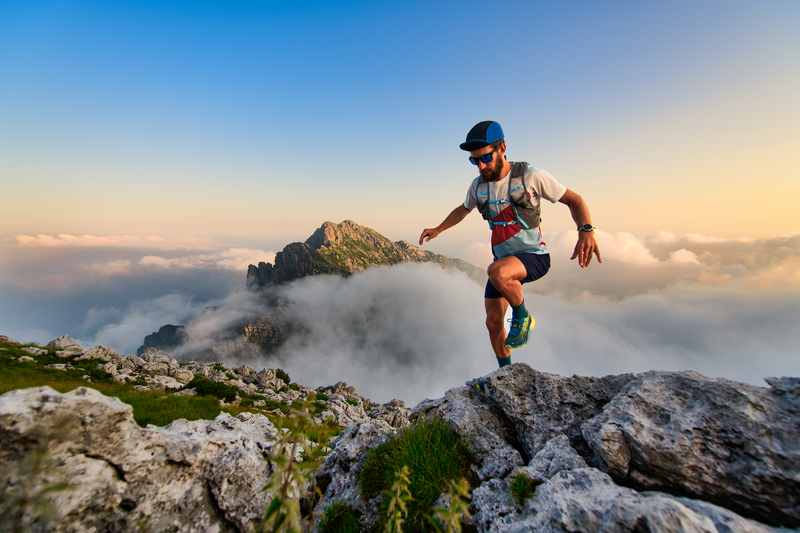
95% of researchers rate our articles as excellent or good
Learn more about the work of our research integrity team to safeguard the quality of each article we publish.
Find out more
SYSTEMATIC REVIEW article
Front. Ecol. Evol. , 20 March 2019
Sec. Behavioral and Evolutionary Ecology
Volume 7 - 2019 | https://doi.org/10.3389/fevo.2019.00072
This article is part of the Research Topic Integrating Predation Risk Across Scales: from Neurons to Ecosystems and Milliseconds to Generations View all 16 articles
Ecosystems are shaped by complex interactions between species and their environment. However, humans are rapidly changing the environment through increased carbon dioxide (CO2) emissions, creating global warming and elevated CO2 levels that affect ecological communities through multiple processes. Understanding community responses to climate change requires examining the consequences of changing behavioral interactions between species, such as those affecting predator and prey. Understanding the underlying sensory process that govern these interactions and how they may be affected by climate change provides a predictive framework, but many studies examine behavioral outcomes only. This review summarizes the current knowledge of global warming and elevated CO2 impacts on predator-prey interactions with respect to the relevant aspects of sensory ecology, and we discuss the potential consequences of these effects. Our specific questions concern how climate change affects the ability of predators and prey to collect information and how this affects predator-prey interactions. We develop a framework for understanding how warming and elevated CO2 can alter behavioral interactions by examining how the processes (steps) of sensory cue (or signal) production, transmission and reception may change. This includes both direct effects on cue production and reception resulting from changes in organismal physiology, but also effects on cue transmission resulting from modulation of the physical environment via physical and biotic changes. We suggest that some modalities may be particularly prone to disruption, and that aquatic environments may suffer more serious disruptions as a result of elevated CO2 and warming that collectively affect all steps of the signaling process. Temperature by itself may primarily operate on aspects of cue generation and transmission, implying that sensory-mediated disruptions in terrestrial environments may be less severe. However, significant biases in the literature in terms of modalities (chemosensation), taxa (fish), and stressors (elevated CO2) examined currently prevents accurate generalizations. Significant issues such as multimodal compensation and altered transmission or other environmental effects remain largely unaddressed. Future studies should strive to fill these knowledge gaps in order to better understand and predict shifts in predator-prey interactions in a changing climate.
Ecological communities are regulated by key biotic interactions (e.g., predation, competition, mutualism, and parasitism) that are affected by information obtained from and affected by the physical and biological environment (Dusenberry, 1992). In particular, predator-prey interactions are powerful regulators of community dynamics (Paine, 1966; Ripple et al., 2001; Schmitz, 2008) and these interactions can depend on behaviors mediated by sensory cues passing between predators and prey. Thus, processes governing the production, transmission, and detection of sensory cues from other organisms strongly affect predator-prey dynamics. Animals use these cues to respond in the appropriate way; i.e., a predator that successfully detects a prey species must be able to pursue and capture it, while prey detecting a predator must be able to employ the appropriate anti-predator defense. Therefore, sensory processes govern, at least partially, the density mediated effects that depend on predators consuming prey (consumptive effects) as well as those that depend on prey responses to predator risk such as flight or induced morphological defenses (non-consumptive effects) (Werner and Peacor, 2003; Weissburg et al., 2014).
The properties of both senders and receivers affect information transfer between predators and prey, but the environment also has an important role. Environmental context affects predator-prey dynamics by modulating the ability of predator and prey to detect one another. For example, in marine systems the fluid environmental context (e.g., bulk flow and turbulence) can reduce consumptive effects by interfering with the ability of predators to find prey using chemical signals (Weissburg and Zimmerfaust, 1994). Fluid motion also can increase non-consumptive effects by enhancing the ability of prey to remotely detect predators via predator scent (Leonard et al., 1998; Shears et al., 2008; Smee et al., 2010; Pruett and Weissburg, 2018). The nature of these effects depends on the intensity and characteristics of the flow environment. Generally speaking, community interactions must be placed in an environmental context to fully understand ecosystem dynamics.
Anthropogenic impacts will strongly affect the environmental context in which predator-prey sensory processes operate. Increased carbon dioxide (CO2) emissions from human activities (e.g., burning of fossil fuels) have caused global atmospheric temperatures to increase over the recent years in both terrestrial and marine systems. Mean temperature increases likely will exceed an additional 2°C by year 2100 (IPCC, 2014). Additionally, oceans and coastal systems (and some freshwater systems) are experiencing direct changes in water chemistry known as ocean acidification (Doney et al., 2009), where increased dissolved CO2 shifts the balance of carbonates to produce more bicarbonate and less carbonate ions, while also reducing pH of seawater by 0.3–0.4 units by 2100 (Orr et al., 2005; IPCC, 2014) from the current value of roughly 8.1.
The changing environmental conditions associated with elevated CO2 and increased temperature can affect the processes of cue generation, propagation, and reception underlying predator prey dynamics (i.e., the sensory transduction cascade), or place limits on the subsequent behavioral responses. Although there has been increasing attention to alterations in predator foraging rates and behavioral responses to climate change across taxa (Briffa et al., 2012; Rosenblatt and Schmitz, 2016; Beever et al., 2017), these studies often fail to indicate clearly the mechanism. We are just beginning to understand how climate change alters sensory processes and the consequences of such alterations for predator-prey interactions. But this research agenda is especially important given the potential of climate-induced change in species interactions to scale up to community effects (e.g., Goldenberg et al., 2018). It also may inform species and environmental conservation efforts by identifying which sensory modalities drive predator-prey behaviors in a changing climate and potential mitigation strategies.
This review is an initial step in developing a framework for studying impacts of climate change (i.e., warming and elevated CO2) on sensory and behavioral mechanisms altering predator-prey interactions. We review the current research on climate change effects on predator prey interactions in light of the general processes governing cue production, transmission, reception, and response. Our goals are to suggest which elements in this sequence are affected, how they may be affected, and whether there are modality-specific patterns in climate change effects. We also attempt to distinguish between effects due to increased temperature and elevated CO2.
