- Division of Marine Science and Conservation, Duke University, Beaufort, NC, United States
Coastal wetlands are among the most productive ecosystems in the world. They generate critical services for humans including shoreline protection, carbon storage, pollution mitigation, and fisheries production. Restoration of coastal wetlands has historically been viewed as a secondary conservation strategy, but recently—given the continued loss of wetlands worldwide—many non-governmental and governmental organizations have elevated habitat restoration to be a primary method for wetland conservation. The long-held paradigm in coastal wetland restoration has been to restore target habitats by reducing physical stressors and avoiding competition among outplants, such as mangrove saplings or Spartina plugs. Recent ecological research, however, reveals that positive species interactions, such as facilitation, are critical to wetland recovery after disturbance. Here, we review the scientific evidence for the importance of positive species interactions in the recovery of salt-marsh and mangrove ecosystems and assess the extent to which they have been integrated into restoration studies. We found that only a small proportion of studies of marsh and mangrove restoration examined the effects of positive species interactions, despite the important role they play in the regrowth of coastal wetlands. We outline how positive species interactions can be systematically incorporated into future restoration work and discuss how this incorporation can help the reestablishment of coastal wetland biota through: (1) trophic facilitation, (2) stress reduction, and (3) associational defenses. The absence of positive interactions in restoration designs may partially explain the significant disparities between the functioning of natural and restored coastal plant ecosystems.
The Importance of Coastal Wetlands and the Threats They Face
Coastal wetlands are among the most productive ecosystems on Earth and generate vital services that benefit human societies around the world. Sediment-stabilization by wetlands such as salt marshes and mangroves serves to protect coastal communities from storm-waves, flooding, and land erosion (Gedan et al., 2011). Coastal wetlands also reduce pollution from human waste (Kadlec and Wallace, 2008; Yang et al., 2008), remove excess nutrients from the water column (Ouyang and Guo, 2016), trap pollutants (Gambrell, 1994), and sequester carbon (Mcleod et al., 2011). Further, near-shore wetlands act as both essential nursery habitats and feeding grounds for game fish, supporting a diverse group of economically important species (Lipcius et al., 2005; Mumby, 2006; Aburto-Oropeza et al., 2008; Nagelkerken et al., 2008).
Despite their immense ecological and economic importance, coastal wetland ecosystems are in decline. In this review, we focus on salt marshes and mangroves, which, like many other coastal wetlands, are facing serious threats from coastal development, a rapidly changing climate, pollution, invasive species, and overfishing. North American salt marshes, for instance, have decreased by 30–90% (Gedan and Silliman, 2009a). Similarly, in 2001 scientists estimated that over 35% of mangroves had been lost worldwide, which surpasses habitat losses in other ecosystems such as rainforests (Valiela et al., 2001). Furthermore, mangrove deforestation is not halting; the Food and Agriculture Organization of the United Nations estimates that 1–3% of the remaining area of mangroves is lost each year (FAO, 2007).
Increased physical stress resulting from coastal development, climate change, and pollution is one of the key mechanisms underlying coastal wetland degradation and loss. Dredging, for example, can alter marsh hydrologic regimes that are vital for determining marsh function (Crain et al., 2009) and economic development can lead to the degradation of coastal ecosystems (He et al., 2014). Climate change and pollution also cause wetland loss by increasing drought, toxicity, and nutrient stress (Bertness et al., 2002; Gedan et al., 2009). Human-induced sea level rise poses a drowning threat to coastal wetlands (Ellison and Stoddart, 1991; Nicholls et al., 1999; Craft et al., 2009), particularly where shoreline development impedes plant migration to higher ground (Schuerch et al., 2018). Similarly, subsidence as a result of sediment starvation is a serious threat to salt marshes around the world (Gedan et al., 2009). On a regional and local level, oil spills frequently harm marsh-associated animals (Zengel et al., 2016); can kill salt-marsh plants, which causes erosion and further ecosystem loss (Silliman et al., 2016); and are responsible for the loss of ~120,000 ha of mangrove forests (Duke, 2016).
In most coastal wetlands, these physical forces do not act alone; biotic forces also contribute to coastal wetland loss and degradation. An increasingly recognized biotic mechanism of coastal wetland degradation is trophic downgrading (i.e., loss of high trophic-level consumers) as well as its associated interactions with climate stress. Overfishing of consumers, for example, can damage coastal wetlands by releasing grazers from top-down control. Particularly in salt marshes, overfishing of predators has been shown to drive coastal wetland loss by increasing grazing on salt-marsh plants which leads to the development of expansive salt and mudflats (Silliman and Bertness, 2002; Altieri et al., 2012; Silliman et al., 2013; He et al., 2017). In China, Argentina, Canada, and the southern U.S., drought stress has been shown to interact with top-down effects, which can lead to wetland loss (Silliman et al., 2005; Costa et al., 2009; Henry and Jefferies, 2009; He et al., 2017). During droughts, salts build up in the soils and make plants more susceptible to grazing. Once plants die-off and mud flats are formed, elevated salt concentrations in soils and intensified grazing pressure can prevent marsh recovery, resulting in the emergence of a stable alternate mud flat state (Henry and Jefferies, 2009; He et al., 2017). Similarly, reduced habitat complexity, which can be a feature of restored marshes (Lawrence et al., 2018), can affect biological interactions that hasten marsh decline. Finke and Denno (2006) found that marsh habitat complexity increases the beneficial impacts of predatory arthropods that control Spartina consumers, while in simplified habitats the beneficial effects of these predators are weakened. In these two cases, both biotic (i.e., grazing, predation) and abiotic (i.e., drought, complexity) factors interact to drive ecosystem change.
Invasive species have also altered the structure and functioning of coastal wetlands. A meta-analysis of aquatic biotic invasions revealed that invasive species decrease native species abundances as well as alter nutrient cycling across diverse ecosystems, including coastal wetlands (Gallardo et al., 2016). In eastern U.S. salt marshes, for instance, invasive species such as Phragmites australis can alter trophic structure (Gratton and Denno, 2005, 2006) and outcompete native species, reducing ecosystem diversity (Silliman and Bertness, 2004; Minchinton et al., 2006). In Chinese, western U.S., Australian, and Mediterranean salt marshes, Spartina species and their hybrids are outcompeting native species and taking over mudflats (Nieva et al., 2005; An et al., 2007; Strong and Ayres, 2009). Although less studied, mangroves are also affected by species invasions (Biswas et al., 2007), which likely influences community functioning. In many cases, these invasions can be exacerbated by human-induced changes, such as eutrophication, which have the potential to facilitate the spread of non-native species (e.g., Tyler et al., 2007). Additionally, younger ecosystems may be more susceptible to species invasions (e.g., native invasion in Veeneklaas et al., 2013) and estuarian wetlands are particularly susceptible to invasions (Byers, 2009), making a thorough understanding of species interactions particularly relevant to coastal wetland restoration projects.
The Role of Restoration in the Future of Coastal Wetlands
Given the current state of coastal wetlands, immediate action is needed to slow the loss of these systems and bolster the services they provide. Although preservation is typically seen as the best way to conserve ecosystems, in some cases restoration may be a better or key supplemental option (Possingham et al., 2015) and vital to the future of natural habitats (Dobson et al., 1997). Particularly if sea level rises faster than shoreline wetlands can migrate, if migration is impeded by coastal development, or if environmental disturbances increase, restoration that facilitates wetland migration and/or re-colonization after die off may be essential to ensure wetland functional longevity. Indeed, major non-governmental and governmental organizations are beginning to discuss whether restoration at large scales (10–1,000 s km2) can be an effective way to not only halt decline, but actually increase total wetland coverage, and the United Nations declared the upcoming 10 years the decade of restoration.
Many restored ecosystems, however, fail to completely achieve the structure and functioning of natural communities. Recovering wetland diversity through restoration can take decades (Borja et al., 2010) and many projects never achieve the desired state, which can limit the ecosystem services they provide. A global meta-analysis across a variety of ecosystems revealed that restored sites consistently have lower biodiversity than natural sites (Rey Benayas et al., 2009). A study in western India found that restored mangroves contributed only 22% of what natural forests contribute to associated fisheries (Das, 2017) and a study in southeast England found that even after 100 years most restored salt marshes failed to replicate natural species richness (Garbutt and Wolters, 2008). In addition to having lower supporting and regulating services (Meli et al., 2014), restored ecosystems may have altered levels of biogeochemical function. Even decades after restoration is initiated, important nutrients like carbon and nitrogen may be dramatically lower. A 2012 meta-analysis of coastal and inland wetlands by Moreno-Mateos et al. (2012) found that restored sites had 26% lower functioning in terms of structural services and 23% lower functioning in terms of biogeochemical services.
In addition to ecological challenges, restoration can be costly and impractical at large scales, particularly if restored areas are slow to become self-sustaining. In 2010 the median reported restoration cost was $8,961 for one hectare of mangrove and $67,128 for one hectare of salt marsh (Bayraktarov et al., 2016). If restoration is going to be a primary mechanism for wetland recovery, as conservation organizations have proposed, we need to improve restoration efficiency (e.g., the cost to amount of restored habitat ratio) and implement restoration designs that support the rapid creation of natural, high-functioning wetlands. Although restoration ecology often draws from ecological theory and understanding (Palmer et al., 1997; Young et al., 2005), marine managers often do not integrate important ecological concepts (e.g., positive species interactions) into restoration projects, which may mean coastal wetland restoration is underperforming (e.g., Shaver and Silliman, 2017; Zhang et al., 2018).
Importance of Positive Interactions in Coastal Wetlands
Positive interactions are interactions between organisms where at least one partner benefits and no partners are adversely impacted (e.g., mutualism, commensalism, facilitation). Over the past few decades, ecological experiments have shown that positive interactions are critical to the success and recovery of ecosystems (Stachowicz, 2001; Bruno et al., 2003; Hay et al., 2004). For example, oysters recruit to other oyster shells, which offer predator protection (O'Beirn et al., 2000); seagrass can reduce desiccation stress for other seagrasses (Tsai et al., 2010); and adult sea urchins protect urchin larvae (Quinn et al., 1993).
