- 1Smithsonian Conservation Biology Institute, Front Royal, VA, United States
- 2Section of Ecology, Behavior and Evolution in the Division of Biological Sciences, University of California, San Diego, San Diego, CA, United States
- 3Trunks & Leaves Inc., San Diego, CA, United States
Decisions based on trends in population abundance and distribution may fail to protect populations of slow-breeding, long-lived megafauna from irrevocable decline if they ignore demographic constraints. For such taxa, we urge that effort be directed at understanding the interactions among vital rates governing population growth rates, rather than on predicting probabilities of extinction. The proximity of a population to demographic tipping points, i.e., where growth rate switches from positive to negative, can signal vulnerability to perturbation long before numbers drop below a point of no return. We define the “demographic safe space” as the combination of key vital rates that support a non-negative growth rate and illustrate this approach for Asian elephants. Through simulations, we find that even with optimal reproduction, Asian elephant populations cannot tolerate annual female mortality rates exceeding 7.5%. If adult mortality is very low (3%/year), populations can tolerate high annual mortality in calves below age 3 (up to 31.5%/year), or slow female reproduction (primiparity at 30 years or average inter-birth interval of up to 7.68 years). We then evaluate the potential impact of current threats, showing that near-optimal reproduction and high calf survival is necessary to offset even modestly increased mortality among adult female age classes. We suggest that rather than rely on simple counts or “viability” assessments, conservation planners for slow-breeding megafauna should consider demographic tipping points and strive to keep populations within their safe spaces.
Introduction
Large body sizes make megafauna exceedingly influential and vulnerable members of their ecosystems (Campos-Arceiz and Blake, 2011; Haynes, 2012; Ripple et al., 2015, 2016; Doughty et al., 2016; Malhi et al., 2016). Vulnerabilities include extrinsic threats, such as overharvest or habitat loss, as well as intrinsic attributes, such as slower generation times and large area requirements (Purvis et al., 2000; Cardillo et al., 2005). Conservation action is frequently motivated by one of two contrasting yet complementary paradigms: that of declining populations vs. that of small populations (Caughley, 1994). The former focuses on diagnosing and treating the causes of decline, as exemplified by criteria A-D under Section V of the IUCN Red List; v.3.1, 2001, while the latter emphasizes action when populations become small enough to risk near-term extinction, as exemplified by criteria D-E, IUCN Red List v.3.1 2001(Mace et al., 2008). Practitioners and policy makers frequently make such assessments on the basis of trends in abundance and distribution, but these can be misleading for slow-breeding, long-lived taxa because short-term fluctuations may mask long-term trends. Thus, decisions made in the absence of demographic understanding may be ill-informed.
Where empirical data on age- or stage-structure, survival, and reproduction are available, demographic modeling may be used to project population trends. Although the two paradigms highlighted by Caughley (1994) are ideally applied in tandem, population viability analyses (PVA) frequently emphasize the small population paradigm. This is perhaps owing to its well-developed theoretical underpinnings and readily available toolkits (Boyce, 1992; Beissinger and Westphal, 1998; Coulson et al., 2001; Reed et al., 2002). One of the most common approaches is to estimate extinction (or quasi-extinction) probabilities over some timescale, which may be accompanied by an estimate of the minimum viable population size (MVP), corresponding to an “acceptable” level of risk in the face of demographic, environmental or genetic stochasticity, and natural catastrophes (Shaffer, 1981; Boyce, 1992; Traill et al., 2010; Pe'er et al., 2013). At the site-level, MVPs may inform management targets and policy decisions due to their perceived simplicity. MVPs then become “rules of thumb,” guided by general species attributes such as body size (Traill et al., 2010; Brook et al., 2011; Flather et al., 2011; Hilbers et al., 2017). Such practices are highly questionable (Coulson et al., 2001), particularly if parameterized with data borrowed from other sites or even species.
First, there can be no single target for population size that is appropriate across biological contexts (Flather et al., 2011), and depending on the specifics, the estimate may be higher than practitioners are prepared to acknowledge (Reed et al., 2003; Traill et al., 2010). Second, PVA outcomes can vary widely depending on data quality and timescales, which can be inadequate or arbitrary for endangered species (Beissinger and Westphal, 1998; Coulson et al., 2001; Reed et al., 2002; Traill et al., 2010; Flather et al., 2011; Pe'er et al., 2013). Third, incomplete parameter specifications and implicit assumptions make PVA results difficult or impossible to reproduce (Burdett et al., 2010; Pe'er et al., 2013). Fourth, if one focuses on “the moment at which a declining population becomes a small population” (Traill et al. 2010, authors' own emphasis), i.e., when risk of extinction is no longer negligible, one risks setting targets that are inadequate for long-term persistence (Traill et al., 2010). In fact, the concept of an MVP was originally proposed only as a means of evaluating risks for small populations under conditions that otherwise favor population growth, not as minimal floors for populations already in decline (Shaffer, 1981; Flather et al., 2011). Finally, and perhaps most importantly, MVP estimates should not substitute the diagnosis and treatment of systematic threats to persistence (Murray et al., 2014). Over-reliance on MVPs impedes timely action to identify and tackle the underlying problems (Reed et al., 2002; Flather et al., 2011).
These issues are especially salient for mega-herbivores, as simulations have shown extinction probabilities for long-lived, slow-breeding species could appear negligible on the shorter timescales that are considered to be conservation-relevant (Beissinger and Westphal, 1998; Armbruster et al., 1999; Coulson et al., 2001). History bears this out. Genomic studies of the last mammoths isolated on Wrangel Island have shown that, although they were able to persist for thousands of years beyond the extinction of mainland populations with an effective population size of just ~300 individuals, they had accumulated numerous genetic mutations that may have eventually contributed to their extinction (Palkopoulou et al., 2015; Rogers and Slatkin, 2017). Thus, populations of megafauna can persist on timescales far exceeding those typically considered relevant, yet still be biologically inviable. Meanwhile, extant species may not receive sufficient attention until populations collapse (Linklater, 2003). For example, PVA results were used to argue in favor of captive breeding over in situ protection and reintroductions of the critically endangered Sumatran rhinoceros once crisis was acknowledged (Maguire et al., 1987), but the gamble has not yet paid off owing to unexpectedly high mortality and low reproductive success in captivity (Rabinowitz, 1995; Hance, 2018). Populations of slow-breeding taxa need proactive management well before numbers become critically low, when returns on investment are potentially greater and populations less likely committed to extinction (Figure 1).
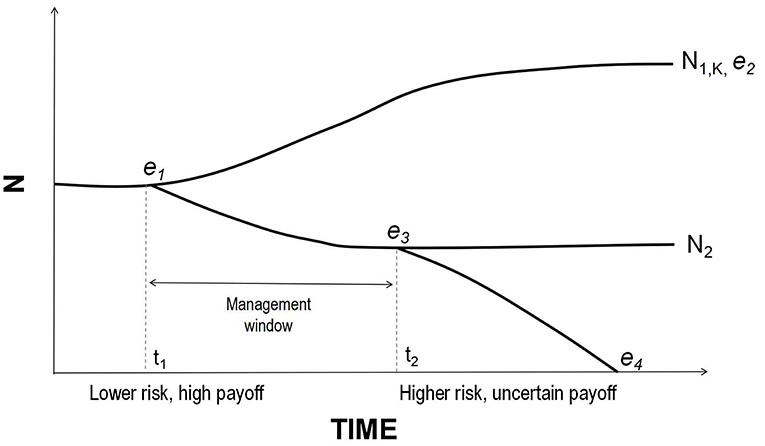
Figure 1. A schematic of possible population growth trajectories. At time t1 the population exhibits vital rates yielding a growth rate close to zero. Equilibrium e1 may be unstable; if conditions improve slightly, it could move toward the stable equilibrium e2 at K. It could also decline and stabilize at e3 below carrying capacity. However, perturbations at t2 could push the population into an extinction vortex. Management actions may only be effective prior to crossing e3 but it may be difficult to determine whether a declining population is past a point of no return because all equilibria (except extinction) look alike with respect to r. Thus, managers must assess whether the factors driving decline are likely to be chronic or temporary and intervene as early as possible in the former instance (for visual clarity curves are here depicted as smooth lines, without the short-term fluctuations characteristic of real populations).
