- UMR LEHNA, CNRS, ENTPE, Université de Lyon, Lyon1, Villeurbanne, France
Movement, both within an individual's home range and at the scale of dispersal, is a fundamental aspect of an animal's life. The field of movement ecology has established a conceptual framework to analyze the lifetime movement of an organism, offering a sound basis for conservation actions since the movement range of many species has been altered by habitat fragmentation and degradation. An organism's lifetime movement is organized around three main functions—exploitation, exploration, and relocation—which are associated with specific behavioral mechanisms and spatio-temporal scales. The movement ecology framework is a valuable tool as applied to amphibians, as managing these spatially structured populations requires in-depth knowledge of the behavioral mechanisms that determine movement. In terms of exploitation, these animals have a complex lifecycle, which involves migrating between different types of habitat, thus requiring them to cross a landscape matrix that may be more or less inhospitable. In terms of exploration and relocation, between-pond movements within the pond archipelagoes of a given population are frequent and strongly contribute to population resilience. Relocation also occurs at a larger scale, through long-distance dispersal to colonize new patches, exposing the individuals to unknown environments. Each function, at each scale, involves specific interactions between individual motivation (phenotype dependence) and environmental quality (context dependence) that determine decision-making and fitness outputs. Long-term exposure to local selective pressures can lead to differentiation in coping types that could be considered as Evolutionarily Significant Units (ESUs) for conservation. At the scale of a patch, the optimal direction of migration can be inherited, thus allowing the optimization of migration routes for juveniles. At the regional scale, a dispersal syndrome resulting in a greater propensity for boldness and exploration could be a response to unpredictable breeding sites or the high benefits of colonizing a rich habitat. Greater knowledge about such behavioral adaptations to specific situations would allow more targeted development of conservation measures or help to stop the spread of invasive species. The evolutionary context of movement behavior is thus of primary interest in designing effective conservation actions in a changing world.
Introduction
Since the advent of agriculture 9,000 years ago, with all of its by-products (e.g., industry, urbanization, transportation networks, etc.), many landscapes around the world have been dramatically modified, converting wild ecosystems into artificial environments (agrosystems, urban areas, etc.). The use of fossil fuels as an energy source since the nineteenth century has significantly hastened this “artificialization,” leading to agricultural intensification (Mazzerole and Villard, 1999), expansion of urban areas (Hamer and McDonnell, 2008), and the construction of extensive transportation infrastructure (Fahrig et al., 1995). Unable to inhabit these artificial environments, most species are constrained to live in the remaining more or less wild fragments, suffering the effects of reduced population numbers, loss of genetic diversity, and increased extinction risks (Pereira et al., 2010).
Since high human densities often make it impossible to “rewild” areas by reversing the artificialization process, frequently the only way to mitigate the deleterious effects of habitat fragmentation is to maintain or enhance demographic flows between habitat patches. One of the major themes of conservation biology has been to explore ways these objectives could be reached, resulting in hundreds of studies in recent decades that address questions that require multidisciplinary integrative approaches (Fahrig and Merriam, 1985; Moilanen and Hanski, 1998; Gilbert-Norton et al., 2010; Baguette et al., 2013). Of these questions, the movements of animals across the landscape are central, and movement ecology has become a crucial field of investigation for conservation actions (Nathan et al., 2008; Fraser et al., 2018). One of the tools proposed by movement ecology is a multi-scale conceptual framework that connects movement processes with life histories, population dynamics, and functional outputs (Morales et al., 2010).
Amphibians are recognized as one of the animal groups most threatened by human-driven landscape modification, and their conservation is an urgent task (Houlahan et al., 2000; Beebee and Griffiths, 2005). There are many arguments for conserving amphibians based on the main values motivating biodiversity conservation (utility, amenity, functional, and intrinsic values) (Blaustein and Kiesecker, 2002). Pond-breeding amphibians are especially concerned by landscape threats, as their breeding habitats have historically been, and continue to be, destroyed because of lack of immediate economic profitability (Lehtinen and Galatowitsch, 1999; Becker et al., 2007). Their complex lifecycles also involve seasonal migrations between aquatic breeding habitats (i.e., ponds and wetlands) and terrestrial maintenance habitats (e.g., forests and moorlands) (Cushman, 2006). The success of these migrations depends on interactions between movement capacity (e.g., locomotion performance, orientation mechanisms, and stress management) and the harshness (e.g., aridity, toxicity, danger) of the landscape to be crossed. These interactions determine landscape connectivity (Joly et al., 2001), which in turn determines metapopulation functioning through dispersal flows between populations or the colonization of empty patches (Marsh and Trenham, 2008). As movement and connectivity are the core issues in pond-breeding amphibian conservation, the aim of this review is to focus on amphibian conservation in light of movement ecology. Since the work in this area by Sinsch (2014) and Pittman et al. (2014), numerous studies have been published that bring new insights, especially regarding amphibian responses to landscape fragmentation and dispersal syndromes.
Because of physiological and ecological specific features, amphibian fitness depends on physical and ecological characteristics of their life milieu, making them valuable indicators of anthropogenic modifications of the environment. Moreover, because they also are small predators, they can be used as umbrella species in the assessment of conservation actions for many taxonomic groups inhabiting wetlands and land/wetland ecotones (Joly and Morand, 1996). Humidity, heat, and predation are the primary factors that constrain amphibian movement. Amphibians rely on moisture because they are subject to permanent evaporative water loss and have to continuously replenish their water reserves by actively taking up water through the ventral skin (Shoemaker et al., 1992; Brunelli et al., 2007). This process is greatly impeded when the substrate water potential reaches high values. Heat influences amphibians because they are ectothermic, so external heat is necessary to enhance metabolism and movement. Finally, most amphibians are exposed to high predation pressure by reptiles, birds, and mammals due to their small body size. The response to predation, whatever its nature (the secretion of toxins or hiding strategies), has a cost that potentially impacts habitat use and movement potential (Winandy et al., 2017). Added to these constitutive factors, there are now a range of human-driven constraining factors, including the presence of pesticides, the expansion of new pathogens (e.g., Ranavirus, Batrachochytrium) and new predators (e.g., fish, crayfish, Xenopus), as well as increasing landscape fragmentation (Cushman, 2006; Swanson et al., 2018; Tornabene et al., 2018). Empirical studies on the movement ecology of amphibians are multiplying, taking advantage of technical progress in the miniaturization of tags and transmitters, the simplification of genetic tools, and the emergence of long-term monitoring data (Leskovar and Sinsch, 2005; Heard et al., 2012; Connette and Semlitsch, 2013; Ousterhout and Semlitsch, 2014).
In this review, after a short introduction to the theoretical framework of movement ecology, I will consider three spatial-temporal scales from that of exploitation activities corresponding to the familiar home range to that of exploration corresponding to information sampling around the home range to lastly that of long-distance relocation corresponding to dispersal. I will then consider the evolution of movement in response to selective pressures coming from anthropogenic modifications of the environment, from variation of personality frequencies to the emergency of dispersal syndromes at the population level. In a last section I will explore the use of this conceptual and empirical framework in amphibian conservation, from landscape management to reintroduction strategies.
Theoretical Framework of Movement Ecology
The lifetime movement of an individual is structured around three main activity types: exploitation, exploration, and relocation. These are defined by timing, distance amplitude, pathway characteristics (e.g., linear and angular speeds, sinuosity), behavioral mechanisms (e.g., orientation/navigation, informed or exploratory, reactive or proactive), and functional consequences (e.g., food acquisition, breeding success, avoidance of inbreeding, level of competition), which act as rewards validating or invalidating previous decisions (Nathan et al., 2008). Each movement type occurs at different spatial/temporal scales around which the lifetime functions of an individual are organized. Figure 1 proposes a template structured according to movement characteristics, from slow and sinuous to rapid and straight (Y-axis), and related spatial scale (X-axis). The spatial scales are from familiar home range to long-distance dispersal, including near relocation, exploration and migration. The movement linked to each animal's activity can be assigned a place on this template. Most paths can be distributed along an axis from slow and sinuous to fast and straight. Slow and sinuous paths are related to restricted area, usually corresponding to familiar home range, while fast and straight paths are related to long trips between different habitat patches, or dispersal. The functional significance of these characteristics will be detailed in the following sections. Figure 2 provides the conceptual framework of movement ecology, showing the interactions between behavioral factors (phenotype dependence) and environmental factors (context dependence) that determine activity types and the subsequent characteristics of associated movements, the plasticity of the system and its evolutionary potential.
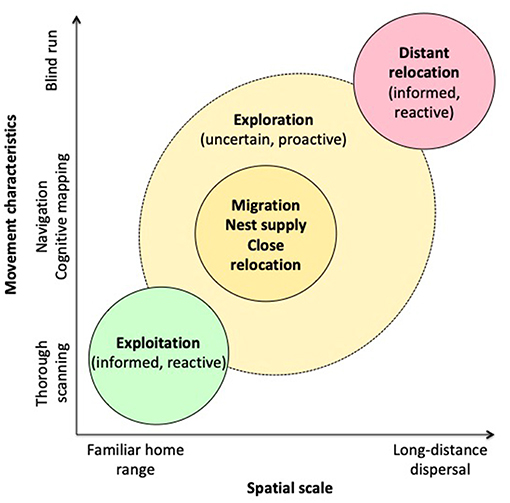
Figure 1. Relationships between movement characteristics and spatial scale for different types of activity in animals, based on the movement ecology concepts used in this paper. Movement characteristics are distributed along a gradient from slow and sinuous strongly organized by stimuli associated with resources (food, sexual partners, social partners, commodities) to more rapid and less sinuous movement organized by complex orientation mechanisms (navigation, cognitive map) associated with migration between habitat patches or exploration to lastly rapid and straight movement across unknown areas associated with dispersal and definitive long-distance relocation (emigration). X-axis gives the related spatial scales from familiar home range to long-distance dispersal. In species with habitat supplementation or complementation (patchy resources, complex lifecycles, exploitation of contrasted habitats), movements between habitat patches are governed by complex orientation mechanisms, such as navigation or cognitive mapping that reduce sinuosity and increase speed (migrations, nest supply). Exploratory movements have also intermediate characteristics since they are related to environment sampling usually for resources linked to homeostasis and they can lead to near relocation. They can be promoted by specific motivation (proactive behavior with latent learning).
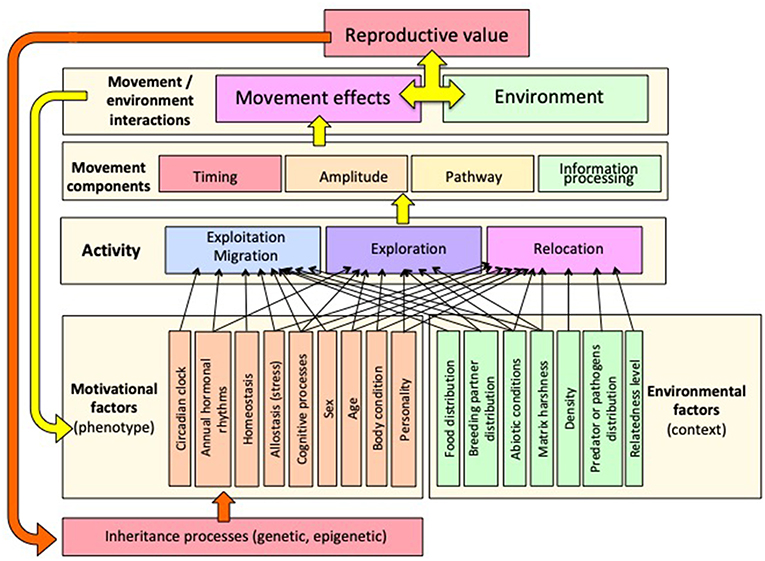
Figure 2. Conceptual framework of movement ecology, organized around a multi-scale typology (exploitation, exploration, and long-distance relocation—see Figure 1). A complex interplay between phenotype-dependent variables and environmental variables (context-dependence) determines activity type and movement characteristics. Movement is characterized through four basic components [timing, distance amplitude (spatial scale), pathway (speed and sinuosity), and information processing (orientation mechanisms)]. The yellow arrow expresses plasticity feedback at the scale of the individual, including learning processes. The orange arrow expresses the evolutionary feedback at an intergenerational scale based on selection or on epigenetic processes.
Exploitation Behavior
At the local scale of a familiar home range, movements obey a reactive mode, exhibiting high sinuosity and low linear speed, and correspond to exploitation activities, such as foraging, breeding, or ensuring safety or physiological comfort. The involved mechanisms are an interaction of motivations related to homeostasis, allostasis, or reproduction, and require the processing of information obtained by thorough environment scanning and saving processes. However, when resources are spread over large distances, patch exploitation can require individuals to cross more or less hostile environments, leading to direct movements from one resource patch to another (i.e., resource supplementation). In addition, annual cycles that alternate between cold and warm or dry and wet seasons can involve long migrations between regions with contrasting climatic conditions, resulting in multi-nuclear home ranges (this is the case for many butterflies, birds, bats, large herbivores, and whales, for example) (Holt and Fryxell, 2011). In species with complex lifecycles, larval habitats differ significantly from those occupied at the juvenile and adult stage, requiring seasonal migrations between these habitats (i.e., habitat complementation, which is found in anadromous fish and pond-breeding amphibians, for example; Pope et al., 2000). When migrating, the animals follow straight and fast movements, governed by more or less complex orientation mechanisms (navigation, cognitive maps, dead reckoning) (Wiltschko et al., 1981; Wallraff, 1983; Berthold, 1991; Able, 1993).