Recolonization and succession processes, which are integral to restoration, are influenced by positive interactions. In the case of seagrass, facultative rhyizophytic algae can help plants overcome nutrient limitations, which facilitates the recolonization of nutrient-limited seagrasses (Williams, 1990). In the rocky intertidal zone, coralline algae and understory species can facilitate kelp recruitment and canopy cover (Barner et al., 2016). Similarly, in mangroves and salt marshes, neighboring plants can reduce both soil salt and low oxygen stress, while aboveground plant structure can create habitat that facilitates biodiversity by lowering predation and insolation stress for associated species (Figures 1, 2). These interactions often create positive feedback loops, where the establishment of new species is aided by the addition of another species—a process that can and should be harnessed in restoration and recolonization. When these interactions are missing, communities may struggle to recover and the development of biodiversity and ecosystem functioning may be hindered.
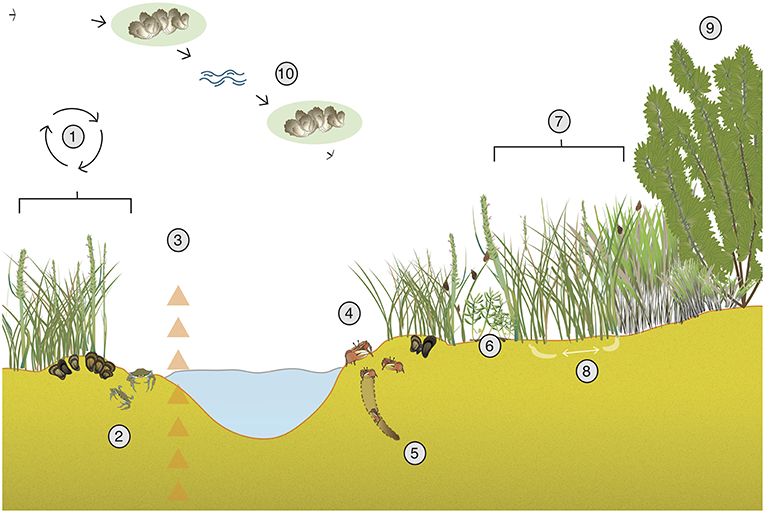
Figure 1. Examples of positive interactions in salt-marsh ecosystems. (1) Mussels provide nutrients to marsh grasses, reduce sulfide stress (Derksen-Hooijberg et al., 2018), enhance biodiversity (Angelini et al., 2015), and increase marsh resistance to drought (Angelini et al., 2016). Cordgrass facilitates mussel establishment (Bertness and Grosholz, 1985) and reduces heat stress (Angelini et al., 2015). (2) Predators such as blue and green crabs act as important top-down controls on herbivores and help prevent run-away grazing on marsh grasses (Silliman and Bertness, 2002; Bertness and Coverdale, 2013). (3) High densities of salt-marsh plants increase wave attenuation, promote sedimentation, and thus increase sediment accumulation (Borsje et al., 2011). (4) Fiddler crabs can mitigate stress caused by snail grazing (Gittman and Keller, 2013) and the facultative effect of mussels and fiddler crabs can be increased when present together (Hughes et al., 2014). (5) Fiddler crab burrowing can increase oxygen in marsh sediments (Bertness, 1985). Further, crab burrowing can faciliate mycorrhizal fungi, which can account for ~35% of plant growth (Daleo et al., 2007). (6) Biodiverse assemblages increase biomass and nitrogen accumulation (Callaway et al., 2003). (7) Marsh grasses mixed with other plants can provide a refuge for snails from predators and indirectly protect grasses from grazing (Hughes, 2012). (8) Close by marsh grasses can help reduce communal stress as they provide shade (Whitcraft and Levin, 2007) and oxygen to sediments (Howes et al., 1986). (9) Spartina alterniflora can act as a nurse plant for other species (Egerova et al., 2003). (10) Salt marshes provide nutrients to neighboring ecosystems (van de Koppel et al., 2015) and long-distance facilitation from nearby systems such as oyster reefs can reduce wave stress (Meyer et al., 1997). See acknowledgments for vector credits.
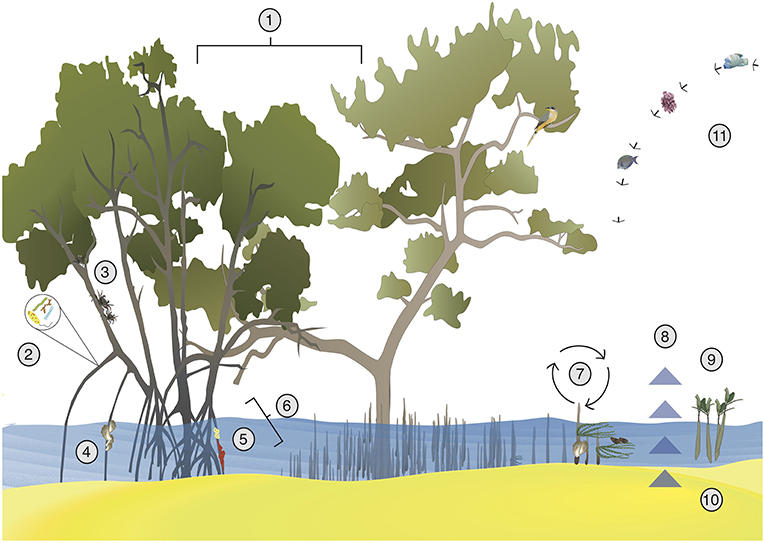
Figure 2. Examples of positive interactions in mangrove ecosystems. (1) Multi-species plantations can sequester more carbon in sediments (Chen et al., 2012) and can increase root yields (Lang'at et al., 2013). (2) Microbial communities receive food from mangrove root exudates and can help recycle nutrients (Bashan and Holguin, 2002). (3) Mixed stands of mangroves can provide association defense against herbivory (Erickson et al., 2012). (4) Mangrove roots allow for oyster recruitment and reduce sedimentation stress, and the presence of oysters in turn supports other species (Aquino-Thomas and Proffitt, 2014). (5) Mangroves provide carbon to sponges and sponges provide nitrogen to mangroves (Ellison et al., 1996). (6) Mangrove roots provide habitat for sponges and tunicates, which protect mangrove roots from harmful isopods (Ellison and Farnsworth, 1990). (7) In a facilitation cascade, mangrove pneumatophores trap algae and oysters, which act as secondary foundation species and support diverse mollusk communities (Bishop et al., 2012). (8) Mangrove plantations sequester carbon in sediments (Mcleod et al., 2011), which is affected by planting density and may be facilitated by top predators (Atwood et al., 2015). (9) Other plant species can increase recruitment of mangroves by trapping seeds and ameliorating abiotic stress (McKee et al., 2007). (10) Higher densities of mangroves can increase seedling success and sediment accumulation, which may allow for more resilience to sea level rise (Huxham et al., 2010). (11) Nearby coral reefs protect mangroves from wave action, while mangroves reduce sedimentation stress and increase reef fish biomass on reefs (van de Koppel et al., 2015). See acknowledgments for vector credits.
A recent multi-ecosystem meta-analysis (He et al., 2013) revealed that positive interactions are particularly important in stressful environments (i.e., the Stress Gradient Hypothesis) such as tidal flats, which experience extreme salinity and tidal fluctuations. These results also revealed that positive interactions are critical for plant community diversity and function during ecosystem recovery from disturbance (e.g., large scale plant mortality due to drought). To elucidate the potential importance of positive species interactions to coastal wetland restoration efforts, we first examine how positive interactions are crucial to salt marsh and mangrove recovery from disturbance via (1) stress amelioration, (2) associational defenses, and (3) trophic facilitation (Figures 1, 2) and then outline how these positive interactions can be systematically incorporated into coastal wetland restoration plans.
Stress Amelioration
Natural clumping of foundation species such as oysters, mussels, or mangroves is common in stressful environments where survivorship of individuals is higher in groups than when found alone. Clumped organisms can reduce both physical (e.g., low oxygen) and biological (e.g., predation) stress, which facilitates conspecifics as well as other associated species, allowing for community development. For instance, oxygen stress in mudflats is often alleviated through positive interactions among clumped marsh plants and mangrove saplings. Salt-marsh grasses and mangroves planted closely together benefit from oxygen leaking out of the roots of nearby plants (Howes et al., 1986; Gedan and Silliman, 2009b) and plants in clumps can grow 2–3x faster (Silliman et al., 2015). Similarly, mangroves can lessen sedimentation and salinity stress for other mangroves or other foundation species such as oysters (Aquino-Thomas and Proffitt, 2014). Species that benefit from the establishment of these foundation species can generate further positive feedbacks through reciprocal facilitation. For instance, fiddler crabs benefit from the conditions provided by foundation species and in turn increase oxygen availability for marsh plants through burrowing (Bertness, 1985); detritivores recycle nutrients in mangrove ecosystems (Nagelkerken et al., 2008); and mangrove crabs reduce anoxic conditions in sediments (Stieglitz et al., 2000), improving conditions for associated species.
Positive interactions can also ameliorate nutrient stress in coastal wetlands. For example, sponges on mangrove roots provide nitrogen to and receive carbon from mangroves, which promotes the growth of both species (Ellison et al., 1996). Similarly, mutualistic mussels can augment Spartina success and resilience after disturbance by reducing sulfide and nutrient stress (Derksen-Hooijberg et al., 2018) and marsh crabs can slow soil nitrogen depletion, promoting the growth of marsh plants (Zhang et al., 2013). Although less visually apparent, microorganisms living symbiotically with marsh plants or mangroves in natural communities can also promote plant growth and aid in restoration yields (Bashan and Holguin, 2002; Daleo et al., 2007; Bledsoe and Boopathy, 2016). In these cases, proximity to other macro- or micro- organisms helps ameliorate the stress of living in a highly dynamic environment. Given that we are discovering an increasing number of positive interactions among organisms that may be pivotal to long-term ecosystem functioning, these examples of stress-reducing, positive species interactions are likely only the beginning of our understanding of facilitation in coastal wetlands.
