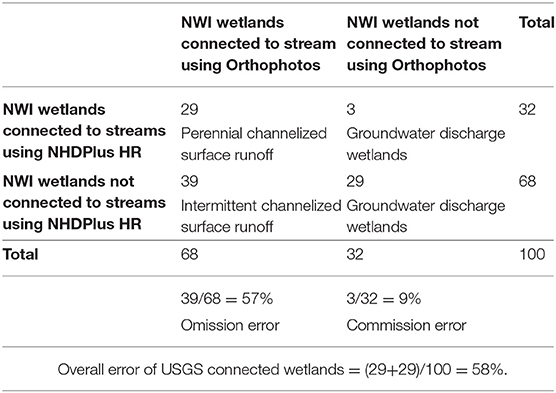
Table 2. Matrix of hydrologic connections of NWI wetlands to streams using 1:300 orthophotography and NHDPlus high resolution (HR) 1:24,000 stream coverage.
Whole-Reach Gross Nitrate-N Uptake and Retention
Ambient concentration ranged from no detect (< 0.02 mg N L−1) to 0.04 mg N L−1. We only calculated whole-reach gross nitrate-N uptake from slug tests when the complete breakthrough curve of Br− concentrations was obtained 30 m downstream from the injection. Across these 65 slug tests, the time to the peak concentration at the 30 m station had a median of 15 min (IQR: 10–27 min; Table 3). The tail end of the breakthrough curve had a longer duration allowing ample time for N processing; the median transit time for the entire Br- slug over the 30 m stream reach was 107 min (Table 3).
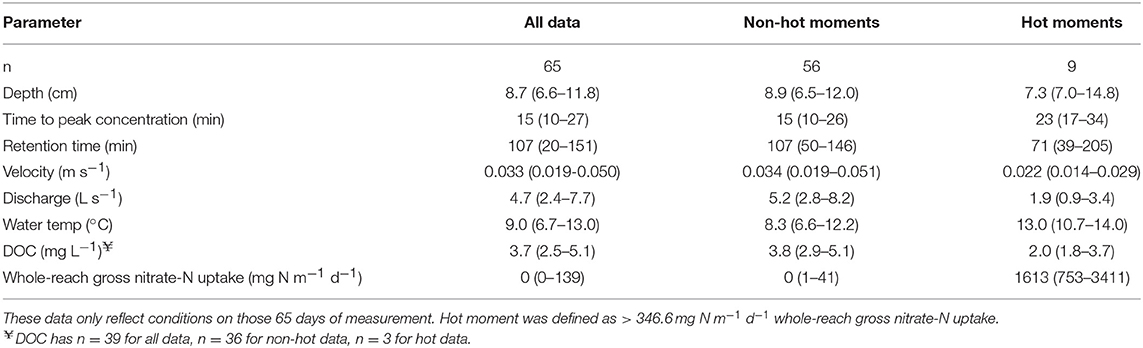
Table 3. Flow characteristics [Median, (interquartile range)] during slug testing to estimate whole-reach gross nitrate-N uptake across six intermittent streams over three years.
The whole-reach gross uptake rates displayed high temporal variability; detectable rates were noted in 37% of pulse experiments with occasional “hot moments” when rates were statistically much higher (Figure 4; McClain et al., 2003). During these 65 slug tests we reported the flow conditions, temperature, DOC, and whole-reach gross uptake rates for the overall dataset and sub-databases based on hot moment and non-hot moment conditions (Table 3). Whole-reach gross uptake rates across all sites and seasons ranged from 0 to 6114 mg N m−1 d−1 (median: 0; third quartile: 138.6; hot moment > 346.6 mg N m−1 d−1, n: 65). Measurable whole-reach gross uptake was noted in five out of six intermittent streams that were subjected to repeated pulse additions in multiple seasons. There were no significant differences between sites (Kruskal-Wallis ANOVA, p > 0.05). In each of the five sites with measurable whole-reach gross uptake, there were a total of 1-3 hot moments. No significant differences in the distribution of whole-reach gross uptake was observed between seasons (Kruskal-Wallis ANOVA). Across all five sites (n = 65), there were nine hot moments – two in the fall and seven in the spring (Figure 4).
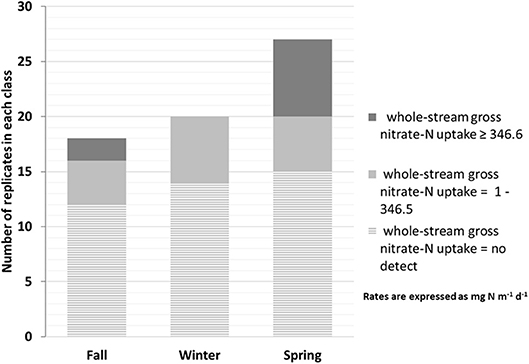
Figure 4. Distribution of whole-reach gross nitrate-N uptake rate measurements by season organized by whole-reach gross uptake rate = 0, rate = 1 – 346.5 mg N m−1 d−1, and rate ≥ 346.6 mg N m−1 d−1, above this rate is considered a “hot moment”) in streams 1–5 from pulse addition experiments. We did not observe measurable whole-reach gross uptake rates at site 6.
Median water temperature across spring pulse addition replicates was 13.7°C, whereas the fall median was 7.4°C. Whole-reach gross uptake rates were significantly positively correlated (Spearman Rank) with water temperature (n: 65, rs: 0.28, p: 00231). Whole-reach gross uptake rates were significantly inversely correlated (Spearman Rank) with depth (n: 65, rs: −0.31, p: 0.0124), Q (n: 65, rs: −0.57, p: 0.000001), and velocity (n: 65, rs: −0.37, p: 0.0026). We considered if the periods immediately after dry phases may be more biogeochemically active as found by Leigh et al. (2015). We examined the wet-dry periods before the fall pulse additions as the streams began to rewet and flow. However, the fall addition experiments were often 26 to 124 days after the stream channel had rewetted. Significant correlations (Spearman Rank, n = 15) did not emerge between whole-reach gross uptake and the number of days since rewetting. While we are not able to directly correlate loss of debris dams and pools to rainfall events, we observed that whole-reach gross uptake rates were inversely related to the rainfall event total proceeding the pulse addition (Spearman Rank; n: 65, rs: −0.25, p: 0.0417). The median ambient DOC concentration across the six sites was 3.7 mg C L−1 (IQR: 2.5–5.1). We found no significant correlation between whole-reach gross uptake and DOC concentration (Spearman Rank, n = 39). Parsing out the parameters into non-hot moments and hot moments (Table 3), echoes the trends in the correlations. Hot moment slug tests generally had shallower stream depth, lower velocity, lower Q, and warmer water temperatures.