We conducted comprehensive literature searches in Web of Science and Google Scholar for publications within the last 20 years using the keywords “climate change,” “carbon dioxide/CO2” “warming,” “acidification,” “sensory/visual/auditory/chemosensory/olfactory/mechanosensory,” “predator/prey,” and “behavior.” We sought studies from terrestrial, freshwater, and marine environments that tested some component of sensory ecology in any taxon (i.e., fish, insects, other invertebrates), which resulted in ~60 relevant studies. We then supplemented our search to include relevant publications from the references sections of papers from the initial literature search, but that did not have these keywords explicitly listed in the title, keywords, or abstract (~10 additional studies). We also included relevant papers that have cited studies from the initial search (i.e., forward citation search; ~15 additional studies), and several topical reviews on behavioral responses to climate change stressors. We then read the abstracts to exclude studies based on the following criteria: (1) while we recognize the large body of knowledge documenting physiological responses to climate change, studies that did not include sensory-based behavioral metrics relevant to predator-prey responses were excluded; (2) we focused on studies examining how cues from predators or prey affected interactions and we excluded any study where the cues eliciting behavior were not obviously connected to prey finding or predator sensing; (3) freshwater studies that did not explicitly test CO2-induced acidification (i.e., conditions in naturally acidic streams) were excluded (~5 studies), as acidification in freshwater systems can also occur from acid rain and thus possibly confound analyses in this review; (4) terrestrial studies involving herbivore-plant interactions were not explicitly considered as this is a specific subfield and signaling aspects of climate change effects have been reviewed recently (Peñuelas and Staudt, 2010; Ode et al., 2014).
We identified 57 studies that matched our criteria and which document an effect of climate change (i.e., warming and/or elevated CO2) on a component of the sensory transduction cascade governing predator-prey interactions. We categorized these studies by sensory modality and sensory step affected, species tested, and relevant habitat, and climate change stressor; and we summarized the results of each study (Table 1). We synthesize emerging global patterns from these studies below, and we recognize that it is possible that we did not include every study that fit our criteria due to synonymous terminology used to describe these studies. We also included in our discussion papers on general sensory biology relevant to our review that gave insight to sensory processes impacted by climate change.
Table 1. Summary of studies on impacts of warming and elevated CO2 on sensory behavior in predator-prey interactions.
One emerging pattern identified from our analysis is that warming and elevated CO2 affect interactions targeting different components of the sensory transduction pathway: warming largely will affect consumptive effects by physiological changes in activity and metabolic rates (with some effects on cue production, transmission, and reception), while elevated CO2 largely can affect non-consumptive effects by behavioral changes in the sensory transduction pathway (with some effects on metabolism), changing various aspects of predator-prey information exchange (Figure 1). However, many investigations only examine behavioral endpoints and not specific mechanisms, and literature is biased toward a few environments and taxonomic groups, preventing robust generalizations. Further, the ecological consequences often will depend on how both predator and prey are affected, since both use information about the presence of the other to regulate their behavior. Almost no studies examine how the sensory ecology of predator-prey interactions may or may not change when predators and prey both are challenged with warming and/or elevated CO2. We discuss these issues below and how climate change stressors affect information exchange and interactions governed by specific modalities.
Figure 1. Effects of global warming and elevated CO2 on the sensory transduction pathway governing predator-prey interactions. The sender controls cue production and emission into the environment, which modulates cue transmission to the receiver. The receiver controls detection and processing of cue to determine behavioral response. Elevated CO2 can directly affect sensory behavior via changes in cue production by the sender, transmission through the environment, and detection and processing by the receiver. Warming can affect cue production indirectly via physiological changes in activity and metabolic rates and can directly affect cue transmission. Note in this depiction that the sender is predator and the receiver is prey, but cues also pass from prey to predator so the roles also can be reversed. Symbols for diagrams courtesy of the Integration and Application Network, University of Maryland Center for Environmental Science (ian.umces.edu/symbols).
On a basic level we may distinguish effects of climate change that depend on general changes to metabolic processes vs. those that operate by changing the production, transmission, and reception of sensory cues (Figure 1). Increased temperatures may change predator-prey interactions by increasing metabolic rates of predators, therefore increasing foraging demand in order to meet the same requirements for a given growth rate. This has been demonstrated in multiple species of fish and crustacean consumers (Wu et al., 2017; Goldenberg et al., 2018) and sharks (Pistevos et al., 2015). A similar phenomenon occurs in prey species, although the effect is reversed; warming has been shown to reduce prey activity (Kidawa et al., 2010) and suppress prey metabolism (Paganini et al., 2014), potentially reducing escape responses from predators. Since predator consumptive effects are based on activity levels of both predator and prey, warming may increase predator consumptive effects via changes to metabolic processes (but see Miller et al., 2014 for warming increasing non-consumptive effects). However, activity level also may affect the production of sensory cues that mediate predator-prey interactions. Movement, for instance, produces mechanical and visual cues that both predators and prey may use. Thus, warming may indirectly affect cue production via changes in metabolism or activity and has some direct effects on cue transmission (see below).
Elevated CO2 can affect directly all elements in the transduction cascade and may strongly impact sensory processes in receivers that determine the ability of animals to detect and process cues, although this conclusion is based on limited studies focused on chemosensation (Table 1). Both predators and prey may be affected. Smooth dogfish shark predators (Mustelus canis) in high CO2 conditions avoid food chemical cues while their general activity levels remain unaffected, suggesting a deficit in processing (Dixson et al., 2015). Elevated CO2 also can decrease foraging success of Port Jackson shark predators (Heterodontus portusjacksoni) by dramatically increasing time to chemically locate prey (although it is unclear if this is a result of changes to cue production, detection or processing), thus leading to reduced growth rates overall (Pistevos et al., 2015). Prey species also may show reduced ability to identify predators, leading to behaviors that increase their susceptibility. Pea aphids under increased CO2 produce less alarm pheromone and respond less when exposed to alarm pheromones (Boullis et al., 2017). Simpson et al. (2011) report that high CO2 reduces the response of clown fish to predator sounds, and degraded reefs are known to have significantly different sound scales than healthy ones (Gordon et al., 2018). Elevated CO2 also decreases the intensity of anti-predator behaviors in juvenile damselfish in response to visual predation risk (Ferrari et al., 2012).
Elevated CO2 may cause some indirect physiological effects with behavioral consequences for predator-prey interactions. High CO2 levels can indirectly decrease foraging success of brown crab predators (Cancer pagurus) through increased resting metabolic rates and thus less energy allocated to foraging behavior (Wang et al., 2018). Thick shell mussels (Mytilus coruscus) also experience reduced excretion rates in elevated CO2 conditions, which may indirectly affect chemical cues used by predators although this has not been tested (Wang et al., 2015). Despite substantial studies on physiological changes in response to climate change stressors (Pörtner and Farrell, 2008; Kroeker et al., 2013), it is not yet clear how often these indirect effects occur or their importance in the sensory transduction pathway.
Sensory behavior involves information transfer via a series of steps from the sender to receiver: production and release of cue, transmission through the environment, detection and processing of cue (collectively known as cue reception), and behavioral response (Figure 1, Table 1). These steps are all important in regulating predator-prey interactions (Weissburg et al., 2014). Cue production is dependent on characteristics of the sender; for example, acoustic or visual cues depend on movement. Cue emission is closely related to cue production, which as defined here corresponds to cue intensity, and emission duration and frequency. The environment plays a large role in transmitting sensory cues and consequently has great potential to alter cue intensity and persistence (Smee et al., 2010; Weissburg et al., 2014), which modulates how well the cue can be perceived against background “noise.” Cue intensity diminishes as it propagates through the environment, and other properties also may change to affect how or whether the cue may be perceived (Table 2). For example, physical properties of water attenuate light, lowering light levels in deeper waters in a frequency-dependent manner to shift the color spectrum toward blues (Dusenberry, 1992). This reduces contrast and the ability to detect particular colors. The chemical, spectral and auditory environments can therefore prevent cues from being detected or discriminated from the background, a process we refer to generally as “masking” (Dusenberry, 1992). Note that masking can occur directly as a result of environmental changes or indirectly when the environment changes biotic factors that in turn affect background sensory properties. For instance, terrestrial environments differ in background spectral quality as a result of the interaction of biological features and physical properties, which makes certain colors stand out and masks others (Endler, 1993). A receiver able to detect a cue and distinguish it from the environment uses this information to detect potential prey or predation risk and respond appropriately to the sender. Climate change (i.e., warming and elevated CO2) has the potential to directly or indirectly affect any of these sensory steps (Figure 1), and the sections below discuss modality-specific effects (Table 2).