Associational Defenses
Wetland species can benefit from the associational defenses of co-occurring organisms. For example, sponges and ascidians on mangrove roots protect mangroves from isopods that impede root growth (Ellison and Farnsworth, 1990). Between plants, mixed stands of mangroves defend trees from crab herbivory (Erickson et al., 2012) and co-occurring marsh plants can reduce snail herbivory on Spartina (Hughes, 2012). Although largely unexplored, there may also be facilitation among different genotypes of coastal wetland plant species. For example, different Spartina genotypes have different morphologies [e.g., heights and stem lengths: (Proffitt et al., 2003)] as well as different interactions with herbivores (Zerebecki et al., 2017). In other plants, genotype has been shown to affect plant interactions with associated species (e.g., ants and aphids on milkweed: Mooney and Agrawal, 2008), which—if present in wetlands—may provide associational defenses to clumps of foundation species. Associated species can also facilitate non-foundation species, leading to greater ecosystem diversity. For instance, mangrove sponges can encourage growth of other sponge species in return for protection from predators (Engel and Pawlik, 2005).
Trophic Facilitation
Historically, coastal wetland research focused on how “bottom-up” factors, such as nutrient and hydrologic regimes, controlled communities. However, research over the past 15 years has shown that consumer or “top-down” controls on plants are also important (He and Silliman, 2016). Indeed, grazer control of salt-marsh and mangrove plants has been found on every continent where coastal wetlands occur (He and Silliman, 2016). Top-down control in food webs can lead to trophic cascades, which indirectly affect plant success. In these cascades predators control grazers, which facilitates plant growth and has positive impacts on coastal wetland structure and function. The exact species operating in these trophic cascades vary geographically, but generally consist of a top predator that controls an intermediate species, which in turn influences a foundation species (Figure 3). Because foundation species are critical to ecosystem functioning, decreases in top predators—which lead to increases in intermediate species and decreases in foundation species—can have ricocheting effects on the entire ecosystem (Figure 3).
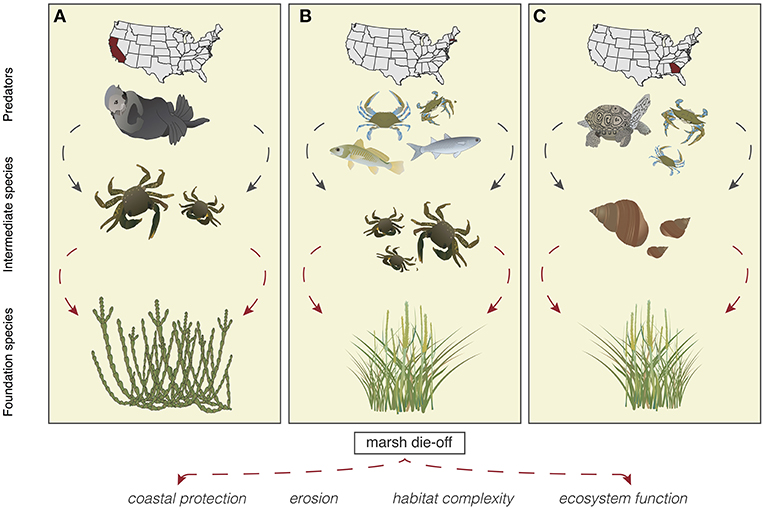
Figure 3. Demonstrated trophic cascades in United States salt marshes. (A) In the western U.S. sea otters control herbivorous crabs, which can cause erosion and loss of salt marshes (Hughes et al., in personal communication). (B) In New England Blue crabs control herbivorous crabs, which can cause runaway grazing on cordgrasses (Altieri et al., 2012). (C) In the Southeast U.S. blue crabs and terrapins control periwinkle snails, which graze on marsh grasses (Silliman and Bertness, 2002). See acknowledgments for vector credits.
In U.S. salt marshes, consumers such as predatory crabs and fish regulate grazers that can decimate cordgrass populations. Overfishing of blue crabs and fish has been linked to marsh vegetation loss through reduced consumer pressure on grazing snails and crabs (Silliman and Bertness, 2002). Trophic cascades are less well-documented in mangrove systems, but are likely important (He and Silliman, 2016). Traditional competition alone does not explain large scale patterns of phytophagous insects and the incorporation of facilitation may improve our understanding of the insects that exert top-down control on plant communities (Kaplan and Denno, 2007), such as those in mangroves.
Ants have been shown to indirectly facilitate mangroves by removing insect herbivores, which in turn makes tree leaves less susceptible to crab herbivory (Offenberg et al., 2006) and predator presence may facilitate carbon sequestration (Atwood et al., 2015). Beyond trophic facilitation via direct consumption, predators can facilitate communities through fear, which can alter herbivore behavior and help maintain important foundation species (Kimbro, 2012).
In addition to protecting coastal wetlands from overgrazing, recent research has shown predators may also facilitate wetland recovery. In New England, for instance, invasive green crabs were found to compensate for native predator declines and facilitate marsh vegetation recovery on Cape Cod by reducing herbivory (Bertness and Coverdale, 2013). Similarly, in seagrass ecosystems Hughes and co-authors found that sea otters could help seagrass resilience by promoting epigrazers (Hughes et al., 2016) and sea otter presence could reduce the impacts of eutrophication on seagrass populations (Hughes et al., 2013). Species that consume fruits may also play an under-appreciated role in ecosystem functions such as seed dispersal. Mangrove-associated birds are important seed dispersers and may facilitate invertebrate dispersal through wading (Buelow and Sheaves, 2015). Similarly, other grazing vertebrates can facilitate marsh seed dispersal by moving uneaten seeds (Shanholtzer, 2012).
The importance of predators in facilitating plants is likely to become more extreme as abiotic stressors increase in coastal wetlands worldwide. For instance in a Chinese salt marsh, He et al. (2017) showed that drought conditions led to complete ecosystem loss in the presence of herbivorous crabs, whereas marsh plants survived drought in areas protected from crabs. Similarly Silliman et al. (2013) found that grazers had a disproportionately large negative impact on marsh cover in declining marshes because grazers flocked to remaining marsh grasses, increasing the relative top-down pressure on surviving grasses. Related research found that wolf spiders can be effective in controlling important Spartina consumers (Prokelisia dolus and Prokelisia marginata), but that the strength of this top-down control was mediated by nutrients and vegetation structure (Denno et al., 2002). Restoration groups that do not address the top-down pressures, as well as bottom-up pressures, on re-vegetated marshes can suffer significant losses and be forced to continually replant (e.g., He et al., 2017). Robust intact predator assemblages are vital to coastal wetlands as they mediate the effect of primary consumers on foundation species. Through the introduction of predator species, trophic cascades can likely be leveraged to promote restoration.
Positive Interactions are Underrepresented in Current Coastal Wetland Restoration
The coastal wetland restoration paradigm is in part borrowed from plantation methodology and forest restoration, which center on the idea that plant populations are limited by physical stress and negative species interactions, such as competition. Accordingly, managers generally disperse outplants and focus on ameliorating abiotic stresses, such as unnatural hydrologic regimes (Silliman et al., 2015). To examine whether the scientific research in coastal wetland restoration reflects these paradigms, we conducted a literature review of relevant articles since 1980. We searched Web of Science for English articles using the search terms: “TS = (restoration) AND TS = (salt marsh OR mangrove)” from 1980 to 2017, which yielded 1,415 results. We reviewed abstracts and only included studies about coastal salt marshes and mangroves that were about restoration or conducted in a restored area, which reduced the number of studies to 605. Of those, only 22 examined positive interactions. The vast majority of studies did not examine biotic interactions, instead focusing on other topics (e.g., physical factors such as hydrologic regimes, policy/management structures) (Figure 4), which may help explain the lag in incorporating facilitation into restoration projects.
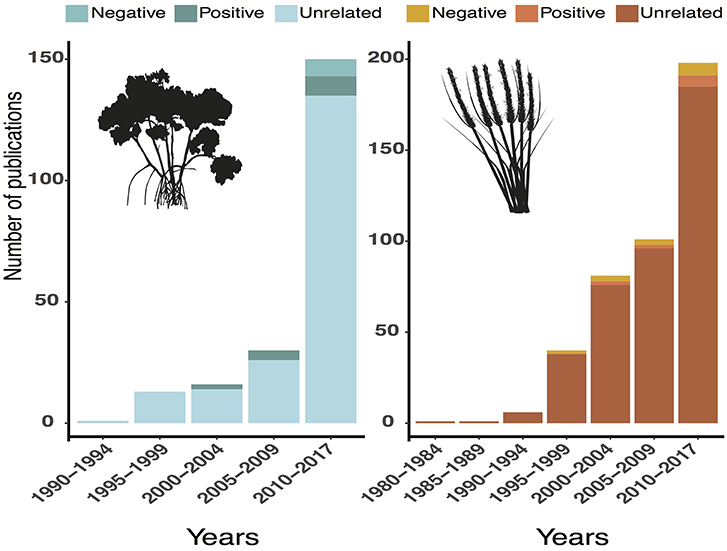
Figure 4. Results from a Web of Science literature review for articles about salt-marsh and mangrove restoration from 1980 to 2017. See acknowledgments for vector credits.
There were fewer studies conducted in mangrove than in salt-marsh ecosystems. Of the 605 relevant studies, 395 were about salt marshes, 177 were about mangroves, and 33 looked at both systems. The disproportionately low number of mangrove studies (less than half the number of salt-marsh studies) could partially explain why there are fewer documented trophic cascades and positive interactions in the current body of mangrove research. However, over the past few decades the proportion of mangrove relative to salt-marsh research has increased (Figure 5). The number of new relevant studies was relatively consistent from 1984 to 1995, with only a few studies coming out each year. Beginning around 1995, however, the annual number of new studies began increasing, with over 60 mangrove and salt-marsh restoration studies published in 2017 (Figure 5).