N Cycling in Stream Substrates
Denitrification potential differed with stream substrates (Table 4). In the intermittent streams, pools and riffles had higher mean denitrification potential compared to debris dams (an order of magnitude higher). The denitrification potential, potential net mineralization and nitrification was higher in the pools and riffles compared to the debris dams (Table 4). We found microbial respiration was substantially higher in debris dams of the intermittent streams compared to the other features (Table 4).
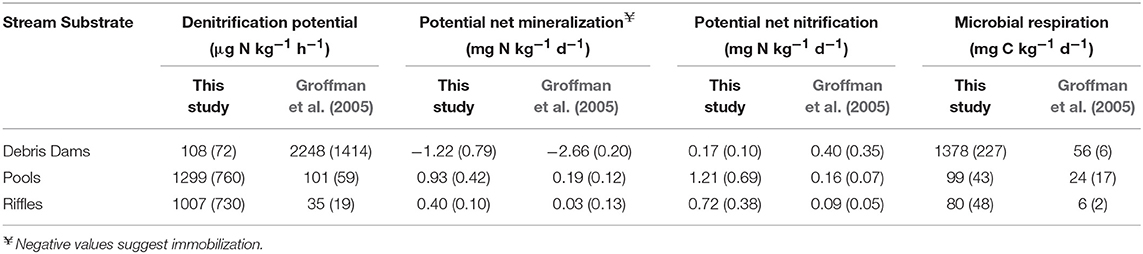
Table 4. Mean rates (SE) from incubations of different stream substrates (n = 6 for intermittent streams; n = 3 for Groffman et al., 2005).
Discussion
Hydroperiod, Wetland Attributes & Transient Storage
Both the hydrophytic vegetation and hydric soil surveys indicate that the intermittent stream channels and their riparian areas have wetland characteristics. The similarity in the geospatial characteristics of the study intermittent streams and their source wetlands with those analyzed in a larger region (Table 1) suggests that these sites were representative of other intermittent streams in the region. The progression of wet-dry-rewetting stages observed in the intermittent streams (Figure 2) echoes the description in the review summary by Datry et al. (2017).
The variability in normalized Q (Figure 3) indicates differences between the groundwater and surface water recharge areas which is common in heterogeneous media that can result from glacial drift sediments in the study area (Winter et al., 2003). This complex pattern of media may create complicated flowpaths that impact the hydroperiod, transient storage, and whole-reach gross uptake. Stormflow contributed between 3 and 26% of the annual flow at the four sites where we completed baseflow separation. These values are typical for forested watersheds dominated by groundwater flow with very little overland runoff as observed in Chambers et al. (2017) in the watershed of Cork Brook, RI, USA – a forested, first order stream where stormflow contributed 8% of the annual flow.
Transient storage values obtained from the OTIS-P model in five of the intermittent streams were comparable to or higher than other perennial streams cited in the literature (Valett et al., 1996, 1997; Haggard et al., 2001; Thomas et al., 2003; Lautz and Siegel, 2007; Dodds et al., 2008; Duff et al., 2008; Pennino et al., 2014; Arango et al., 2015). The intermittent stream As:A IQR was comparable to that in the perennial streams in the literature (IQR: 0.05-0.33). The intermittent stream F was higher than the perennial stream studies with a median of 3.1% (IQR: 0.8–16.8). The data indicate that intermittent streams may have similar or more transient storage relative to a wide assortment of perennial streams which could lead to higher retention.
Geospatial Analysis of Connectivity
We compared the extent that intermittent streams serve as outlets of channelized flow for wetlands that appear to have only groundwater discharge based on widely available “high resolution” hydrographic data (USGS, 2018; 1:24,000) to much higher resolution True Color orthophotography (RIDEM, 2011; 1:300). Lane and D'Amico (2016) conducted a similar hydrologic connectivity analysis of NWI wetlands to USGS NHDPlusV2 streams across the USA. Using a buffer of 10 m they estimated that groundwater discharge wetlands in Rhode Island occur at a density of 2.4 wetlands km−2 which is comparable to our study results using the NHDPlus HR (68% yielding a density of 2.6 groundwater discharge wetlands km−2). Field surveys in nine regions of the US estimated that most regions have more than 200% intermittent stream channel length than depicted in NHDPlus maps (1: 24,000 or 1: 100,000 scales; Fritz et al., 2013). Nadeau et al. (2015) also found poor agreement between field verified intermittent streams and USGS 1:24,000 maps in the Pacific Northwest. We found that the percent of wetlands connected to stream outlets by intermittent streams was 135% higher than our estimate of such wetlands from NHDPlus HR geospatial data.
By examining long-term average streamflow gauged by USGS (Armstrong et al., 2004), we evaluated the relative importance of these intermittent streams in contributing streamflow to local perennial streams in different seasons. In the south coastal region of southern New England where the study site is located, median streamflow was estimated at 2.11 and 1.12 mm d−1 in the spring (March to May) and fall (Oct to Dec), respectively (Armstrong et al., 2004). In the spring, median flow of intermittent streams (generated from dataloggers in 2012 at Sites 1, 2, 4, and 6; Figure 3) was 0.50 mm d−1 (0.33 mm d−1, adjusted for an intermittent stream density of 1.5 km−2) thereby intermittent streams that serve as the outlets of wetlands may contribute 24% of the spring flow from perennial streams in this region. In the fall, median flow observed in the same intermittent streams in 2012 was 0.54 mm d−1 (0.35 mm d−1 adjusted for density of 1.5 km−2) yielding 47% of the fall flow from perennial streams in this region. We assume that groundwater baseflow constitutes the bulk of difference in normalized flow between the intermittent streams and the perennial streams. Thus, many wetlands that are mapped as groundwater discharge sites appear to be directly connected to the stream network by intermittent streams—thus provide a substantial portion of perennial flows in spring and fall when the flow regime is elevated in this region.
Potential Drivers of Whole-Reach Nitrate-N Uptake
To compare to other intermittent and low order streams, we converted our non-zero rates to total uptake (U in units of μg N m−2 h−1). The complete range of U in this study's intermittent streams was within the range of rates reported in other studies (Table 5). There are many possible drivers, some of which may co-vary or correlate, of whole-reach gross uptake in the intermittent streams. Like this study, many others have found higher uptake with lower Q, velocity or water depth in headwater and perennial streams (Poff et al., 1997; Alexander et al., 2000, 2007, 2009; Peterson et al., 2001; Seitzinger et al., 2002). When we plot the significantly negative relationship between each of these factors v. whole-reach gross uptake rates, we discovered cut-offs above which no whole-reach gross uptake occurred. With Q (Figure 5), we found that substantial whole-reach gross uptake only occurs when Q is less than 7 L s−1. Velocity and depth co-vary with Q so accordingly, we found that substantial whole-reach gross uptake only occurs when velocity is < 0.043 m s−1 and depth is <15 cm. Kellogg et al. (2010) was able to estimate in-stream denitrification rates in streams using water depth, travel time, and Q.