Sound perception occurs over somewhat large spatial scales (i.e., tens of meters), and can be used to detect habitats or potential predators or prey (Weissburg et al., 2014). Sound speed and distance traveled is dependent on density of medium, nearby objects, and potential mixing forces (Dusenberry, 1992). Elevated CO2 has been the focal stressor of the limited studies examining changes in behavior to auditory cues (Table 2, Figure 2), although there is theoretical evidence for warming effects (Table 3). Warming may indirectly affect auditory cue production via changes in metabolism and activity levels that would affect how much or how often sound is produced. These effects may occur in interactions such as bat predators detecting prey in cluttered environments where echolocation is not effective, and where detection and localization of prey is modulated by prey-generated sounds (Arlettaz et al., 2001). However, these effects of warming on auditory cue production remain unexplored. Elevated CO2 also reduces the frequency and intensity of predatory snapping sounds (Rossi et al., 2016a). General activity levels in this case were not affected by elevated CO2 but other potentially confounding metabolic effects were not examined (see below).
Figure 2. Percentages of studies on predator-prey sensory processes and climate change by (a) sensory modality, (b) climate change stressor, (c) ecosystem, and (d) organism type.
Warming has a direct effect on auditory cue transmission; sound speed in seawater increases by ~4 ms−1 per 1°C rise in ocean temperature (Munk and Forbes, 1989), and an increased sound speed of 0.6 ms−1 per 1°C temperature rise in air. Since auditory location depends partially on time of arrival differences at sound-receiving organs, increasing the speed of sound may reduce the ability of animals (particularly small ones), to discern sound direction (Miles et al., 1995). Sound attenuation also is predicted to change with warming, which has consequences for animals that use sounds to detect other organisms. Global warming will change sound transmission of echolocating bats, and the direction of change depends on call frequency and local climate conditions; bats living in temperate regions and producing higher frequency calls will lose prey detection space, while tropical bats with lower frequency calls will be able to detect more prey (Luo et al., 2013). Changes in community composition are likely to result from these changes in sound transmission, with stronger impacts in temperate regions. Ambient or background noise also may directly increase as a result of ocean acidification as the environment can modulate reflection and attenuation of sounds; increased CO2 will reduce sound absorption in the oceans, particularly for low-frequency sounds (<10 kHz), via shifts in chemical reactions of sound-absorbing compounds (e.g., magnesium sulfate and boric acid), making for a noisier environment that reduces transmission and detection of ecologically relevant sounds such as hearing and communication in cetaceans (Hester et al., 2008; Ilyina et al., 2010). In combination with warming, an ocean pH reduction of 0.3–0.6 units will reduce absorption of sounds below 1 kHz by almost 40–60%, especially in regions that receive increased amounts of low-frequency noise from human activities such as shipping (Hester et al., 2008; Ilyina et al., 2010).
Elevated CO2 also can directly change the receptors of auditory cues such as otoliths used by fishes to detect sound waves. Bignami et al. (2013) found that ocean acidification increases otolith size and auditory sensitivity; despite the potential benefit of detecting relevant cues with larger auditory receiving structures, hypersensitivity may impair the ability to discriminate these cues from “background noise.” This would make it more difficult to locate food or avoid predators using auditory cues.
In addition to affecting the sound reception organ, elevated CO2 also can affect auditory processing. Juvenile clownfish (Amphiprion percula) in ambient conditions avoid daytime reef habitat noise indicative of predation risk. However, clownfish in high-CO2 laboratory conditions showed neither avoidance of, nor attraction to, habitat noise. The lack of response was not due to changes in the sound reception organ; otolith morphology was unaffected suggesting an inability to process the auditory inputs effectively (Simpson et al., 2011). These results have important implications for habitat selection to minimize predation risk, and of course also affect responses to attractive auditory stimuli. Habitat sounds can be attractive cues used for orientation during settlement, with elevated CO2 levels reducing these attractive behaviors as demonstrated in settlement-stage tropical and temperate fishes of several species (Rossi et al., 2015, 2016b). Reduced responses to both attractive and aversive auditory cues suggest deficits in cue detection or processing may be the sensory mechanism in these cases, although this remains to be explicitly tested using physiological investigations of sensory receptor responses or central nervous system function (e.g., Nilsson et al., 2012; Porteus et al., 2018).
Chemical cues are prevalent in sensory-mediated predator-prey behaviors and may mediate detection of both prey (Weissburg et al., 2002) and predators (Kats and Dill, 1998) as these cues have the potential to persist over large distances (i.e., tens of meters) and long times (i.e., minutes) (Weissburg et al., 2014). Chemical cue production and emission is controlled by the sender; prey often determine predation risk from chemical waste products unavoidably released by predators (Scherer and Smee, 2016; Poulin et al., 2018) or by cues released by conspecific prey (e.g., alarm pheromones). The amount of cue produced can be an indicator of biomass and thus predator threat (Hill and Weissburg, 2013), and predator diet can change the identity of metabolites released by predators (Poulin et al., 2018) to chemically influence risk assessment by prey (Schoeppner and Relyea, 2005; Turner, 2008; Scherer et al., 2016). The ubiquity of chemical signaling processes in aquatic environments has engendered a great deal of effort investigating the consequences of climate change to predators and prey (Table 1, Figure 2).
Chemical cue production can change due to shifts in metabolic rates from increased temperatures that cause predators to consume more prey. This may be especially important in dose-dependent responses to chemical metabolites used in predator-prey interactions (e.g., Weissburg and Beauvais, 2015). Increased carbon dioxide also can directly reduce production of chemical cues, as shown in alarm signaling in aphids discussed previously (Boullis et al., 2017). Interestingly, elevated CO2 changes the chemical composition of aphid honeydew, a metabolic byproduct containing kairomones used by aphid predators to detect prey. The qualitative changes in composition were insufficient to impact predator search behavior in this study, suggesting that this interaction is robust to climate change (Boullis et al., 2018). That cue quality may be sufficiently conserved so that behavior is not impacted may be due to the cue being composed of primary metabolites that are the product of fundamental metabolic processes. These cues are important in other cases, such as mud crab prey detecting primary metabolites of blue crab predators (Poulin et al., 2018).
Chemical cues have been suggested to be modified directly in future environments, although the specific mechanisms have been defined poorly or not at all. Potential mechanisms of altered chemical cues include protonation of peptides from ocean acidification, which appears to alter peptide signaling molecules used in other contexts (Roggatz et al., 2016; Lecchini et al., 2017). Warming also may denature proteins or make them more subject to decomposition. Although these examples do not evaluate predator-prey cues specifically they illustrate the general possibility of acidified environments changing the composition of conspecific chemical cues, which has been suggested but not tested for chemical alarm cues in Atlantic salmon (Leduc et al., 2010), fathead minnows and finescale dace (Brown et al., 2002), and European sea bass (Porteus et al., 2018).
The mechanisms by which climate change may affect chemical cues indicate that proteins and peptides would likely be most affected by climate change; this implies broad impacts on chemically-mediated interactions, especially in marine systems. Proteins and associated amino acids are arguably the most commonly used compounds in predator-prey interactions (Rittschof, 1990; Carr et al., 1996), for they are often released as water-soluble metabolites that rapidly spread and degrade. These chemical classes are important in attracting predators to prey (Hay, 2009) and also may be important constituents of risk cues released by predators (Poulin et al., 2018). However, other molecules, such as alarm substance released by prey, may be more specialized (i.e., secondary metabolites) and less prone to environmental degradation (Chivers and Smith, 1998; Ferrari et al., 2010). Unfortunately, there are only a handful of studies that have identified water born cues used by prey to detect predators (Poulin et al., 2018).