PVA are also applied in this context, for instance to help practitioners decide which vital rates or age-/stage-classes require management. Sensitivity and elasticity analyses are typically used to establish which variables are most influential on the growth rate r, and by extension, extinction risk. Unfortunately, data limitations hinder rigorous sensitivity tests for many threatened taxa (McCarthy et al., 1995; Mills et al., 1999; Heppell et al., 2000; Coulson et al., 2001; Cross and Beissinger, 2001; Holmes, 2001; Brook et al., 2011; Pe'er et al., 2013; Hilbers et al., 2017). As an ad hoc workaround, one may use life-history characteristics as a guide for which vital rates or life stages are most critical (Heppell et al., 2000). For instance, for species with “slow” life histories (characterized by longevity, late maturity and low fecundity), adult survival is likely to influence growth rate more strongly than fecundity, whereas the opposite is assumed for species with “fast” life histories (Heppell et al., 2000). The issue with such generalizations, however, is that they offer little insight on what actually constitutes healthy vital rates in any given situation because rates interact with one another. For instance, although there is no doubt that adult survival is critical for slow-breeding species, reproductive rates mediate a population's resilience to disturbance and are especially crucial for recovering taxa that are in decline (Manlik et al., 2016). Alternatives to extinction probability as the primary indicator of population vulnerability are therefore required.
We propose another means of evaluating a population's demographic health based on the species' physiological constraints and the population's time-averaged growth rate. We refer to this as “tipping point analysis” to distinguish it from classical PVA, and define the “demographic safe space” as the set of values of vital rates, for a given population, that support a non-negative growth rate on average, irrespective of short-term stochastic fluctuations. Its boundaries are set by natural limits on reproduction and mortality, together with those at which the average growth rate, r, is zero. The latter represent demographic “tipping points” (c.f. Drake and Griffen, 2010; Dai et al., 2012). The tipping point concept has varied uses in ecology and may be applied at the scale of populations, communities, ecosystems, or even the planet, but fundamentally signifies a bifurcation point (or unstable equilibrium) between alternative states (or stable equilibria) (Scheffer et al., 2009; Lenton, 2011; Hughes et al., 2013; Lynch et al., 2014; Selkoe et al., 2015). Demographic tipping points are the combinations of vital rates at which a population switches from growth to decline (Figure 1). All demographic variables can have tipping points, not just population size. Near such thresholds, small changes in demographic rates alter population trajectories many generations before extinction (Scheffer et al., 2009; Traill et al., 2010). Unlike sensitivity/elasticity analyses of traditional PVAs, tipping point analyses highlight risk of decline rather than extinction (see also Akçakaya and Sjogren-Gulve, 2000; Holmes, 2001; Gerber, 2006). We encourage conservation practitioners to assess whether populations of mega-herbivores are within their demographic safe spaces, then act accordingly (Figure 2).
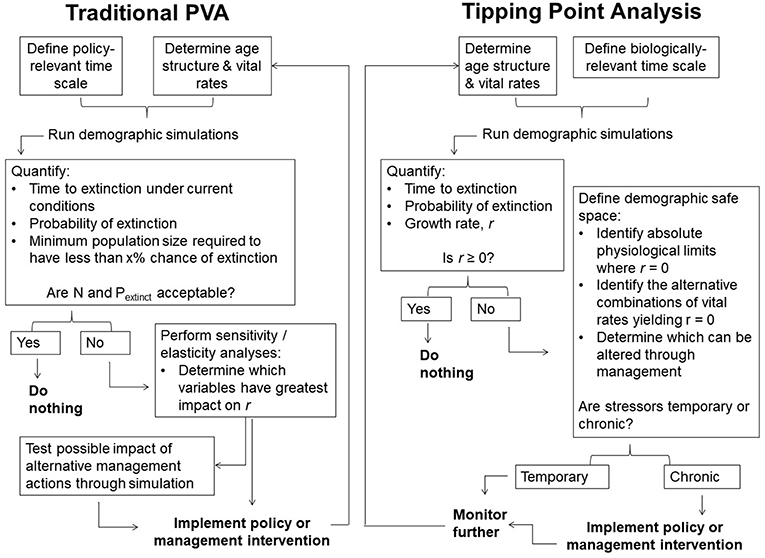
Figure 2. Traditional Population Viability Analysis vs. Tipping Point Analysis. Two possible decision cycles highlighting distinctions between traditional PVA and the proposed approach. Both use models predicting population dynamics, but emphasize different outcomes. Traditional PVA emphasizes extinction probability and corresponding population sizes, with action taken when these become unacceptable over some near term. Tipping point analyses emphasize the population growth rate, with action encouraged when the growth rate is negative and the underlying causes are deemed to be chronic. The time scale of analyses doesn't matter except to provide an accurate sense of the long-term trajectory. Sensitivity/elasticity analyses are avoided by focusing on interactions among variables of concern and their putative limits.
We demonstrate how characterizing tipping points is informative for managing a slow-breeding, endangered ecosystem engineer, the Asian elephant (Elephas maximus). Extrinsic threats to Asian elephants include overharvest, human-elephant conflict, and range loss. Ivory trade presents a limited threat to Asian elephants as all females and a fraction of males are tuskless (Figure 3a), but trafficking of live animals, skin and parts (Figure 3b) is prevalent (Nijman, 2014; Nijman and Shepherd, 2014; Sampson et al., 2018). Elephants can also be killed through human-elephant conflict, non-targeted hunting devices, and suffer indirect harm through rapid habitat loss (Figure 3c). Less than half of elephant range consists of un-fragmented wildlands (Leimgruber et al., 2003), with nearly all of the remaining range falling within ecoregions at a very high risk of conversion (Watson et al., 2016). Many populations in southeast Asia suffer from both small size and fragmentation, with most surveyed populations numbering fewer than 500 individuals and several in double digits (Hedges et al., 2005; Moßbrucker et al., 2015;AsERM, 2017).
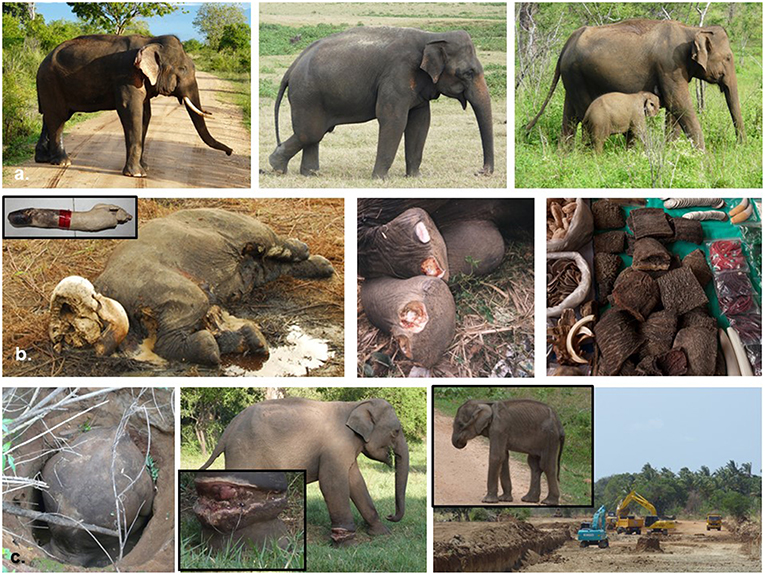
Figure 3. Asian elephants and their threats. (a) Top row: Adult male with tusks, adult male without tusks and female nursing a healthy calf (Photos: Uda Walawe Elephant Research Project). (b) Middle row: Poaching for skins and parts is emerging as a phenomenon that can potentially devastate wild populations. Left to right: Critically endangered Sumatran elephant poached for undeveloped tusks, or “tushes” (Photo: Frankfurt Zoological Society); Elephant carcass with toenails removed (Photo: Wildlife Conservation Society); Elephant skin alongside other animal products for sale in Mong-La, Myanmar (Photo: Alex Hofford). (c) Bottom row: Anthropogenic hazards such as open wells and snares can cause visible mortality, but reproductive depression and deaths among all age classes from wholesale habitat destruction can be less obvious (Photos: Uda Walawe Elephant Research Project).