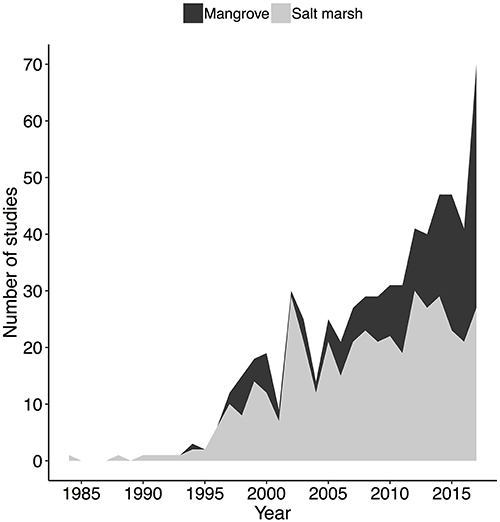
Figure 5. The number of studies from 1980 to 2017 that examined salt-marsh or mangrove restoration. Studies that examined both mangroves and salt marshes were included in both groups.
Over the past decade, in response to primary research showing the importance of positive species interactions in aquatic communities, there have been multiple calls to expand the traditional, abiotic- and competition-centric view of coastal wetland restoration in order to take advantage of positive interactions (Halpern et al., 2007; Silliman et al., 2015; Shaver and Silliman, 2017). Our review, however, suggests that positive interaction research and implementation is lacking in coastal wetland restoration science. Below we outline potential methods for harnessing positive interactions to increase coastal wetland efficiency and success.
Context-Dependent Clumping Design: Moving Away From a Past Paradigm to Reduce Physical Stress
According to the stress-gradient hypothesis (Bertness and Callaway, 1994), positive interactions are more prevalent, and more important, in stressful environments. In the case of high physical stress, positive interactions help lessen abiotic factors by making the local habitat more suitable. In the case of high consumer pressure, positive interactions become important in the form of associational defenses. At medium levels of stress, competitive interactions tend to dominate. This ecological theory can be translated into restoration practice by implementing a planting design that changes relative to abiotic stresses.
Some of the most prominent stressors in coastal wetlands come from tidal fluctuations. Organisms that live in the intertidal zone must be adapted to deal with an extreme range of temperature, moisture, oxygen, and salinity. For the purposes of restoration, stress can be approximated by tidal changes, with outplants planted more closely together where the environment is most stressful and outplants planted farther apart where competitive interactions are more likely to be important (Figure 6).
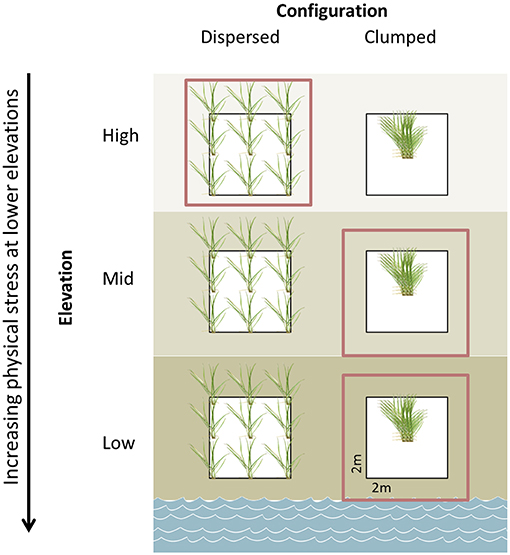
Figure 6. Visual depiction of clumped vs. dispersed restoration designs in a salt marsh with increasing physical stress along an elevation gradient. Red boxes indicate the more efficient method of planting. Created by Amanda Sonti.
In the intertidal zone, a dense restoration design fosters positive interactions between neighboring conspecifics. For example, clumped planting designs in salt marshes at small scales (1 m2) nearly doubled restoration success (Silliman et al., 2015), and mangrove restoration with a clumped design can result in significantly lower mortality when compared with a uniform design (Sofawi et al., 2017). Clumps of foundation species, such as cordgrass and mangroves, trap sediment, share oxygen, and reduce evaporation through shading. Bare ground can hamper restoration by lowering the redox potential of marsh soils (Mossman et al., 2012), which can be alleviated by denser clumping patters that promote sedimentation and allow colonization by other species (Castellanos et al., 1994). Dispersed designs, which have been prevalent in restoration, lose these benefits because these interactions act on such a local scale (e.g., oxygen leaking from the roots of one plant is only available to a close neighbor). Although less-studied, clumped designs may also allow outplants to reap the benefits of neighbors with different genotypes and promote resilience in periods of stress [e.g., in seagrasses: (Hughes and Stachowicz, 2009)]. However, where competitive interactions dominate, such as in the high intertidal zone where salt and oxygen stress tend to be lower, these interactions become more competitive. Competition for resources then outweighs the benefits provided by conspecifics and a clumped design could result in higher outplant mortality due to limited resources (Silliman et al., 2015). In this case, outplants should be planted in the traditional dispersed design. Therefore, a context-dependent clumping plan that changes dispersal distance with physical and consumer stress (Figure 6) has the potential to increase restoration yields as well as reduce restoration costs.
Holistic, Community-Level Restoration Design: Co-introducing Predators and Biodiverse Assemblages
In addition to restoring the physical and hydrologic regimes needed to sustain coastal wetlands, managers should restore biotic regimes that support critical foundation species in salt marshes and mangroves. Top predators often have disproportionate impacts on ecosystems (Duffy, 2003) and mediate many of the aforementioned trophic cascades. Although restoration managers have traditionally focused on bottom up controls on restoration (e.g., hydrologic regime, nutrient loading, etc.), top-down controls are vital to coastal wetland ecosystems (He and Silliman, 2016). Loss of predators from coastal wetlands may be one reason for the initial degradation, such as in salt marshes, where overfishing of predatory fish and crabs can lead to marsh die-off through a trophic cascade that facilitates runaway grazing on cordgrass. In these cases, restored coastal wetlands are unlikely to be able to fully recover without predator restoration or restoring wetlands in areas where predators are protected.
In other marine ecosystems, species richness has been shown to improve restoration outcomes (Williams et al., 2017), highlighting the importance of biodiverse assemblages in restoration. Passive restoration designs that rely on dispersal from nearby wetlands can limit restoration projects (e.g., river floodplain: (Bischoff, 2002); freshwater marsh: Neff and Baldwin, 2005) because of the relatively short dispersal distance of species, unless there is a close, well-developed wetland. By introducing a multifarious community of plants and animals along with the focal foundation species, managers introduce diverse services to the ecosystem, which support community development. This introduction leverages documented associational defenses in salt marshes and mangroves, while potentially also leveraging undocumented interactions. Given that positive interactions such as associational defenses are under-studied, introducing an array of organisms similar to the natural community allows for undescribed positive interactions to support wetland functioning. Further, dispersal from these species could potentially “spill-over” into neighboring communities and support populations outside of the restoration area—a phenomenon noted in marine reserves (Halpern and Warner, 2003).
Positive interactions among a suite of community members are likely important to wetland establishment and restoration success. Large-scale meta-analyses suggest that, even in highly diverse marine environments, there is little redundancy (Micheli and Halpern, 2005; Worm et al., 2006), and most organisms provide unique services in an ecosystem. For example, herbaceous plant species can facilitate mangrove restoration after a disturbance, with different species performing different functions, such as trapping propagules, providing abiotic stress ameliorations, and creating structural support (McKee et al., 2007). Along the same train of thought, positive interactions are not necessarily linear and some associated species may increase facilitation by other species. For example, the interactive effect of ribbed mussels and fiddler crabs on Spartina is greater when the two species are together than either alone (Hughes et al., 2014). Similarly, organisms associated with foundation species may only have observable positive effects when present together (e.g., Stachowicz and Whitlatch, 2005) and protective animals may be most effective in the presence of other co-defenders (e.g., McKeon et al., 2012). If biodiverse assemblages of associated species are co-introduced with focal foundation species, these positive interaction networks can be used to restore damaged ecosystems.
Systematic Incorporation of Positive Species Interactions Into Coastal Wetland Restoration
Positive interactions can be coupled with traditional restoration strategies to create a more efficient strategy for restoring coastal wetlands (Figure 7). When selecting a site, land managers should continue to consider abiotic factors, such as hydrologic and nutrient regimes, but also consider the proximity of a site to other wetlands that can help a developing community through dispersal and long-distance facilitation. Restoration groups can implement a context-dependent clumping design, where clumped designs are used in the most stressful parts of the site and dispersed designs are used in less stressful areas. Introducing both genetically diverse outplants and diverse species assemblages as part of the restoration plan should allow for a suite of positive interactions among wetland organisms and help facilitate the development of a natural community (see Table 1 for examples). Similarly, co-introducing predators, or choosing a site with healthy predator populations, may help increase the resilience of the ecosystem. Although co-introduction of all species would be ideal from an ecological perspective, it is financially prohibitive in many cases. Restoration managers will ultimately need to choose which species to introduce and there will need to be additional research on what introduction combinations are most effective for restoration goals. Beyond initial introductions, consistent monitoring and evaluation of restoration progress (biodiversity, ecosystem function, etc.) is vital to effective restoration. Results from monitoring should be leveraged to adapt restoration strategies as needed, can be used to develop accurate cost-benefit analyses for species co-introductions, and will further inform our understanding of how positive interactions influence coastal wetland restoration.
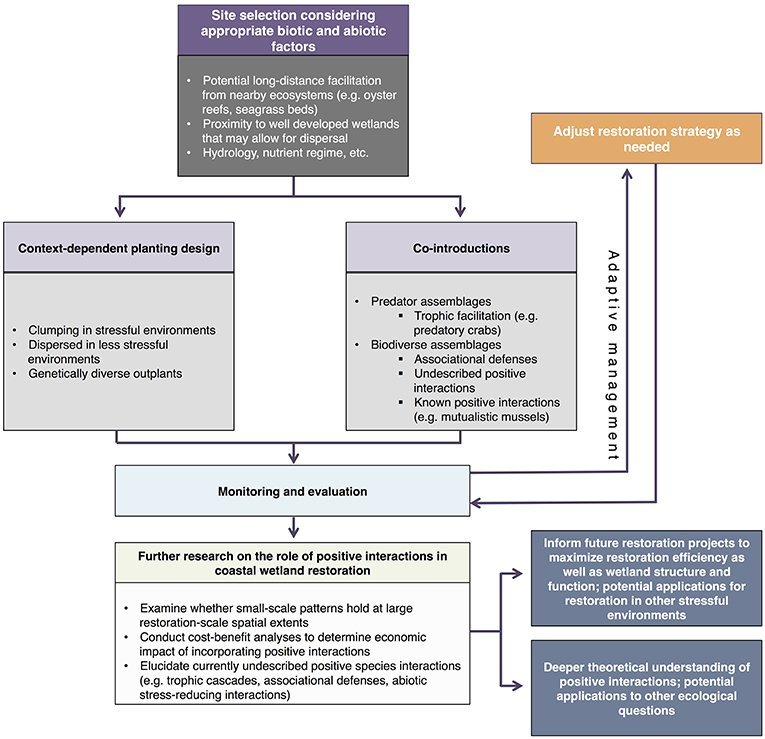
Figure 7. Theoretical diagram describing a potential workflow for systematically incorporating positive species interactions into restoration design.