Climate change may have indirect consequences to predator and prey information transfer by modifying biotic factors affecting perception. Lönnstedt et al. (2013a) tested antipredator responses of tropical marine damselfish (Pomacentrus amboinensis) to chemical cues indicative of predation risk, in degraded (i.e., bleached or dead) vs. healthy coral communities. By crossing healthy and degraded habitat types with exposure and testing, this study found that fish did not respond (i.e., no changes in feeding rate, swimming, and shelter use) to conspecific damaged-released chemical cues when in dead coral habitats. Chemical cues in degraded habitats can be masked by other compounds present in the environment, especially if the habitat has drastically changed (e.g., coral overgrown by algae). Algae and cyanobacteria that become dominant on degraded reefs produce chemicals that mask anti-predator cues released by conspecific prey (McCormick et al., 2017). In Lönnstedt et al. (2013a) study, reversibility (i.e., masking) of the chemical alarm cue was not supported; this suggests that degraded habitats can directly alter chemical structure of alarm cue and thus reduce antipredator responses, although the chemical compounds present in degraded vs. healthy habitats were not explicitly examined. As chemical alarm cues are naturally broken down throughout the day (Ferner et al., 2005; Ferrari et al., 2007; Bytheway et al., 2013; Chivers et al., 2013), warming may accelerate these processes in all ecosystems and reduce the effectiveness of chemical alarm cues as reliable indicators of predation risk. In contrast, olfactory preference of dottyback predators toward damaged-released damselfish prey cues is maintained in both healthy and degraded coral environments (Natt et al., 2017). This suggests that some predators may have a sensory advantage over prey in degraded coral habitats, potentially altering the outcome of predator-prey interactions.
Much climate change behavioral research has focused on cue detection and processing in the sensory transduction pathway. Effects of ocean acidification effects on chemosensation in aquatic systems have been especially well-examined (Table 1, Figure 2). Chemical reception (i.e., detection and processing) relies on membrane bound receptors and combinatorial responses to diverse and novel combinations of chemicals (Bargmann, 2006; Symonds and Elgar, 2008). Changes to the structure of the membrane-bound chemosensory receptors could conceivably alter detection of chemical cues. Changes in central nervous system processing that underlies recognition also can affect the ability of predators or prey to respond to each other (Nilsson et al., 2012).
Elevated CO2 effects on chemosensory reception mostly examine only behavioral endpoints and do not directly reveal the underlying mechanism. Some studies show changes in detection and response to chemical food cues as a result of elevated CO2. This includes reduced attraction to prey chemical cues, as shown in brown dottyback predators (Cripps et al., 2011). Some shark predators in high CO2 conditions have been shown to fail to track, or even avoid, prey odors (Dixson et al., 2015; Pistevos et al., 2015), suggesting both cue detection and processing are being affected. Hermit crabs in high CO2 conditions take longer to locate chemical food cues and spend less time in contact with cue, suggesting deficits in cue detection as there were no effects of cue pH on these behaviors (de la Haye et al., 2012); however, small changes in general motility also occurred from reduced pH and cannot be excluded completely from this analysis. Importantly, all of these studies ruled out changes to cue structure by testing cues in both elevated and ambient CO2 conditions. Studies show both reductions in activity levels related to chemosensory-mediated foraging (e.g., handling time, time to respond) in crab predators (Dodd et al., 2015; Glaspie et al., 2017) and increases in activity of predatory reef fish (Cripps et al., 2011). These imply that elevated CO2 levels may cause some underlying physiological changes in addition to behavioral changes, but there currently is not enough evidence to support this hypothesis strongly within the scope of sensory ecology.
Prey chemosensory abilities also are affected. Manríquez et al. (2014) tested the effects of ocean acidification on chemical detection of predators and food by a muricid snail using y-maze experiments. Orientation of juvenile snails to food cues in elevated CO2 conditions was not suppressed by the presence of predator odor; orientation behavior in attractive + aversive cues was similar to that in response to only attractive cues. The inability to detect aversive cues or discriminate them from attractive cues could increase predation risk. Aversive predator cues often inhibit or eliminate attraction to food in untreated animals (Moir and Weissburg, 2009; Weissburg et al., 2012). These studies, like most others, do not suggest a mechanism. It could be that detection of specific predator cues were affected whereas food cues were not, as they are typically composed of a number of different molecules (Hay, 2009). Elevated CO2 has been demonstrated to reduce olfactory sensitivity in juvenile European sea bass (Dicentrarchus labrax), possibly by changing the affinity the receptors have for odorants (Porteus et al., 2018), although this could involve either changes to the receptor or changes to the chemical itself (e.g., Roggatz et al., 2016). Alternately, as discussed below, elevated CO2 levels can interfere with central nervous system processes that allow animals to discriminate attractive from aversive cues.
Some studies show sharp changes in behavioral responses to chemical risk that arise clearly from deficits in sensory processing by the central nervous system. For example, tropical larval clownfish (A. percula) are attracted to predator chemical cues rather than avoiding them and do not distinguish predator from non-predator cues, which translates to higher mortality rates on natural marine reefs (Dixson et al., 2010; Munday et al., 2010). These studies rule out changes in cue production or changes during transmission; predators were held in ambient conditions and prey tested in normal seawater, thus implicating changes in the receiver (prey). High CO2 conditions do not change olfactory receptor morphology (Munday et al., 2009), suggesting some central neural process is responsible. Predator cue avoidance can be restored by using the GABA-A antagonist gabazine to rescue neurotransmitter function in animals exposed to elevated CO2 conditions (Nilsson et al., 2012; but see Abboud et al., 2019). Lack of ability to identify, distinguish, and avoid chemical cues indicative of predation risk also has been shown in damselfish (Munday et al., 2010; Chivers et al., 2014), snails (Manríquez et al., 2014; Abboud et al., 2019), trout (Munday et al., 2013), and salmon (Ou et al., 2015). Future atmospheric conditions have also been shown to mask carbon dioxide cues used by mosquitos to find their hosts (Majeed et al., 2014), with elevated CO2 background levels causing a reduction in olfactory receptor sensitivity and potentially habituation in higher-order neurons (i.e., cue processing). Disruptions of processing in the olfactory bulb receptor pathway also has been demonstrated in ocean-phase coho salmon that show reduced avoidance of chemical alarm cues as a result of elevated CO2 (Williams et al., 2018).
However, other studies have shown no changes in chemosensory reception of predators and prey in response to elevated CO2. Goldsinny wrasse predators (Ctenolabrus rupestris) acclimated and tested in ambient or elevated CO2 conditions maintain olfactory-mediated behaviors to food cues (Sundin and Jutfelt, 2016). In a similar fashion, Atlantic cod (Gadus morhua) avoid predator chemical cues regardless of CO2 level that they were acclimated and tested in Jutfelt and Hedgärde (2013). Likewise, damselfish (Acanthochromis polyacanthus) continue to respond to predator chemical cues by reducing activity in both ambient and elevated CO2 treatments, especially when exposure to high CO2 is longer-term and the predator is also exposed to high CO2 conditions (Sundin et al., 2017). Interestingly, increased foraging behavior of speckled sanddab in response to damaged-released chemical cues from conspecifics is maintained in elevated CO2 (Andrade et al., 2018). Other temperate fish from naturally high CO2 environments (i.e., CO2 seeps) also do not show disruption of GABA-mediated olfactory predator recognition (Cattano et al., 2017). These studies suggest that variation in behavioral responses to elevated CO2 may arise from predisposed adaptations to local habitat conditions, allowing for chemically-mediated behaviors in some species to remain robust in a variable environment. This is supported by Jarrold et al. (2017), which suggests that fluctuating elevated CO2 environments that reflect diel CO2 cycles can reduce the negative effects of stable elevated CO2 on antipredator behavior (e.g., lateralization and predator cue avoidance) (but see Jellison et al., 2016). Given that these studies rule out effects on cue production, transmission or detection/processing, the robustness of chemical information transfer must reflect properties of the receiver.
Warming has only been directly tested on isolated chemosensory reception in the Antarctic sea star Odontaster validus (Kidawa et al., 2010); in addition to reducing ability for sea stars to right after being flipped and reducing locomotor activity, this study reported that warming weakened attraction to food odor cues. This suggests that warming can indirectly reduce chemosensory detection and processing by reducing overall activity.