We first systematically define demographic tipping points under known physiological limits assuming idealized conditions. We then perform simulations of more realistic population dynamics modeled on available literature, including for the first time data from the only individual-based longitudinal study of a wild population (de Silva et al., 2011, 2013). We explore interactions of vital rates when perturbed to illustrate the concept of safe space, then evaluate extinction risk under realistic conditions, including situations in which mortality exceeds reproductive capacity. We show that Asian elephants are highly vulnerable to the simultaneous disruption of more than one key demographic variable and are fairly constrained in the space of vital rates required to maintain stable populations. We discuss the implications of these outcomes for conserving elephants specifically and mega-herbivores at large.
Methods
Modeling Environment and Basic Life History
We performed all analyses in VORTEX, an individual-based, stochastic demographic modeling environment widely used for population viability studies (Brook et al., 2000; Lacy, 2016; Morrison et al., 2016), and follow recommendations for reporting as outlined by Pe'er et al., (2013), insofar as they apply to the approach presented. We ran all simulations over 1,000 years and performed 500 iterations per scenario type. For simplicity, we considered only a single population (or super-population). We set the initial population size at 1,000 individuals and defined the initial age structure as given in Table S1 (based on de Silva et al., 2013). We fixed carrying capacity K at 5,000 individuals, a number large enough to avoid adverse impacts on populations (Brook et al., 2006; Traill et al., 2010; Flather et al., 2011). We did not assume a stable age distribution, as this is highly unlikely in wild elephants (de Silva, 2010). The birth sex ratio was set at 1:1 (de Silva et al., 2013). We assumed females were dependent on their mothers until the age of three, at which calves that lost their mothers before weaning did not survive (Mar et al., 2012; de Silva et al., 2013; Lahdenperä et al., 2015).
Because there is no one-to-one mapping between threat types and their impact on demographic variables, we divided simulations into two broad classes of scenarios: idealized, and naturalistic. In the idealized scenarios we initially fixed the critical reproductive and mortality parameters at their minimal values and varied each one independently to determine the maximum range of each, all else being optimal. In the naturalistic scenarios, we adjusted multiple variables simultaneously, as would be expected under various disturbance regimes. The following simulations, therefore, do not represent any particular population, but are parameterized from known populations. A summary of the variables of interest are provided in Table 1. Variables that were systematically varied are given in Table 2 and described further below. Complete specifications of all variables and how they are implemented in VORTEX are provided in the Supplementary Text.
Limits to Growth Under Idealized Conditions (Scenario Set 1)
We initially assumed a 3% mortality rate among adult females and calves below age 3. All age-specific mortality rates are given in Table S2. We initially set male mortality after the age of 13 to be twice as high as that of females because they are documented to incur a three times greater risk of injury and death upon dispersal (de Silva and de Silva, 2007; de Silva et al., 2013). The mortality rate for individuals over 60 was set to be twice as high as that of other adults of the same sex and the maximum lifespan for both sexes was set at 65 (de Silva et al., 2013).
Primiparity in females can occur at 10 years, but this is probably unrealistic in the wild and is more healthy for mother and calf at 11 years or more, therefore this was taken to be the minimal age (Sukumar, 2003; de Silva et al., 2013). The age at first reproduction for males was set at 20, reflecting the earliest age at which they are likely to have competitive rather than physical ability (Flower, 1943; Poole, 1987). The maximum age of reproduction for females was set at 60 and that of males was set at 65 (de Silva et al., 2013). Because elephants can come into oestrus only about a year after giving birth if the calf survives, and then have a gestation period of 20–22 months, the IBI cannot be <3 years and is optimal at 4 years (Flower, 1943; de Silva et al., 2013). The IBI was approximated via the “% females breeding” variable and females allowed to reproduce only when they had no dependent offspring (see variable settings in Supplementary File 1 and sample numerical calculations converting IBI to % breeding provided in Supplementary File 2).
The growth rate, r, is calculated as the average of growth rates over all 500 iterations (“stochastic growth rate” in VORTEX). The magnitude of r is the distance between the population's current position and its tipping points, whereas the sign of r indicates whether it is inside or outside these thresholds. We visualized a portion of the safe space with r as a function of three critical underlying variables: primiparity p, IBI b, and adult female mortality m. The boundaries of this space are either physiological limits, or where r(p, b, m) = 0 when all other variables are fixed as defined. Because the safe space in fact depends on more than just these three variables, this simplification is used for illustrative purposes only. One may use such a visualization to explore relationships among any of the variables of interest.
Naturalistic Scenarios (Scenarios 2–5)
We next consider scenarios more closely resembling a real population subject to multiple stressors. The baseline adult female mortality rate was set to 5%, age at primiparity was set to 13 years, and median IBI was raised to ~6 years, reflecting values measured in the wild through a longitudinal study at Udawalawe National Park, Sri Lanka (de Silva et al., 2011, 2013). Mortality rates in other age/sex classes were initially set as in Leimgruber et al. (2008). We first varied only adult female mortality from 3 to 11%, representing the 95% confidence intervals of the estimate at Udawalawe based on capture-recapture sampling (de Silva et al. 2011). As it is not practical to model all possible interactions among demographic variables over their entire range of possible values, we explored a subset of combinations illustrating certain realistic scenarios (Table 2).
We then quantified extinction risk for populations beyond their safe space, operationalized in terms of the probability of extinction and time to taken to do so. The probability of extinction for a population is the proportion of times it went extinct (defined as only one sex remaining) within 1,000 years, out of 500 iterations. The mean time to extinction is the average length of time elapsed for those that did. Those with a higher probability of extinction and shorter time to extinction are understood to be at higher risk than those with a lower probability and longer time to extinction. We first examined the effect of increased mortality only among adult females, and then the effect of a 1 or 5% increase across all age classes, as might occur with either indiscriminate hunting or severe loss of habitat. We explicitly examined the effect of initial population size on extinction risk by reducing this to 500, 250, 100, 50, or 15 individuals, respectively.
Small Populations (Scenarios a–c)
We finally present contrasting scenarios that distinguish among small populations that are (a) otherwise healthy (b) male-biased, or (c) biased toward older females. Scenario (a) represents what might happen if a small population of healthy juveniles and breeding adults are introduced to a new environment, modeled by setting vital rates at their optimal values but limiting the initial population size to 5 or 50 individuals, respectively. Scenario (b) represents a landscape in which females are initially outnumbered by males (Table S3). Scenario (c) represents a relict population consisting of aging females but a typical distribution of males (Table S3). We initially set the proportion of breeding females in this scenario to 50% (IBI ~4.01 years), however, because such a population in reality will be likely to experience much lower birth rates, we then assume a reduction to 15% (IBI ~8.7 years).
Results
Idealized Outcomes
Even under idealized conditions with optimal reproduction and high calf survivorship, an elephant population can absorb an annual mortality rate of no more than 7.5% among breeding females (Table 3). Though higher mortality could be tolerated with IBI < 4 years, we note that shorter periods are only observed in the wild when females lose their calves within the first year (de Silva et al., 2013). If adult female mortality is low, a population can sustain the loss of nearly one third of un-weaned calves. If both cow and calf mortality remain at 3%, a population can afford to have either long birth intervals (~7.5 years on average) or late time to maturity (~30 years). However, if stressors affect multiple variables simultaneously, the potential response space becomes far more constrained (Figure 4; Table 3).
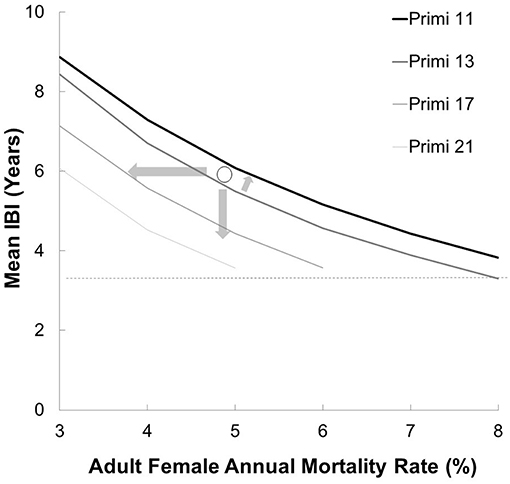
Figure 4. The demographic safe space with respect to age primiparity (p) and IBI (b) and adult female mortality rate (m) for a naturalistic population (Table 1). Curves represent the threshold at which r(p,b,m)~0 (actual data points not shown for visual clarity) for populations under naturalistic conditions (Table 1), as found through simulation. As the age of primiparity for females increases, the IBI and mortality rate must decrease to compensate, thus safe space is the area between each respective curve and the minimal IBI of 3.5 years (dashed line). The data point shown represents a population with p = 13 years, b = 6 years and m = 5% (scenario 2), which puts it outside its safe space. It can be rescued by reducing any of the three variables (arrows).