In pond-breeding amphibians, the pond is an essential site around which the population is organized. Ponds are often clustered in archipelagoes in which movements from one pond to another are frequent, leading to panmictic, multinuclear populations (Trenham et al., 2001; Petranka and Holbrook, 2006; Decout et al., 2012; Heard et al., 2012; Sinsch, 2014). A patch in this case consists of a set of ponds, terrestrial habitats for juveniles and adults, and wintering habitats, corresponding to the concept of habitat complementation (Pope et al., 2000). Both the aquatic and terrestrial habitats exhibit highly variable physical conditions, mainly due to climate and rainfall stochasticity. The ponds are usually in a process of ecological succession, and the degradation of the quality of these habitats selects for dispersal propensity. The terrestrial habitats are often forested, but some species prefer open environments, such as dunes, grasslands, steppes, or croplands (e.g., Acris blanchardi, Epidalea calamita, Anaxyrus americanus; Youngquist and Boone, 2014; Frei et al., 2016; Koumaris and Fahrig, 2016), while others show great flexibility in habitat use (e.g., R. sphenocephala, R. temporaria, B. terrestris; Vos et al., 2007; Graeter et al., 2008). Landscape structure and fragmentation have a direct influence on the costs of migration, and subsequently on individual fitness and the population growth rate. Migratory movements often follow a straight line (Ambystoma maculatum: Pittman and Semlitsch, 2013; Lithobates sylvaticus: Groff et al., 2017), but biased random walks or lateral movements have also been described in forests (Pittman and Semlitsch, 2013; Coster et al., 2014). The straightness of migratory movements is likely due to navigation mechanisms that rely on a combination of geomagnetic location and target-emanating olfactory cues, without any evidence of the use of a cognitive map (Rodda and Phillips, 1992; Joly and Miaud, 1993; Phillips, 1996; Sinsch, 2006; Diego-Rasilla et al., 2008; Landler and Gollmann, 2011). Tadpoles' learned knowledge of the different chemical characteristics of their natal pond could be used in later migratory movements, either positively in philopatric individuals, or negatively in dispersers (Ogurtsov and Bastakov, 2001). As a consequence of straight movements, the availability of terrestrial habitats could depend on the width of biological corridors; this hypothesis has been supported in European newts (Joly et al., 2001). A specific puzzle is that of juvenile migration, since juveniles have no experience of the route to take at the end of the metamorphosis process. Stevens et al. (2006) and Pittman and Semlitsch (2013) hypothesize that juveniles are primarily guided by aversion to water through a move-away process, until the individual reaches a favorable environment, where a random walk is adopted. However, recent studies have shown that juveniles also express an inherited migratory direction, thus improving migration success (Miaud et al., 2005; Janin, 2010).
The success of migration depends on movement costs when crossing a more or less hostile landscape matrix. This has been investigated through both simulation modeling and experimental approaches. In percolation models, the erosion of the migratory potential of an individual is related to the resistance costs of each type of land cover encountered along the migratory path, which is used to simulate friction maps and migration areas (Ray et al., 2002; Joly et al., 2003). In Janin et al. (2009), these resistance costs were estimated through a calibration-validation procedure, which identified urbanized areas and intensive farmland as the most resistant types of land cover for the common toad, Bufo bufo. In Decout et al. (2012), this approach was combined with graph theory to design functional patches at the regional scale. Experimental approaches can also provide empirical data that can be used in models. For example, measuring stress hormone levels demonstrated the harshness of a farmland matrix for common toads (Janin et al., 2011, 2012a), while other studies have revealed the negative influence of long travel distances (Marsh et al., 2004), desiccation risks (Mazzerole and Desrochers, 2005; Nowakowski et al., 2015), or unsuitable land cover, including cultivated crops and roads (Rittenhouse and Semlitsch, 2006; Consentino et al., 2011; Trochet et al., 2019). A key conclusion to draw from all these studies is that movements linked to habitat complementation at the scale of a patch are essential components of population persistence and are strongly impacted by multiple human-driven landscape modifications. When it is not possible to preserve a buffer zone of favorable terrestrial habitats around a pond (Semlitsch, 1997; McDonough and Patton, 2007), the best way to restore connectivity at this scale is to maintain a permeable matrix or straight-line corridors between essential habitat components (i.e., ponds, terrestrial habitats, and wintering habitats) (Groff et al., 2017). Stable corridors should serve to enhance the inheritance of migration direction, increasing connectivity effectiveness in the long term.
Exploration Behavior
The second movement type is exploration, in which individuals regularly move outside their usual exploitation range before finally returning to this familiar area, or exploit a new zone in the vicinity of the home range (near relocation). These movement pathways differ from those of exploitation activities, exhibiting reduced sinuosity and increased speed. They can be elicited in laboratory conditions; for instance, through complex maze experiments in which a well-fed rat creates a cognitive map of the maze without receiving any kind of reward when exploring it (the concept of latent learning, Tolman, 1948). This behavior is linked to a specific motivation in which the exploratory action itself, and the expected information gained along the journey, constitutes the reward. It is a proactive behavior (as opposed to a response to a stimulus) to cope with environmental uncertainty (Bartumeus et al., 2016).
Exploratory behavior has been deeply neglected in studies on amphibians. In the many recent studies on monitoring movement in the wild using telemetry, any movement is qualified as exploratory behavior, while the term itself is never used (Miaud et al., 2000; Muths, 2003; Indermaur et al., 2009; Constible et al., 2010; Heemeyer and Lannoo, 2012; Humphries and Sisson, 2012; Sinsch et al., 2012; Liang, 2013; Browne and Paszkowski, 2014; Timm et al., 2014; Frei et al., 2016; Groff et al., 2017; Pitt et al., 2017). As the main objectives of these studies were to establish the length of landward migrations, the size of the home range, and the components of terrestrial habitats, the movement characteristics themselves (e.g., the pathway or function) were most often not analyzed. Most studies have found adult individuals to be highly sedentary. However, it is known that individuals can move toward a new place in response to abiotic (e.g., humidity or flooding) or biotic (e.g., availability of prey) factors, without any previous exploration. In this case, near relocation results in the use of elementary orientation mechanisms, such as kineses or taxes, immediately followed by settlement, without any previous sampling of the environment (Fraenkel and Gunn, 1961). Movements from one pond to another within a pond archipelago could result from these elementary mechanisms.
Exploration is a complex behavior that is expected to contribute to fitness if the individual is able to memorize the information gained. Such storing of spatial information requires constructing a cognitive representation of the environment based on specific information processing. The question of the ability of lower vertebrates (such as fish and amphibians) to elaborate such cognitive maps is a subject of investigation. Some positive results have been obtained in fish using basic mazes (with 2 or 4 branches) (Rodriguez et al., 1994; Gomez-Laplaza and Gerlai, 2010), nevertheless there is no evidence that these results could be extrapolated to larger scales requiring more complex configurations to be memorized on the long term. In amphibians, exploratory behavior relies on the navigation mechanism involved and depends on the balance between the pay-offs of exploration, the costs of the neural mechanisms it requires, and the complexity (grain) of the landscape (Benhamou, 1997; Fagan et al., 2013). At present, no findings support the hypothesis of location-based navigation involving cognitive mapping and exocentric memory in pond-breeding amphibians, thus explaining the paucity of observations of exploratory behavior that returns to the starting point. However, location-based navigation seems to have evolved in species in which males carry the tadpoles from the ground to small waterbodies located in the forest canopy (Pasukonis et al., 2016), thus calling for more attention to be paid to navigation and memory processing in amphibian movement.
Dispersal
The third movement type occurs at a larger scale and corresponds to long-distance relocation or dispersal. This is exhibited when an individual breeds in a place that is different than that of its birth or of its previous breeding events (Ronce, 2007). In a lifetime, the frequency of this type of relocation behavior is usually low, except in nomadic individuals. It can be described as informed and reactive, since the individual decides to definitively leave the home range of its parents, which contains suitable resources, as attested by its own birth. This type of movement is typically rapid, extensive and ballistic (Bartumeus et al., 2016).
The term “philopatry” describes the absence of dispersal, i.e., when an individual breeds in the site where it was born. In all animal species, dispersal is expected to occur more frequently during the juvenile stage (natal dispersal) than during the adult stage (breeding dispersal) due to the absence of breeding costs and because juveniles have an immediate appraisal of the quality of their birthplace through their own body condition, which reflects current food availability and the level of competition. In iteroparous species, natal dispersal also allows the avoidance of kin competition, especially with parents (Harts et al., 2015). However, the costs of natal dispersal can outweigh these advantages if the small body size of juvenile individuals restricts their movement capacity, affords low physiological resilience, or exposes them to high predation risks. Moreover, as juveniles do not yet have knowledge of the environment to be crossed, dispersal behavior may involve simply moving away from the birthplace rather than following a pathway with a particular target. In contrast, adult (breeding) dispersal could benefit from the higher movement capacity of a larger body and a better knowledge of the location. Furthermore, in iteroparous species, it is hypothesized that adults would use dispersal as a strategy at an age when their own reproductive value becomes inferior to the sum of the reproductive value of their offspring (Morris, 1982). All these factors point to the coexistence of both natal and breeding dispersal in long-lived iteroparous species.
One of the results of dispersal is gene flows between populations (Baguette et al., 2013). By spreading advantageous alleles, gene flows contribute to a population's genetic diversity and adaptation. They also reduce the risks of inbreeding and genetic drift (Lowe and Allendorf, 2010). Additionally, dispersal has demographic consequences: for example, through rescue effects that could preserve a population from local extinction (Brown and Kodric-Brown, 1977). Unfortunately, obtaining empirical evidence of emigration and immigration rates to model the demographic functioning of spatially structured populations remains a difficult task (Lowe and Allendorf, 2010; Benton and Bowler, 2012). However, the increasing miniaturization of tags and radio transmitters, together with advances in modeling techniques, are improving the reliability of quantitative estimates of demographic flows (Cayuela et al., 2018a).
The dispersal process consists of three phases: departure, transfer, and settlement. Each involves different behavioral mechanisms (Ims and Yoccoz, 1997). Today we have substantial insights into departure decisions, which result from a number of interacting causes, from phenotype state (e.g., age, sex, physical condition, hormonal state, personality) to current environment quality (e.g., genetic relatedness with other residents, density, presence of predators or parasites, food shortage, degradation of the physical milieu) (see Matthysen, 2012 for a review) (Figure 2).
The transfer phase is the least understood, as it has not been described with sufficient accuracy to allow experimental investigation. Tracking dispersing individuals is a difficult task, firstly because it is impossible to forecast which individual will disperse and when the process will start. Secondly, because technical devices are lacking that allow small juvenile individuals to be tracked, which restricts monitoring to the identification at the arrival site by means of the recapture of marked individuals. While this gives insights into traveled distance and overall direction through the calculation of dispersal kernels (Nathan and Muller-Landau, 2000), it provides only indirect information about the route that has been followed and the behavioral mechanisms involved. If dispersal is considered a reactive process, the objective of which is to get away from the departure site, we would expect dispersal movement to follow a straight path that avoids any doubling back through random wandering (Selonen and Hanski, 2006). However, a “biased random walk” dispersal pathway is also a working hypothesis (Barton et al., 2009). Here an individual's indirect knowledge of the direction of potential target habitats is an important question. Airborne olfactory cues could inform an individual about a distant habitat patch and contribute to orienting dispersal movement. The direction of a distant patch could also be indicated by the arrival point of immigrating individuals, who may also provide information about the potential quality of the patch they departed from through their apparent body condition (Cote and Clobert, 2007). The transfer phase in dispersal would be expected to be highly costly, as an individual must cross unfamiliar and hostile environments where it may be exposed to predation risks and harsh physical conditions. However, empirical estimates of mortality during the transfer phase remain scanty.
The settlement phase, i.e., settling in the arrival site, assumes an evaluation of habitat quality by the disperser. Numerous studies have shown that the presence of conspecifics and/or their reproductive success provide reliable cues about habitat quality (Boulinier and Danchin, 1997; Stamps, 1998; Doligez et al., 2002). However, other characteristics of a niche (physical condition, food availability, absence of predators) can also attract disperser individuals, thus allowing the colonization of empty habitat patches (Le Galliard et al., 2005). Two behavioral phenotypes (pioneer and joiner) can coexist as a stable evolutionary strategy in the same population (Clobert et al., 2009). The strength of habitat selection for settlement is negatively influenced by the duration of the transfer phase, suggesting that increasing habitat fragmentation could lead to a decrease in the fitness of immigrants (Stamps et al., 2005).
Considering the three phases simultaneously is crucial, as the selective forces acting on each may diverge. Furthermore, the decision to emigrate is also likely to depend on tradeoffs between the necessary investment in mobility (e.g., energy reserves, limb length, muscle strength) and forthcoming fecundity. The form of this tradeoff is expected to interact with mortality risks during the transfer phase to determine a more or less safe movement pathway. When mobility costs are low and the distance to be covered is short, the emigration rate is expected to be high and movements tortuous (e.g., exploratory prospections), leading to density variation at the metapopulation level (Delgado et al., 2011; Travis et al., 2012). In contrast, when mortality risks during the transfer phase increase, movement is expected to follow a straighter, more direct path.
Behavioral Ecology of Dispersal in Amphibians
Philopatry and site fidelity have long been commonly accepted in amphibian biology (Twitty, 1959; Heusser, 1968). Site fidelity appears to be a statistical property of adult migration since many individuals return to the breeding site used the previous year, and this choice is deliberate (Joly and Miaud, 1989; Sinsch and Kirst, 2016). However, a non-negligible proportion of adult individuals do not exhibit site fidelity in homing experiments. Moreover, a detectable level of transience in mark–recapture (MR) studies confirms the reality of nomadism in natural populations, showing that breeding dispersal can be frequent (Perret et al., 2003; Muths et al., 2018). Dispersal rates vary between amphibian species and populations, from near zero to 50% (Reading et al., 1991; Hamer et al., 2008; Garwood, 2009; see Cayuela et al., 2018 submitted for review). The respective proportions of natal and breeding dispersal also vary greatly according to species and populations: natal dispersal dominates in Ranid frogs (Berven and Grudzien, 1990; Garwood, 2009), while the data is not consistent in other taxa. The distribution of dispersal distance is strongly skewed, with many species moving very long distances—up to 16 km in some anurans (Smith and Green, 2005) (Table 1). Indeed, long-distance dispersal is probably more frequent than currently observed, since we lack the methodological means to detect it. For instance, while the longest movement that has been observed in the natterjack toad is 4.4 km, the estimated dispersal distance based on a leptokurtic dispersal function is 12.2 km: a distance that is in line with estimated gene flows in this species (Oromi et al., 2012; Sinsch et al., 2012).