Vision is important for detecting predation risk as well as potential prey, especially in well-lit systems such as coral reefs with predators that possess good visual systems. The success of visual cue information transfer is highly dependent on degree of movement, light availability and contrast against an environmental background to transmit the cue, as well as physiological eye function (Table 2). Consequently, visual information transfer is highly variable across spatial (i.e., within one meter or over tens of meters) and temporal (i.e., seconds or minutes) scales (Weissburg et al., 2014). All of these factors have been shown to be affected by climate change stressors, although there are limited studies (Table 1; Figure 2). Increased predator movement from warming has been shown in European sea bass (D. labrax) with higher levels of swimming and other activity (Manciocco et al., 2015), which may indicate higher predation risk to potential prey. In a similar fashion, increased prey metabolism from warming can increase production of prey visual cues via increased activity levels, causing prey to become more susceptible to predation. This has been suggested with increased activity levels (and consequently encounters) between spider predators and fly prey from increased temperatures (Kruse et al., 2008), but the sensory ecology of these interactions remains to be tested. Elevated CO2 can increase activity levels in juvenile coral trout (Plectropomus leopardus), and produces bolder behavior and less time spent in shelter (Munday et al., 2013). Pygmy squid also are more active and use less cryptic behaviors in high CO2 conditions (Spady et al., 2014). These changes increase production of visual cues by prey and potentially increase predation risk.
Warming is predicted to have a strong impact on transmission of visual cues. Coker et al. (2009) demonstrated that piscivorous dottybacks (Pseudochromis fuscus) initially avoid bleached corals as a degraded habitat but increase strike rates at prey positioned against the white background of bleached corals. This indicates a mismatch between camouflaged prey and background, which can lead to increased predation as a consequence of increased temperature. Degraded habitats have also been shown to maintain transmission of visual predation risk via reduced habitat complexity (McCormick and Lönnstedt, 2013), strengthening the visual transduction pathway between predator and prey.
Warming also is predicted to have indirect effects on visually mediated predator-prey dynamics. Climate change conditions favor algal blooms (Paerl and Huisman, 2009) that can increase turbidity and reduce light availability. Turbidity has been shown to directly interfere with visually-mediated foraging success of pinfish predators on crustacean prey (Lunt and Smee, 2015) by reducing reactive distance (Sweka and Hartman, 2003), although other fish predators with well-developed visual systems (e.g., Atlantic cod) may not experience as dramatic effects (Meager et al., 2005). Turbidity also drastically reduces the distance at which cormorant (Phalacrocorax carbo sinensis) predators can visually detect fish prey (Strod et al., 2008). Increased turbidity also has been demonstrated to decrease transmission of visual cues that are imperative for avoiding predators (Swanbrow Becker and Gabor, 2012). This has been tested in freshwater systems, where perception of predation risk is reduced in turbid waters (Abrahams and Kattenfeld, 1997; Hartman and Abrahams, 2000). Together, these studies suggest that turbidity can change trophic interactions by reducing indirect effects of predators (i.e., predation risk assessment) and shifting top-down control to only direct (i.e., consumptive) effects as encounters become random (Van de Meutter et al., 2005).
Elevated CO2 effects on visual detection and processing have been tested directly in a few cases. Larval temperate gobies (Gobiusculus flavescens) in elevated CO2 conditions are more attracted to light (i.e., increased phototaxis), which suggests that visual hypersensitivity may be costly by attracting to weak or fluctuating light stimuli (Forsgren et al., 2013); the specific fitness effects of this behavioral change remain unknown, but may include attraction to new habitats with higher or lower predation rates. Retinal responses of a marine tropical damselfish species (A. polyacanthus) are slowed after exposure to high CO2 waters, which suggests impaired visual assessment of fast events such as predator or prey movement via disruption of cue detection (Chung et al., 2014). Antipredator responses (e.g., reductions in foraging and activity, bobbing behavior) of another juvenile tropical damselfish (P. amboinensis) to the sight of a predator are reduced or lost (i.e., no antipredator response) in high CO2 conditions, suggesting that elevated CO2 can reduce visual risk assessment and promote bolder prey behavior via disruption of cue processing (Ferrari et al., 2012). Prey reactivity (i.e., reactive distance, looming threshold) to visual predation risk also has been shown to be reduced by warming and elevated CO2, but the outcome of predation depends on stressor effects on the predator as well as prey (Allan et al., 2013, 2017).
Mechanosensation, such as by lateral lines in fishes and mechanoreceptors in arthropods, is used to detect changes in pressure or fluid motion around the organism (Dusenberry, 1992). Detecting nearby (i.e., within 1 m–Weissburg et al., 2014) disturbances such as movement can be useful in foraging or avoiding predators and other mortality risks, but there are very limited studies on effects of climate change on mechanosensory information transfer (Table 1; Figure 2).
As noted previously, changes in movement via changes in metabolism from warming may cause mechanosensory cues to be altered in both terrestrial and aquatic systems. Specifically, increased movement or activity would increase mechanosensory cue production, strengthening the specific location of predator or prey. Directionality and escape responses of damselfish to a startle stimulus increase after short-term exposure to elevated temperatures (Warren et al., 2017). However, longer-term exposure to warming allows escape responses to return to control levels, demonstrating that physiological plasticity is dependent on length of exposure. Damselfish acclimated to elevated CO2 conditions are less responsive and they take longer to respond to a stimulus (i.e., a weight dropped on the surface of the water) while locomotor activities are maintained, suggesting a deficit in processing these mechanosensory cues (Munday et al., 2016). Likewise, the probability of a startle response from marine medaka to a mechanosensory stimulus is decreased in elevated CO2 conditions due a deficit in processing (Wang et al., 2017).
Although studying responses to individual cues can help explain mechanisms or drivers of behavior, it is important to note that multiple senses often are utilized by organisms in natural ecosystems, especially when one sensory modality is compromised by an environmental stressor. Therefore, studies incorporating multisensory responses to warming and elevated CO2 provide a more realistic prediction of changes in overall behavior. These studies are limited, however, and mostly explore the interaction and possible “sensory redundancy” (Lönnstedt et al., 2013b) between chemical and visual cues (Table 1). Manciocco et al. (2015) studied sea bass from temperate estuaries in warmed conditions and found that attraction to food chemical cues and avoidance of visual aversive cue (i.e., mirror test) both increased in intensity and occurred at faster rates (i.e., shorter behavioral response time to cues). This emphasizes how warming changes the speed of sensory information transfer in multiple modalities and consequent behavioral responses. Elevated CO2 also has been shown to affect visual and chemical cues in damselfish found in tropical reefs (Lönnstedt et al., 2013b): while elevated CO2 caused fish to fail to identify (i.e., no response) chemical alarm cues completely, antipredator responses to visual predator cues were reduced but not completely lost. When visual and chemical cues were combined, responses to visual cues were able to partially (but not fully) compensate for lack of responses to chemical cues. Goldenberg et al. (2018) used mesocosm experiments with multiple species of fish and crustacean consumers found in temperate marine systems, testing effects of warming and elevated CO2 on the efficacy of visual and chemical cues in isolation and together. Elevated CO2 but not warming reduced attraction to food chemical and visual cues, but the consumers restored their attraction to food when chemical and visual cues were combined regardless of temperature or CO2 level. These studies suggest that sensory redundancy can mitigate the effects of climate change on individual sensory modalities, and some ecological interactions and processes may remain relatively resilient to these stressors. Consequently, certain community dynamics may be relatively robust to climate change when multiple sensory cues are employed.
However, multimodal cues may not be as reliable in degraded habitats as in normal conditions because degraded habitats may create environments where one cue is strongly favored or disfavored. As previously mentioned, McCormick et al. explored the effects of habitat degradation on predation risk assessment by marine damselfish P. amboinensis using visual and chemical cues. Visual predation risk cues become more important in degraded habitats with less structure as prey are more easily able to detect predators (Lönnstedt et al., 2013a); chemical predation risk cues become more important in topographically complex (i.e., healthy reef) habitats when visual risk cues are restricted, and prey are more vigilant (i.e., reduced feeding and activity, increased shelter use) (McCormick and Lönnstedt, 2013). Habitat degradation may increase the availability of visual cues, but this may come at a cost of degraded chemical cues (Lönnstedt et al., 2013a). Damselfish are less likely to use degraded coral (i.e., loss of live coral tissue) as shelter in response to predation risk, potentially due to altered visual or chemical cues (Boström-Einarsson et al., 2018). Together, these findings suggest that fishes in degraded habitats may experience higher mortality rates from predators if habitat changes alter the availability of one type of cue relative to one another, especially when chemical cues are important sources of information regarding predation risk.