Naturalistic Outcomes
A population with an average birth interval of 5.93 years (25 % of females breeding), slightly delayed primiparity (13 years) and 5% annual mortality among adult females is outside its safe space (Figure 4), and therefore declines (r ± SD = −0.002 ± 0.021, Figure 5). This can be compensated for either by reducing calf mortality to ≤ 2%, reducing the average birth interval to 5.54 years (28% of females breeding) or decreasing the age of primiparity to below 12 years (Table 3). As expected under a polygynous mating system, heavily male-biased mortality (30% among adults) has little impact on population trajectories when adult female mortality is held at 3% (Figure 5).
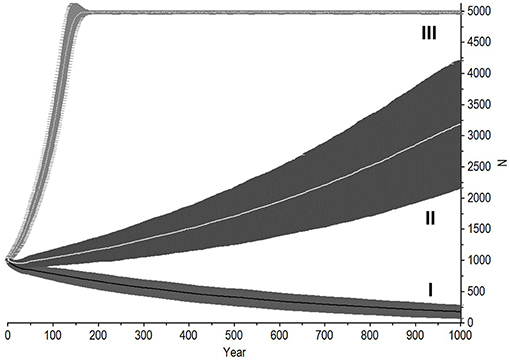
Figure 5. Interaction of adult female mortality rate, inter-birth intervals, and adult male mortality rate under naturalistic conditions. Shaded regions indicate standard deviations. (I) Scenario 2 baseline (Tables 1, 3), with 5% mortality in adult females and IBI of 5.93 years (25% females breeding). (II) Scenario 3, IBI shortened to 5.54 years (28% females breeding, Tables 1, 3). (III) Scenario 4, mortality reduced to 3% in adult females but increased to 30% in males over the age of 13, while maintaining IBI at 5.93 years (Tables 1, 2).
With 5% mortality rate in adult females, there is only a 1.4% chance of extinction within 1,000 years, despite decline (Figures 5, 6A, Table 4). Even with an unsustainable mortality rate of 11% among adult females, the time to extinction can be over a century. Smaller populations with these attributes fare worse, unsurprisingly (Figure 6A; Table 4). A mere 1% increase in mortality across all age classes results in certain extinction, though this can still take over a century for larger populations if conditions remain unchanged over that time (Table 5). A 5% increase can lead to collapse within less than a century. By contrast, if age structure and vital rates are healthy, small populations can be expected to grow if there are no catastrophic events (Figure 6B). Nevertheless, very small populations still have much higher extinction risk due to initial fluctuations, as would be expected, illustrating the original concerns behind the small population paradigm. They also plateau before reaching carrying capacity. On the other hand, populations consisting of older individuals with longer birth intervals rapidly become extinct (Figure 6C).
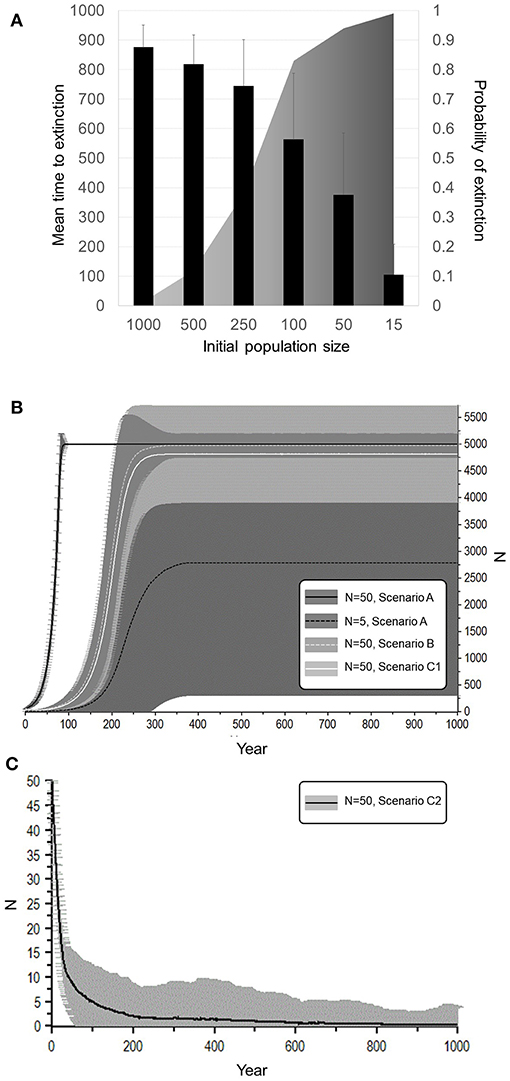
Figure 6. Dependence of extinction risk on initial population size and other attributes. (A) A population that has a negative growth rate (Scenario 2, Figure 5 curve I). (B) Scenario A represents population that has a healthy sex ratio, age structure, and optimal reproductive rates, with an initial population size of 5 or 50 individuals. The larger of these never went extinct whereas the smaller had a 44.2% chance of extinction within 32.6 years on average. Scenario B represents a population of 50 individuals with an initially highly male-biased sex ratio. It had a 16.8% chance of extinction within 62 years on average. Scenario C1 is also male-biased and females are older, but fast breeding (50% breeding or IBI 4.01). It had a 3.4% chance of extinction within 75.4 years on average. (C) Scenario C2 on lower panel shows the same population with lengthened birth intervals (15% breeding or IBI 8.7 years). It had a 99.4% chance of extinction within 120.2 years on average.
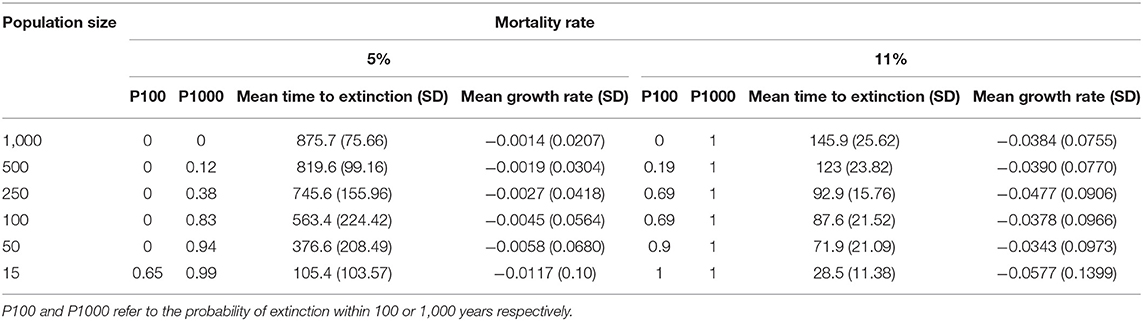
Table 4. Extinction risk based on population size and adult female mortality rate under naturalistic conditions (scenario 2, Figure 6).
Discussion
Slow reproduction increases extinction risk across taxa and is especially a concern for large vertebrates (Purvis et al., 2000; Cardillo et al., 2005), but their longevity may make extinction risk appear negligible on shorter timescales (Armbruster et al., 1999; Traill et al., 2010). For these reasons, the notion of “viability,” defined on the basis of extinction probability on some arbitrary timescale, is at best a distraction and at worst misleading. Even large populations, if in decline, will eventually pass a point of no return that is difficult to predict in advance. Rather than wait for this eventuality, we suggest that demographic tipping points, and the corresponding safe spaces they define, can offer impetus to act early even when data are scarce. Such assessments can also serve as reality checks on management policies, illustrated here for Asian elephants.