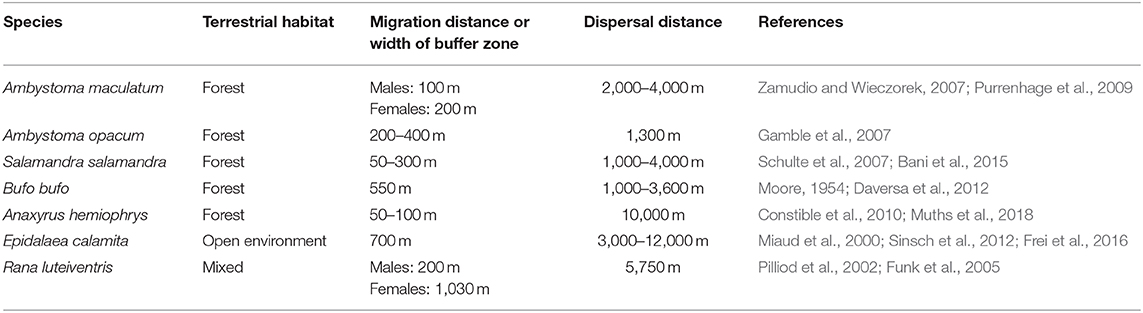
Table 1. Some examples of the distance differences between migration (intrapopulation round-trip movements to and away from breeding sites) and dispersal (inter-population one-way movements from a birthplace to a breeding site) in selected amphibians.
In contrast to most dispersal studies for other animal groups, the departure phase of amphibian movement has not received detailed attention. Findings from the studies that have been done show that the emigration of adult individuals is negatively related to density; individuals leave less-populated sites in favor of the most-populated ones (Gamble et al., 2007; Cayuela et al., 2019). The influence of sex on dispersal is not consistent, varying between species and populations (Cayuela et al. submitted). Little is known about the influence of kin competition and inbreeding on emigration propensity, aside from the recognized ability of kin recognition (Blaustein and Waldman, 1992; Masters and Forester, 1995). Dispersing individuals are often larger than residents (Denoël et al., 2018) and can exhibit a morphology adapted to movement, with longer forelimbs (in a salamander: Lowe and McPeek, 2012) or longer hindlimbs (in a toad: Phillips et al., 2006; Hudson et al., 2016). Their survival is higher than that of resident individuals (Lowe, 2009, 2010; Denoël et al., 2018). It is likely that dispersers also differ from residents in personality traits. In Australia's cane toad (Rhinella marina) invasion, dispersers at the front lines are more aggressive and less social than residents, and exhibit more exploratory and risk-prone behavior (Fogarty et al., 2011; Gruber et al., 2017). Despite a relative paucity of data, these results converge with the body of knowledge that has been established for other groups (Clobert et al., 2012).
The transfer phase has received more attention, probably because moving across the landscape matrix is a crucial topic in conservation biology. However, dispersal behavior, especially natal dispersal, has never been observed and monitored, so we have very little data about the followed pathways and the orientation processes involved. Most knowledge in this area relies on the analysis of gene flows or recapture data that indirectly provides information about movement pathways (Rowe et al., 2000). For example, Mark-Recapture data suggests that in the boreal toad, breeding dispersers follow straight pathways (Muths et al., 2018). As predicted by the theory of population genetics, the isolation of amphibian populations decreases genetic diversity as a result of gene flow impediment, genetic drift, and inbreeding when the effective population size is small (Andersen et al., 2004; Broquet et al., 2010; Rhoads et al., 2017). Several studies have established a negative relationship between gene flow and the resistance of the landscape matrix, which is related to agricultural intensification and the expansion of urbanization (Stevens et al., 2006; Van Buskirk, 2012; Crawford et al., 2016; Sawatzky et al., 2019). The barrier role of highways varies depending on the studied species, the age of the infrastructure, and, probably, the density of tunnels under the roadway. While highways or high-speed railways were not found to affect the genetic structure of Alpine newt populations (Prunier et al., 2014; Luqman et al., 2018), they did impact gene flows in the cricket frog and the Japanese brown frog (Youngquist et al., 2017; Kobayashi et al., 2018). Large rivers or concrete-banked waterways can act as barriers to gene flows in some species (Ambystoma maculatum, Rana japonica), but not in others (Hyla arborea, Ichtyosaura alpestris, Lithobates sylvaticus) (Angelone et al., 2011; Richardson, 2012; Kobayashi et al., 2018; Luqman et al., 2018). While valuable, this indirect evidence of large-scale movements provides imperfect information about the behavioral mechanisms involved in the transfer phase, which is of crucial importance in restoring connectivity. This is especially vital for the design of underpasses that allow the safe crossing of transportation infrastructures (Jarvis et al., 2019).
During the settlement phase, studies have shown that the presence of conspecifics can exert an attractive influence (Aragon et al., 2000). In the few species for which this question has been addressed, immigrants were attracted to sites occupied by a large population (Ambystoma opacum: Gamble et al., 2007; Litorea aurea: Bower et al., 2013; Triturus cristatus: Cayuela et al., 2019). Attraction by conspecifics could be related to Allee effects, such as sharing public information about food location (Martin and Caillère, 1982; Aragon, 2009) or finding sexual partners. Yet if kin are present, recognized by olfactory cues, this may act as a repellent (Ogurtsov, 2004).
The influence of heterospecifics on settlement is more debatable (Buxton and Sperry, 2017). Great crested newts select ponds where the density of both Alpine and palmate newts is high (Cayuela et al., 2018b). Newts can also be attracted by auditory cues from toads and frogs (Diego-Rasilla and Luengo, 2004; Pupin et al., 2007; Madden and Jehle, 2017). Anurans often use chorus calls as an attractor (Gerhardt and Klump, 1988; Swanson et al., 2007). Dispersers are also attracted by non-social cues, since newly created ponds devoid of any amphibians are rapidly colonized (Joly and Grolet, 1996; Baker and Halliday, 1999; Rannap et al., 2009; Weinbach et al., 2018). To add further complexity, these non-social cues can be specific to the local population (Sanuy and Joly, 2009). However, these results concern adult individuals that are typically involved in migration or breeding dispersal. No studies have focused on natal dispersal, probably because marking small individuals remains difficult, and low recapture probability entails a lot of fieldwork effort for uncertain results.
Evolution of Dispersal and Dispersal Syndromes
Each of the three phases of the dispersal process is under strong selective pressure due to the variability of local environmental and global environmental changes. As dispersal is determined by a combination of phenotype-dependent and context-dependent causes, the selection targets are multiple, and one might expect that changes in one element of the phenotype involve changes in the others. However, the overall evolutionary challenge is to predict the propensity of leaving the natal population. A first causation lies in phenotypic plasticity, which would confer to each individual a similar capacity to respond to habitat cues (Stearns and Koella, 1986; Via, 1993; Massot and Clobert, 2000; Tufto, 2000). In fact, several studies have shown that individuals differ in their motivation to leave a site, and that dispersal behavior could be predicted by particular phenotype traits (Bowler and Benton, 2005; Börger et al., 2008; Sih and Bell, 2008; Cote et al., 2010a). The concept of dispersal syndrome has been developed to establish a framework linking several internal-state components, such as physiology, behavior, morphology, and life history—in order to identify different phenotypes with respect to dispersal propensity (Careau et al., 2008; Clobert et al., 2009; Careau, 2012). A behavioral syndrome is a suite of correlated behaviors across situations (Boissy, 1995). It becomes an evolutionary solution to environmental variability when plasticity reaches its functional limits (Sih et al., 2004). Individual personality can be characterized according to five behavioral gradients: boldness, exploration, activity, sociability and aggression (Réale et al., 2010). From these, two fundamental coping styles have been detected in fish and birds: a proactive behavioral style characterized by aggressiveness, boldness, exploratory propensity, dispersal propensity, and mating success, and a reactive style characterized by shyness, neophobia, and an ability to adjust to the current environment. These coping styles are heritable and could influence population differentiation (Drent et al., 2003; Dochtermann et al., 2014). Behavioral styles have profound ecological implications on a range of outcomes—from population performance to biotic interactions—that could determine colonization ability and adaptation to human-modified environments (Sih et al., 2012). Different personality types can coexist in a population, with individuals expressing consistent differences in behavior, or suites of behaviors (functional types), and plasticity (Stamps, 2004, 2016; Dingemanse and Wolf, 2010). From an evolutionary perspective, different personalities can coexist when the fitness payoffs of each type depend on the frequency of the competing strategies—a result of frequency-dependent selection (Dall et al., 2004). This explanation is fairly well supported by empirical observation, suggesting a tradeoff along a boldness/shyness gradient (Smith and Blumstein, 2008; Stamps and Groothuis, 2010). The coexistence of several personalities within a population could thus contribute to the species' ability to persist. Further empirical investigation on the evolution of the personality spectrum under the selective pressures of habitat fragmentation would be valuable. Lastly, within the field of movement ecology, recent advances in the modeling of dispersal evolution need to be incorporated at the population level.
The dispersal syndrome concept assumes genetic correlations between traits through linkage disequilibrium or the pleiotropic effects of genes linked with dispersal (Saastamoinen et al., 2018). At the scale of personality, the traits related to dispersal propensity are aggressiveness, low sociability, low neophobia, readiness to explore, risk proneness, and boldness (Ronce and Clobert, 2012). The coexistence of two contrasting dispersal personalities within a population could be enhanced by habitat fragmentation, which concurrently increases both dispersal costs and inbreeding risks (Cote et al., 2010b, 2017; Sih et al., 2012). Looking for other correlations with life-history traits, large-scale meta-analyses have found a positive association of dispersal with early maturation and longevity, as well as a trend with fecundity (Stevens et al., 2014; Comte and Olden, 2018). Whereas, dispersal distance is positively related to body size, dispersal willingness is higher in generalist than in specialist species. High investment in reproduction (e.g., parental care, large eggs) is negatively related to dispersal propensity, identifying a conflict in resource allocation between dispersal and reproduction.
The coexistence of contrasting personalities could also act as a safeguard against habitat fragmentation, affording a population greater capacity to mitigate an increase in dispersal costs. Bold individuals can act as keystones, ensuring long-distance dispersal, colonizing new habitat patches, or gathering information about food distribution (Sih et al., 2012). A directional selection toward one coping style could induce a behavioral syndrome at the population level; a step toward local adaptation and the emergence of an ecotype, which may become an Evolutionarily Significant Unit for conservation (ESU). In contrast, directional selection could weaken the resilience of a population by leading to the impoverishment of personality diversity, restricting the population's ability to cope with temporal environmental variation and/or local habitat conditions (Sih et al., 2012; Cornelius et al., 2017).
As in other animal groups, amphibians exhibit variation in individual personality types (see Kelleher et al., 2018 for a review of this topic). Tadpoles vary in boldness and activity, characteristics that can be correlated (Koprivnikar et al., 2012; Wilson and Krause, 2012; Brodin et al., 2013; Urszan et al., 2015). Juveniles and adults also show differences in personality traits. Juvenile Epidalea calamita toads exhibit correlations between activity, movement speed, and exploratory behavior related to their ability to use corridors (Maes et al., 2012). In the invasive cane toad in Australia, individuals occur along a shyness/boldness gradient, with the more social being on the shy side (Gonzalez-Bernal et al., 2014). Xenopus tropicalis captured in the wild show strong differences in exploration intensity, though without any clear correlation to activity rate or morphology (Videlier et al., 2015).
In amphibians, dispersal propensity and dispersal distance are thought to quickly evolve under the selective pressures of habitat availability and/or habitat fragmentation (Maes et al., 2012; Wilson and Krause, 2012; Kelleher et al., 2017). In Australia, the cane toad invasion has provided researchers with a mine of knowledge regarding the rapid evolution of the phenotype of a disperser at an invasion front. Compared to individuals at the back, toads in the front lines have longer hindlimbs, which allows them to move farther and faster. Moreover, they move for twice as long and follow more direct pathways, proving to be more exploratory and risk-prone, and less social (Phillips et al., 2006; Fogarty et al., 2011; Lindström et al., 2013; Hudson et al., 2016; Gruber et al., 2017). This well-documented invasion process shows how efficiently dispersal traits can evolve: they are inheritable and can accelerate the rate of dispersal by a factor of five (Phillips et al., 2010).
Habitat fragmentation and disturbance also exert selective pressures that can promote or inhibit dispersal depending on the cost–benefit tradeoff. Mean activity rate, exploratory propensity, and boldness can vary between populations. In the common frog (Rana temporaria), individuals from island populations (newly formed islands) exhibit more boldness and higher activity than individuals in mainland populations, probably as a result of founder effects when the islands have been colonized (Brodin et al., 2013). In contrast, salamanders that inhabit stands of old forest are more active than those that inhabit young stands (Consentino and Droney, 2016). When reared in the same garden, post-metamorphic toadlets from highly fragmented landscapes exhibit more risk-prone and exploratory behavior than toadlets from less-fragmented landscapes (Janin et al., 2012b). A comparison of populations of Bombina variegata breeding in forests with those breeding along riverbanks detected a dispersal syndrome in the forest-dwellers characterized by a high dispersal rate, high fecundity, and a short lifespan, probably linked to the unpredictability caused by logging activities (Cayuela et al., 2016). Similarly, fire salamanders have been found to respond to pond instability by exhibiting higher activity rates and dispersing farther distances than those breeding in more stable small streams (Hendrix et al., 2017).