Sensory behaviors stemming from different modalities may share universal disruptions in central nervous system processing, exemplified in changes to behavioral lateralization of marine fish challenged with high CO2 conditions (Domenici et al., 2012; Jutfelt et al., 2013; Welch et al., 2014; Näslund et al., 2015). These studies have attempted to show how elevated CO2 disrupts neural processing by assuming lateralization requires minimal visual input from the environment and is driven by brain functional asymmetries that create a right- or left-side preference. In these studies using T-mazes to measure turning preference in damselfish, elevated CO2 has been shown to reduce lateralization (i.e., no turning preference). Nilsson et al. (2012) further demonstrated that the addition of an antagonist of the GABA-A receptor (i.e., gabazine) reversed elevated CO2 effects in both lateralization and chemical cue preference, suggesting high CO2 affects neural processing of multiple sensory systems by interfering with neurotransmitter function. This is supported by Heuer et al. (2016), which demonstrates that elevated CO2 causes altered ion gradients in the GABA-A receptor of fish as a means of CO2 compensation. Impaired neurotransmitter function from elevated CO2 also has been demonstrated to decrease learning of predator identity in juvenile damselfish prey, consequently reducing survivorship in the field after being treated in the laboratory (Chivers et al., 2014). The availability of multiple sensory cues may be insufficient to allow predators or prey to regain normal sensory function in the presence of such fundamental disruptions of neural processing.
Global warming and elevated CO2 will have extensive impacts on sensory behavior in predator-prey interactions. However, lack of a framework for identifying the underlying mechanisms makes it difficult to establish the generality of effects or their magnitude. Using a behavioral endpoint to examine stressor effects on predation (e.g., Li et al., 2015; Sui et al., 2015) often fails to identify whether effects stem from cue production, transmission, or reception (Figure 1) unless the study is properly constructed. Studies should examine stressor effects on predator and prey separately as well as together, as well as isolating the effect of differential transmission from changes to sender and receiver properties.
Understanding which process is affected is essential to fully understand subsequent effects as changes to particular processes have different consequences. For instance, transmission may alter the effective distance of predator-prey signaling, whereas deficits in reception by predators or prey have global effects. Since warming and elevated CO2 seem to affect different steps in the transduction cascade (Figure 1), clearly identifying the nature of the disruption also can predict synergistic or antagonistic responses. In particular, warming seems to affect metabolism and activity (in addition to transmission in some cases), whereas elevated CO2 often impacts the ability to receive or process cues. The effects of warming on metabolism, and in turn, the impacts on foraging ability, suggests warming may primarily alter consumptive effects of predators despite some changes to the signaling process. Elevated CO2 clearly affects all different steps in the predator-prey signaling cascade, and may therefore change both consumptive and non-consumptive effects depending on which organism is most compromised in a given interaction. If sensing by predators is more affected then non-consumptive effects may increase, whereas consumptive effects will increase when sensing by prey is strongly affected.
Future studies should address both behavioral endpoints and identify in so far as possible the step (production, transmission, or reception) that is affected. Although this can be cumbersome, a substitutive design where acclimated animals are placed in normal environments and vice versa, can at least identify environmental effects on transmission. Independent measures of cue strength also can be helpful, particularly when movement related cues are responsible for predator-prey information transfer and stressors are shown to affect activity. Chemical cues that are metabolic by-products may be difficult to quantify, but metabolomics approaches offer promise (e.g., Poulin et al., 2018) and can at least establish quantitative differences in production even when the cue remains unidentified. Deficits in neural processing can be difficult to document without examining physiology of sensory receptor cells or central nervous system properties, which has been done only in some cases (e.g., Munday et al., 2009; Boullis et al., 2017; Porteus et al., 2018). Careful consideration is also needed when determining measurements of sensory responses that distinguish these from changes in general motility or activity (e.g., lacking in Kidawa et al., 2010). General observations on movement in response to a stimulus can be confounded by changes in metabolism and/or motor function, potentially misidentifying sensory effects (e.g., de la Haye et al., 2012). Possible sensory tests should include at least a choice between stimulus and blank control (e.g., Dixson et al., 2010).
A second major challenge going forward is to integrate both predator and prey responses into studies examining the effect of climate change stressors. Predators and prey participate in a duet where both parties can gather information about the presence of the other, and the effects of climate change on predator-prey dynamics will depend strongly on which participant is more compromised. For example, ocean acidification has a greater negative effect on mud crab foraging behaviors (i.e., consumption rate, handling time, duration of unsuccessful predation attempts) than calcification rates of oyster prey, resulting in overall reduced prey consumption (Dodd et al., 2015). Moreover, the extent to which predators vs. prey are affected may change the nature of predator control as well as its magnitude (e.g., Goldenberg et al., 2018). We predict a shift to consumptive effects if climate change stressors more strongly influence prey, whereas the importance of NCEs will increase if predator abilities are more impacted.
Knowledge gaps remain due to biases in the literature. Approximately 56% of climate change studies focused on sensory cues governing predator-prey interactions use marine reef fish as model organisms, especially for elevated CO2 effects (Figure 2). Invertebrate predators have also been shown to be important community regulators such as blue crabs in estuarine habitats (Silliman and Bertness, 2002; O'Connor et al., 2008; Hill and Weissburg, 2013), which often rely on chemical cues to locate prey. Invertebrates as model organisms should be tested more frequently in future studies given their ecological importance.
Studies of sensory modalities affected by climate change have emphasized chemosensation over others (e.g., vision, audition, mechanosensation), with ~61% of climate change sensory studies using chemosensation as the primary modality (Figure 2; Table 3). Cue detection/processing deficits have been shown in chemosensory, visual, and possibly mechanosensory interactions, but few studies to date have tested auditory processing. Some animals utilize specialized senses, such as electrosensation in sharks and other elasmobranchs. Currently, there are no known studies that examine how responses to these cues might change in the face of climate change. Changes in neurotransmitter function induced by climate change (Nilsson et al., 2012) suggest that processing deficits may be occurring in multiple sensory systems.
Environmental effects are important in changing sound propagation and altering the visual and chemical environment so as to mask incoming cues from predators and prey, or enhance the utility of one modality vs. another. These effects also might be important in terrestrial systems despite the lack of studies (Figure 2; Table 3). Vegetation affects both spectral and acoustic properties and can alter both cue transmission and ambient sound and light levels, and change airflow patterns affecting mechanosensory signaling (Dusenberry, 1992; Endler, 1993; Ladich, 2000; Slabbekoorn and Smith, 2002; Brumm and Slabbekoorn, 2005; Casas and Dangles, 2010). Changes to vegetative structure produced by changing climate (e.g. Cramer et al., 2001) therefore may alter predator-prey information exchange in terrestrial habitats. The environment may also modify cues directly as occurs with waterborne or airborne chemicals. Most studies to date that manipulate only the predator or prey do not capture these effects, and so further studies should attempt to incorporate the sensory environment (Figure 1) to correctly capture future interactions. It also is critical to keep in mind that these potential changes in the environment are independent of changes to the receiver or sender, but may interact with these changes in both opposing or complementary fashion.
This field has been disproportionally represented by elevated CO2 studies rather than warming, promoting a consequent bias in marine systems and very few terrestrial examples (Figure 2; Table 3). Temperature is the primary global stressor affecting sensory-mediated interactions in terrestrial systems, and so the current state of knowledge implies that these interactions may be less severely affected. However, warming needs to be studied in animals whose metabolic processes and consequent behavior is dependent on temperature, such as invertebrates. Metabolic changes from temperature can also change cue production and processing speed, and these effects should be more explicitly tested.