Asian elephants are both flagships and keystones of disappearing habitats in Asia, yet range-wide assessments of abundance and distributions remain speculative at best, with trends often unknown until extirpations occur (Blake and Hedges, 2004; Choudhury et al., 2008; Hedges et al., 2008; Fernando and Pastorini, 2011). It has previously been suggested that elephant populations numbering as few as 100–300 individuals could be viable on 100-year timescales, but 1,000–3,000 may be necessary on longer timescales (Sukumar, 2003). Such prescriptions are too simplistic, because they ignore the vital rates themselves. Life history attributes already predict that Asian elephants should be extremely sensitive to increases in adult female mortality (Heppell et al., 2000). Our results further show that although elephants may be able to tolerate substantial sub-optimality in any single key variable, they cannot absorb the simultaneous perturbation of multiple variables.
The attention of the international community has been extensively focused on the ivory trade, given its visible devastation of African elephant populations (Wasser et al., 2010; Bennett, 2014). But for the Asian species, the disproportionate hunting of males for ivory is unlikely to present a problem for larger populations with tuskless bulls that can compensate as breeders (Figures 3, 5). In fact, even substantially elevated male removal rates may be tolerable so long as other vital rates are favorable. The trade in skins, parts and calves represent far greater threats as they affect all age/sex classes (Figure 3, Sampson et al., 2018). Populations cannot withstand even modest increases in mortality across age classes, especially of females and calves, which typically have not been a focus of conservation attention (Tables 3, 5). Elephants in Southeast Asia are the most vulnerable due to their remoteness, fragmentation, and small sizes (Leimgruber et al., 2003; Gopala et al., 2011; Moßbrucker et al., 2015; Moßbrucker, 2016). Several subpopulations within China, Laos and Vietnam were estimated to number fewer than 20 individuals over a decade ago (Hedges et al., 2008). Obtaining demographic data, perhaps through the use of tools such as camera traps, will be as important as improving security, given that the chances for recovery depend crucially not just on numbers but on age structure and associated vital rates (Figures 5, 6; Tables 4, 5).
At least a quarter of the Asian elephant population is thought to be in captivity (Kurt et al., 2008). In Myanmar semi-captive elephants now likely outnumber those remaining in the wild (Leimgruber et al., 2008). Adults continue to be killed for parts while calves are smuggled into captivity (Nijman, 2014; Sampson et al., 2018). In Thailand, the decline of the timber industry has spurred use of captive elephants in tourism. The appeal and trainability of juvenile elephants, together with socio-cultural concerns surrounding mahout livelihoods and traditions, create perverse incentives to continue capturing. Live calves have been valued at upwards of $33,000 USD (Nijman, 2014). Our results show that calf survivorship is likely to be more crucial for population persistence than one might expect based on ideal conditions or life history (Table 3), thus the live trade could in itself easily drive populations to extinction, especially if breeding females are also killed.
On the flip side, captive-bred populations are unlikely to rescue the species, as there are currently none that are sustainable either in situ or ex situ (Wiese, 2000; Faust et al., 2006; Leimgruber et al., 2008). Nor have plans been developed for integrating or restoring captive-bred or ex-working elephants to the wild, although there are programs for restoration of orphaned wild juveniles to the wild in India and Sri Lanka. Ultimately, the disappearance of these mega-herbivores from the wild implies their disappearance from captivity as well, therefore, resources directed toward wild populations must at least match those invested in captivity from a conservation (rather than welfare) standpoint. The fact that small populations can potentially grow (Figure 6B) offers some hope that re-wilding may be demographically feasible, but only if the chronic threats are brought under control.
Meanwhile, elephant “drives,” capture, and translocation continue to be accepted management practices in range states where elephants seem more plentiful. At Yala National Park in Sri Lanka, as much as 25–50% of females were reportedly dying before adulthood due to range constriction (Kurt, 1974) while herds surviving today continue to experience calf mortality rates as high as 50% (Fernando, 2015). Drives in 2004-2005 are also contributing factors, with effects persisting for more than a decade following the initial disturbance (P. Fernando, personal communication). Among semi-captive elephants, individuals born under high stress conditions themselves have reduced lifetime reproductive success (Mumby et al., 2015), suggesting multi-generational negative feedback. Unfortunately, the collapse at Yala has gone unremarked despite its former status as a MIKE (Monitoring the Illegal Killing of Elephants) site because it was driven by land use rather than “illegal killing.” Moreover, the original MIKE sites were protected areas, whereas much of elephant range and deaths occur outside them (Leimgruber et al., 2003). Conservation initiatives need to emphasize understanding how stressors actually affect demographic processes of a population, without presuming to know the primary mechanism of decline.
Despite the species' longevity, and the well-known trade-off between early reproduction and late-life survival, there seems to be selection for early reproductive investment because older females have higher calf mortality and lower fecundity (de Silva et al., 2013; Hayward et al., 2014). This likely creates hidden extinction debt as populations age (Figure 6C), yet the relationship between age structure and vital rates is not well-known or appreciated. Ironically, the perception that populations are “increasing” in parts of India and Sri Lanka has prompted discussion as to whether to consider immunocontraception or culling in order to reduce conflict (Desai and Riddle, 2015). But there has been no reflection on whether the observed trends are accurate (see also Puyravaud et al., 2017), and if so, what is driving them. Perceived “increases” in local populations, faster than allowed by the reproductive rate, could signal the influx of individuals that have been displaced from elsewhere, the exposure of resident elephants due to habitat loss, or faulty assumptions and estimation methods. Our results show that elephants must reproduce at near-optimal rates, merely to keep up with even slight increases in mortality (Table 3). Such long-lasting interventions targeting individuals of prime breeding age on landscapes with high rates of conflict-related mortality could dangerously undermine long-term resilience (Goswami et al., 2014). We urge that management actions be based on data rather than expert opinion or speculation.
Limitations of the Approach
We have ignored spatial structure and catastrophes here, but these are obviously important for population persistence (Reed et al., 2003; Pe'er et al., 2013; Young, 2018). One may of course include these, if informed by sufficient data. Where there is population turnover, estimates of vital rates may still be obtained from a subset of longitudinally observed individuals (e.g., de Silva et al., 2011, 2013). Such samples can illustrate the demographic responses of a population even if time series of abundance are inaccurate or, as is more often the case, unavailable. We advise caution, however, because the more complex the model, the more data and decisions required for implementation without necessarily yielding greater insight (Pe'er et al., 2013). Simulations should not be mistaken for reality, especially when data are scarce (Caughley, 1994; Coulson et al., 2001).
Models also cannot substitute basic familiarity with a system (Figure 2). A population that is near a stable equilibrium (i.e., at K) may appear to exhibit the same demographic rates as one that is poised near an unstable equilibrium; one that is small or declining may or may not be capable of stabilizing or recovering (Figures 1, 6). Key variables, including juvenile survival, age at first reproduction, fecundity, and adult survival, might be expected to respond to population density when dispersal is constrained (Bonenfant et al., 2009). Distinguishing natural variation in key vital rates from density effects and sampling errors with slow-breeding taxa can present serious practical challenges, not least because of the long observation times that would be required to do so (Reed et al., 2003; Freckleton et al., 2006). For elephants specifically, evidence of density dependence in the wild is equivocal and has only been studied at all in African systems (Gough and Kerley, 2006; Chamaillé-jammes et al., 2008), which are typically more water-limited than Asian habitats. While we expect that there must be some form of density-dependent feedback when populations are restricted (as highlighted in the case of Yala National Park), there are currently insufficient data to explore these effects. Managers must carefully consider whether the pressures a population face are temporary or lasting, and whether density-dependent factors may be contributing, when interpreting results. Although the approach we present is more forgiving of incomplete data as it de-emphasizes distant outcomes, it does not obviate the need for baselines. We reiterate the call for more systematic longitudinal studies of these species in the wild (Shaffer et al., 2002; Reed et al., 2003; de Silva, 2016).
Tipping points are coupled to one another, therefore, if circumstances change one or more critical near-threshold variables, the boundaries of safe-space may be altered, requiring a fresh evaluation. This is easy to understand if one considers that, for any given set of conditions, there may be some reproductive rate that offsets the overall mortality rate, but there are different combinations of vital rates through which this balance could be achieved. However, the absolute ceiling for the mortality rate a population can tolerate is set by the fastest possible reproductive rate, which is fixed by the species' reproductive physiology. If the predicted growth rate is negative, while it is certainly possible that conditions could spontaneously improve to reverse the trajectory, it is more likely that active intervention is needed to improve long-term resilience. Early steps taken to arrest further deterioration could be more practical and cost-effective than those that would be required later on (Selkoe et al., 2015).