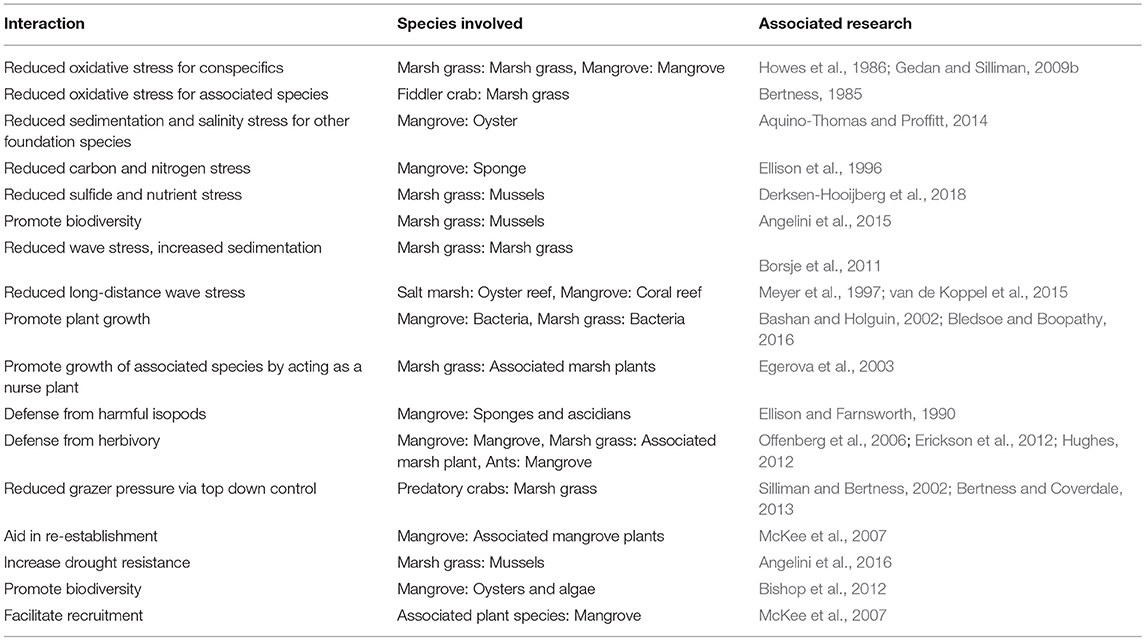
Table 1. Examples of positive species interactions in salt marshes and mangroves that may be useful for restoration managers.
Avenues for further investigation
Despite mounting evidence of the importance of positive interactions in coastal wetlands, surveys show that the negative interactions model is still an entrenched view in restoration organizations (Silliman et al., 2015). One potential reason for this lag is scale. Although studies on small scales have shown the potential benefit of positive interactions to restoration, there is no guarantee that these results will scale up to whole-wetland-sized projects. More experiments are needed at scales that are relevant to real-world restoration projects, both to better inform managers and to see whether new, unpredicted patterns emerge at larger experimental areas. For example, although many benefits of a clumped planting design are noticeable at small scales (e.g., oxygen benefits), others (e.g., wave attenuation) may be more evident with large experimental units. Large-scale experiments would also allow for a cost-benefit analysis that quantifies money saved—or lost—by incorporating positive interactions into restoration. These cost benefits could come in the form of labor reductions or resource reductions (e.g., fewer plants planted). Concrete numbers on potential economic savings could prove persuasive for restoration organizations considering incorporating facilitation into foundation species restoration projects.
Coastal wetlands are valued for their ability to sequester carbon, which is largely unparalleled in other ecosystems. Although carbon sequestration by wetlands is studied around the world, we have not quantified how positive interactions may change the ability of coastal wetlands to sequester carbon. This quantification could act as an economic mechanism for restoration, particularly when carbon incentives are in place. Given that positive interactions can alter plant productivity, plants may sequester more carbon through growth and given the heightened sediment-trapping abilities of closely planted species, higher planting densities can be related to higher sediment organic carbon levels (e.g., Chen et al., 2017). Facilitation in the form of predator reintroduction could also positively benefit blue carbon stocks (Atwood et al., 2015), which further emphasizes the importance of holistic, community-scale restoration designs for both ecosystem services (e.g., carbon sequestration) and community development.
Despite these gaps, there is a large body of evidence that supports the importance of positive interactions in coastal wetland functioning. While we focus on three categories of positive interactions, there are many instances of positive interactions present in wetlands (e.g., Figures 1, 2) and likely still more to be discovered, such as hitherto undescribed instances of long-distance facilitation and undescribed facilitation cascades. Harnessing the current research on positive interactions, along with insights from future research, to improve restoration is an untapped opportunity that could dramatically improve the outlook of wetland restoration—and the future of the vital ecosystem services they provide.
Author Contributions
BS conceived of the idea for the manuscript. JR wrote the initial draft, conducted the literature review, and created Figures 1–5 as well as Figure 7. QH and BS helped develop the concepts presented here, and supervised the project. All authors contributed to the fundamental ideas presented in and reviewing of the manuscript.
Funding
Funding was provided by the Lenfest Ocean Program Grant and JR was supported by a National Science Foundation Graduate Research Fellowship (NSF Grant DGE-1644868).
Conflict of Interest Statement
The authors declare that the research was conducted in the absence of any commercial or financial relationships that could be construed as a potential conflict of interest.
Acknowledgments
Non-original vector graphics for Figures 1–4 were adapted from Tracey Saxby, Dieter Tracey, Kim Kraeer, Lucy Van Essen-Fishman, Sally Bell, Jane Thomas, Chip Chenery, and Joanna Woerner. Integration and Application Network, University of Maryland Center for Environmental Science (http://ian.umces.edu/imagelibrary/). We would also like to thank the two reviewers that provided helpful feedback on the manuscript.
References
Aburto-Oropeza, O., Ezcurra, E., Danemann, G., Valdez, V., Murray, J., and Sala, E. (2008). Mangroves in the Gulf of California increase fishery yields. Proc Natl Acad Sci U.S.A. 105, 10456–10459. doi: 10.1073/pnas.0804601105
Altieri, A. H., Bertness, M. D., Coverdale, T. C., Herrmann, N. C., and Angelini, C. (2012). A trophic cascade triggers collapse of a salt-marsh ecosystem with intensive recreational fishing. Ecology 93, 1402–1410. doi: 10.1890/11-1314.1
An, S. Q., Gu, B. H., Zhou, C. F., Wang, Z. S., Deng, Z. F., Zhi, Y. B., et al. (2007). Spartina invasion in China: implications for invasive species management and future research. Weed Res. 47, 183–191. doi: 10.1111/j.1365-3180.2007.00559.x
Angelini, C., Griffin, J. N., van de Koppel, J., Lamers, L. P., Smolders, A. J., Derksen-Hooijberg, M., et al. (2016). A keystone mutualism underpins resilience of a coastal ecosystem to drought. Nat. Comm. 7:12473. doi: 10.1038/ncomms12473
Angelini, C., van der Heide, T., Griffin, J. N., Morton, J. P., Derksen-Hooijberg, M., Lamers, L. P., et al. (2015). Foundation species' overlap enhances biodiversity and multifunctionality from the patch to landscape scale in southeastern United States salt marshes. Proc. R. Soc. B. 282:1811. doi: 10.1098/rspb.2015.0421
Aquino-Thomas, J., and Proffitt, C. E. (2014). Oysters Crassostrea virginica on red mangrove Rhizophora mangle prop roots: facilitation of one foundation species by another. Mar. Ecol. Prog. Ser. 503, 177–194. doi: 10.3354/meps10742
Atwood, T. B., Connolly, R. M., Ritchie, E. G., Lovelock, C. E., Heithaus, M. R., Hays, G. C., et al. (2015). Predators help protect carbon stocks in blue carbon ecosystems. Nature Clim. Chan. 5, 1038–1045. doi: 10.1038/nclimate2763
Barner, A. K., Hacker, S. D., Menge, B. A., and Nielsen, K. J. (2016). The complex net effect of reciprocal interactions and recruitment facilitation maintains an intertidal kelp community. J. Ecol. 104, 33–43. doi: 10.1111/1365-2745.12495
Bashan, Y., and Holguin, G. (2002). Plant growth-promoting bacteria: a potential tool for arid mangrove reforestation. Trees 16, 159–166. doi: 10.1007/s00468-001-0152-4
Bayraktarov, E., Saunders, M. I., Abdullah, S., Mills, M., Beher, J., Possingham, H. P., et al. (2016). The cost and feasibility of marine coastal restoration. Ecol. App. 26, 1055–1074. doi: 10.1890/15-1077
Bertness, M. D. (1985). Fiddler crab regulation of Spartina alterniflora production on a New England salt marsh. Ecology 66, 1042–1055. doi: 10.2307/1940564
Bertness, M. D., and Callaway, R. (1994). Positive interactions in communities. Trends Ecol. Evol. 9, 191–193. doi: 10.1016/0169-5347(94)90088-4
Bertness, M. D., and Coverdale, T. C. (2013). An invasive species facilitates the recovery of salt marsh ecosystems on Cape Cod. Ecol. 94, 1937–1943. doi: 10.1890/12-2150.1
Bertness, M. D., Ewanchuk, P. J., and Silliman, B. R. (2002). Anthropogenic modification of New England salt marsh landscapes. Proc. Natl. Acad. Sci. U.S.A. 99, 1395–1398. doi: 10.1073/pnas.022447299
Bertness, M. D., and Grosholz, E. (1985). Population dynamics of the ribbed mussel, Geukensia demissa: the costs and benefits of an aggregated distribution. Oecologia 67, 192–204. doi: 10.1007/BF00384283
Bischoff, A. (2002). Dispersal and establishment of floodplain grassland species as limiting factors in restoration. Bio. Conserv. 104, 25–33. doi: 10.1016/S0006-3207(01)00151-3
Bishop, M. J., Byers, J. E., Marcek, B. J., and Gribben, P. E. (2012). Density-dependent facilitation cascades determine epifaunal community structure in temperate Australian mangroves. Ecology 93, 1388–1401. doi: 10.1890/10-2296.1
Biswas, S. R., Choudhury, J. K., Nishat, A., and Rahman, M. M. (2007). Do invasive plants threaten the Sundarbans mangrove forest of Bangladesh? Forest Ecol. Manag. 245, 1–9. doi: 10.1016/j.foreco.2007.02.011
Bledsoe, R., and Boopathy, R. (2016). Bioaugmentation of microbes to restore coastal wetland plants to protect land from coastal erosion. Internat. Biodeter. Biodeg. 113, 155–160. doi: 10.1016/j.ibiod.2016.02.010
Borja, Á., Dauer, D. M., Elliott, M., and Simenstad, C. A. (2010). Medium-and long-term recovery of estuarine and coastal ecosystems: patterns, rates and restoration effectiveness. Est. Coast. 33, 1249–1260. doi: 10.1007/s12237-010-9347-5
Borsje, B. W., van Wesenbeeck, B. K., Dekker, F., Paalvast, P., Bouma, T. J., van Katwijk, M. M., et al. (2011). How ecological engineering can serve in coastal protection. Ecol. Eng. 37, 113–122. doi: 10.1016/j.ecoleng.2010.11.027
Bruno, J. F., Stachowicz, J. J., and Bertness, M. D. (2003). Inclusion of facilitation into ecological theory. Trends Ecol. Evol. 18, 119–125. doi: 10.1016/S0169-5347(02)00045-9
Buelow, C., and Sheaves, M. (2015). A birds-eye view of biological connectivity in mangrove systems. Est. Coast. Shelf Sci. 152, 33–43. doi: 10.1016/j.ecss.2014.10.014
Byers, J. E. (2009). “Invasive animals in marshes” in Human Impacts on Salt Marshes: A Global Perspective, eds B. R. Silliman, E. Grosholz, and M. D. Bertness (Berkeley, CA: University of California Press), 41–56.