It is important to note that while warming and elevated CO2 can have independent effects on sensory systems, there is potential for these global stressors to interact as both are predicted to occur in the future (IPCC, 2014). There are several studies examining multiple stressors on behavioral interactions that have potential sensory mechanisms, which were not explicitly tested. For example, warming and elevated CO2 act synergistically to increase overall predation rate of dottyback predators (P. fuscus) on multiple species of damselfish prey (P. amboinensis and P. nagasakiensis), whereas each of these stressors independently have no effect on predation rate (Ferrari et al., 2015). This effect could not be predicted from general changes in metabolism alone, which is argued to demonstrate that trophic outcomes are not driven solely by physiological tolerances to these climate change stressors. Also shown in this study were antagonistic interactions between warming and elevated CO2; each climate stressor independently reversed prey selectivity between the two damselfish prey species, but the combined stressors override prey selectivity so that prey are consumed in equal proportions. In this case, specific sensory cues used by predator and prey were not tested, but further research may provide insight on which sensory modalities are driving these interactive effects. It is possible that warming can interact with elevated CO2 by changing metabolic rates that affect cue production and reception, potentially amplifying any direct effects on the sensory transduction pathway (Figure 1).
There are very limited studies that examine how multiple stressors affect the same transduction pathway, and the underlying mechanisms. As previously mentioned, warming and elevated CO2 effects have been tested in fish and crustaceans responding to visual and chemical cues (Goldenberg et al., 2018). Testing these stressors in isolation and together revealed that only elevated CO2 was driving behavioral responses when both stressors were present. A similar pattern was found in dogwhelks (Queirós et al., 2015) and sharks (Pistevos et al., 2015), where only elevated CO2 disrupted chemosensory perception of prey while warming had no effect on these behaviors. Identifying multiple stressor effects on a single sensory modality can allow the correct identification of specific stressor effects on different senses [e.g., chemosensation (Dixson et al., 2010), vision (Ferrari et al., 2012), and audition (Simpson et al., 2011) in coral reef fish]. Again, we suggest that warming and elevated CO2 may target different parts of the transduction cascade and may interact strongly in systems where cue production depends on activity.
Interaction of global stressors (i.e., warming and elevated CO2) with local stressors also needs to be studied, as local stressors (e.g., sediment pollution: O'Connor et al., 2016) have been found to disrupt sensory cues and change behavioral responses. One study tested the global stressor of elevated CO2 and local stressors of sediment runoff and pesticides separately, but did not cross the stressors (Lecchini et al., 2017); each stressor had negative effects on attraction to conspecific chemical cues for both fish and crustacean species, but the possibility of interactive effects (synergism or antagonism) between these stressors remains unexplored.
The continuation of these efforts, especially more realistic laboratory experiments and field experiments when possible, has the potential to identify and address the complex changes in future predator-prey interactions. This expansion will yield a better understanding of climate change impacts on sensory ecology, which can be applied to the success of conservation and restoration efforts for protecting and maintaining ecosystems.
AD conceived the manuscript, led the literature review, and produced the figures. MW contributed to the literature search and core concepts for this paper, and both authors contributed to writing and revising the final manuscript.
This work was funded by NSF grant Bio-OCE #1234449 awarded to MW.
The authors declare that the research was conducted in the absence of any commercial or financial relationships that could be construed as a potential conflict of interest.
We would like to thank Dr. Mark Hay, Dr. Julia Kubanek, and Dr. Dave Hudson for providing feedback on earlier versions of the manuscript. We would also like to thank Dr. Evan Preisser and two reviewers for important comments and valuable guidance that improved the manuscript.
Abboud, J.-C., Bartolome, E. A., Blanco, M., Kress, A. C., Ellis, I. Y., Yazzolino, P. K., et al. (2019). Carbon dioxide enrichment alters predator avoidance and sex determination but only sex is mediated by GABAA receptors. Hydrobiologia 829, 307–322. doi: 10.1007/s10750-018-3841-3
Abrahams, M. V., and Kattenfeld, M. G. (1997). The role of turbidity as a constraint on predator-prey interactions in aquatic environments. Behav. Ecol. Sociobiol. 40, 169–174. doi: 10.1007/s002650050330
Allan, B. J., Domenici, P., McCormick, M. I., Watson, S.-A., and Munday, P. L. (2013). Elevated CO2 affects predator-prey interactions through altered performance. PLoS ONE. 8:e58520. doi: 10.1371/journal.pone.0058520
Allan, B. J. M., Domenici, P., Watson, S. A., Munday, P. L., and McCormick, M. I. (2017). Warming has a greater effect than elevated CO2 on predator–prey interactions in coral reef fish. Proc. R. Soc. B 284:20170784. doi: 10.1098/rspb.2017.0784
Andrade, J. F., Hurst, T. P., and Miller, J. A. (2018). Behavioral responses of a coastal flatfish to predation-associated cues and elevated CO2. J. Sea Res. 140, 11–21. doi: 10.1016/j.seares.2018.06.013
Arlettaz, R., Jones, G., and Racey, P. A. (2001). Effect of acoustic clutter on prey detection by bats. Nature 414, 742–745. doi: 10.1038/414742a
Bargmann, C. I. (2006). Comparative chemosensation from receptors to ecology. Nature 444, 295–301. doi: 10.1038/nature05402
Barry, J. P., Lovera, C., Buck, K. R., Peltzer, E. T., Taylor, J. R., Walz, P., et al. (2014). Use of a free ocean CO2 enrichment (FOCE) system to evaluate the effects of ocean acidification on the foraging behavior of a deep-Sea Urchin. Environ. Sci. Technol. 48, 9890–9897. doi: 10.1021/es501603r
Beever, E. A., Hall, L. E., Varner, J., Loosen, A. E., Dunham, J. B., Gahl, M. K., et al. (2017). Behavioral flexibility as a mechanism for coping with climate change. Front. Ecol. Environ. 15:1502. doi: 10.1002/fee.1502
Bignami, S., Enochs, I. C., Manzello, D. P., Sponaugle, S., and Cowen, R. K. (2013). Ocean acidification alters the otoliths of a pantropical fish species with implications for sensory function. Proc. Natl. Acad. Sci. U.S.A. 110, 7366–7370. doi: 10.1073/pnas.1301365110
Boström-Einarsson, L., Bonin, M. C., Munday, P. L., and Jones, G. P. (2018). Loss of live coral compromises predator-avoidance behaviour in coral reef damselfish. Sci. Rep. 8:7795. doi: 10.1038/s41598-018-26090-4
Boullis, A., Blanchard, S., Francis, F., and Verheggen, F. (2018). Elevated CO2 concentrations impact the semiochemistry of aphid honeydew without having a cascade effect on an aphid predator. Insects 9:47. doi: 10.3390/insects9020047
Boullis, A., Fassotte, B., Sarles, L., Lognay, G., Heuskin, S., Vanderplanck, M., et al. (2017). Elevated carbon dioxide concentration reduces alarm signaling in aphids. J. Chem. Ecol. 43, 164–171. doi: 10.1007/s10886-017-0818-z
Briffa, M., de la Haye, K., and Munday, P. L. (2012). High CO2 and marine animal behaviour: potential mechanisms and ecological consequences. Mar. Pollut. Bull. 64, 1519–1528. doi: 10.1016/j.marpolbul.2012.05.032