Studies focusing on personality have been clearly overlooked in amphibians as compared to other vertebrate groups (Kelleher et al., 2018) and very little attention has been paid to the sociality and aggression gradient. The personality spectrum could have implications in the spread of pathogenic agents if behavior influences the number of contacts between individuals. However, results regarding this are contradictory since while social individuals are more susceptible to transmitting a disease to other individuals, the boldest individuals are more susceptible to spreading it over long distances (Koprivnikar et al., 2012; Araujo et al., 2016). Given contrasting observations, the role of personality gradients in the spatial use of patchy habitats is an important avenue to explore: the consequences of this polymorphism on the functioning of amphibian populations are far from understood. Further research into these topics would be valuable in understanding the problems linked to habitat fragmentation.
Movement Ecology and Amphibian Conservation
For a given population, all the resources needed for breeding success are found in the habitat, which has a geographical structure. Land cover types that do not provide sufficient resources for each niche component are non-habitat areas, which make up the landscape matrix. The influence of this landscape matrix on functional connectivity has received much attention in ecology (Fahrig and Merriam, 1985; Moilanen and Hanski, 1998). A given matrix is composed of land cover with contrasting impacts on animal movement, from mildly resistant to impassable. Together with the risks taken when dispersing, land cover modulates the costs of movement at each step of the lifetime movement path. At the scale of a habitat patch, both resource supplementation and complementation processes drive movements across the landscape matrix (Pope et al., 2000). At a larger scale, movements are prospective and exploratory. If the environment is homogenous or fine-grained, these movements can lead to short-distance dispersal, resulting in a patchy population with high inertia (Harrison, 1991; Delgado et al., 2011). In contrast, if the environment is hostile or coarse-grained, movements from one patch to another result in long-distance dispersal with high mortality risks, which selects for straight pathways and high movement capacity (Travis et al., 2012; Cheptou et al., 2017). Fragmentation leads to reduced local population sizes, thus altering fitness by impeding Allee effects and increasing both genetic drift and inbreeding risks. When fragmentation dynamics are slow, these processes may be compensated by an increase in dispersal capacity (Thomas, 2000; Hanski et al., 2004). However, human-driven fragmentation is often a rapid process, characterized by the introduction of harsh landscape matrices (e.g., intensive agriculture or urbanization) that dramatically impede dispersal movements. As a consequence, the most dispersive individuals are counter-selected, driving the population toward the dominance of sedentary individuals (Massol et al., 2011). Genetic drift and inbreeding can then transitorily induce local differentiation that may promote local adaptation. However, the resulting decrease in evolutionary potential weakens the population's capacity to respond to environmental changes, thus increasing extinction risks. It is thus crucial to maintain a degree of connectivity to promote polymorphism through balanced fitness gains between disperser and resident phenotypes (Mathias et al., 2001). Empirical evaluations of this conceptual framework are nevertheless inconsistent and scanty, calling for further investigation. In the leaf beetle Phaedon cochleariae, for example, inbreeding is related to a bold personality, suggesting that risk-proneness may be promoted by a decline in reproductive value (Müller and Juskauskas, 2018).
Evaluating the impact of the matrix on movements is challenging due to technical limitations in direct movement tracking. Most knowledge comes from analyses of occurrence data and gene flows (Zeller et al., 2012) with a strong bias toward dispersal processes (Cosgrove et al., 2018). Different modeling options respond to different questions. Least-cost path analysis, graph theory and circuit analysis (e.g., Circuitscape) allow connectivity to be modeled and are usually carried out with gene flow data (Stevens et al., 2006; McRae and Beier, 2007; Foltête et al., 2012). However, these techniques are not based on behaviorally realistic rules, whereas individual-based simulation models, especially stochastic movement simulators, allow the integration of decision-making rules based on the perceptual range of the species. This enables them to draw powerful predictions (Knowlton and Graham, 2010; Palmer et al., 2011; Coulon et al., 2015). However, for a great majority of species, there is insufficient knowledge about movement mechanisms to effectively inform these models with realistic rules. It would also be valuable to integrate the increasing knowledge concerning the personality spectrum within a population in modeling procedures to improve prediction reliability (Fogarty et al., 2011; Chapple et al., 2012; Hirsch et al., 2017).
Understanding amphibian movement ecology is critical in developing effective conservation measures. These movements can be organized according to a spatial-temporal framework (Figure 3). At the scale of a patch, knowledge about habitat complementation processes used by individuals to seek resources would help to ensure functional connectivity, from the design of corridors to the mitigation of the barrier role of roads and railways with underpass technology (Bain et al., 2017). Safeguarding connectivity requires a better understanding of the orientation mechanisms involved in migration, and the potential inheritance of migration direction in juveniles. The putative negative influence of pesticides on orientation mechanisms must also be investigated (Janin, 2010).
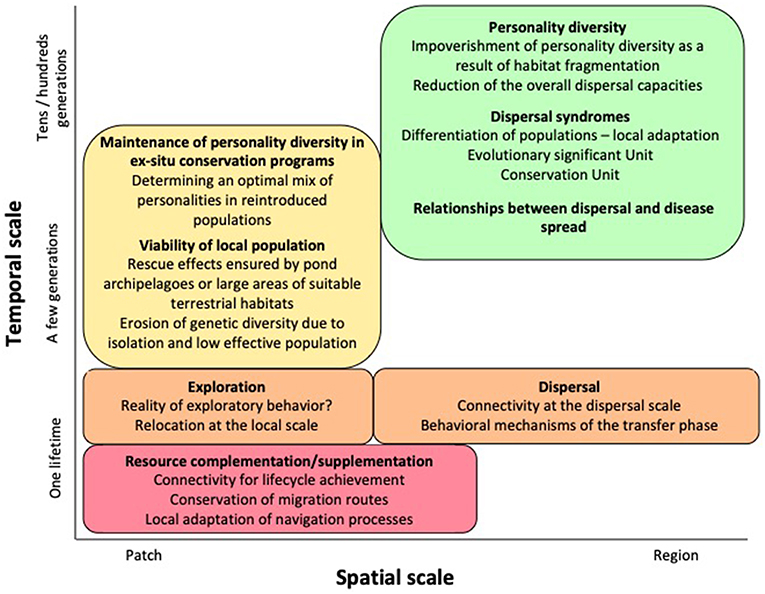
Figure 3. Aspects of amphibian movement ecology at different spatial and temporal scales that need to be better understood for conservation planning.
At the scale of a population, it is important to find the optimal configuration of pond archipelagoes that ensures the greatest viability and resilience through rescue effects and spreading the risk of extinction, especially in the context of climate change (Petranka and Holbrook, 2006; Weinbach et al., 2018). The great disparity of demographic responses to climatic variation calls for detailed investigations into the relationships between pace of life, movement ecology, and local adaptation to improve the predictive power of population models (Cayuela et al., 2017; Muths et al., 2017). Allee effects can explain the positive dependence on density of adult amphibians for fitness components, such as sexual selection or information sharing (Cayuela et al., 2019). In metapopulations, these effects can drive a population toward extinction (“evolutionary suicide”) unless these dynamics are counterbalanced by juvenile dispersal (Parvinen, 2007; Fowler, 2009). The knowledge juveniles have of a pond's current breeding conditions could promote such a compensatory process, but this cost–benefit tradeoff in breeding aggregations remains to be tested.
At the scale of a landscape, we do not know if the rules governing longer-distance dispersal movement could be extrapolated from knowledge regarding habitat complementation since the causes and functions of these two processes differ significantly. The fact that individuals, populations and species respond inconsistently to landscape components highlights the need for coupling an analysis of movement determinants with life histories, habitat preferences and the personality spectrum at different scales (Koumaris and Fahrig, 2016).
Another important issue for conservation is the assessment of the role of dispersal syndromes in local adaptation, including in response to human-modified landscapes, to test the hypothesis of Evolutionarily Significant Units and how to conserve these (Neal et al., 2018; Saastamoinen et al., 2018). While the conceptual framework of personality has opened the way to experimental approaches, approaches are also needed at the scale of dispersal to examine the determinants of emigration. This task is difficult since dispersal is likely to cover a bimodal distribution of traveled distances (exploration-based vs. long-distance dispersal), themselves expressing different behavioral processes.
In captive-breeding and reintroduction programs, a functional assessment of the personality spectrum should be a priority. By constraining captive individuals to live within restricted spaces, breeding programs may select for the most reactive individuals, leading to a deficit of dispersers in the reintroduced population (McDougall et al., 2006; Kelleher et al., 2018). This possibility makes it crucial to develop rearing methods that maintain an optimal mix of personalities in captive-breeding programs.
Greater knowledge of how amphibians move across landscapes is of critical interest for conservation biology. This information would allow demographic flows at the landscape scale to be modeled using graph theory (Foltête et al., 2014; Clauzel et al., 2015; Peterman et al., 2018) or individual-based modeling (Coulon et al., 2015). Movement ecology, by considering animal movement at different spatial and temporal scales, is an important step in improving this knowledge. The theoretical framework it has developed for assessing the distribution of biodiversity in changing landscapes is a sound basis for planning future studies and conservation actions.
Author Contributions
The author confirms being the sole contributor of this work and has approved it for publication.
Conflict of Interest Statement
The author declares that the research was conducted in the absence of any commercial or financial relationships that could be construed as a potential conflict of interest.
Acknowledgments
This study received funding from the Institut Universitaire de France. I thank two referees for their helpful comments that have allowed to significantly improving the manuscript.
References
Able, K. P. (1993). Orientation cues used by migratory birds: a review of cue-conflict experiments. TREE 10, 367–371. doi: 10.1016/0169-5347(93)90221-A
Andersen, L. W., Fog, K., and Damgaard, C. (2004). Habitat fragmentation causes bottlenecks and inbreeding in the European treefrog (Hyla arborea). Proc. R. Soc. B 271, 1293–1302. doi: 10.1098/rspb.2004.2720
Angelone, S., Kienast, F., and Holderegger, R. (2011). Where movement happens: scale-dependent landscape effects ongenetic differentiation in the European tree frog. Ecography 34, 714–722. doi: 10.1111/j.1600-0587.2010.06494.x
Aragon, P. (2009). Sex-dependent use of information on conspecific feeding activities in an amphibian urodelian. Funct. Ecol. 23, 380–388. doi: 10.1111/j.1365-2435.2008.01519.x
Aragon, P., Lopez, P., and Martin, J. (2000). Conspecific chemical cues influence pond selection by male newts Triturus boscai. Copeia 2000, 874–878. doi: 10.1643/0045-8511(2000)000[0874:CCCIPS]2.0.CO;2
Araujo, A., Kirschman, L., and Warne, R. W. (2016). Behavioural phenotypes predict disease susceptibility and infectiousness. Biol. Lett. 12:20160480. doi: 10.1098/rsbl.2016.0480
Baguette, M., Blanchet, S., Legrand, D., Stevens, V. M., and Turlure, C. (2013). Individual dispersal, landscape connectivity and ecological networks. Biol. Rev. 88, 310–326. doi: 10.1111/brv.12000
Bain, T. K., Cook, D. G., and Girman, D. J. (2017). Evaluating the effects of abiotic and biotic factors on movement through wildlife crossing tunnels during migration of the California tiger salamander, Ambystoma californiense. Herp. Conserv. Biol. 12, 192–201.
Baker, J. M. R., and Halliday, T. R. (1999). Amphibian colonization of new ponds in an agricultural landscape. Herp. J. 9, 55–63.
Bani, L., Pisa, G., Luppi, M., Spilotros, G., Fabbri, E., Randi, E., et al. (2015). Ecological connectivity assessment in a strongly structured fire salamander (Salamandra salamandra) population. Ecol. Evol. 5, 3472–3485. doi: 10.1002/ece3.1617
Barton, K. A., Phillips, B. I., Morales, J. M., and Travis, J. M. J. (2009). The evolution of an ‘intelligent' dispersal strategy: biased correlated random walk. Oikos 118:309–319. doi: 10.1111/j.1600-0706.2008.16936.x
Bartumeus, F., Campos, D., Ryu, W. S., Lloret-Cabot, R., Mendez, V., and Catalan, J. (2016). Foraging success under uncertainty: search tradeoffs and optimal space use. Ecol. Lett. 19, 1299–1313. doi: 10.1111/ele.12660
Becker, C. G., Fonseca, C. R., Haddad, C. F. B., Batista, R. F., and Prado, P. I. (2007). Habitat split and the global decline of amphibians. Science 318, 1775–1777. doi: 10.1126/science.1149374
Beebee, T. J. C., and Griffiths, R. A. (2005). The amphibian decline crisis: a watershed for conservation biology? Biol. Conserv. 125, 271–285. doi: 10.1016/j.biocon.2005.04.009
Benhamou, S. (1997). On systems of reference involved in spatial memory. Behav. Proc. 40, 149–163. doi: 10.1016/S0376-6357(97)00775-4
Benton, T. G., and Bowler, D. E. (2012). “Linking dispersal to spatial dynamics,” in Dispersal Ecology and Evolution, eds J. Clobert, M. Baguette, T. G. Benton, and J. M. Bullock (Oxford: Oxford UP), 251–265.