Callaway, J. C., Sullivan, G., and Zedler, J. B. (2003). Species-rich plantings increase biomass and nitrogen accumulation in a wetland restoration experiment. Ecol. App. 13, 1626–1639. doi: 10.1890/02-5144
Castellanos, E. M., Figueroa, M. E., and Davy, A. J. (1994). Nucleation and facilitation in saltmarsh succession: interactions between Spartina maritima and Arthrocnemum perenne. J. Ecol. 82, 239–248. doi: 10.2307/2261292
Chen, L., Zeng, X., Tam, N. F., Lu, W., Luo, Z., Du, X., et al. (2012). Comparing carbon sequestration and stand structure of monoculture and mixed mangrove plantations of Sonneratia caseolaris and S. apetala in Southern China. Forest Ecol. Manag. 284, 222–229. doi: 10.1016/j.foreco.2012.06.058
Chen, W., Ge, Z. M., Fei, B. L., Zhang, C., Liu, Q. X., and Zhang, L. Q. (2017). Soil carbon and nitrogen storage in recently restored and mature native Scirpus marshes in the Yangtze Estuary, China: implications for restoration. Ecol. Eng. 104, 150–157. doi: 10.1016/j.ecoleng.2017.04.027
Costa, C. B., Iribarne, O. O., and Farina, J. M. (2009). “Human impacts and threats to the conservation of South American salt marshes” in Human Impacts on Salt Marshes: a Global Perspective, eds. B. R. Silliman, E. Grosholz, and M. D.Bertness (Berkeley, CA: University of California Press), 337–359.
Craft, C., Clough, J., Ehman, J., Joye, S., Park, R., Pennings, S., et al. (2009). Forecasting the effects of accelerated sea-level rise on tidal marsh ecosystem services. Front. Ecol. Env. 7, 73–78. doi: 10.1890/070219
Crain, C. M., Gedan, K. B., and Dionne, M. (2009). “Tidal restrictions and mosquito ditching in New England marshes” in Human Impacts on Salt Marshes: a Global Perspective, eds. B. R. Silliman, E. Grosholz, and M. D. Bertness (Berkeley, CA: University of California Press), 149–69.
Daleo, P., Fanjul, E., Mendez Casariego, A., Silliman, B. R., Bertness, M. D., and Iribarne, O. (2007). Ecosystem engineers activate mycorrhizal mutualism in salt marshes. Ecol. Lett. 10, 902–908. doi: 10.1111/j.1461-0248.2007.01082.x
Das, S. (2017). Ecological restoration and livelihood: contribution of planted mangroves as nursery and habitat for artisanal and commercial fishery. World Dev. 94, 492–502. doi: 10.1016/j.worlddev.2017.02.010
Denno, R. F., Gratton, C., Peterson, M. A., Langellotto, G. A., Finke, D. L., and Huberty, A. F. (2002). Bottom-up forces mediate natural-enemy impact in a phytophagous insect community. Ecol. 83, 1443–1458. doi: 10.1890/0012-9658(2002)083[1443:BUFMNE]2.0.CO;2
Derksen-Hooijberg, M., Angelini, C., Lamers, L. P., Borst, A., Smolders, A., Hoogveld, J. R., et al. (2018). Mutualistic interactions amplify saltmarsh restoration success. J. App. Ecol. 55, 405–414. doi: 10.1111/1365-2664.12960
Dobson, A. P., Bradshaw, A. D., and Baker, A. Á. (1997). Hopes for the future: restoration ecology and conservation biology. Science 277, 515–522. doi: 10.1126/science.277.5325.515
Duffy, J. E. (2003). Biodiversity loss, trophic skew and ecosystem functioning. Ecol. Lett. 6, 680–687. doi: 10.1046/j.1461-0248.2003.00494.x
Duke, N. C. (2016). Oil spill impacts on mangroves: recommendations for operational planning and action based on a global review. Mar. Poll. Bullet. 109, 700–715. doi: 10.1016/j.marpolbul.2016.06.082
Egerova, J., Proffitt, C. E., and Travis, S. E. (2003). Facilitation of survival and growth of Baccharis halimifolia L. by Spartina alterniflora Loisel. in a created Louisiana salt marsh. Wetlands 23, 250–256. doi: 10.1672/4-20
Ellison, A., and Farnsworth, E. (1990). The ecology of Belizean mangrove-root fouling communities. I. Epibenthic fauna are barriers to isopod attack of red mangrove roots. J. Exp. Mar. Bio. Ecol. 142, 91–104. doi: 10.1016/0022-0981(90)90139-4
Ellison, A. M., Farnsworth, E. J., and Twilley, R. R. (1996). Facultative mutualism between red mangroves and root-fouling sponges in Belizean mangal. Ecology 77, 2431–2444. doi: 10.2307/2265744
Ellison, J. C., and Stoddart, D. R. (1991). Mangrove ecosystem collapse during predicted sea-level rise: holocene analogues and implications. J. Coast. Res. 7, 151–165.
Engel, S., and Pawlik, J. R. (2005). Interactions among Florida sponges. II. Mangrove habitats. Mar. Ecol. Prog. Ser. 303, 145–152. doi: 10.3354/meps303145
Erickson, A. A., Bell, S. S., and Dawes, C. J. (2012). Associational resistance protects mangrove leaves from crab herbivory. Acta Oecol. 41, 46–57. doi: 10.1016/j.actao.2012.04.002
FAO (2007). “The World's mangroves 1980-2005,” in FAO Forestry Paper 153. (Rome: Forest Resources Division, Food and Agriculture Organization of the United Nations), 77.
Finke, D. L., and Denno, R. F. (2006). Spatial refuge from intraguild predation: implications for prey suppression and trophic cascades. Oecologia 149, 265–275. doi: 10.1007/s00442-006-0443-y
Gallardo, B., Clavero, M., Sánchez, M. I., and Vilà, M. (2016). Global ecological impacts of invasive species in aquatic ecosystems. Glob. Change Bio. 22, 151–163. doi: 10.1111/gcb.13004
Gambrell, R. P. (1994). Trace and toxic metals in wetlands—a review. J. Env. Q. 23:883. doi: 10.2134/jeq1994.00472425002300050005x
Garbutt, A., and Wolters, M. (2008). The natural regeneration of salt marsh on formerly reclaimed land. App. Veg. Sci. 11, 335–344. doi: 10.3170/2008-7-18451
Gedan, K. B., Kirwan, M. L., Wolanski, E., Barbier, E. B., and Silliman, B. R. (2011). The present and future role of coastal wetland vegetation in protecting shorelines: answering recent challenges to the paradigm. Clim. Change 106, 7–29. doi: 10.1007/s10584-010-0003-7
Gedan, K. B., and Silliman, B. R. (2009a). “Patterns of salt marsh loss within coastal regions of North America: Presettlement to present.” in Human Impacts on Salt Marshes: A Global Perspective, eds B. R. Silliman, E. D. Grosholz, and M. D. Bertness (Los Angeles, CA: University of California Press), 253–266.