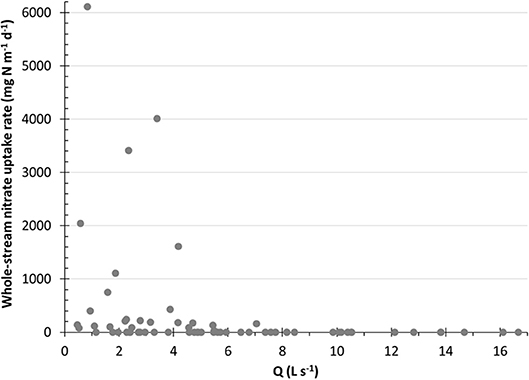
Figure 5. Whole-reach gross nitrate-N uptake rates (mg N m−1 d−1) from pulse addition experiments vs. discharge (L s−1). Above 7 L s−1, we did not observe measurable whole-reach gross uptake during the pulse addition experiments. The entire dataset from pulse addition experiments is included.
The higher incidence of hot moments in the spring vs. fall corresponded to higher temperatures. The occurrence of hot moments in the spring may also have been driven by sunlight. Hefferman and Cohen (2010) estimated that 35% of denitrification in their river may be fueled by photosynthesis in the prior day. Lupon et al. (2016) attributed diel fluctuations in stream concentrations to photoautotrophic N uptake. While we did not look at diel patterns or light intensity, sunlight may have contributed to the higher incidence of hot moments of whole-reach gross uptake in the spring as canopy cover was not completely full until the summer.
Other studies have demonstrated that progressive drying of stream beds drives an increase of in soils that will release with rewetting (Pohlon et al., 2013; von Schiller et al., 2017; Arce et al., 2018), but a model by Tritthart et al. (2011) indicated microbial respiration increases when streams rewet which may lead to some uptake of . Furthermore, Fromin et al. (2010) found that the structure of microbial communities was not strongly reduced after drying events. Sporadic rainfall during dry periods – which we generally observe in our humid, temperate climate—may modulate these N effects (von Schiller et al., 2017; Arce et al., 2018).
Transient storage can be a driver of uptake in stream ecosystems. Mulholland et al. (2009) found that denitrification was related to longer residence times in transient storage indicated by F . Transient storage within a stream reach can temporarily detain solutes in the hyporheic zone, small eddies, or stagnant pools of water that are flowing slower than the main channel; streams with high transient storage have been found to serve as substantial sinks for (Baker et al., 2011; Stewart et al., 2011). The extended transient storage in these intermittent streams may be hotspots of whole-reach gross uptake. We expect that the structure of these streams may be related to the observed extended transient storage in the study streams. The channels are supported by woody debris from the forest; debris dams create pools with enhanced opportunity for water residence on the surface and within the subsurface. However, the debris dams and pools of intermittent streams are not stable as we noted qualitatively in frequent visits to the sites. We noted that debris dams disappeared, new debris dams established, and new pools developed in the intermittent streams multiple times over a year as observed by Uehlinger (2000), Smith and Kaushal (2015), and Reisinger et al. (2017). Flow related to storm events has been related to displaced debris dams and pools along with scoured biofilms within the stream (Kaushal et al., 2018).
Dissolved organic carbon can be a potential driver of whole-reach gross uptake in streams directly by being consumed in uptake (Sobczak et al., 2003; Goodale et al., 2005) or indirectly by stimulating stream metabolism that may then lead to the uptake (Bernhardt and Likens, 2002). Bernal et al. (2005) noted that stream Q in intermittent streams greatly impacts DOC concentration and the ratio of C:N. In addition to lower storage time during higher flows, DOC could also be limiting in those conditions. In addition, the lability of the DOC may be important than the DOC concentration (Sobczak et al., 2003) which could explain why we did not see a correlation between whole-reach gross uptake and DOC. If the lability of the DOC is low, it may result in low microbial activity and less potential for removal.
N Cycling in Stream Substrates
Microbial assays demonstrated that denitrification potential in intermittent stream sediments can be substantial (Table 4). Denitrification potential in the intermittent stream substrates was within the same order of magnitude of stream substrates in three low order, perennial streams in MD, USA (Groffman et al., 2005). However, the trends were reversed—in this study, pools and riffles were an order of magnitude greater in denitrification potential whereas in the Groffman et al. (2005) perennial streams, the debris dams had the highest mean denitrification potential. Groffman et al. (2005) attributed the high rates in the debris dams to high organic carbon levels in these features that contained primarily mineral soil. The leaf-dominated debris dams in the study intermittent streams, which were routinely displaced and recreated, was largely undecomposed and exposed to more aerobic conditions than the debris dams in the perennial streams observed by Groffman et al. (2005). In these intermittent streams, the summer contraction of the streams also lowers the rate of leaf breakdown even for extended periods after flow resumes (Datry et al., 2011) which also supports why the intermittent stream in this study were not as active. However, the high rates in the pools and riffles may foster denitrification in intermittent streams.
Rates of potential net mineralization, potential net nitrification, and microbial respiration were generally higher in the intermittent streams than observed in the low order perennial streams studied by Groffman et al. (2005) (Table 4). The transport, deposition and aeration patterns of sediments associated with the annual hydrograph (i.e., elevated flows following by extended periods of drying) of intermittent streams are likely to enhance both the pool of organic N in the sediments and the subsequent rates of mineralization and nitrification. Since pools increase the residence time of stream , this substrate may be important for whole-reach gross uptake in intermittent streams. Microbial respiration in the intermittent stream debris dams was nearly 14 times higher than that of the pools and riffles which agrees with work by Tank et al. (1993) and Hedin (1990) that observed that small woody debris can play a significant role as a substrate for microbial metabolism.
Scaling Up Nitrate-N Dynamics
We examined the whole-reach gross uptake in the intermittent streams during baseflow conditions, rather than during the storm events or the lowest flows or dry conditions in summer. Therefore, the data reflect only a portion of the flow regime (albeit, the most common portion, representing a median of 75% of the year). Bernhardt et al. (2017) demonstrated the importance of rare events for characterizing ecosystem fluxes; thus, we scaled up our observations of whole-reach gross uptake rates to assess its significance in the hilly, forested landscapes of the Northeast USA. Based on the pulse addition experiments during baseflow, we estimated that 37% of the days with flow had measurable whole-reach gross uptake. Across all 12 sites over 3 years, flow was observed an average of 277 d yr−1. Combining these estimates, 103 d yr−1 would have measurable whole-reach gross uptake rates. When the whole-reach gross uptake was measurable, we coupled the interquartile range of the non-zero whole-reach gross uptake rates from the pulse additions (132.9 to 842.3 mg N m−1 d−1) with the interquartile range of the length of the intermittent streams (201 to 618 m, Table 1) and the density of intermittent streams (1.5 km−2).