Berthold, P. (1991). Genetic control of migratory behaviour in birds. TREE 6, 254–257. doi: 10.1016/0169-5347(91)90072-6
Berven, K. A., and Grudzien, T. A. (1990). Dispersal in the wood frog (Rana sylvatica): implications for genetic population structure. Evolution 44, 2047–2056. doi: 10.1111/j.1558-5646.1990.tb04310.x
Blaustein, A. R., and Kiesecker, J. M. (2002). Complexity in conservation: lessons from the global decline of amphibian populations. Ecol. Lett. 5, 597–608. doi: 10.1046/j.1461-0248.2002.00352.x
Blaustein, A. R., and Waldman, B. (1992). Kin recognition in anurans. Anim. Behav. 44, 207–221. doi: 10.1016/0003-3472(92)90027-7
Boissy, A. (1995). Fear and fearfulness in animals. Quart. Rev. Biol. 70, 165–191. doi: 10.1086/418981
Börger, L., Datziel, B. D., and Fryxell, J. M. (2008). Are there general mechanisms of animal home range behavior? A review and prospects for future research. Ecol. Lett. 11, 637–650. doi: 10.1111/j.1461-0248.2008.01182.x
Boulinier, T., and Danchin, E. (1997). The use of conspecific reproductive success for breeding patch selection in terrestrial migratory species. Evol. Ecol. 11, 505–517. doi: 10.1007/s10682-997-1507-0
Bower, D. S., Stockwell, M. P., Pollard, C. J., Pickett, E. J., Garnham, J. I., Clulow, J., et al. (2013). Life stage specific variation in the occupancy of ponds by Litoria aurea, a threatened amphibian. Austral Ecol. 38, 543–547. doi: 10.1111/j.1442-9993.2012.02452.x
Bowler, D. E., and Benton, T. G. (2005). Causes and consequences of animal dispersal strategies: relating individual behavior to spatial dynamics. Biol. Rev. 80, 205–225. doi: 10.1017/S1464793104006645
Brodin, T., Lind, M. I., Wiberg, M. K., and Johansson, F. (2013). Personality trait differences between mainland and island populations in the common frog (Rana temporaria). Behav. Ecol. Sociobiol. 67, 135–143. doi: 10.1007/s00265-012-1433-1
Broquet, T., Angelone, S., Jaquiery, J., Joly, P., Léna, J.P., Lengagne, T., et al. (2010). Genetic bottlenecks driven by population disconnection. Conserv. Biol. 24, 1596–1605. doi: 10.1111/j.1523-1739.2010.01556.x
Brown, J. H., and Kodric-Brown, A. (1977). Turnover rates in insular biogeography: effect of immigration on extinction. Ecology 58, 445–449. doi: 10.2307/1935620
Browne, C. L., and Paszkowski, C. A. (2014). The influence of habitat composition, season and gender on habitat selection by western toads (Anaxyrus boreas). Herp. Conserv. Biol. 9, 417–427. Available online at: https://www.researchgate.net/profile/Constance_Browne/.
Brunelli, E., Perrota, I., Bonacci, A., and Tripepi, S. (2007). Differential expression of aquaporin 3 in Triturus italicus from larval to adult epidermal conversion. Eur. J. Histochem. 51, 25–32. Available online at: https://ejh.it.
Buxton, V. L., and Sperry, J. H. (2017). Reproductive decisions in anurans: a review of how predation and competition affects the deposition of eggs and tadpoles. Bioscience 67, 26–38. doi: 10.1093/biosci/biw149
Careau, V. (2012). Performance, personality, and energetics: correlation, causation, and mechanism. Physiol. Biochem. Zool. 85, 543–571. doi: 10.1086/666970
Careau, V., Thomas, D., Humphries, M. M., and Réale, D. (2008). Energy metabolism and animal personality. Oikos 117, 641–653. doi: 10.1111/j.0030-1299.2008.16513.x
Cayuela, H., Boualit, R., Arsovski, D., Bonnaire, E., Pichenot, J., Bellec, A., et al. (2016). Does habitat unpredictility promote the evolution of a colonizer syndrome in amphibian metapopulations? Ecology 97, 2658–2670. doi: 10.1002/ecy.1489
Cayuela, H., Grolet, O., and Joly, P. (2019). Context-dependent dispersal, public information and heterospecific attraction in newts. Oecologia 188, 1069–1080. doi: 10.1007/s00442-018-4267-3
Cayuela, H., Joly, P., Schmidt, B.R., Pichenot, J., Bonnaire, E., Priol, P., et al. (2017). Life history tactics shape amphibians' demographic responses to the North Atlantic Oscillation. Global Change Biol. 23, 4620–4638. doi: 10.1111/gcb.13672
Cayuela, H., Rougemont, Q., Prunier, J. G., Moore, J. S., Clobert, J., Besnard, A., et al. (2018a). Demographic and genetic approaches to study dispersal in wild animal populations: a methodological review. Mol. Ecol. 27, 3976–4010. doi: 10.1111/mec.14848
Cayuela, H., Schmidt, B. R., Weinbach, A., Besnard, A., and Joly, P. (2018b). Multiple density-dependent processes shape the dynamics of a spatially structured amphibian population. J. Anim. Ecol. 88, 164–177. doi: 10.1111/1365-2656.12906
Cayuela, H., Valenzuela-Sanchez, A., Teulier, L., Martínez-Solano, I., Léna, J. P., Merilä, J., et al. (2018). Determinants and consequences of dispersal in vertebrates with complex life cycles: a review of pond-breeding amphibians. Peer J. 6:e27394v1. doi: 10.7287/peerj.preprints.27394v1
Chapple, D. G., Simmonds, S. M., and Wong, B. B. M. (2012). Can behavioral and personality traits influence the success of unintentional species introductions? TREE 27, 57–64. doi: 10.1016/j.tree.2011.09.010
Cheptou, P. O., Hargreaves, A. L., Bonte, D., and Jacquemyn, H. (2017). Adaptation to fragmentation: evolutionary dynamics driven by human influences. Philos. Trans. R. Soc. B 372:20160037. doi: 10.1098/rstb.2016.0037
Clauzel, C., Bannwarth, C., and Foltête, J. C. (2015). Integrating regional-scale connectivity in habitat restoration: An application for amphibian conservation in eastern France. J. Nat. Conserv. 23, 98–107. doi: 10.1016/j.jnc.2014.07.001
Clobert, J., Baguette, M., Benton, T. G., and Bullock, J. M. (2012). Dispersal Ecology and Evolution. Oxford: Oxford University Press, 462p.
Clobert, J., Le Galliard, J. F., Cote, J., Meylan, S., and Massot, M. (2009). Informed dispersal, heterogeneity in animal dispersal syndromes and the dynamics of spatially structured populations. Ecol. Lett. 12, 197–209. doi: 10.1111/j.1461-0248.2008.01267.x
Comte, L., and Olden, J. D. (2018). Evidence for dispersal syndromes in freshwater fishes. Proc. R. Soc. B 285:20172214. doi: 10.1098/rspb.2017.2214
Connette, G. M., and Semlitsch, R. D. (2013). Context-dependent movement behavior of woodland salamanders (Plethodon) in two habitat types. Zoology 116, 325–330. doi: 10.1016/j.zool.2013.08.004
Consentino, B. J., and Droney, D. C. (2016). Movement behaviour of woodland salamanders is repeatable and varies with forest age in a fragmented landscape. Anim. Behav. 121, 137–146. doi: 10.1016/j.anbehav.2016.08.013
Consentino, B. J., Schooley, R. L., and Phillips, C. A. (2011). Connectivity of agroecosystems: dispersal costs can vary among crops. Landsc. Ecol. 26, 371–379. doi: 10.1007/s10980-010-9563-1
Constible, J. M., Gregory, P. T., and Larsen, K. V. (2010). The pitfalls of extrapolation in conservation: movements and habitat use of a threatened toad are different in the boreal forest. Anim. Conserv. 13, 43–52. doi: 10.1111/j.1469-1795.2009.00291.x
Cornelius, C., Awade, M., Candia-Gallardo, C., Sieving, K. E., and Metzger, J. P. (2017). Habitat fragmentation drives inter-population variation in dispersal behavior in a neotropical rainforest bird. Perspect. Ecol. Conserv. 15, 3–9. doi: 10.1016/j.pecon.2017.02.002
Cosgrove, A. J., McWhorter, T. J., and Maron, M. (2018). Consequences of impediments of animal movements at different scales: a conceptual framework and review. Divers. Distrib. 24, 448–459. doi: 10.1111/ddi.12699
Coster, S. S., Powell, J. S. V., and Babbit, K. J. (2014). Characterizing the width of amphibian movements during postbreeding migration. Conserv. Biol. 28, 756–762. doi: 10.1111/cobi.12214
Cote, J., Bestion, E., Jacob, S., Travis, J., Legrand, D., and Baguette, M. (2017). Evolution of dispersal strategies and dispersal syndromes in fragmented landscapes. Ecography 40, 56–73. doi: 10.1111/ecog.02538
Cote, J., and Clobert, J. (2007). Social information and emigration: lessons from immigrants. Ecol. Lett. 10:411–417. doi: 10.1111/j.1461-0248.2007.01032.x
Cote, J., Clobert, J., and Brodin, T. (2010a). Personality-dependent dispersal: characterization, ontogeny and consequences for spatially structured populations. Philos. Trans. R. Soc. B 365, 4065–4076. doi: 10.1098/rstb.2010.0176
Cote, J., Clobert, J., Brodin, T., Fogarty, S., and Sih, A. (2010b). Personality-dependent dispersal: characterization, ontogeny and consequences for spatially structured populations. Philos. Trans. R. Soc. B 365, 4065–4076.
Coulon, A., Aben, J., Palmer, C. F. S., Stevens, V. M., Callens, T., Strube, D., et al. (2015). A stochastic movement simulator improves estimates of landscape connectivity. Ecology 96, 2203–2213. doi: 10.1890/14-1690.1
Crawford, J. A., Peterman, W. E., Kuhns, A. R., and Eggert, L. S. (2016). Altered functional connectivity and genetic diversity of a threatened salamander in an agroecosystem. Landsc. Ecol. 31, 2231–2244. doi: 10.1007/s10980-016-0394-6
Cushman, S. A. (2006). Effects of habitat loss and fragmentation on amphibians: a review and prospectus. Biol. Conserv. 128, 231–240. doi: 10.1016/j.biocon.2005.09.031
Dall, S. R. X., Houston, A. I., and McNamara, J. M. (2004). The behavioural ecology of personality: consistent individual differences from an adaptive perspective. Ecol. Lett. 7, 734–739. doi: 10.1111/j.1461-0248.2004.00618.x
Daversa, D. R., Muths, E., and Bosch, J. (2012). Terrestrial movement patterns of the common toad (Bufo bufo) in Central Spain reveal habitat of conservation importance. J. Herp. 46, 658–664. doi: 10.1670/11-012
Decout, S., Manel, S., Miaud, C., and Luque, S. (2012) Integrative approach for landscape-based graph connectivity analysis: a case study with the common frog (Rana temporaria) in human-dominated landscapes. Landsc. Ecol. 27, 267–279. doi: 10.1007/s10980-011-9694-z
Delgado, M. M., Ratikainen, I. I., and Kokko, H. (2011). Inertia: the discrepancy between individual and common good in dispersal and prospecting behaviour. Biol. Rev. 86, 717–732. doi: 10.1111/j.1469-185X.2010.00167.x
Denoël, M., Dalleur, S., Langrand, E., Besnard, A., and Cayuela, H. (2018). Dispersal and alternative breeding site fidelity strategies in an amphibian. Ecography 41, 1543–1555. doi: 10.1111/ecog.03296
Diego-Rasilla, F. J., and Luengo, R. M. (2004). Heterospecific call recognition and phonotaxis in the orientation behavior of the marbled newt, Triturus marmoratus. Behav. Ecol. Sociobiol. 55, 556–560. doi: 10.1007/s00265-003-0740-y
Diego-Rasilla, F. J., Luengo, R. M., and Phillips, J. B. (2008). Use of a magnetic compass for nocturnal homing orientation in the palmate newt, Lissotriton helveticus. Ethology 114, 808–815. doi: 10.1111/j.1439-0310.2008.01532.x
Dingemanse, N. J., and Wolf, M. (2010). Recent models for adaptive personality differences: a review. Phil. Trans. R. Soc. B 365, 3947–3958. doi: 10.1098/rstb.2010.0221
Dochtermann, N. A., Schwab, T., and Sih, A. (2014). The contribution to additive genetic variation to personality variation: heritability of personality. Proc. R. Soc. B 282:20142201. doi: 10.1098/rspb.2014.2201
Doligez, B., Danchin, E., and Clobert, J. (2002). Public information and breeding habitat selection in a wild bird population. Science 297, 1168–1170. doi: 10.1126/science.1072838
Drent, P. J., van Oers, K., and van Nordwick, A. J. (2003). Realized heritability of personalities in the great tit (Parus major). Proc. R. Soc. Lond. B 270, 45–51. doi: 10.1098/rspb.2002.2168
Fagan, W. F., Lewis, M. A., Auger-Méthé, M., Avgar, T., Benhamou, S., Breed, G., et al. (2013). Spatial memory and animal movement. Ecol. Lett. 16, 1316–1329. doi: 10.1111/ele.12165
Fahrig, L., and Merriam, G. (1985). Habitat patch connectivity and population survival. Ecology 66, 1762–1768. doi: 10.2307/2937372
Fahrig, L., Pedlar, J. H., Pope, S. E., Taylor, P. D., and Wegner, J. F. (1995). Effect of road traffic on amphibian density. Biol. Conserv. 73, 177–182. doi: 10.1016/0006-3207(94)00102-V
Fogarty, S., Cote, J., and Sih, A. (2011). Social personality polymorphism and the spread of invasive species: a model. Amer. Nat. 177, 273–287. doi: 10.1086/658174
Foltête, J. C., Clauzel, C., Vuidel, G., and Tournant, P. (2012). Integrating graph-based connectivity metrics into species distribution models. Landsc. Ecol. 27, 557–569. doi: 10.1007/s10980-012-9709-4
Foltête, J. C., Girardet, X., and Clauzel, C. (2014). A methodological framework for the use of landscape graphs in land-use planning. Landsc. Urban Plan. 124, 140–150. doi: 10.1016/j.landurbplan.2013.12.012
Fowler, M. S. (2009). Density dependent dispersal decisions and the Allee effect. Oikos 118, 604–614. doi: 10.1111/j.1600-0706.2008.17321.x
Fraenkel, G. S., and Gunn, D. L. (1961). The Orientation of Animals. New York, NY: Dover Publ., 324.