Gedan, K. B., and Silliman, B. R. (2009b). Using facilitation theory to enhance mangrove restoration. Ambio 38:109. doi: 10.1579/0044-7447-38.2.109
Gedan, K. B., Silliman, B. R., and Bertness, M. D. (2009). Centuries of human-driven change in salt marsh ecosystems. Ann. Rev. Mar. Sci. 1, 117–141. doi: 10.1146/annurev.marine.010908.163930
Gittman, R. K., and Keller, D. A. (2013). Fiddler crabs facilitate Spartina alterniflora growth, mitigating periwinkle overgrazing of marsh habitat. Ecology. 94, 2709–2718. doi: 10.1890/13-0152.1
Gratton, C., and Denno, R. F. (2005). Restoration of arthropod assemblages in a Spartina salt marsh following removal of the invasive plant Phragmites australis. Restorat. Ecol. 13, 358–372. doi: 10.1111/j.1526-100X.2005.00045.x
Gratton, C., and Denno, R. F. (2006). Arthropod food web restoration following removal of an invasive wetland plant. Ecol. App. 16, 622–631. doi: 10.1890/1051-0761(2006)016[0622:AFWRFR]2.0.CO;2
Halpern, B. S., Silliman, B. R., Olden, J. D., Bruno, J. P., and Bertness, M. D. (2007). Incorporating positive interactions in aquatic restoration and conservation. Front. Ecol. Env. 5, 153–160. doi: 10.1890/1540-9295(2007)5[153:IPIIAR]2.0.CO;2
Halpern, B. S., and Warner, R. R. (2003). Matching marine reserve design to reserve objectives. Proc. R. Soc. B. 270, 1871–1878. doi: 10.1098/rspb.2003.2405
Hay, M. E., Parker, J. D., Burkepile, D. E., Caudill, C. C., Wilson, A. E., Hallinan, Z. P., et al. (2004). Mutualisms and aquatic community structure: the enemy of my enemy is my friend. Ann. Rev. Ecol. Evo. Syst. 35, 175–197. doi: 10.1146/annurev.ecolsys.34.011802.132357
He, Q., Bertness, M. D., and Altieri, A. H. (2013). Global shifts towards positive species interactions with increasing environmental stress. Ecol. Lett. 16, 695–706. doi: 10.1111/ele.12080
He, Q., Bertness, M. D., Bruno, J. F., Li, B., Chen, G., Coverdale, et al. (2014). Economic development and coastal ecosystem change in China. Sci. Rep. 4:5995. doi: 10.1038/srep05995
He, Q., and Silliman, B. R. (2016). Consumer control as a common driver of coastal vegetation worldwide. Ecol. Mono. 86, 278–294. doi: 10.1002/ecm.1221
He, Q., Silliman, B. R., Liu, Z., and Cui, B. (2017). Natural enemies govern ecosystem resilience in the face of extreme droughts. Ecol. Lett. 20, 194–201. doi: 10.1111/ele.12721
Henry, H. A. L., and Jefferies, R. L. (2009). “Opportunistic herbivores, migratory connectivity, and catastrophic shifts in Arctic coastal systems” in Human Impacts on Salt Marshes: a Global Perspective, eds. B. R. Silliman, E. Grosholz, and M. D. Bertness (Berkeley, CA: University of California Press), 85–102.
Howes, B. L., Dacey, J. W. H., and Goehringer, D. D. (1986). Factors controlling the growth form of Spartina alterniflora: feedbacks between above-ground production, sediment oxidation, nitrogen and salinity. J. Ecol. 74, 881–898. doi: 10.2307/2260404
Hughes, A. R. (2012). A neighboring plant species creates associational refuge for consumer and host. Ecology 93, 1411–1420. doi: 10.1890/11-1555.1
Hughes, A. R., Moore, A. F., and Piehler, M. F. (2014). Independent and interactive effects of two facilitators on their habitat-providing host plant, Spartina alterniflora. Oikos 123, 488–499. doi: 10.1111/j.1600-0706.2013.01035.x
Hughes, A. R., and Stachowicz, J. J. (2009). Ecological impacts of genotypic diversity in the clonal seagrass Zostera marina. Ecology 90, 1412–1419. doi: 10.1890/07-2030.1
Hughes, B. B., Eby, R., Van Dyke, E., Tinker, M. T., Marks, C. I., Johnson, K. S., et al. (2013). Recovery of a top predator mediates negative eutrophic effects on seagrass. Proc. Natl. Acad. Sci. U.S.A. 110, 15313–15318. doi: 10.1073/pnas.1302805110
Hughes, B. B., Hammerstrom, K. K., Grant, N. E., Hoshijima, U., Eby, R., and Wasson, K. (2016). Trophic cascades on the edge: fostering seagrass resilience via a novel pathway. Oecologia 182, 231–241. doi: 10.1007/s00442-016-3652-z
Huxham, M., Kumara, M. P., Jayatissa, L. P., Krauss, K. W., Kairo, J., Langat, J., et al. (2010). Intra-and interspecific facilitation in mangroves may increase resilience to climate change threats. Philosoph. Transact. R. Soc. Lond. Bio. Sci. 365:2127. doi: 10.1098/rstb.2010.0094
Kaplan, I., and Denno, R. F. (2007). Interspecific interactions in phytophagous insects revisited: a quantitative assessment of competition theory. Ecol. Lett. 10, 977–994. doi: 10.1111/j.1461-0248.2007.01093.x
Kimbro, D. L. (2012). Tidal regime dictates the cascading consumptive and nonconsumptive effects of multiple predators on a marsh plant. Ecology 93, 334–344. doi: 10.1890/11-0596.1
Lang'at, J. K., Kirui, B. K., Skov, M. W., Kairo, J. G., Mencuccini, M., and Huxham, M. (2013). Species mixing boosts root yield in mangrove trees. Oecologia 172, 271–278. doi: 10.1007/s00442-012-2490-x
Lawrence, P. J., Smith, G. R., Sullivan, M. J., and Mossman, H. L. (2018). Restored saltmarshes lack the topographic diversity found in natural habitat. Ecol. Eng. 115, 58–66. doi: 10.1016/j.ecoleng.2018.02.007
Lipcius, R. N., Seitz, R. D., Seebo, M. S., and Colón-Carrión, D. (2005). Density, abundance and survival of the blue crab in seagrass and unstructured salt marsh nurseries of Chesapeake Bay. J. Exp. Mar. Bio. Ecol. 319, 69–80. doi: 10.1016/j.jembe.2004.12.034
McKee, K. L., Rooth, J. E., and Feller, I. C. (2007). Mangrove recruitment after forest disturbance is facilitated by herbaceous species in the Caribbean. Ecol. App. 17:1678. doi: 10.1890/06-1614.1
McKeon, C. S., Stier, A. C., McIlroy, S. E., and Bolker, B. M. (2012). Multiple defender effects: synergistic coral defense by mutualist crustaceans. Oecologia 169, 1095–1103. doi: 10.1007/s00442-012-2275-2
Mcleod, E., Chmura, G. L., Bouillon, S., Salm, R., Björk, M., Duarte, C. M., et al. (2011). A blueprint for blue carbon: toward an improved understanding of the role of vegetated coastal habitats in sequestering CO2. Front. Ecol. Env. 9, 552–560. doi: 10.1890/110004
Meli, P., Rey Benayas, J. M., Balvanera, P., and Martínez Ramos, M. (2014). Restoration enhances wetland biodiversity and ecosystem service supply, but results are context-dependent: a meta-analysis. PLoS ONE. 9:e93507. doi: 10.1371/journal.pone.0093507
Meyer, D. L., Townsend, E. C., and Thayer, G. W. (1997). Stabilization and erosion control value of oyster cultch for intertidal marsh. Restorat. Ecol. 5:93. doi: 10.1046/j.1526-100X.1997.09710.x
Micheli, F., and Halpern, B. S. (2005). Low functional redundancy in coastal marine assemblages. Ecol. Lett. 8, 391–400. doi: 10.1111/j.1461-0248.2005.00731.x
Minchinton, T. E., Simpson, J. C., and Bertness, M. D. (2006). Mechanisms of exclusion of native coastal marsh plants by an invasive grass. J. Ecol. 94, 342–354. doi: 10.1111/j.1365-2745.2006.01099.x
Mooney, K. A., and Agrawal, A. A. (2008). Plant genotype shapes ant-aphid interactions: implications for community structure and indirect plant defense. Am. Nat. 171:E195. doi: 10.1086/587758
Moreno-Mateos, D., Power, M. E., Comín, F. A., and Yockteng, R. (2012). Structural and functional loss in restored wetland ecosystems. PLoS Bio. 10:e1001247. doi: 10.1371/journal.pbio.1001247
Mossman, H. L., Brown, M. J., Davy, A. J., and Grant, A. (2012). Constraints on salt marsh development following managed coastal realignment: dispersal limitation or environmental tolerance? Restorat. Ecol. 20, 65–75. doi: 10.1111/j.1526-100X.2010.00745.x
Mumby, P. J. (2006). Connectivity of reef fish between mangroves and coral reefs: algorithms for the design of marine reserves at seascape scales. Bio. Conserv. 128, 215–222. doi: 10.1016/j.biocon.2005.09.042
Nagelkerken, I. S. J. M., Blaber, S. J. M., Bouillon, S., Green, P., Haywood, M., Kirton, L. G., et al. (2008). The habitat function of mangroves for terrestrial and marine fauna: a review. Aquat. Bot. 89, 155–185. doi: 10.1016/j.aquabot.2007.12.007
Neff, K. P., and Baldwin, A. H. (2005). Seed dispersal into wetlands: techniques and results for a restored tidal freshwater marsh. Wetlands 25, 392–404. doi: 10.1672/14
Nicholls, R. J., Hoozemans, F. M., and Marchand, M. (1999). Increasing flood risk and wetland losses due to global sea-level rise: regional and global analyses. Glob. Env. Chan. 9:S69. doi: 10.1016/S0959-3780(99)00019-9
Nieva, F. J. J., Castellanos, E. M., Castillo, J. M., and Figueroa, M. E. (2005). Clonal growth and tiller demography of the invader cordgrass Spartina densiflora Brongn. at two contrasting habitats in SW European salt marshes. Wetlands 25, 122–129. doi: 10.1672/0277-5212(2005)025[0122:CGATDO]2.0.CO;2
O'Beirn, F. X., Luchenbach, M. W., Nestlerode, J. A., and Coates, G. M. (2000). Toward design criteria in constructed oyster reefs: oyster recruitment as a function of substrate type and tidal height. J Shellfish Res. 19, 387–395.