Conclusions
Arresting the global decline of terrestrial mega-herbivores requires an understanding of what is driving trends, not only in terms of the external threats and their obvious effect on numerical abundance, but also their hidden impacts on critical vital rates. Animal populations can be resilient, but only within physiological limits (Lynch et al., 2014). Data on vital rates outside of captive or farmed conditions are scarce or nonexistent for many slow-breeding species. This must be overcome in order to manage populations appropriately. Attempting to rescue populations that are in reproductive collapse, no matter how valiant the effort, may be analogous to trying to change the course of a demographic train that has not only left the station but plunged off a cliff. In contrast, the declining population paradigm calls for diagnosing and acting on the drivers of decline. While tipping point assessments may be unnecessary for taxa with short generation times, explicitly defining these limits provides a basis for evaluating the health of populations even if data are imperfect, and encourages timely action that may be crucial for conserving large, long-lived species.
Author Contributions
SdS and PL conceived of the work. SdS designed and performed analyses and wrote the manuscript. PL guided analyses and edited the manuscript.
Conflict of Interest Statement
The authors declare that the research was conducted in the absence of any commercial or financial relationships that could be construed as a potential conflict of interest.
Acknowledgments
This work was supported by a James Smithson Fellowship to SdS at the Smithsonian Conservation Biology Institute as well as funding from the Ecology, Behavior, and Evolution Section of the Division of Biological Sciences at UC San Diego. SdS further wishes to thank John Ballou and Kathy Traylor-Holzer for advice on VORTEX specifications, as well as Philip Nyhus, John Jackson, and Sergey Kryazhimskiy for feedback on drafts of the manuscript.
Supplementary Material
The Supplementary Material for this article can be found online at: https://www.frontiersin.org/articles/10.3389/fevo.2019.00171/full#supplementary-material
References
Akçakaya, H. R., and Sjogren-Gulve, P. (2000). Population viability analyses in conservation planning: an overview. Ecol. Bull. 48, 9–21.
Armbruster, P., Fernando, P., and Lande, R. (1999). Time frames for population viability analysis of species with long generations: an example with Asian elephants. Anim. Conserv. 2, 69–73. doi: 10.1111/j.1469-1795.1999.tb00050.x
AsERM (2017). Asian elephant range states meeting (AsERSM) final report, 2017. Jakarta, Indonesia, 1–60.
Beissinger, S. R., and Westphal, M. I. (1998). On the use of demographic models of population viability in endangered species management. J. Wildl. Manag. 62, 821–841. doi: 10.2307/3802534
Bennett, E. L. (2014). Legal ivory trade in a corrupt world and its impact on African elephant populations. Conserv. Biol. 00, 1–7. doi: 10.1111/cobi.12377
Blake, S., and Hedges, S. (2004). Sinking the flagship: the case of forest elephants in Asia and Africa. Conserv. Biol. 18, 1191–1202. doi: 10.1111/j.1523-1739.2004.01860.x
Bonenfant, C., Gaillard, J. M., Coulson, T. I. M., Bianchet, M. F., Loison, A., Garel, M., et al. (2009). Empirical evidence of density-dependence in populations of large herbivores. Adv. Ecol. Res. 41, 313–357. doi: 10.1016/S0065-2504(09)00405-X
Boyce, M. S. (1992). Population viability analysis. Annu. Rev. Ecol. Syst. 23, 481–506. doi: 10.1146/annurev.es.23.110192.002405
Brook, B. W., Bradshaw, C. J. A., Traill, L. W., and Frankham, R. (2011). Minimum viable population size: not magic, but necessary. Trends Ecol. Evol. 26, 619–620. doi: 10.1016/j.tree.2011.09.006
Brook, B. W., O'Grady, J. J., Chapman, a, P., Burgman, M. A., Akçakaya, H. R., and Frankham, R. (2000). Predictive accuracy of population viability analysis in conservation biology. Nature 404, 385–387. doi: 10.1038/35006050
Brook, B. W., Traill, L. W., and Bradshaw, C. J. A. (2006). Minimum viable population sizes and global extinction risk are unrelated. Ecol. Lett. 9, 375–382. doi: 10.1111/j.1461-0248.2006.00883.x
Burdett, C. L., Crooks, K. R., Theobald, D. M., Wilson, K. R., Boydston, E. E., and Lyren, L. M., et al. (2010). Interfacing models of wildlife habitat and human development to predict the future distribution of puma habitat. Ecosphere 1, 1–21. doi: 10.1890/ES10-00005.1
Campos-Arceiz, A., and Blake, S. (2011). Megagardeners of the forest – the role of elephants in seed dispersal. Acta Oecol. 37, 542–553. doi: 10.1016/j.actao.2011.01.014
Cardillo, M., Mace, G. M., Jones, K. E., Bielby, J., Bininda-emonds, O. R. P., and Sechrest, W. (2005). Multiple causes of high extinction risk in large mammal species. Science 309, 1239–1241. doi: 10.1126/science.1116030
Caughley, G. (1994). Directions in conservation biology. J. Anim. Ecol. 63, 215–244. doi: 10.2307/5542
Chamaillé-jammes, S., Fritz, H., Valeix, M., Murindagomo, F., and Clobert, J. (2008). Resource variability, aggregation and direct density dependence in an open context: the local regulation of an African elephant population. J. Anim. Ecol. 77, 135–144. doi: 10.1111/j.1365-2656.2007.01307.x
Choudhury, A. L., Choudhury, D. K., Desai, A., Duckworth, J. W., Easa, P. S., and Johnsingh, A. J. T., et al. (2008). Elephas maximus. IUCN Red List Threat. Species. Available online at: http://www.iucnredlist.org/details/7140/0 (accessed January 23, 2017).
Coulson, T., Mace, G. M., Hudson, E., and Possingham, H. P. (2001). The use and abuse of population viability analysis. Trends Ecol. Evol. 16, 219–221. doi: 10.1016/S0169-5347(01)02137-1
Cross, P. C., and Beissinger, S. R. (2001). Using logistic regression to analyze the sensitivity of PVA models: a comparison of methods based on African wild dog models. Conserv. Biol. 15, 1335–1346. doi: 10.1046/j.1523-1739.2001.00031.x
Dai, L., Vorselen, D., Korolev, K. S., and Gore, J. (2012). Generic indicators for loss of resilience before a tipping point leading to population collapse. Science 336, 1175–1177. doi: 10.1126/science.1219805
de Silva, S. (2016). Need for longitudinal studies of Asian wildlife in the face of crises. Glob. Ecol. Conserv. 6, 276–285. doi: 10.1016/j.gecco.2016.03.010
de Silva, S., Ranjeewa, A. D. G., and Weerakoon, D. (2011). Demography of Asian elephants (Elephas maximus) at Uda Walawe National Park, Sri Lanka based on identified individuals. Biol. Conserv. 144, 1742–1752. doi: 10.1016/j.biocon.2011.03.011
de Silva, S., Webber, C. E., Weerathunga, U. S., Pushpakumara, T. V., Weerakoon, D. D. K., Wittemyer, G., et al. (2013). Demographic variables for wild Asian elephants using longitudinal observations. PLoS ONE 8:e82788. doi: 10.1371/journal.pone.0082788
Desai, A. A., and Riddle, H. S. (2015). Human-Elephant Conflict in Asia. Available online at: https://www.fws.gov/international/pdf/Human-Elephant-Conflict-in-Asia-June2015.pdf
Doughty, C. E., Roman, J., Faurby, S., Wolf, A., Haque, A., and Bakker, E. S., et al. (2016). Global nutrient transport in a world of giants. Proc. Natl. Acad. Sci. U.S.A. 113, 868–873. doi: 10.1073/pnas.1502549112
Drake, J. M., and Griffen, B. D. (2010). Early warning signals of extinction in deteriorating environments. Nature 467, 456–459. doi: 10.1038/nature09389
Faust, L. J., Thompson, S. D., and Earnhardt, J. M. (2006). Is reversing the decline of Asian elephants in North American zoos possible? An individual-based modeling approach. Zoo Biol. 25, 201–218. doi: 10.1002/zoo.20054
Fernando, P. (2015). Managing elephants in Sri Lanka: where we are and where we need to be. Ceylon J. Sci. 44, 1–11. doi: 10.4038/cjsbs.v44i1.7336
Fernando, P., and Pastorini, J. (2011). Range-wide status of Asian elephants. Gajah 35, 15–20. doi: 10.5167/uzh-59036
Flather, C. H., Hayward, G. D., Beissinger, S. R., and Stephens, P. A. (2011). Minimum viable populations: is there a “magic number” for conservation practitioners? Trends Ecol. Evol. 26, 307–316. doi: 10.1016/j.tree.2011.03.001
Flower, S. S. (1943). Notes on age at sexual maturity, gestation period and growth of the Indian elephant, Elephas maximus. J. Zool. 113, 21–26. doi: 10.1111/j.1096-3642.1943.tb00062.x
Freckleton, R. P., Watkinson, A. R., Green, R. E., William, J., Freckleton, R. P., and Watkinson, A. R., et al. (2006). Census error and the detection of density dependence. J. Anim. Ecol. 75, 837–851. doi: 10.1111/j.1365-2656.2006.01121.x
Gerber, L. R. (2006). Including behavioral data in demographic models improves estimates of population viability. Front. Ecol. Environ. 4, 419–427. doi: 10.1890/1540-9295(2006)4[419:IBDIDM]2.0.CO;2
Gopala, A., Hadian, O., Sunarto Sitompul, A., Williams, A., Leimgruber, P., et al. (2011). Elephas maximus ssp. sumatranus. IUCN Red List Threat. Species 2011, e.T199856A9129626.