Fraser, K. C., Davies, K. T. A., Davy, C. M., Ford, A. T., Tyler Flockhart, D. T., and Martins, E. G. (2018). Tracking the conservation promise of movement ecology. Front. Ecol. Evol. 6:150. doi: 10.3389/fevo.2018.00150
Frei, M., Csencsics, D., Brodbeck, S., Schweizer, E., Bühler, C., Gugerli, F., et al. (2016). Combining landscape genetics, radio-tracking and long-term monitoring to derive management implications for Natterjack toads (Epidalea calamita) in agricultural landscapes. J. Nature Conserv. 32, 22–34. doi: 10.1016/j.jnc.2016.04.002
Funk, W. C., Greene, A. E., Corn, P. S., and Allendorf, F. W. (2005). High dispersal in a frog species suggests that it is vulnerable to habitat fragmentation. Biol. Lett. 1, 13–16. doi: 10.1098/rsbl.2004.0270
Gamble, L. R., McGarigal, K., and Compton, B. W. (2007). Fidelity and dispersal in the pond-breeding amphibian, Ambystoma opacum: implications for spatio-temporal population dynamics and conservation. Biol. Conserv. 139, 247–257. doi: 10.1016/j.biocon.2007.07.001
Garwood, J. M. (2009). Spatial Ecology of the Cascades Frog: Identifying Dispersal, Migration, and Resource Uses at Multiple Spatial Scales. PhD Thesis, Humboldt State University, California.
Gerhardt, H. C., and Klump, G. M. (1988). Phonotactic responses and selectivity of barking treefrogs (Hyla gratiosa) to chorus sounds. J. Comp. Physiol. A 163, 795–802. doi: 10.1007/BF00604056
Gilbert-Norton, L., Wilson, R., Stevens, J. R., and Beards, K. H. (2010). A meta-analytic review of corridor effectiveness. Conserv. Biol. 24, 660–668. doi: 10.1111/j.1523-1739.2010.01450.x
Gomez-Laplaza, L. M., and Gerlai, R. (2010). Latent learning in zebrafish (Danio rerio). Behav. Brain Res. 208, 509–515. doi: 10.1016/j.bbr.2009.12.031
Gonzalez-Bernal, E., Brown, G. P., and Shine, R. (2014). Invasive cane toads: social facilitation depends upon an individual's personality. PLos ONE 9:e102880. doi: 10.1371/journal.pone.0102880
Graeter, G. J., Rothermel, B. B., and Gibbons, J. W. (2008). Habitat selection and movement of pond-breeding amphibians in experimentally fragmented pine forests. J. Wildl. Manag. 72, 473–482. doi: 10.2193/2006-330
Groff, L. A., Calhoun, A. J. K., and Loftin, C. S. (2017). Amphibian terrestrial habitat selection and movement patterns vary with annual life history period. Can. J. Zool. 95, 433–442. doi: 10.1139/cjz-2016-0148
Gruber, J., Whiting, M. J., Brown, G., and Shine, R. (2017). The loneliness of the long-distance toad: invasion history and social attraction in cane toads (Rhinella marina). Biol. Lett. 13:20170445. doi: 10.1098/rsbl.2017.0445
Hamer, A. J., Lane, S. L., and Mahony, M. J. (2008). Movement patterns of adult green and golden bell frogs Litoria aurea and the implications for conservation management. J. Herpetol. 42, 397–407. doi: 10.1670/07-0862.1
Hamer, A. J., and McDonnell, M. J. (2008). Amphibian ecology and conservation in the urbanising world: a review. Biol. Conserv.141, 2432–2449. doi: 10.1016/j.biocon.2008.07.020
Hanski, I., Erälahti, C., Kankare, M., Ovaskainen, O., and Siren, H. (2004). Variation in migration propensity among individuals maintained by landscape structure. Ecol. Lett. 10, 958–966. doi: 10.1111/j.1461-0248.2004.00654.x
Harrison, S. (1991). Local extinction in a metapopulation context: an empirical evaluation. Biol. J. Linn. Soc. 42, 73–88. doi: 10.1111/j.1095-8312.1991.tb00552.x
Harts, A. M. F., Jaatinen, K., and Kokko, H. (2015). Evolution of natal and breeding dispersal: when is a territory an asset worth protecting? Behav. Ecol. 27, 287–294. doi: 10.1093/beheco/arv148
Heard, G. W., Scroggie, M. P., and Malone, B. S. (2012). Classical metapopulation theory as a useful paradigm for the conservation of an endangered amphibian. Biol. Conserv.148, 156–166. doi: 10.1016/j.biocon.2012.01.018
Heemeyer, J. L., and Lannoo, M. J. (2012). Breeding migrations in crayfish frogs (Lithobates areolatus): long-distance movements, burrow philopatry, and mortality in a near-threatened species. Copeia 2012, 440–450. doi: 10.1643/CE-11-107
Hendrix, R., Schmidt, B. R., Schaub, M., Krause, E. T., and Steinfartz, S. (2017). Differentiation of movement behaviour in an adaptively diverging salamander population. Molec. Ecol. 26, 6400–6413. doi: 10.1111/mec.14345
Heusser, H. (1968). Die Lebensweise der Erdkröte Bufo bufo (L.); Wanderungen und Sommerquartiere. Rev. Suisse Zool. 75, 927–982.
Hirsch, P. E., Thorlacius, M., Brodin, T., and Burkhardt-Holm, P. (2017). An approach to incorporate individual personality in modeling fish dispersal across in-stream barriers. Ecol. Evol. 7, 720–732. doi: 10.1002/ece3.2629
Holt, R. D., and Fryxell, J. M. (2011). “Theoretical reflections on the evolution of migration,” in Animal Migration: A Synthesis, eds E. J. Milner-Gulland, J. M. Fryxell, and A. R. E. Sinclair (Oxford: Oxford University Press), 17–31.
Houlahan, J. E., Findlay, C. S., Schmidt, B. R., Meyer, A. H., and Kuzmin, S. L. (2000). Quantitative evidence for global amphibian declines. Nature 404, 752–755. doi: 10.1038/35008052
Hudson, C. M., McCurry, M. R., Lundgren, P., McHenry, C. R., and Shine, R. (2016). Constructing an invasion machine: the rapid evolution of a dispersal-enhancing phenotype during the cane toad invasion of Australia. PLoS ONE 11:e0156950. doi: 10.1371/journal.pone.0156950
Humphries, W. J., and Sisson, M. A. (2012). Long-distance migrations, landuse, and vulnerability of prescribed fire of the gopher frog (Lithobates capito). J. Herpetol. 46, 665–670. doi: 10.1670/11-124
Ims, R. A., and Yoccoz, N. G. (1997). “Studying transfer processes in metapopulations: emigration, migration, and colonization,” in Metapopulation Biology, eds I. Hanski, and M. E. Gilpin (Elsevier: Academic Press), 247–265.
Indermaur, L., Gehring, M., Wehrle, W., Tockner, K., and Naef-Daenzer, B. (2009). Behavior-based scale definitions for determining space use: requirements of two amphibians. Amer. Nat. 173, 60–71. doi: 10.1086/593355
Janin, A. (2010). Assessing Connectivity in Fragmented Landscapes: From Behavioral Ecology to Conservation. PhD Thesis, Université Lyon1, OCLC 869270730.
Janin, A., Léna, J. P., Deblois, S., and Joly, P. (2012a). Use of stress-hormone levels and habitat selection to assess functional connectivity of a landscape for an amphibian. Conserv. Biol. 26, 923–931. doi: 10.1111/j.1523-1739.2012.01910.x
Janin, A., Léna, J. P., and Joly, P. (2011). Beyond occurrence: body condition and stress hormone as integrative indicators of habitat availability and fragmentation in the common toad. Biol. Conserv. 144, 1008–1016. doi: 10.1016/j.biocon.2010.12.009
Janin, A., Léna, J. P., and Joly, P. (2012b). Habitat fragmentation affects movement behavior of migrating juvenile common toads. Behav. Ecol. Sociobiol. 66, 1351–1356. doi: 10.1007/s00265-012-1390-8
Janin, A., Léna, J. P., Ray, N., Delacourt, C., Allemand, P., and Joly, P. (2009). Assessing landscape connectivity with calibrated cost-distance modelling: predicting common toad distribution in a context of spreading agriculture. J. Appl. Ecol. 46, 833–841. doi: 10.1111/j.1365-2664.2009.01665.x
Jarvis, L. E., Hartup, M., and Petrovan, S. O. (2019). Road mitigation using tunnels and fences promotes site connectivity and population expansion for a protected amphibian. Eur. J. Wildl. Res. 65:27. doi: 10.1007/s10344-019-1263-9
Joly, P., and Grolet, O. (1996). Colonization dynamics of new ponds, and the age structure of colonizing Alpine newts, Triturus alpestris. Acta Oecol. 17, 599–608.
Joly, P., and Miaud, C. (1989). Fidelity to the breeding site in the Alpine newt (Triturus alpestris). Behav. Process. 19, 47–56. doi: 10.1016/0376-6357(89)90030-2
Joly, P., and Miaud, C. (1993). How does a newt find its pond: the role of chemical cues in migrating alpine newt. Ethol. Ecol. Evol. 5, 447–455.
Joly, P., Miaud, C., Lehmann, A., and Grolet, O. (2001). Habitat matrix effects on pond occupancy in newts. Conserv. Biol. 15, 239–248. doi: 10.1046/j.1523-1739.2001.99200.x
Joly, P., and Morand, A. (1996). “Amphibian diversity and land-water ecotones,” in Biodiversity in Land-Inland Water Ecotones, eds J. B. Lachavanne, and R. Juge (Nashville, TN: Unesco and Parthenon Publ. Group), 161–182.
Joly, P., Morand, C., and Cohas, A. (2003). Habitat fragmentation and amphibian conservation: building a tool for assessing landscape matrix connectivity. Comptes Rendus Biol. 326, S132–S139. doi: 10.1016/S1631-0691(03)00050-7
Kelleher, S. R., Silla, A. J., and Byrne, P. G. (2018). Animal personality and behavioral syndromes in amphibians: a review of the evidence, experimental approaches, and implications for conservation. Behav. Ecol. Sociobiol. 72:79. doi: 10.1007/s00265-018-2493-7
Kelleher, S. R., Silla, A. J., Dingemanse, N. J., and Byrne, P. G. (2017). Body size predicts between-individual differences in exploration behaviour in the southern corroboree frog. Anim. Behav. 129, 161–170. doi: 10.1016/j.anbehav.2017.05.013
Knowlton, J. L., and Graham, C. H. (2010). Using behavioural landscape to predict species' responses to land use and climate change. Biol. Conserv. 143, 1342–1354. doi: 10.1016/j.biocon.2010.03.011
Kobayashi, S., Abe, S., Tomita, M., and Matsuki, R. (2018). Fine-scale genetic structure and estimation of gene flow of the Japanese brown frog Rana japonica in a Satoyama landscape on the western side of Inba Lake, Eastern Japan. Curr. Herp. 37, 11–22. doi: 10.5358/hsj.37.11
Koprivnikar, J., Gibson, C. H., and Redfern, J. C. (2012). Infectious personalities: behavioural syndromes and disease risks in larval amphibians. Proc. R. Soc. Lond. B 279, 1544–1550. doi: 10.1098/rspb.2011.2156
Koumaris, A., and Fahrig, L. (2016). Different anuran species show different relationships to agricultural intensity. Wetlands 36, 731–744. doi: 10.1007/s13157-016-0781-4
Landler, L., and Gollmann, G. (2011). Magnetic orientation of the common toad: establishing an arena approach for adult anurans. Front. Zool. 8:6. doi: 10.1186/1742-9994-8-6
Le Galliard, J. F., Ferrière, R., and Clobert, J. (2005). Effect of patch occupancy on immigration in the common lizard. J. Anim. Ecol. 74, 241–249. doi: 10.1111/j.1365-2656.2005.00912.x
Lehtinen, R. M., and Galatowitsch, S. M. (1999). Consequences of habitat loss and fragmentation for wetland amphibian assemblages. Wetlands 9, 1–12. doi: 10.1007/BF03161728
Leskovar, C., and Sinsch, U. (2005). Harmonic direction finding: a novel tool to monitor the dispersal of small sized anurans. Herp. J. 15, 173−180. Available online at: http://www.researchgate.net/publication/233599101.