Our assessment suggests that intermittent streams can potentially remove 4.1 to 80.4 kg km−2. This estimate is relatively modest compared to the median N load delivered to the catchment outlet (336 kg N km−2) for New England (Moore et al., 2004). However, the whole-reach gross uptake estimates may be low since we excluded six hot moments that were above the upper quartile of the analysis; these removed values were 1.3 to 7.2 times the upper quartile value. The transient storage metrics measured in the six intermittent streams were comparable or higher than estimates from perennial streams, indicating the potential for high cycling. In addition, our results did not include times when the stream channels were at their lowest while still flowing; nutrient retention is expected to be highest due to high water area to biological active surfaces (Fisher et al., 1998; von Schiller et al., 2017) and low dissolved oxygen conditions (von Schiller et al., 2011, 2017). However, based on data from low order, perennial streams (Vaughan et al., 2017), nutrient flux at these low flow conditions are expected to represent a small proportion of annual flux, suggesting that this omission does not markedly alter the estimates of the role of intermittent streams in nutrient export. In addition to providing substantial flows in spring and fall to perennial streams, intermittent streams in temperate, humid climates like the Northeast USA appear to contribute to whole-reach gross uptake and retention in the headwaters.
Conclusion
We have presented evidence that intermittent streams in the Northeast USA provide valuable ecosystem services. Intermittent streams frequently connect wetlands that appear to be groundwater discharge on traditional map scales (1:24,000 scale) to perennial streams during most of the year, provide 24–47% flow to perennial streams, and contribute to whole-reach gross uptake, especially during hot moments of low Q. These wetlands that do not have an outlet to a channelized stream (i.e., groundwater discharge wetlands) are frequently referred to as “hydrologically isolated”—a phrase that carries weight in governmental politics and regulations. Our study demonstrates that high resolution maps show that the extent of wetlands with channelized outlets is substantially higher than expected when using traditional maps—with potential ramifications for regulations and policies that exempt geographically isolated wetlands from protection. We have provided evidence of intermittent stream contribution to the physical and biogeochemical integrity to downstream waters during the 75% of the year they have continuous flow.
Non-perennial streams are at risk of being buried or degraded worldwide (Acuña et al., 2014). In the Northeast USA, the most common threats are urbanization and recreational developments, (e.g., golf courses); other areas at risk from agricultural development and intensification. As alterations to intermittent streams increase, perennial streams lose a portion of their headwaters and potential whole-reach gross uptake areas may become sources to downstream areas. We encourage policy makers to manage the landscape as a complete river system encompassing both the intermittent and perennial waters (Acuña et al., 2014; Leigh et al., 2015).
Data Availability
The raw data supporting the conclusions of this manuscript will be made available by the authors, without undue reservation, to any qualified researcher.
Author Contributions
KA: project manager, synthesis of data, and lead writer of the manuscript. AG: principal investigator and associate synthesizer/writer/editor of the manuscript. MW: lead field assistant and data processor. PA: geospatial analysis guidance. MS: soils guidance. CA: ran OTIS-P to model hydraulic transport parameters. PG: nitrogen dynamics guidance and editing of the manuscript.
Funding
This work was supported by the USDA National Institute of Food and Agriculture, Multi-State Hatch S-1063 (project accession no. 1005742) and Integrated Project (project accession no. 0211433).
Conflict of Interest Statement
The authors declare that the research was conducted in the absence of any commercial or financial relationships that could be construed as a potential conflict of interest.
Acknowledgments
Many hands were needed to complete this project. Thanks to all who assisted with field and lab work: Suzanne Cox, Aimee Welch, M. Nicole Gutierrez, Ellen Vancelette, Cody Miller, Charleve Carey, Liz Elmstrom, Matt Wallace, and Colin Massa. Thanks to Bianca Ross and Matt Ricker for soil descriptions. GIS work was made possible by Sarah Frazer and Seaver Anderson. Thanks to Joseph Loffredo for additional data analysis. Thanks to Autumn Oczkowski for DOC analysis.
Supplementary Material
The Supplementary Material for this article can be found online at: https://www.frontiersin.org/articles/10.3389/fevo.2019.00225/full#supplementary-material
Abbreviations
DEA, Denitrification enzyme activity; DOC, Dissolved organic carbon; NHD, National Hydrography Dataset; NWI, National Wetlands Inventory; Q, discharge; U, total nitrate-N uptake.
References
Acuña, V., Datry, T., Marshall, J., Barceló, D., Dahm, C. N., Ginebreda, A., et al. (2014). Why should we care about temporary waterways? Science 343, 1080–1081. doi: 10.1126/science.1246666
Alexander, R. B., Bohlke, J. K., Boyer, E. B., David, M. B., Harvey, J. W., Mulholland, P. J., et al. (2009). Dynamic modeling of nitrogen losses in river networks unravels the coupled effects of hydrological and biogeochemical processes. Biogeochemistry 93, 91–116. doi: 10.1007/s10533-008-9274-8
Alexander, R. B., Boyer, E. W., Smith, R. A., Schwarz, G. E., and Moore, R. B. (2007). The role of headwater streams in downstream water quality. J. Am. Water Res. Assoc. 43, 41–59. doi: 10.1111/j.1752-1688.2007.00005.x
Alexander, R. B., Smith, R. A., and Schwarz, G. E. (2000). Effect of stream channel size on the delivery of nitrogen to the Gulf of Mexico. Nature 403, 758–761. doi: 10.1038/35001562
Arango, C. P., James, P. W., and Hatch, K. B. (2015). Rapid ecosystem response to restoration in an urban stream. Hydrobiologia 749, 197–211. doi: 10.1007/s10750-014-2167-z
Arce, M. I., del Mar Sanchez-Montoya, M., and Gomez, R. (2015). Nitrogen processing following experimental sediment rewetting in isolated pools in an agricultural stream of a semiarid region. Ecol. Eng. 77, 233–241. doi: 10.1016/j.ecoleng.2015.01.035
Arce, M. I., von Schiller, D., Bengtsson, M. M., Hinze, C., Jung, H., Alves, R. J. E., et al. (2018). Drying and rainfall shape the structure and functioning of nitrifying microbial communities in riverbed sediments. Front. Microbiol. 9:2794. doi: 10.3389/fmicb.2018.02794
Armstrong, D. S., Parker, G. W., and Richards, T. W. (2004). Evaluation of Streamflow Requirements for Habitat Protection by Comparison to Streamflow Characteristics a Index Streamflow-Gaging Stations in Southern New England. Water-Resources Investigations Report 03-04332. Reston, VA: U.S. Geological Survey.