Goswami, V., Vasudev, D., and Oli, M. K. (2014). The importance of conflict-induced mortality for conservation planning in areas of human–elephant co-occurrence. Biol. Conserv. 176, 191–198. doi: 10.1016/j.biocon.2014.05.026
Gough, K. F., and Kerley, G. I. H. (2006). Demography and population dynamics in the elephants Loxodonta africana of Addo Elephant National Park, South Africa: is there evidence of density dependent regulation? Oryx 40, 434–441. doi: 10.1017/S0030605306001189
Hance, J. (2018). The rhino reckoning. Mongabay. Available online at: https://news.mongabay.com/2018/10/the-rhino-reckoning/ (accessed October 2, 2018).
Haynes, G. (2012). Elephants (and extinct relatives) as earth-movers and ecosystem engineers. Geomorphology 157–158, 99–107. doi: 10.1016/j.geomorph.2011.04.045
Hayward, A. D., Mar, K. U., Lahdenperä, M., and Lummaa, V. (2014). Early reproductive investment, senescence and lifetime reproductive success in female Asian elephants. J. Evol. Biol. 27, 772–783. doi: 10.1111/jeb.12350
Hedges, S., Fisher, K., and Rose, R. (2008). Range-Wide Mapping Workshop for Asian Elephants (Elephas maximus). Available online at: https://www.fws.gov/international/pdf/report-asian-elephant-range-wide-mapping-workshop-2008.pdf
Hedges, S., Tyson, M. J., Sitompul, A. F., Kinnaird, M. F., and Gunaryadi, D. (2005). Distribution, status, and conservation needs of Asian elephants (Elephas maximus) in Lampung Province, Sumatra, Indonesia. Biol. Conserv. 124, 35–48. doi: 10.1016/j.biocon.2005.01.004
Heppell, S. S., Caswell, H., and Crowder, L. B. (2000). Life histories and elasticity patterns: perturbation analysis for species with minimal demographic data. 81, 654–665. doi: 10.1890/0012-9658(2000)081[0654:LHAEPP]2.0.CO;2
Hilbers, J. P., Santini, L., Visconti, P., Schipper, A. M., Pinto, C., Rondinini, C., et al. (2017). Setting population targets for mammals using body mass as a predictor of population persistence. Conserv. Biol. 31, 385–393. doi: 10.1111/cobi.12846
Holmes, E. E. (2001). Estimating risks in declining populations with poor data. Proc. Natl. Acad. Sci. U.S.A. 98, 5072–5077. doi: 10.1073/pnas.081055898
Hughes, T. P., Carpenter, S., Rockström, J., Scheffer, M., and Walker, B. (2013). Multiscale regime shifts and planetary boundaries. Trends Ecol. Evol. 28, 389–395. doi: 10.1016/j.tree.2013.05.019
Kurt, F. (1974). “Remarks on the social structure and ecology of the Ceylon elephant in the Yala National Park.,” in The Behaviour of Ungulates and Its Relation to Management, eds V. Geist and F. Walther (Morges: International Union for Conservation of Nature and Natural Resources), 618–634.
Kurt, F., Mar, K. U., and Garai, M. (2008). “Giants in chains: History, biology and preservation of Asian elephants in Asia.,” in Elephants and Ethics: Toward a Morality of Coexistence, eds C. Wemmer and C. Christen (Baltimore, MD: The John Hopkins University Press), 327–345.
Lahdenperä, M., Mar, K. U., and Lummaa, V. (2015). Short-term and delayed effects of mother death on calf mortality in Asian elephants. Behav. Ecol. 27, 166–174. doi: 10.1093/beheco/arv136
Leimgruber, P., Gagnon, J. B., Wemmer, C., Kelly, D. S., Songer, M. A., and Selig, E. R. (2003). Fragmentation of Asia's remaining wildlands: implications for Asian elephant conservation. Anim. Conserv. 6, 347–359. doi: 10.1017/S1367943003003421
Leimgruber, P., Senior, B., Aung, M., Songer, M. a, Mueller, T., et al. (2008). Modeling population viability of captive elephants in Myanmar (Burma): implications for wild populations. Anim. Conserv. 11, 198–205. doi: 10.1111/j.1469-1795.2008.00172.x
Lenton, T. M. (2011). Early warning of climate tipping points. Nat. Clim. Change 1, 201–209. doi: 10.1038/nclimate1143
Linklater, W. L. (2003). Science and management in a conservation crisis: a case study with rhinoceros. Conserv. Biol. 17, 968–975. doi: 10.1046/j.1523-1739.2003.01449.x
Lynch, H. J., Rhainds, M., Calabrese, J. M., Cantrell, S., Cosner, C., and Fagan, W. F. (2014). How climate extremes—not means—define a species' geographic range boundary via a demographic tipping point. Ecol. Monogr. 84, 131–149. doi: 10.1890/12-2235.1
Mace, G. M., Collar, N. J., Gaston, K. J., Hilton-Taylor, C., Akçakaya, H. R., and Leader-Williams, N., et al. (2008). Quantification of extinction risk: IUCN's system for classifying threatened species. Conserv. Biol. 22, 1424–1442. doi: 10.1111/j.1523-1739.2008.01044.x
Maguire, L., Seal, U., and Brussard, P. (1987). “Managing critically endangered species: the Sumatran rhino as a case study,” in Viable Populations for Conservation, ed M. Soulé (Cambridge: Cambridge University Press), 141–158. doi: 10.1017/CBO9780511623400.009
Malhi, Y., Doughty, C. E., Galetti, M., Smith, F. A., Svenning, J.-C., and Terborgh, J. W. (2016). Megafauna and ecosystem function from the Pleistocene to the Anthropocene. Proc. Natl. Acad. Sci. U.S.A. 113, 838–846. doi: 10.1073/pnas.1502540113
Manlik, O., Mcdonald, J. A., Mann, J., Raudino, H. C., Bejder, L., and Krützen, M., et al. (2016). The relative importance of reproduction and survival for the conservation of two dolphin populations. Ecol. Evol. 6, 3496–3512. doi: 10.1002/ece3.2130
Mar, K. U., Lahdenperä, M., and Lummaa, V. (2012). Causes and correlates of calf mortality in captive Asian elephants (Elephas maximus). PLoS ONE 7:e32335. doi: 10.1371/journal.pone.0032335
McCarthy, M. A., Burgman, M. A., and Ferson, S. (1995). Sensitivity analysis for models of population viability. Biol. Conserv. 73, 93–100. doi: 10.1016/0006-3207(95)90029-2
Mills, L. S., Doak, D. F., and Wisdom, M. J. (1999). Reliability of conservation actions based on elasticity analysis of matrix models. Conserv. Biol. 13, 815–829. doi: 10.1046/j.1523-1739.1999.98232.x
Morrison, C., Wardle, C., and Castley, J. G. (2016). Repeatability and reproducibility of population viability analysis (PVA) and the implications for threatened species management. Front. Ecol. Evol. 4:98. doi: 10.3389/fevo.2016.00098
Moßbrucker, A. M. (2016). Modeling the fate of Sumatran elephants in Bukit Tigapuluh Indonesia: research needs and implications for population management. J. Forest Sci. 10, 5–18. doi: 10.22146/jik.12622
Moßbrucker, A. M., Apriyana, I., Fickel, J., Imron, M. A., and Pudyatmoko, S. (2015). Non-invasive genotyping of Sumatran elephants: implications for conservation. Trop. Conserv. Sci. 8, 745–759. doi: 10.1177/194008291500800312
Mumby, H. S., Mar, K. U., Hayward, A. D., Htut, W., and Htut-aung, Y. (2015). Elephants born in the high stress season have faster reproductive ageing. Sci. Rep. 5:13946. doi: 10.1038/srep13946
Murray, K. A., Verde Arregoitia, L. D., Davidson, A., Di Marco, M., and Di Fonzo, M. M. I. (2014). Threat to the point: improving the value of comparative extinction risk analysis for conservation action. Glob. Change Biol. 20, 483–494. doi: 10.1111/gcb.12366
Nijman, V. (2014). An Assessment of the Live Elephant Trade in Thailand. Available online at: http://static1.1.sqspcdn.com/static/f/157301/25264217/1406803061440/Elephant-trade.pdf?token=1GF9PkcFJDL0/5fxcaSZufscUnY= (accessed July 19, 2016).