Liang, C. T. (2013). Movements and habitat use of Yosemite toads (Anaxyrus (formerly Bufo) canorus) in the Sierre National Forest, California. J. Herpetol. 47, 555–564. doi: 10.1670/12-054
Lindström, T., Brown, G. P., Sisson, S. A., Phillips, B. L., and Shine, R. (2013). Rapid shifts in dispersal behavior on an expanding range edge. Proc. Natl. Acad. Sci. U.S.A. 110, 13452–13456. doi: 10.1073/pnas.1303157110
Lowe, W. H. (2009). What drives long-distance dispersal? A test of theoretical predictions. Ecology 90, 1456–1462. doi: 10.1890/08-1903.1
Lowe, W. H. (2010). Explaining long-distance dispersal: effects of dispersal distance on survival and growth in a stream salamander. Ecology 91, 3008–3015. doi: 10.1890/09-1458.1
Lowe, W. H., and Allendorf, F. W. (2010). What can genetics tell us about population connectivity? Mol. Ecol. 19, 3038–3051. doi: 10.1111/j.1365-294X.2010.04688.x
Lowe, W. H., and McPeek, M. A. (2012). Can natural selection maintain long-distance dispersal? Insight from a stream salamander system. Ecol. Evol. 26, 11–24. doi: 10.1007/s10682-011-9500-z
Luqman, H., Muller, R., Vaupel, A., Brodbeck, S., Bollinger, J., and Gugerli, F. (2018). No distinct barrier effects of highways and a wide river of the genetic structure of the Alpine newt (Ichtyosaura alpestris) in a densely settled landscape. Conserv. Genet. 19, 673–685. doi: 10.1007/s10592-018-1046-y
Madden, N., and Jehle, R. (2017). Acoustic orientation in the great crested newt (Triturus cristatus). Amphib. Rept. 38, 57–65. doi: 10.1163/15685381-00003083
Maes, J., van Damme, R., and Matthysen, E. (2012). Individual and among-population variation in dispersal-related traits in Natterjack toads. Behav. Ecol. 24, 521–531. doi: 10.1093/beheco/ars193
Marsh, D. M., Kanishka, A. T., Kimberley, C. B., and Clarke, L. B. (2004). Dispersal and colonization through open fields by a woodland terrestrial salamander. Ecology 85, 3396–3405. doi: 10.1890/03-0713
Marsh, D. M., and Trenham, P. C. (2008). Metapopulation dynamics and amphibian conservation. Conserv. Biol. 15, 40–49. doi: 10.1111/j.1523-1739.2001.00129.x
Martin, E., and Caillère, L. (1982). Local enhancement in the Alpine newt Triturus alpestris (Amphibia Urodela) in aquatic phase. CR Acad. Sci. Paris Life Sci. 294, 1105–1108.
Massol, F., Duputie, A., David, P., and Jarne, P. (2011). Asymmetric patch size distribution leads to disruptive selection on dispersal. Evolution 65, 490–500. doi: 10.1111/j.1558-5646.2010.01143.x
Massot, M., and Clobert, J. (2000). Processes at the origin of similarities in dispersal behaviour among siblings. J. Evol. Biol. 13, 707–719. doi: 10.1046/j.1420-9101.2000.00202.x
Masters, B. S., and Forester, D. C. (1995). Kin recognition in a brooding salamander. Proc. R. Soc. Lond. B 261, 43–48. doi: 10.1098/rspb.1995.0115
Mathias, A., Kisdi, E., and Olivieri, I. (2001). Divergent evolution of dispersal in a heterogeneous landscape. Evolution 55, 246–259. doi: 10.1111/j.0014-3820.2001.tb01290.x
Matthysen, E. (2012). “Multicausality of dispersal: a review,” in Dispersal Ecology and Evolution, eds J. Clobert, M. Baguette, T. G. Benton, and J. M. Bullock (Oxford: Oxford UP), 3–18.
Mazzerole, M. J., and Desrochers, A. (2005). Landscape resistance to frog movements. Can. J. Zool. 83, 455–464. doi: 10.1139/z05-032
Mazzerole, M. J., and Villard, M. A. (1999). Patch characteristics and landscape context as predictors of species presence and abundance: a review. Ecoscience 6, 117–124. doi: 10.1080/11956860.1999.11952204
McDonough, C., and Patton, P. W. C. (2007). Salamander dispersal across a forested landscape fragmented by a golf course. J. Wildl. Manag. 71, 1163–1169. doi: 10.2193/2006-380
McDougall, P. T., Réale, D., Sol, D., and Reader, S. M. (2006). Wildlife conservation and animal temperament: causes and consequences of evolutionary change for captive, reintroduced, and wild populations. Anim. Conserv. 9, 39–48. doi: 10.1111/j.1469-1795.2005.00004.x
McRae, B. H., and Beier, P. (2007). Circuit theory predicts gene flow in plants and animals. Proc. Nat. Acad. Sci. U.S.A. 104, 19885–19890. doi: 10.1073/pnas.0706568104
Miaud, C., Sanuy, D., and Avrillier, J. N. (2000). Terrestrial movements of the natterjack toad Bufo calamita (Amphibia, Anura) in a semi-arid, agricultural landscape. Amphib. Rept. 21, 357–369. doi: 10.1163/156853800507426
Miaud, C., Sérandour, J., Martin, R., and Pidancier, N. (2005). “Preliminary results on the genetic control of dispersal in common frog Rana temporaria froglets,” in Herpetologica Petropolitana, eds N. Ananjeva and O. Tsinenko (Saint-Petersburg: Proc. 12th Congress of SHE), 193–197.
Moilanen, A., and Hanski, I. (1998). Metapopulation dynamics: effect of habitat quality and landscape structure. Ecology 79, 2503–2515. doi: 10.1890/0012-9658(1998)079[2503:MDEOHQ]2.0.CO;2
Moore, H. J. (1954). Some observations on the migration of the toad (Bufo bufo). Br. J. Herpetol. 1, 194–224.
Morales, J. M., Moorcroft, P. R., Matthiopoulos, J., Ftair, J. L., Kie, J. G., Powell, R. A., et al. (2010). Building the bridge between animal movement and population dynamics. Philos. Trans. R. Soc B 365, 2289–2301. doi: 10.1098/rstb.2010.0082
Morris, D. W. (1982). Age-specific dispersal strategies in iteroparous species: who leaves when? Evol. Theory 6, 53–65.
Müller, T., and Juskauskas, A. (2018). Inbreeding affects personality and fitness of a leaf beetle. Anim. Behav. 138, 29–37. doi: 10.1016/j.anbehav.2018.02.002
Muths, E. (2003). Home range and movements of boreal toads in undisturbed habitat. Copeia 2003, 161–165. doi: 10.1643/0045-8511(2003)003[0160:HRAMOB]2.0.CO;2
Muths, E., Bailey, L. L., Lambert, B. A., and Schneider, S. C. (2018). Estimating the probability of movement and partitioning seasonal survival in an amphibian metapopulation. Ecosphere 9:e02480. doi: 10.1002/ecs2.2480
Muths, E., Chambert, T., Schmidt, B., Miller, D., Hossack, B., Joly, P., et al. (2017). Heterogeneous responses of amphibian populations to climate change complicates conservation planning. Sci. Rep. 7:17102. doi: 10.1038/s41598-017-17105-7
Nathan, R., Getz, W.M., Revilla, E., Holyoak, M., Kadmon, R., Salz, D., et al. (2008). A movement ecology paradigm for unifying organismal movement research. Proc. Natl. Acad. Sci. U.S.A. 105, 19052–19059. doi: 10.1073/pnas.0800375105
Nathan, R., and Muller-Landau, H. C. (2000). Spatial patterns of seed dispersal, their determinants and consequences for recruitment. Trends Ecol. Evol. 15, 278–285. doi: 10.1016/S0169-5347(00)01874-7
Neal, K. M., Johnson, B. B., and Shaffer, H. B. (2018). Genetic structure and environmental niche modeling confirm two evolutionary and conservation units within the western spadefoot (Spea hammondii). Conserv. Genet. 19, 937–946. doi: 10.1007/s10592-018-1066-7
Nowakowski, A. J., Veiman-Echeverria, M., Kurz, D. J., and Donnelly, M. A. (2015). Evaluating connectivity for tropical amphibians using empirically derived resistance surfaces. Ecol. Appl. 25, 928–942. doi: 10.1890/14-0833.1
Ogurtsov, S. V., and Bastakov, V. A. (2001). “Imprinting on native pond odour in the pool frog Rana lessonae Cam,” in Chemical Signals in Vertebrates 9, eds A. Marchlewska-Koj, J. J. Lepri, and D. Muller-Schwarze (New York, NY: Kluwer Academic/Plenum Publishers), 433–438.
Oromi, N., Richter-Boix, A., Sanuy, D., and Fila, J. (2012). Genetic variability in geographic populations of the natterjack toad (Bufo calamita). Ecol. Evol. 2, 2018–2026. doi: 10.1002/ece3.323
Ousterhout, B. H., and Semlitsch, R. D. (2014). Measuring terrestrial movement behavior using passive integrated transponder (PIT) tags: effects of tag size on detection, movement, survival, and growth. Behav. Ecol. Sociobiol. 68, 343–350. doi: 10.1007/s00265-013-1656-9
Palmer, C. F., Coulon, A., and Travis, J. M. J. (2011). Introducing a ‘stochastic movement simulator' for estimating habitat connectivity. Methods Ecol. Evol. 2, 258–268. doi: 10.1111/j.2041-210X.2010.00073.x
Parvinen, K. (2007). Evolutionary suicide in a discrete-time metapopulation model. Evol. Ecol. Res. 9, 619–633.
Pasukonis, A., Trenkwalder, K., Ringler, M., Ringler, E., Mangione, R., Steininger, J., et al. (2016). The significance of spatial memory for water finding in a tadpole-transporting frog. Anim. Behav. 116, 89–98. doi: 10.1016/j.anbehav.2016.02.023
Pereira, H. M., Leadley, P. W., Proença, V., Alkemade, R., Scharlemann, J. P. W., Fernandez-Manjarrés, J. F., et al. (2010). Scenarios for global biodiversity in the 21st century. Science 330, 1496–1501. doi: 10.1126/science.1196624
Perret, N., Pradel, R., Miaud, C., Grolet, O., and Joly, P. (2003). Transience, dispersal and survival rates in newt patchy populations. J. Anim. Ecol.72, 567–575. doi: 10.1046/j.1365-2656.2003.00726.x
Peterman, W. E., Anderson, T. L., Ousterhout, B. H., Drake, D. L., Burkhart, J. J., Rowland, F., et al. (2018). Using spatial demographic network models to optimize habitat management decisions. J. Wildl. Manage. 82, 649–659. doi: 10.1002/jwmg.21393
Petranka, J. W., and Holbrook, P. T. (2006). Wetland restoration for amphibians: Should local sites be designed to support metapopulations or patchy populations? Restor. Ecol. 14, 404–411. doi: 10.1111/j.1526-100X.2006.00148.x
Phillips, B. L., Brown, G. P., and Shine, R. (2010). Evolutionarily accelerated invasions: the rate of dispersal evolves upwards during the range advance of cane toads. J. Evol. Biol. 23, 2595–2601. doi: 10.1111/j.1420-9101.2010.02118.x
Phillips, B. L., Brown, G. P., Webb, J. K., and Shine, R. (2006). Invasion and the evolution of speed in toads. Nature 439:803. doi: 10.1038/439803a
Phillips, J. B. (1996). Magnetic navigation. J. Theor. Biol. 180, 309–319. doi: 10.1006/jtbi.1996.0105
Pilliod, D. S., Peterson, C. R., and Ritson, P. I. (2002). Seasonal migration of Columbia spotted frog (Rana luteiventris) among complementary resources in a high mountain basin. Can. J. Zool. 80, 1849–1862. doi: 10.1139/z02-175
Pitt, A. L., Tavano, J. J., Baldwin, R. F., and Stegenda, B. S. (2017). Movement ecology and habitat use of three sympatric anuran species. Herp. Conserv. Biol. 12, 212–224.
Pittman, S. E., Osbourn, M. S., and Semlitsch, R. D. (2014). Movement ecology of amphibians: a missing component for understanding population declines. Biol. Conserv. 169, 44–53. doi: 10.1016/j.biocon.2013.10.020
Pittman, S. E., and Semlitsch, R. D. (2013). Habitat type and distance to edge affect movement behavior of juvenile pond-breeding salamanders. J. Zool. 291, 154–162. doi: 10.1111/jzo.12055
Pope, S. E., Fahrig, L., and Merriam, H. G. (2000). Landscape complementation and metapopulation effects on leopard frog populations. Ecology 81, 2498–2508. doi: 10.1890/0012-9658(2000)081[2498:LCAMEO]2.0.CO;2
Prunier, J., Kaufmann, B., Léna, J. P., Fenet, S., Pompanon, F., and Joly, P. (2014). A forty-year-old divided highway does not prevent gene flow in the alpine newt Ichthyosaura alpestris. Conserv. Genet. 15, 453–468. doi: 10.1007/s10592-013-0553-0
Pupin, F., Sacchi, R., Gentilli, A., Galeotti, P., and Fasola, M. (2007). Discrimination of toad calls by smooth newts: support for the heterospecific attraction hypothesis. Anim. Behav. 74, 1683–1690. doi: 10.1016/j.anbehav.2007.03.020
Purrenhage, J. L., Niewiarowski, P. H., and Moore, F. B. G. (2009). Population structure of spotted salamanders (Ambystoma maculatum) in a fragmented landscape. Mol. Ecol. 18, 235–247. doi: 10.1111/j.1365-294X.2008.04024.x
Rannap, R., Lohmus, A., and Briggs, L. (2009). Restoring ponds for amphibians: a success story. Hydrobiologia 634, 87–95. doi: 10.1007/s10750-009-9884-8
Ray, N., Lehmann, A., and Joly, P. (2002). Modeling spatial distribution of amphibian populations: a GIS approach based on habitat matrix permeability. Biodiv. Conserv. 11, 2143–2165. doi: 10.1023/A:1021390527698
Reading, C. J., Loman, J., and Madsen, T. (1991). Breeding pond fidelity in the common toad (Bufo bufo). J. Zool. Lond. 225, 201–211. doi: 10.1111/j.1469-7998.1991.tb03811.x
Réale, D., Dingemanse, N. J., Kazem, A. J. M., and Wright, J. (2010). Evolutionary and ecological approaches to the study of personality. Philos. Trans. R. Soc. B 365, 3937–3946. doi: 10.1098/rstb.2010.0222
Rhoads, E. A., Williams, P. K., and Crane, C. M. (2017). High inbreeding and low connectivity among Ambystoma texanum populations in fragmented Ohio forests. Ecol. Evol. 7, 11135–11147. doi: 10.1002/ece3.3637
Richardson, J. L. (2012). Divergent landscape effects on population connectivity in two co-occurring amphibian species. Molec. Ecol. 21:4537. doi: 10.1111/j.1365-294X.2012.05708.x
Rittenhouse, T. A. G., and Semlitsch, R. D. (2006). Grasslands as movement barriers for a forest-associated salamander: migration behavior of adult and juvenile salamanders at a distinct habitat edge. Biol. Conserv. 131, 14–22. doi: 10.1016/j.biocon.2006.01.024
Rodda, G. H., and Phillips, J. B. (1992). Navigational systems develop along similar lines in amphibians, reptiles, and birds. Ethol. Ecol. Evol. 4, 43–51. doi: 10.1080/08927014.1992.9525349
Rodriguez, F., Duran, E., Vargas, J. P., Torres, B., and Salas, C. (1994). Performance of goldfish trained in allocentric and egocentric maze procedures suggests the presence of a cognitive mapping system in fishes. Anim. Learn. Behav. 22, 409–420. doi: 10.3758/BF03209160
Ronce, O. (2007). How does it feel to be like a rolling stone? Ten questions about dispersal evolution. Ann. Rev. Ecol. Evol. Syst. 38: 231–253. doi: 10.1146/annurev.ecolsys.38.091206.095611
Ronce, O., and Clobert, J. (2012). “Dispersal syndromes,” in Dispersal Ecology and Evolution, eds J. Clobert, M. Baguette, T. G. Benton, and J. M. Bullock (Oxford: Oxford University Press), 119–138.