Baker, D. W., Beldsoe, B. P., and Price, J. M. (2011). Stream nitrate uptake and transient storage over a gradient of geomorphic complexity, north-central Colorado, USA. Hydrol. Process. 26, 3241–3252. doi: 10.1002/hyp.8385
Benstead, J. P., and Leigh, D. S. (2012). An expanded role for river networks. Nature Geosci. 5, 678–679. doi: 10.1038/ngeo1593
Bernal, S., Butturini, A., and Sabater, F. (2005). Seasonal variations of dissolved nitrogen and DOC:DON ratios in an intermittent Mediterranean stream. Biogeochemistry 75, 351–372. doi: 10.1007/s10533-005-1246-7
Bernal, S., and Sabater, F. (2012). Changes in discharge and solute dynamics between hillslope and valley-bottom intermittent streams. Hydrol. Earth Syst. Sci. 16, 1595–1605. doi: 10.5194/hess-16-1595-2012
Bernal, S., von Schiller, D., Marti, E., and Sabater, F. (2012). In-stream net uptake regulated inorganic nitrogen export from catchments under base flow conditions. J. Geophys. Res. 117:G00N05. doi: 10.1029/2012JG001985
Bernhardt, E. S., Blaszczack J. R., Ficken J. R., Fork C. D., Kaiser M. L., Seybold, E. C., et al. (2017). Control points in ecosystems: moving beyond the hot spot hot moment concept. Ecosystems 20, 65–682. doi: 10.1007/s10021-016-0103-y
Bernhardt, E. S., and Likens, G. E. (2002). Dissolved organic carbon enrichment alters nitrogen dynamics in a forest stream. Ecology 83, 1689–1700. doi: 10.1890/0012-9658(2002)083[1689:DOCEAN]2.0.CO;2
Biggs, J., von Fumetti, S., and Kelly-Quinn, M. (2017). The importance of small waterbodies for biodiversity and ecosystem services: implications for policy makers. Hydrobiologia 793, 3–39. doi: 10.1007/s10750-016-3007-0
Bilby, R. E., and Likens, G. E. (1980). Importance of organic debris in the structure and function of stream ecosystems. Ecology 61, 1107–1113. doi: 10.2307/1936830
Boulton, A. J. (2003). Parallels and contrasts in the effects of drought on stream macroinvertebrate assemblages. Freshw. Biol. 48, 1173–1185. doi: 10.1046/j.1365-2427.2003.01084.x
Chambers, B., Pradhanang, S. M., and Gold, A. J. (2017). Assessing thermally stressful events in a Rhode Island coldwater fish habitat using the SWAT model. Water 9:667. doi: 10.3390/w9090667
Costigan, K. H., Jaeger, K. L., Goss, C. W., Fritz, K. M., and Goebel, P. C. (2016). Understanding controls on flow permanence in intermittent rivers to aid ecological research: integrating meteorology, geology and land cover. Ecohydrology 9, 1141–1153. doi: 10.1002/eco.1712
Datry, T., Bonada, N., and Boulton, A. J. (2017). “Conclusions: recent advances and future prospects in the ecology and management of intermittent rivers and ephemeral streams” in Intermittent Rivers and Ephemeral Streams, eds. T. Datry, N. Bonada, A. Boulton (Cambridge, MA: Academic Press), 563–584. doi: 10.1016/B978-0-12-803835-2.00031-0
Datry, T., Corti, R., and Claret, C. (2011). Flow intermittence controls leaf litter breakdown in a French temporary alluvial river: the “drying memory.” Aquat. Sci. 73, 471–483. doi: 10.1007/s00027-011-0193-8
Datry, T., Larned, S. T., and Tockner, K. (2014). Intermittent rivers: a challenge for freshwater ecology. Bioscience 64, 229–235. doi: 10.1093/biosci/bit027
Dodds, W. K., Beaulieu, J. J., Eichmiller, J. J., Fischer, J. R., Franssen, N. R., Gudder, D. A., et al. (2008). Nitrogen cycling and metabolism in the thalweg of a prairie river. J. Geophy. Res. 113:G04029. doi: 10.1029/2008JG000696
Duff, J. H., Tesoriero, A. J., Richardson, W. B., Strauss, E. A., and Munn, M. D. (2008). Whole-stream response to nitrate loading in three streams draining agricultural landscapes. J. Environ. Qual. 37, 1133–1144. doi: 10.2134/jeq2007.0187
Eaton, A. D., Clesceri, L. S., Greenberg, A. E., and Franson, M. H. (1998). Standard Methods for the Examination of Water and Wastewater. Washington, DC: APHA, AWWA, and WEF.
Elmore, A. J., and Kaushal, S. S. (2008). Disappearing headwaters: patterns of stream burial due to urbanization. Front. Ecol. Environ. 6, 308–12. doi: 10.1890/070101
Environmental Systems Research Institute ESRI (2011). ArcGIS Desktop: Release 10. Redlands, CA: Environmental Systems Research Institute.
European Commission (2000). Directive 2000/60/EC of the European Parliament and of the Council of 23 October 2000 Establishing a Framework for Community Action in the Field of Water Policy. Off. J. Eur. Communities and L 327, 1.
Fisher, S. G., Grimm, N. B., Marti, E., Holmes, R. M., and Jones, J. B. Jr., (1998). Material spiraling in stream corridors: a telescoping ecosystem model. Ecosystems 1, 19–34. doi: 10.1007/s100219900003
Freeman, M. C., Pringle, C. M., and Jackson, C. R. (2007). Hydrologic connectivity and the contribution of stream headwaters to ecological integrity at regional scales. J. Am. Water Resour. Assoc. 43, 5–14. doi: 10.1111/j.1752-1688.2007.00002.x
Frissell, C. A., Liss, W. J., Warren, C. E., and Hurley, M. D. (1986). A Hierarchical framework for stream habitat classification: Viewing streams in a watershed context. Environ. Mgmt. 10, 199–121. doi: 10.1007/BF01867358
Fritz, K., Cid, N., and Autrey, B. (2017). “Governance, legislation, and protection of intermittent rivers and ephemeral streams,” in Intermittent Rivers and Ephemeral Streams, eds. T. Datry, N. Bonada, A. Boulton (Cambridge, MA: Academic Press), 477–507. doi: 10.1016/B978-0-12-803835-2.00019-X
Fritz, K. M., Hagenbuch, E., D'Amico, M., Reif, M., Wigington, P. J., et al. (2013). Comparing the extent and permanence of headwater streams from two field surveys to values from hydrographic databases and maps. J. Am. Water Resour. Assoc. 49, 867–882. doi: 10.1111/jawr.12040
Fromin, N., Pinay, G., Montuelle, B., Landais, D., Ourcival, J. M., Joffre, R., et al. (2010). Impact of seasonal sediment desiccation and rewetting on microbial processes involved in greenhouse gas emissions. Ecohydrol. 3, 339–348. doi: 10.1002/eco.115
Goodale, C., Aber, J. D., Vitousek, P. M., and McDowell, W. H. (2005). Long-term decreases in stream nitrate: Successional causes unlikely; possible links to DOC? Ecosystems 8, 334–337. doi: 10.1007/s10021-003-0162-8
Groffman, P. M. (1987). Nitrification and denitrification in soil: A comparison of enzyme assay, incubation and enumeration methods. Plant Soil. 97, 445–450. doi: 10.1007/BF02383235
Groffman, P. M., Altabet, M. A., Böhlke, J. K., Butterbach-Bahl, K., David, M. B., Firestone, M. K., et al. (2006). Methods for measuring denitrification: diverse approaches to a difficult problem. Ecol. Appl. 16, 2091–2122. doi: 10.1890/1051-0761(2006)016[2091:MFMDDA]2.0.CO;2
Groffman, P. M., Dorsey, A. M., and Mayer, P. M. (2005). N processing within geomorphic structures in urban streams. J. North Amer. Benthol. Soc. 24, 613–625. doi: 10.1899/04-026.1
Groffman, P. M., Holland, E., Myrold, D. D., Robertson, G. P., and Zou, X. (1999). “Denitrification” in Standard Soil Methods for Long-Term Ecological Research, eds. G. P. Robertson, C. S. Bledsoe, D. C. Coleman, P. Sollins (New York, NY: Oxford Univ. Press), 272–290.