Offenberg, J., Macintosh, D. J., and Nielsen, M. G. (2006). Indirect ant-protection against crab herbivory: damage-induced susceptibility to crab grazing may lead to its reduction on ant-colonized trees. Funct. Ecol. 20, 52–57. doi: 10.1111/j.1365-2435.2005.01059.x
Ouyang, X., and Guo, F. (2016). Paradigms of mangroves in treatment of anthropogenic wastewater pollution. Sci. Tot. Env. 544, 971–979. doi: 10.1016/j.scitotenv.2015.12.013
Palmer, M. A., Ambrose, R. F., and Poff, N. L. (1997). Ecological theory and community restoration ecology. Restorat. Ecol. 5, 291–300. doi: 10.1046/j.1526-100X.1997.00543.x
Possingham, H. P., Bode, M., and Klein, C. J. (2015). Optimal conservation outcomes require both restoration and protection. PLoS Bio. 13:e1002052. doi: 10.1371/journal.pbio.1002052
Proffitt, C. E., Travis, S. E., and Edwards, K. R. (2003). Genotype and elevation influence Spartina alterniflora colonization and growth in a created salt marsh. Ecol. App. 13, 180–192. doi: 10.1890/1051-0761(2003)013[0180:GAEISA]2.0.CO;2
Quinn, J. F., Wing, S. R., and Botsford, L. W. (1993). Harvest refugia in marine invertebrate fisheries: models and applications to the red sea urchin, Strongylocentrotus franciscanus. Amer. Zool. 33, 537–550. doi: 10.1093/icb/33.6.537
Rey Benayas, J. M., Newton, A. C., Diaz, A., and Bullock, J. M. (2009). Enhancement of biodiversity and ecosystem services by ecological restoration: a meta-analysis. Science 325, 1121–1124. doi: 10.1126/science.1172460
Schuerch, M., Spencer, T., Temmerman, S., Kirwan, M. L., Wolff, C., Lincke, D., et al. (2018). Future response of global coastal wetlands to sea-level rise. Nature 561, 231–234. doi: 10.1038/s41586-018-0476-5
Shanholtzer, G. F. (2012). “Relationship of Vertebrates to Salt Marsh Plants” in Ecology of Halophytes, ed R. J. Mold (New York, NY: Elsevier), 463–474.
Shaver, E. C., and Silliman, B. R. (2017). Time to cash in on positive interactions for coral restoration. PeerJ. 5:e3499. doi: 10.7717/peerj.3499
Silliman, B. R., and Bertness, M. D. (2002). A trophic cascade regulates salt marsh primary production. Proc. Natl. Acad. Sci. U.S.A. 99:10500. doi: 10.1073/pnas.162366599
Silliman, B. R., and Bertness, M. D. (2004). Shoreline development drives invasion of Phragmites australis and the loss of plant diversity on New England salt marshes. Conserv. Bio. 18, 1424–1434. doi: 10.1111/j.1523-1739.2004.00112.x
Silliman, B. R., Dixon, P. M., Wobus, C., He, Q., Daleo, P., Hughes, B. B., et al. (2016). Thresholds in marsh resilience to the Deepwater Horizon oil spill. Sci. Rep. 6:32520. doi: 10.1038/srep32520
Silliman, B. R., McCoy, M. W., Angelini, C., Holt, R. D., Griffin, J. N., and van de Koppel, J. (2013). Consumer fronts, global change, and runaway collapse in ecosystems. Ann. Rev. Ecol. Evol. Syst. 44, 503–538. doi: 10.1146/annurev-ecolsys-110512-135753
Silliman, B. R., Schrack, E., He, Q., Cope, R., Santoni, A., van der Heide, T., et al. (2015). Facilitation shifts paradigms and can amplify coastal restoration efforts. Proc. Natl. Acad. Sci. U.S.A. 112:14295. doi: 10.1073/pnas.1515297112
Silliman, B. R., Van De Koppel, J., Bertness, M. D., Stanton, L. E., and Mendelssohn, I. A. (2005). Drought, snails, and large-scale die-off of southern US salt marshes. Science 310, 1803–1806. doi: 10.1126/science.1118229
Sofawi, A. B., Rozainah, M. Z., Normaniza, O., and Roslan, H. (2017). Mangrove rehabilitation on Carey Island, Malaysia: an evaluation of replanting techniques and sediment properties. Mar. Bio. Res. 13, 390–401. doi: 10.1080/17451000.2016.1267365
Stachowicz, J. J. (2001). Mutualism, facilitation, and the structure of ecological communities: positive interactions play a critical, but underappreciated, role in ecological communities by reducing physical or biotic stresses in existing habitats and by creating new habitats on which many species depend. AIBS Bull. 51, 235–246. doi: 10.1641/0006-3568(2001)051[0235:MFATSO]2.0.CO;2
Stachowicz, J. J., and Whitlatch, R. B. (2005). Multiple mutualists provide complementary benefits to their seaweed host. Ecology 86, 2418–2427. doi: 10.1890/04-0819
Stieglitz, T., Ridd, P., and Müller, P. (2000). Passive irrigation and functional morphology of crustacean burrows in a tropical mangrove swamp. Hydrobiology 421, 69–76. doi: 10.1023/A:1003925502665
Strong, D. R., and Ayres, D. R. (2009). “Spartina Introductions and Consequences in Salt Marshes” in Human Impacts on Salt Marshes: A Global Perspective, eds B. R. Silliman, E. Grosholz, and M. D. Bertness (Berkeley, CA: University of California Press), 3–22.
Tsai, C., Yang, S., Trimble, A. C., and Ruesink, J. L. (2010). Interactions between two introduced species: Zostera japonica (dwarf eelgrass) facilitates itself and reduces condition of Ruditapes philippinarum (Manila clam) on intertidal flats. Mar. Bio. 157, 1929–1936. doi: 10.1007/s00227-010-1462-0
Tyler, A. C., Lambrinos, J. G., and Grosholz, E. D. (2007). Nitrogen inputs promote the spread of an invasive marsh grass. Ecol. App. 17, 1886–18898. doi: 10.1890/06-0822.1
Valiela, I., Bowen, J. L., and York, J. K. (2001). Mangrove forests: one of the World's threatened major tropical environments: at least 35% of the area of mangrove forests has been lost in the past two decades, losses that exceed those for tropical rain forests and coral reefs, two other well-known threatened environments. AIBS Bull. 51, 807–815. doi: 10.1641/0006-3568(2001)051[0807:MFOOTW]2.0.CO;2
van de Koppel, J., van der Heide, T., Altieri, A. H., Eriksson, B. K., Bouma, T. J., Olff, H., et al. (2015). Long-distance interactions regulate the structure and resilience of coastal ecosystems. Ann. Rev. of Mar. Sci. 7, 139–158. doi: 10.1146/annurev-marine-010814-015805
Veeneklaas, R. M., Dijkema, K. S., Hecker, N., and Bakker, J. P. (2013). Spatio-temporal dynamics of the invasive plant species Elytrigia atherica on natural salt marshes. App. Veg. Sci. 16:205. doi: 10.1111/j.1654-109X.2012.01228.x
Whitcraft, C. R., and Levin, L. A. (2007). Regulation of benthic algal and animal communities by salt marsh plants: impact of shading. Ecology 88, 904–917. doi: 10.1890/05-2074
Williams, S. L. (1990). Experimental studies of Caribbean seagrass bed development. Ecol. Mono. 60, 449–469. doi: 10.2307/1943015
Williams, S. L., Ambo-Rappe, R., Sur, C., Abbott, J. M., and Limbong, S. R. (2017). Species richness accelerates marine ecosystem restoration in the Coral Triangle. Proc. Natl. Acad. Sci. U.S.A. 114, 11986–11991. doi: 10.1073/pnas.1707962114
Worm, B., Barbier, E. B., Beaumont, N., Duffy, J. E., Folke, C., Halpern, B. S., et al. (2006). Impacts of biodiversity loss on ocean ecosystem services. Science 314, 787–790. doi: 10.1126/science.1132294
Yang, Q., Tam, N. F., Wong, Y. S., Luan, T. G., Su, W. S., Lan, C. Y., et al. (2008). Potential use of mangroves as constructed wetland for municipal sewage treatment in Futian, Shenzhen, China. Mar. Poll. Bullet. 57, 735–743. doi: 10.1016/j.marpolbul.2008.01.037
Young, T. P., Petersen, D. A., and Clary, J. J. (2005). The ecology of restoration: historical links, emerging issues and unexplored realms. Ecol. Lett. 8, 662–673. doi: 10.1111/j.1461-0248.2005.00764.x
Zengel, S., Pennings, S. C., Silliman, B., Montague, C., Weaver, J., Deis, D. R., et al. (2016). Deepwater Horizon oil spill impacts on salt marsh fiddler crabs (Uca spp.). Estu. Coas. 39, 1154–1163. doi: 10.7266/N7FF3Q9S
Zerebecki, R. A., Crutsinger, G. M., and Hughes, A. R. (2017). Spartina alterniflora genotypic identity affects plant and consumer responses in an experimental marsh community. J. Ecol. 105, 661–673. doi: 10.1111/1365-2745.12703
Zhang, X. D., Jia, X., Chen, Y. Y., Shao, J. J., Wu, X. R., Shang, L., et al. (2013). Crabs mediate interactions between native and invasive salt marsh plants: a mesocosm study. PLoS ONE 8:e74095. doi: 10.1371/journal.pone.0074095
Keywords: salt marshes, mangroves, restoration, positive interactions, facilitation, coastal wetlands
Citation: Renzi JJ, He Q and Silliman BR (2019) Harnessing Positive Species Interactions to Enhance Coastal Wetland Restoration. Front. Ecol. Evol. 7:131. doi: 10.3389/fevo.2019.00131
Received: 30 September 2018; Accepted: 01 April 2019;
Published: 18 April 2019.
Edited by:
Martin Wiggers Skov, Bangor University, United KingdomReviewed by:
Julien Pétillon, University of Rennes 1, FranceAnna R. Armitage, Texas A&M University, United States
Copyright © 2019 Renzi, He and Silliman. This is an open-access article distributed under the terms of the Creative Commons Attribution License (CC BY). The use, distribution or reproduction in other forums is permitted, provided the original author(s) and the copyright owner(s) are credited and that the original publication in this journal is cited, in accordance with accepted academic practice. No use, distribution or reproduction is permitted which does not comply with these terms.
*Correspondence: Julianna J. Renzi, anVsaWFubmEucmVuemlAZHVrZS5lZHU=