Rowe, G., Beebee, T. J. C., and Burke, T. (2000). A microsatellite analysis of natterjack toad, Bufo calamita, metapopulations. Oikos 88, 641–651. doi: 10.1034/j.1600-0706.2000.880321.x
Saastamoinen, M., Bocedi, G., Cote, J., Legrand, D., Guillaume, F., Wheat, C.W., et al. (2018). Genetics of dispersal. Biol. Rev. 93, 574–599. doi: 10.1111/brv.12356
Sanuy, D., and Joly, P. (2009). Olfactory cues and breeding habitat selection in the natterjack toad, Bufo calamita. Amphib. Rept. 30, 555–565. doi: 10.1163/156853809789647158
Sawatzky, M. E., Martin, A. E., and Fahrig, L. (2019). Landscape context is more important than wetland buffers for farmland amphibians. Agric. Ecosyst. Environ. 269, 97–106. doi: 10.1016/j.agee.2018.09.021
Schulte, U., Küsters, D., and Steinfartz, S. (2007). A PIT tag based analysis of annual movement patterns of adult fire salamanders (Salamandra salamandra) in a Middle European habitat. Amphib. Rept. 28, 531–536. doi: 10.1163/156853807782152543
Selonen, V., and Hanski, I. (2006). Habitat exploration and use in dispersing juvenile flying squirrels. J. Anim. Ecol. 75, 1440–1449. doi: 10.1111/j.1365-2656.2006.01168.x
Semlitsch, R. D. (1997). Biological delineation of terrestrial buffer zones for pond-breeding salamanders. Conserv. Biol. 12, 1113–1119. doi: 10.1046/j.1523-1739.1998.97274.x
Shoemaker, V. H., Hillman, S. S., Hillyard, S. D., Jackson, D. C., McClanahan, L. L., Withers, P. C., et al. (1992). “Exchange of water, ions, and respiratory gases in terrestrial amphibians,” in Environmental Physiology of the Amphibians, eds E. Feder, and W. W. Burggren (Chicago, IL: University of Chicago Press), 125–150.
Sih, A., and Bell, A. M. (2008). Insights for behavioral ecology from behavioral syndromes. Adv. Study Behav. 38, 227–281. doi: 10.1016/S0065-3454(08)00005-3
Sih, A., Bell, A., and Johnson, J. C. (2004). Behavioral syndromes: an ecological and evolutionary overview. Trends Ecol. Evol. 19, 372–378. doi: 10.1016/j.tree.2004.04.009
Sih, A., Cote, J., Evans, M., Fogarty, S., and Pruitt, J. (2012). Ecological implications of behavioral syndromes. Ecol. Lett. 15, 278–289. doi: 10.1111/j.1461-0248.2011.01731.x
Sinsch, U. (2006). Orientation and navigation in amphibia. Marine Freshwat. Behav. Physiol. 39, 65–71. doi: 10.1080/10236240600562794
Sinsch, U. (2014). Movement ecology of amphibians: from individual migratory behaviour to spatially structured populations in heterogenous landscapes. Can. J. Zool. 92, 491–502. doi: 10.1139/cjz-2013-0028
Sinsch, U., and Kirst, J. M. (2016). Homeward orientation of displaced newts (Triturus cristatus, Lissotriton vulgaris) is restricted to the range of routine movements. Ethol. Ecol. Evol. 28, 312–328. doi: 10.1080/03949370.2015.1059893
Sinsch, U., Oromi, N., Miaud, C., Denton, J., and Sanuy, D. (2012) Connectivity of local amphibian populations: modelling the migratory capacity of radio-tracked natterjack toads. Anim. Conserv. 15, 388–396. doi: 10.1111/j.1469-1795.2012.00527.x
Smith, B. R., and Blumstein, D. T. (2008). Fitness consequences of personality: a meta-analysis. Behav. Ecol. 19, 448–455. doi: 10.1093/beheco/arm144
Smith, M. A., and Green, D. M. (2005). Dispersal and the metapopulation paradigm in amphibian ecology and conservation: are all amphibian populations metapopulations? Ecography 28, 110–128. doi: 10.1111/j.0906-7590.2005.04042.x
Stamps, J. A. (1998). Conspecific attraction and aggregation in territorial species. Am. Nat. 131, 329–347. doi: 10.1086/284793
Stamps, J. A. (2004). Growth-mortality tradeoffs and ‘personality traits’ in animals. Ecol. Lett. 10, 355–363. doi: 10.1111/j.1461-0248.2007.01034.x
Stamps, J. A. (2016). Individual differences in behavioural personalities. Biol. Rev. 91: 534–567. doi: 10.1111/brv.12186
Stamps, J. A., and Groothuis, T. G. G. (2010). Developmental perspectives on personality: implications for ecological and evolutionary studies of individual differences. Philos. Trans. R. Soc. B 365, 4029–4041. doi: 10.1098/rstb.2010.0218
Stamps, J. A., Krishnan, V. V., and Reid, M. L. (2005). Search costs and habitat selection by dispersers. Ecology 86, 510–518. doi: 10.1890/04-0516
Stearns, S. C., and Koella, J. C. (1986). The evolution of phenotypic plasticity in life-history traits: prediction of reaction norms for age and size at maturity. Evolution 40, 893–913. doi: 10.1111/j.1558-5646.1986.tb00560.x
Stevens, V. M., Verkenne, C., Vandewoestijne, S., Wesselingh, R. A., and Baguette, M. (2006). Gene flow and functional connectivity in the natterjack toad. Mol. Ecol. 15, 2333–2344. doi: 10.1111/j.1365-294X.2006.02936.x
Stevens, V. M., Whitmee, S., Le Galliard, J. F., Clobert, J., Böhning-Gaese, K., Bonte, D., et al. (2014). A comparative analysis of dispersal syndromes in terrestrial and semi-terrestrial animals. Ecol. Lett. 17, 1039–1052. doi: 10.1111/ele.12303
Swanson, E. M., Tekmen, S. M., and Bee, M. A. (2007). Do female frogs exploit inadvertent social information to locate breeding aggregations? Can. J. Zool. 85, 921–932. doi: 10.1139/Z07-074
Swanson, J. E., Muths, E., Pierce, C. L., Dinsmore, S. J., Vandever, M. W., Hladik, M. L., et al. (2018). Exploring the amphibian exposome in an agricultural landscape using telemetry and passive sampling. Sci. Rep. 8:10045.doi: 10.1038/s41598-018-28132-3
Thomas, C. D. (2000). Dispersal and extinction in fragmented landscapes. Proc. R. Soc. B 267, 139–145. doi: 10.1098/rspb.2000.0978
Timm, B. C., McGarigal, K., and Cokk, R. P. (2014). Upland movement patterns and habitat selection of adult Eastern spadefoots (Scaphiopus holbrookii) at Cape Cod national seashore. J. Herpetol. 48, 84–97. doi: 10.1670/12-201
Tolman, E. C. (1948). Cognitive maps in rats and men. Psychol. Rev. 55, 189–208. doi: 10.1037/h0061626
Tornabene, B. J., Blaustein, A. R., Briggs, C. J., Calhoun, D. M., Johnson, P. T. J., McDevitt-Galles, T., et al. (2018). The influence of landscape and environmental factors on ranavirus epidemiology in a California amphibian assemblage. Freshw. Biol. 63, 639–651. doi: 10.1111/fwb.13100
Travis, J. M. J., Mustin, K., Barton, K. A., Benton, T. G., Clobert, J., Delgado, M. M., et al. (2012). Modelling dispersal: an ecoevolutionary framework incorporating emigration, movement, settlement behaviour and the multiple costs involved. Methods Ecol. Evol. 3, 628–641. doi: 10.1111/j.2041-210X.2012.00193.x
Trenham, P. C., Koenig, W. D., and Shaffer, H. B. (2001). Spatially autocorrelated demography and interpond dispersal in the salamander Ambystoma californiense. Ecology 82, 3519–3530. doi: 10.2307/2680169
Trochet, A., Le Chevalier, H., Calvez, O., Riberon, A., Bertrand, R., and Blanchet, S. (2019). Influence of substrate types and morphological traits on movement behavior in a toad and newt species. PeerJ 6:e6053. doi: 10.7717/peerj.6053
Tufto, J. (2000). The evolution of plasticity and nonplastic spatial and temporal adaptations in the presence of imperfect environmental cues. Am. Nat. 156, 121–130. doi: 10.1086/303381
Twitty, V. C. (1959). Migration and speciation in newts. Science 130:3391. doi: 10.1126/science.130.3391.1735
Urszan, T. J., Török, J., Hettvey, A., Garamszegi, L. Z., and Herczeg, G. (2015). Behavioural consistency and life history of Rana dalmatina tadpoles. Oecologia 178, 129–140. doi: 10.1007/s00442-014-3207-0
Van Buskirk, J. (2012). Permeability of the landscape matrix between amphibian breeding sites. Ecol. Evol. 2, 3160–3167. doi: 10.1002/ece3.424
Via, S. (1993). Adaptive phenotypic plasticity: target or by-product of selection in a variable environment? Am. Nat. 142, 352–365. doi: 10.1086/285542
Videlier, M., Bonneaud, C., Cornette, R., and Herrel, A. (2015). Exploration syndromes in the frog Xenopus (Silurana) tropicalis: correlations with morphology and performances. J. Zool. 294, 206–213. doi: 10.1111/jzo.12170
Vos, C. C., Goedhart, P. W., Lammertsma, D. R., and Spitzen-Van der Sluijs, A. M. (2007). Matrix permeability of agricultural landscapes: an analysis of movements of the common frog (Rana temporaria). Herp. J. 17, 169–182.
Wallraff, H. G. (1983). Relevance of atmospheric odours and geomagnetic field to pigeon navigation: what is the “map” basis? Comp. Biol. Physiol. 76A, 643–663. doi: 10.1016/0300-9629(83)90126-3
Weinbach, A., Cayuela, H., Grolet, O., Besnard, A., and Joly, P. (2018). Resilience to climate variation in a spatially structured amphibian population. Sci. Rep. 8:14807. doi: 10.1038/s41598-018-33111-9
Wilson, A. D. M., and Krause, J. (2012). Personality and metamorphosis: is behavioral variation consistent across ontogenetic niche shifts? Behav. Ecol. 23, 1316–1323. doi: 10.1093/beheco/ars123
Wiltschko, R., Nohr, D., and Wiltschko, W. (1981). Pigeons with a deficient sun compass use magnetic compass. Science 214, 343–345. doi: 10.1126/science.7280697
Winandy, L., Legrand, P., and Denoël, M. (2017). Habitat selection and reproduction of newts in networks of fish and fishless aquatic patches. Anim. Behav. 123, 107–115. doi: 10.1016/j.anbehav.2016.10.027
Youngquist, M. B., and Boone, M. D. (2014). Movement of amphibians through agricultural landscapes: the role of habitat on edge permeability. Biol. Conserv. 175, 148–155. doi: 10.1016/j.biocon.2014.04.028
Youngquist, M. B., Inoue, K., Berk, D. J., and Boone, M. D. (2017). Effects of land use on population presence and genetic structure of an amphibian in an agricultural landscape. Landsc. Ecol. 32, 147–162. doi: 10.1007/s10980-016-0438-y
Zamudio, K. R., and Wieczorek, A. M. (2007). Fine-scale spatial genetic structure and dispersal among spotted salamander (Ambystoma maculatum) breeding populations. Mol. Ecol. 16, 257–274. doi: 10.1111/j.1365-294X.2006.03139.x
Keywords: movement ecology, landscape, habitat fragmentation, spatially structured populations, migration, dispersal
Citation: Joly P (2019) Behavior in a Changing Landscape: Using Movement Ecology to Inform the Conservation of Pond-Breeding Amphibians. Front. Ecol. Evol. 7:155. doi: 10.3389/fevo.2019.00155
Received: 19 December 2018; Accepted: 23 April 2019;
Published: 17 May 2019.
Edited by:
Caitlin R. Gabor, Texas State University System, United StatesReviewed by:
David Charles Droney, Hobart and William Smith Colleges, United StatesMonique De Jager, Netherlands Institute of Ecology (NIOO-KNAW), Netherlands
Copyright © 2019 Joly. This is an open-access article distributed under the terms of the Creative Commons Attribution License (CC BY). The use, distribution or reproduction in other forums is permitted, provided the original author(s) and the copyright owner(s) are credited and that the original publication in this journal is cited, in accordance with accepted academic practice. No use, distribution or reproduction is permitted which does not comply with these terms.
*Correspondence: Pierre Joly, cGllcnJlLmpvbHlAdW5pdi1seW9uMS5mcg==