Groffman, P. M., and Tiedje, J.M. (1989). Denitrification in north temperate forest soils: Relationships between denitrification and environmental factors at the landscape scale. Soil Biol. Biochem. 21, 621–626. doi: 10.1016/0038-0717(89)90054-0
Haggard, B. E., Storm, D. E., Tejral, R. D., Popova, Y. A., Keyworth, V. G., and Stanley, E. H. (2001). Stream nutriend retentionin three northeastern Oklahoma agricultural catchments. Trans. ASAE. 44, 597–605. doi: 10.13031/2013.6120
Hall, R. O. Jr., Tank, J. L., Sobota, D. J., Mulholland, P. J., O'Brien, J. M., Dodds, W. K., et al. (2009). Nitrate removal in stream ecosystems measured by 15N addition experiments: total uptake. Limnol. Oceanogr. 54, 653–665. doi: 10.4319/lo.2009.54.3.0653
Hanson, W. F. (2001). “Identifying stream types and management implications. The Science of Managing Forests to Sustain Water Resources,” in International Conference (Sturbridge, MA), 143, 39–46. doi: 10.1016/S0378-1127(00)00503-X
Harvey, J. W., and Wagner, B. J. (2000). “Quantifying hydrologic interacteions between streams and their subsurface hyporheic zones,” in Stream and Ground Waters, eds. J. B.Jones and P. J. Mulholland (San Diego: Academic Press), 1–44. doi: 10.1016/B978-012389845-6/50002-8
Hedin, L. O. (1990). Factors controlling sediment community respiration in woodland stream ecosystems. OIKOS 57, 94–105. doi: 10.2307/3565742
Hefferman, J. B., and Cohen, M. J. (2010). Direct and indirect coupling of primary production and diel nitrate dynamics in a subtropical spring-fed river. Limnol. Oceanog. 55, 677–688. doi: 10.4319/lo.2010.55.2.0677
Kaushal, S., Gold, A. G., Bernal, S., Newcomer Johnson, T., Addy, K., and Burgin, A. (2018). Watershed ‘Chemical Cocktails': forming novel elemental combinations in Anthropocene fresh waters. Biogeochemistry 141, 281–305.- doi: 10.1007/s10533-018-0502-6
Kellogg, D. Q., Gold, A. J., Cox, S., Addy, K., and August, P. V. (2010). A geospatial approach for assessing denitrification sinks within lower-order catchments. Ecol. Eng. 36, 1596–1606. doi: 10.1016/j.ecoleng.2010.02.006
Kemp, M. J., and Dodds, W. K. (2002). Comparisons of nitrification and denitrification in prairie and agriculturally influenced streams. Ecol. Appl. 12, 998–1009. doi: 10.2307/3061032
Kilpatrick, F. A., and Cobb, E. D. (1985) U.S. Geological Survey Techniques of Water-Resources Investigations, Book 3: Applications of Hydraulics. Washington, DC: U.S. Gov. Printing Office, A16.
Lane, C. R., and D'Amico, E. (2016). Identification of putative geographically isolated wetlands of the conterminous United States. J. Am. Water Resour. Assoc. 52, 705–722. doi: 10.1111/1752-1688.12421
Larned, S. T., Datry, T., Arscott, D. B., and Tockner, K. (2010). Emerging concepts in temporary-river ecology. Freshw. Biol. 55, 717–738. doi: 10.1111/j.1365-2427.2009.02322.x
Lautz, L. K., and Siegel, D. I. (2007). The effect of transient storage on nitrate uptake lengths in streams: an inter-site comparison. Hydrol. Process. 21, 3533–3548. doi: 10.1002/hyp.6569
Leibowitz, S. G., Wigington, P. J. Jr., Rains, M. C., and Downing, D. M. (2008). Non-navigable streams and adjacent wetlands: addressing the science needs following the Supreme Court's Raponos decision. Front. Ecol. Environ. 6, 364–371. doi: 10.1890/070068
Leigh, C., Boulton, A. J., Courtwright, J. L., Fritz, K., May, C. L., Walker, R. H., et al. (2015). Ecological research and management of intermittent rivers: an historical review and future directions. Freshw. Biol. 61, 1181–1191. doi: 10.1111/fwb.12646
Lichvar, R. W., Banks, D. L., Kirchner, W. N., and Melvin, N. C. (2016). The national wetland plant list: 2016 wetland ratings. Phytoneuron. 30, 1–17.
Lupon, A., Martí, E., Sabater, F., and Bernal, S. (2016). Green light: gross primary production influences seasonal stream N export by controlling fine-scale N dynamics. Ecology. 97, 133–144. doi: 10.1890/14-2296.1
Martin, K., Parsons, L. L., Murray, R. E., and Smith, M. S. (1988). Dynamics of soil denitrifier populations: relationships between enzyme activity, most probable number counts, and actual N gas loss. Appl. Environ. Microbiol. 54, 2711–2716.
Marton, J. M., Creed, I. F., Lewis, D. B., Lane, C. R., Basu, N. B., Cohen, M. J., et al. (2015). Geographically isolated wetlands are important biogeochemical reactors on the landscape. Bioscience 65, 408–418. doi: 10.1093/biosci/biv009
McClain, M. E., Boyer, E. W., Dent, C. S., Gergel, S. E., Grimm, N. B., Groffman, P. M., et al. (2003). Biogeochemical hot spots and hot moments at the interface of terrestrial and aquatic ecosystems. Ecosystems 6, 301–312. doi: 10.1007/s10021-003-0161-9
McDonagh, O. T., Hosen, J. D., and Palmer, M. A. (2011). “Temporary streams: the hydrology, geography and ecology of non-perennially flowing waters,” in River Ecosystems: Dynamics, Management and Conservation, eds. S. E. Hannah and L. E Martin. (New York, NY: Nova Science Publishers Inc), 259–289.