Nijman, V., and Shepherd, C. R. (2014). Emergence of Mong La on the Myanmar–China border as a global hub for the international trade in ivory and elephant parts. Biol. Conserv. 179, 17–22. doi: 10.1016/j.biocon.2014.08.010
Palkopoulou, E., Mallick, S., Skoglund, P., Enk, J., Rohland, N., Li, H., et al. (2015). Complete genomes reveal signatures of demographic and genetic declines in the woolly mammoth. Curr. Biol. 25, 1395–1400. doi: 10.1016/j.cub.2015.04.007
Pe'er, G., Matsinos, Y. G., Johst, K., Franz, K. W., Turlure, C., Radchuk, V., et al. (2013). A protocol for better design, application, and communication of population viability analyses. Conserv. Biol. 27, 644–656. doi: 10.1111/cobi.12076
Poole, J. H. (1987). Rutting behavior in elephants: the phenomenon of musth in African elephants. Anim. Behav. 102, 283–316. doi: 10.1163/156853986X00171
Purvis, A., Gittleman, J. L., Cowlishaw, G., and Mace, G. M. (2000). Predicting extinction risk in declining species. Proc. R. Soc. London B 267, 1947–1952. doi: 10.1098/rspb.2000.1234
Puyravaud, J. P., Davidar, P., Srivastava, R. K., and Wright, B. (2017). Modelling harvest of Asian elephants Elephas maximus on the basis of faulty assumptions promotes inappropriate management solutions. Oryx 51, 506–512. doi: 10.1017/S003060531600003X
Rabinowitz, A. (1995). Helping a species go extinct: the Sumatran rhino in Borneo. Conserv. Biol. 9, 482–488. doi: 10.1046/j.1523-1739.1995.09030482.x
Reed, D. H., O'Grady, J. J., Brook, B. W., Ballou, J. D., and Frankham, R. (2003). Estimates of minimum viable population sizes for vertebrates and factors influencing those estimates. Biol. Conserv. 113, 23–34. doi: 10.1016/S0006-3207(02)00346-4
Reed, J. M., Mills, L. S., Dunning, J. B., Menges, E. S., McKelvey, K. S., Frye, R., et al. (2002). Emerging issues in population viability analysis. Conserv. Biol. 16, 7–19. doi: 10.1046/j.1523-1739.2002.99419.x
Ripple, W. J., Chapron, G., López-bao, J. V., Durant, S. M., Macdonald, D. W., and Corlett, R. T., et al. (2016). Saving the world's terrestrial megafauna. Bioscience 66, 1–6. doi: 10.1093/biosci/biw092
Ripple, W. J., Newsome, T. M., Wolf, C., Dirzo, R., Everatt, K. T., and Hayward, M. W., et al. (2015). Collapse of the world's largest herbivores. Sci. Adv. 1:e1400103. doi: 10.1126/sciadv.1400103
Rogers, R. L., and Slatkin, M. (2017). Excess of genomic defects in a woolly mammoth on Wrangel Island. PLoS Genet. 13:e1006601. doi: 10.1371/journal.pgen.1006601
Sampson, C., Mcevoy, J., Oo, Z. M., Chit, A. M., Chan, N., Tonkyn, D., et al. (2018). New elephant crisis in Asia — early warning signs from Myanmar. PLoS ONE 13:e0194113. doi: 10.1371/journal.pone.0194113
Scheffer, M., Bascompte, J., Brock, W. A., Brovkin, V., Carpenter, S. R., Dakos, V., et al. (2009). Early-warning signals for critical transitions. Nature 461, 53–59. doi: 10.1038/nature08227
Selkoe, K. A., Blenckner, T., Caldwell, M. R., Larry, B., Erickson, A. L., and Essington, T. E., et al. (2015). Principles for managing marine ecosystems prone to tipping points. Ecosyst. Heal. Sust. 1, 1–18. doi: 10.1890/EHS14-0024.1
Shaffer, M., Watchman, L. H. Snape III, W. J., and Latchis, I. K. (2002). “Population viability analysis and conservation policy,” in Population Viability Analysis, eds S. R. Beissinger and D. R. McCullough (Chicago, IL: University of Chicaco Press), 123–146.
Shaffer, M. L. (1981). Minimum population sizes for species conservation. Bioscience 31, 131–134. doi: 10.2307/1308256
Traill, L. W., Brook, B. W., Frankham, R. R., and Bradshaw, C. J. A. (2010). Pragmatic population viability targets in a rapidly changing world. Biol. Conserv. 143, 28–34. doi: 10.1016/j.biocon.2009.09.001
Wasser, S., Poole, J., Lee, P., Lindsay, K., Dobson, A., Hart, J., et al. (2010). Elephants, ivory, and trade. Science 327, 1331–1332. doi: 10.1126/science.1187811
Watson, J. E. M., Jones, K. R., Fuller, R. A., Di Marco, M., Segan, D. B., and Butchart, S. H. M., et al. (2016). Persistent disparities between recent rates of habitat conversion and protection and implications for future global conservation targets. Conserv. Lett. 9, 413–421. doi: 10.1111/conl.12295
Wiese, R. J. (2000). Asian elephants are not self-sustaining in North America. Zoo Biol. 19, 299–309. doi: 10.1002/1098-2361(2000)19:5<299::AID-ZOO2>3.0.CO;2-Z
Keywords: mega-herbivores, elephant, population viability analysis, population dynamics, Elephas maximus, demographic safe space, extinction risk, alternative stable states
Citation: de Silva S and Leimgruber P (2019) Demographic Tipping Points as Early Indicators of Vulnerability for Slow-Breeding Megafaunal Populations. Front. Ecol. Evol. 7:171. doi: 10.3389/fevo.2019.00171
Received: 19 October 2018; Accepted: 29 April 2019;
Published: 17 May 2019.
Edited by:
Umesh Srinivasan, Princeton University, United StatesReviewed by:
Robert Justin Irvine, James Hutton Institute, United KingdomDevcharan Jathanna, Wildlife Conservation Society, India
Copyright © 2019 de Silva and Leimgruber. This is an open-access article distributed under the terms of the Creative Commons Attribution License (CC BY). The use, distribution or reproduction in other forums is permitted, provided the original author(s) and the copyright owner(s) are credited and that the original publication in this journal is cited, in accordance with accepted academic practice. No use, distribution or reproduction is permitted which does not comply with these terms.
*Correspondence: Shermin de Silva, U2hlcm1pbkB0cnVua3NubGVhdmVzLm9yZw==