Meyer, J. L., Strayer, D. L., Wallace, J. B., Eggert, S. L., Helfman, G. S., and Leonard, N. E. (2007). The contribution of headwater streams to biodiversity in river networks. J. Am. Water Resour. Assoc. 43, 86–103. doi: 10.1111/j.1752-1688.2007.00008.x
Molodovskaya, M., Singurindy, O., Richards, B. K., Warland, J., Johnson, M. S., and Steenhuis, T. S. (2012). Temporal variability of nitrous oxide from fertilized croplands: hot moment analysis. Soil Sci. Soc. Am. J. 76, 1728–1740. doi: 10.2136/sssaj2012.0039
Moore, R. B., Johnston, C. M., Robinson, K. W., and Deacon, J. R. (2004). Estimation of Total Nitrogen and Phosphorus in New England Streams Using Spatially Referenced Regression Models. USGS Scientific Investigations Report 2004-5012. Pembroke, NH: U.S. Geological Survey. doi: 10.3133/sir20045012
Mulholland, P. J., Hall, R. O. Jr., Sobota, D. J., Dodds, W. K., Gindlay, S. E. G., Grimm, N. B., et al. (2009). Nitrate removal in stream ecosystems measured by 15N addition experiments: denitrification. Limnol. Oceanogr. 54, 666–680. doi: 10.4319/lo.2009.54.3.0666
Mulholland, P. J., Valett, H. M., Webster, J. R., Thomas, S. A., Cooper, L. W., Hamilton, S. K., et al. (2004). Stream denitrification and total nitrate uptake rates measured using a field 15N tracer addition approach. Limnol. Oceanogr. 49:809–820. doi: 10.4319/lo.2004.49.3.0809
Nadeau, T. L., Leibowitz, S. G., Wigington, P. J. Jr., Ebersole, J. L., Fritz, K. M., Coulombe, R. A., et al. (2015). Validation of rapid assessment methods to determine streamflow duration classes in the Pacific Northwest, USA. Environ. Manage. 56, 34–53. doi: 10.1007/s00267-015-0466-4
Pennino, M. J., Kaushal, S. S., Beaulieu, J. J., Mayer, P. M., and Arango, C. P. (2014). Effects of urban stream burial on nitrogen uptake and ecosystem metabolism: implications for watershed nitrogen and carbon fluxes. Biogeochemistry 121, 247–269. doi: 10.1007/s10533-014-9958-1
Peterson, B. J., Wollheim, W. M., Mulholland, P. J., Webster, J. R., Meyer, J. L., Tank, J. L., et al. (2001). Control of nitrogen export from watershed by headwater streams. Science 292, 86–90. doi: 10.1126/science.1056874
Poff, N. L., Allan, J. D., Bain, M. B., Karr, J. R., Prestegaard, K. L., Richter, B. D., et al. (1997). The natural flow regime: a paradigm for river conservation and restoration. Bioscience 47, 769–784. doi: 10.2307/1313099
Pohlon, E., Ochoa Fandino, A., and Marxsen, J. (2013). Bacterial community composition and extracellular enzyme activity in temperate streambed sediment during drying and rewetting. PLoS ONE 8:e83365. doi: 10.1371/journal.pone.0083365
Rains, M. C., Leibowitz, S. G., Cohen, M. J., Creed, I. F., Golden, H. E., Jawitz, J. W., et al. (2016). Geographically isolated wetlands are part of the hydrological landscape. Hydrol. Process. 30, 153–160. doi: 10.1002/hyp.10610
Reisinger, A. J., Rosi, E. J., Bechtold, H. A., Doody, T. R., Kaushal, S. S., and Groffman, P. M. (2017). Recovery and resilience of urban stream metabolism following Superstorm Sandy and other floods. Ecosphere 8:e01776. doi: 10.1002/ecs2.1776
RIDEM (2011). 2011 RIDEM multispectral Orthophotography of Rhode Island. Kingston, RI: Environmental Data Center, University of Rhode Island. Available online at http://www.rigis.org (accessed April 15, 2018).
RIGIS (2014). National Wetland Inventory (NWI) for Rhode Island; NWI14. Kingston, Rhode Island: Geographic Information System (RIGIS) Data Distribution System. Available online at: http://www.rigis.org (accessed August 1, 2017).
Runkel, R. L. (1998). One-Dimensional Transport With Inflow and Storage (OTIS): a Solute Transport Model for Streams and Rivers. Water-Resources Investigations Report 98-4018 (Denver, CO: US Geological Survey).
Runkel, R. L. (2002). A new metric for determining the importance of transient storage. J.N. Amer. Benthol. Soc. 21, 529–543. doi: 10.2307/1468428
Ryan, M. A. (2017). Water policy: science versus political realities. Nature Geosci. 10, 806–808. doi: 10.1038/ngeo3049
Schade, J. D., Welter, J. R., Marti, E., and Grimm, N. (2005). Hydrologic exchange and N uptake by riparian vegetation in an arid-land stream. Freshw. Science 24, 19–28. doi: 10.1899/0887-3593(2005)024<0019:HEANUB>2.0.CO;2
Seitzinger, S. P., Styles, R. V., Boyer, E. W., Alexander, R. B., Billen, G., Howarth, R. W., et al. (2002). Nitrogen retention in rivers: model development and application to watersheds in the northeastern USA. Biogeochemistry 57, 199–237. doi: 10.1023/A:1015745629794
Skoulikidis, N. T., Sabater, S., Datry, T., Morais, M. M., Buffagni, A., Dörflinger, G., et al. (2017). Non-perennial Mediterranean rivers in Europe: Status, pressures, and challenges for research and management. Sci. Total Environ. 577, 1–18. doi: 10.1016/j.scitotenv.2016.10.147
Smith, M. S., and Tiedje, J. M. (1979). Phases of denitrification following oxygen depletion in soil. Soil Biol. Biochem. 11, 262–267. doi: 10.1016/0038-0717(79)90071-3
Smith, R. M., and Kaushal, S. S. (2015). Carbon cycle of an urban watershed: exports, sources, and metabolism. Biogeochemistry 126, 173–195. doi: 10.1007/s10533-015-0151-y
Sobczak, W. V., Findlay, S., and Dye, S. (2003). Relationships between DOC bioavailability and nitrate removal in an upland stream: an experimental approach. Biogeochemistry 62, 309–327. doi: 10.1023/A:1021192631423
Stewart, R. J., Wollheim, W. M., Gooseff, M. N., Briggs, M. A., Jacobs, J. M., Peterson, B. J., et al. (2011). Separation of river network-scale nitrogen removal among the main channel and two transient storage compartments. Water Resour. Res. 47:W000J10. doi: 10.1029/2010WR009896
Tank, J. L., Webster J. R., and Benfield, E. F. (1993). Microbial respiration on decaying leaves and sticks in a southern Appalachian stream. J. North Am. Benthol. Soc. 12, 394–405. doi: 10.2307/1467620
Thomas, S. A., Valett, H. M., Webster, J. R., and Mulholland, P. J. (2003). A regression approach to estimating reactive solute uptake in advective and transient storage zones of stream ecosystems. Adv. Water Resour. 26, 965–976. doi: 10.1016/S0309-1708(03)00083-6
Tiner, R. W. (2003). Geographically isolated wetlands of the United States. Wetlands 23, 494–451. doi: 10.1672/0277-5212(2003)023[0494:GIWOTU]2.0.CO;2
Tritthart, M., Welti, N., Bondar-Kunze, E., Pinay, G., Hein, T., and Habersack, H. (2011). Modelling highly variable environmental factors to assess potential microbial respiration in complex floodplain landscapes. Environ. Modell. Softw. 26, 1097–1111. doi: 10.1016/j.envsoft.2011.04.001
U.S. Army Corps of Engineers (2012). Regional Supplement to the Corps of Engineers Wetland Delineation Manual: Northcentral and Northeast Region (Version 2.0), eds. J. S. Wakeley, R. W. Lichvar, C. V. Noble, J. F. Berkowitz (ERDC/EL TR-12-1, Vicksburg, MS: US Army Engineer Research and Development Center).
U.S. Climate Data (2018). Available online at: https://www.usclimatedata.com/climate/kingston/rhode-island/united-states/usri0033 (accessed August 20, 2018).
U.S. Environmental Protection Agency (2015). Connectivity of Streams and Wetlands to Downstream Waters: A Review and Synthesis of the Scientific Evidence. EPA/600/R-14/475F. Washington, DC: US Environmental Protection Agency.
Uehlinger, U. (2000). Resistance and resilience of ecosystem metabolism in a flood-prone river system. Freshw. Biol. 45, 319–332. doi: 10.1111/j.1365-2427.2000.00620.x
U. S. Department of Agriculture (2010). Natural Resources Conservation Service, Field Indicators of Hydric Soils in the United States, Version 7.0., eds L. M. Vasilas, G. W. Hurt, C. V. Noble. (Washington, D.C: USDA, NRCS, in cooperation with the National Technical Committee for Hydric Soils).
USGS (2018). National Hydrography Dataset NHDPlus HR. Available online at: https://nhd.usgs.gov/data.html/ (accessed April 15, 2018).
Valett, H. M., Dahm, C. N., Campana, M. E., Morrice, J. A., Baker, M. A., and Fellows, C. S. (1997). Hydrologic influences on groundwater-surface water ecotones: heterogeneity in nutrient composition and retention. J. North. Am. Benthol. Soc. 16, 239–247. doi: 10.2307/1468254
Valett, H. M., Morrice, J. A., and Dahm, C. N. (1996). Parent lithology, surface-groundwater exchange, and nitrate retention in headwater streams. Limnol. Oceanogr. 41, 333–345. doi: 10.4319/lo.1996.41.2.0333
Vaughan, M. C. H., Bowden, W. B., Shanley, J. B., Vermilyea, A., Sleeper, R., Gold, A. J., et al. (2017). High-frequency dissolved organic carbon and nitrate measurements reveal differences in storm hysteresis and loading in relation to land cover and seasonality. Water Res. Res. 53, 5345–5363. doi: 10.1002/2017WR020491
Viessman, W. Jr., and Lewis, G. L. (2003). Introduction to Hydrology, 5th edn. Upper Saddle River, NJ: Pearson Education Inc.
von Schiller, D., Acuña, V., Graeber, D., Marti, E., Ribot, M., Sabater, S., et al. (2011). Contraction, fragmentation and expansion dynamics determine nutrient availability in a Mediterranean forest stream. Aquat. Sci. 73, 485–497. doi: 10.1007/s00027-011-0195-6
von Schiller, D., Bernal, S., Dahm, N., and Martí, D. (2017). “Nutrient and organic matter dynamics in intermittent rivers and ephemeral streams” in Intermittent Rivers and Ephemeral Streams, eds. T. Datry, N. Bonada, and A. Boulton (Cambridge, MA: Academic Press), 135–160. doi: 10.1016/B978-0-12-803835-2.00006-1
Von Schiller, D., Marti, E., and Riera, J. L. (2009). Nitrate retention and removal in Mediterranean streams bordered by contrasting land uses: a 15N tracer study. Biogeosciences 6, 181–196. doi: 10.5194/bg-6-181-2009
Williams, M. R., Buda, A. R., Elliott, H. A., Collick, A. S., Dell, C., and Kleinman, P. J. (2015). Linking nitroget management, seep chemistry, and stream water quality in two agricultural headwater watersheds. J. Environ. Qual. 44, 910–920. doi: 10.2134/jeq2014.10.0412
Williamson, T. N., Agouridis, C. T., Barton, C. D., Villines, J. A., and Lant, J. G. (2015). Classification of ephemeral, intermittent, and perennial stream reaches using a topmodel-based approach. J. Am. Water Resour. Assoc. 51, 1739–1759. doi: 10.1111/1752-1688.12352
Winter, T. C., Rosenberry, D. O., and LaBaugh, J. W. (2003). Where does the ground water in small watersheds come from? Groundwater. 41, 989–1000. doi: 10.1111/j.1745-6584.2003.tb02440.x
Wohl, E. (2017). The significance of small streams. Front. Earth Sci. 11, 447–456. doi: 10.1007/s11707-017-0647-y
Keywords: nitrate, headwater stream, intermittent stream, wetland, connectivity, transient storage
Citation: Addy K, Gold AJ, Welsh MK, August PV, Stolt MH, Arango CP and Groffman PM (2019) Connectivity and Nitrate Uptake Potential of Intermittent Streams in the Northeast USA. Front. Ecol. Evol. 7:225. doi: 10.3389/fevo.2019.00225
Received: 04 October 2018; Accepted: 29 May 2019;
Published: 19 June 2019.
Edited by:
Gilles Pinay, National Research Institute of Science and Technology for Environment and Agriculture (IRSTEA), FranceReviewed by:
Stephen J. Ventura, University of Wisconsin-Madison, United StatesMatthew Cohen, University of Florida, United States
Copyright © 2019 Addy, Gold, Welsh, August, Stolt, Arango and Groffman. This is an open-access article distributed under the terms of the Creative Commons Attribution License (CC BY). The use, distribution or reproduction in other forums is permitted, provided the original author(s) and the copyright owner(s) are credited and that the original publication in this journal is cited, in accordance with accepted academic practice. No use, distribution or reproduction is permitted which does not comply with these terms.
*Correspondence: Kelly Addy, a2FkZHlAdXJpLmVkdQ==