Brown, G. E., Adrian, Jr. J. C., Lewis, M. G., and Tower, J. M. (2002). The effects of reduced pH on chemical alarm signalling in ostariophysan fishes. Can. J. Fish. Aquat. Sci. 59, 1331–1338. doi: 10.1139/f02-104
Brumm, H., and Slabbekoorn, H. (2005). “Acoustic communication in noise,” in Advances in the Study of Behavior, eds P. J. B. Slater, C. T. Snowdon, H. J. Brockmann, T. J. Roper, and M. Naguib (Waltham, MA: Academic Press), 151–209. doi: 10.1016/S0065-3454(05)35004-2
Bytheway, J. P., Carthey, A. J. R., and Banks, P. B. (2013). Risk vs. reward: how predators and prey respond to aging olfactory cues. Behav. Ecol. Sociobiol. 67, 715–725. doi: 10.1007/s00265-013-1494-9
Carr, W. E. S., Netherton, I. I. I., J. C., Gleeson, R. A., and Derby, C. D. (1996). Stimulants of feeding behavior in fish: analyses of tissues of diverse marine organisms. Biol. Bull. 190, 149–160. doi: 10.2307/1542535
Casas, J., and Dangles, O. (2010). Physical ecology of fluid flow sensing in arthropods. Annu. Rev. Entomol. 55, 505–520. doi: 10.1146/annurev-ento-112408-085342
Cattano, C., Cal,ò, A., Di Franco, A., Firmamento, R., Quattrocchi, F., Sdiri, K., et al. (2017). Ocean acidification does not impair predator recognition but increases juvenile growth in a temperate wrasse off CO2 seeps. Mar. Environ. Res. 132, 33–40. doi: 10.1016/j.marenvres.2017.10.013
Charpentier, C. L., and Cohen, J. H. (2016). Acidification and γ -aminobutyric acid independently alter kairomone-induced behaviour. R. Soc. Open Sci. 3:160311. doi: 10.1098/rsos.160311
Chivers, D. P., Dixson, D. L., White, J. R., McCormick, M. I., and Ferrari, M. C. O. (2013). Degradation of chemical alarm cues and assessment of risk throughout the day. Ecol. Evol. 3, 3925–3934. doi: 10.1002/ece3.760
Chivers, D. P., McCormick, M. I., Nilsson, G. E., Munday, P. L., Watson, S.-A., Meekan, M. G., et al. (2014). Impaired learning of predators and lower prey survival under elevated CO2: a consequence of neurotransmitter interference. Glob. Change Biol. 20, 515–522. doi: 10.1111/gcb.12291
Chivers, D. P., and Smith, R. J. F. (1998). Chemical alarm signalling in aquatic predator-prey systems: a review and prospectus. Écoscience 5, 338–352. doi: 10.1080/11956860.1998.11682471
Chung, W. S., Marshall, N. J., Watson, S.-A., Munday, P. L., and Nilsson, G. E. (2014). Ocean acidification slows retinal function in a damselfish through interference with GABAA receptors. J. Exp. Biol. 217, 323–326. doi: 10.1242/jeb.092478
Coker, D. J., Pratchett, M. S., and Munday, P. L. (2009). Coral bleaching and habitat degradation increase susceptibility to predation for coral-dwelling fishes. Behav. Ecol. 20, 1204–1210. doi: 10.1093/beheco/arp113
Cramer, W., Bondeau, A., Woodward, F. I., Prentice, I. C., Betts, R. A., Brovkin, V., et al. (2001). Global response of terrestrial ecosystem structure and function to CO 2 and climate change: results from six dynamic global vegetation models. Glob. Change Biol. 7, 357–373. doi: 10.1046/j.1365-2486.2001.00383.x
Cripps, I. L., Munday, P. L., and McCormick, M. I. (2011). Ocean acidification affects prey detection by a predatory reef fish. PLoS ONE 6:e22736. doi: 10.1371/journal.pone.0022736
de la Haye, K. L., Spicer, J. I., Widdicombe, S., and Briffa, M. (2012). Reduced pH sea water disrupts chemo-responsive behaviour in an intertidal crustacean. J. Exp. Mar. Biol. Ecol. 412, 134–140. doi: 10.1016/j.jembe.2011.11.013
Dixson, D. L., Jennings, A. R., Atema, J., and Munday, P. L. (2015). Odor tracking in sharks is reduced under future ocean acidification conditions. Glob. Change Biol. 21, 1454–1462. doi: 10.1111/gcb.12678
Dixson, D. L., Munday, P. L., and Jones, G. P. (2010). Ocean acidification disrupts the innate ability of fish to detect predator olfactory cues. Ecol. Lett. 13, 68–75. doi: 10.1111/j.1461-0248.2009.01400.x
Dodd, L. F., Grabowski, J. H., Piehler, M. F., Westfield, I., and Ries, J. B. (2015). Ocean acidification impairs crab foraging behaviour. Proc. Biol. Sci. 282:2015.0333. doi: 10.1098/rspb.2015.0333
Domenici, P., Allan, B., McCormick, M. I., and Munday, P. L. (2012). Elevated carbon dioxide affects behavioural lateralization in a coral reef fish. Biol. Lett. 8, 78–81. doi: 10.1098/rsbl.2011.0591
Doney, S. C., Fabry, V. J., Feely, R. A., and Kleypas, J. A. (2009). Ocean acidification: the other CO2 problem. Annu. Rev. Mar. Sci. 1, 169–192. doi: 10.1146/annurev.marine.010908.163834
Dusenberry, D. B. (1992). Sensory Ecology: How Organisms Acquire and Respond to Information. New York, NY: W.H. Freeman.
Endler, J. A. (1993). The color of light in forests and its implications. Ecol. Monogr. 63, 1–27. doi: 10.2307/2937121
Ferner, M. C., Smee, D. L., and Chang, Y. P. (2005). Cannibalistic crabs respond to the scent of injured conspecifics: danger or dinner? Mar. Ecol. Prog. Ser. 300, 193–200. doi: 10.3354/meps300193
Ferrari, M. C., Munday, P. L., Rummer, J. L., McCormick, M. I., Corkill, K., Watson, S.-A., et al. (2015). Interactive effects of ocean acidification and rising sea temperatures alter predation rate and predator selectivity in reef fish communities. Glob. Change Biol. 21, 1848–1855. doi: 10.1111/gcb.12818
Ferrari, M. C. O., McCormick, M. I., Munday, P. L., Meekan, M. G., Dixson, D. L., Lönnstedt, O., et al. (2012). Effects of ocean acidification on visual risk assessment in coral reef fishes. Funct. Ecol. 26, 553–558. doi: 10.1111/j.1365-2435.2011.01951.x
Ferrari, M. C. O., Messier, F., and Chivers, D. P. (2007). Degradation of chemical alarm cues under natural conditions: risk assessment by larval woodfrogs. Chemoecology 17, 263–266. doi: 10.1007/s00049-007-0381-0
Ferrari, M. C. O., Wisenden, B. D., and Chivers, D. P. (2010). Chemical ecology of predator–prey interactions in aquatic ecosystems: a review and prospectus. Can. J. Zool. 88, 698–724. doi: 10.1139/Z10-029
Forsgren, E., Dupont, S., Jutfelt, F., and Amundsen, T. (2013). Elevated CO 2 affects embryonic development and larval phototaxis in a temperate marine fish. Ecol. Evol. 3, 3637–3646. doi: 10.1002/ece3.709
Glaspie, C. N., Longmire, K., and Seitz, R. D. (2017). Acidification alters predator-prey interactions of blue crab Callinectes sapidus and soft-shell clam Mya arenaria. J. Exp. Mar. Biol. Ecol. 489, 58–65. doi: 10.1016/j.jembe.2016.11.010
Goldenberg, S. U., Nagelkerken, I., Marangon, E., Bonnet, A., Ferreira, C. M., and Connell, S. D. (2018). Ecological complexity buffers the impacts of future climate on marine consumers. Nat. Clim. Change 8, 229–233. doi: 10.1038/s41558-018-0086-0
Gordon, T. A. C., Harding, H. R., Wong, K. E., Merchant, N. D., Meekan, M. G., McCormick, M. I., et al. (2018). Habitat degradation negatively affects auditory settlement behavior of coral reef fishes. Proc. Natl. Acad. Sci. U.S.A. 115, 5193–5198. doi: 10.1073/pnas.1719291115
Hartman, E. J., and Abrahams, M. V. (2000). Sensory compensation and the detection of predators: the interaction between chemical and visual information. Proc. R. Soc. B Biol. Sci. 267, 571–575. doi: 10.1098/rspb.2000.1039
Hay, M. E. (2009). Marine chemical ecology: chemical signals and cues structure marine populations, communities, and ecosystems. Ann. Rev. Mar. Sci. 1, 193–212. doi: 10.1146/annurev.marine.010908.163708
Hester, K. C., Peltzer, E. T., Kirkwood, W. J., and Brewer, P. G. (2008). Unanticipated consequences of ocean acidification: a noisier ocean at lower pH. Geophys. Res. Lett. 35:L19601. doi: 10.1029/2008GL034913
Heuer, R. M., Welch, M. J., Rummer, J. L., Munday, P. L., and Grosell, M. (2016). Altered brain ion gradients following compensation for elevated CO2 are linked to behavioural alterations in a coral reef fish. Sci. Rep. 6:33216. doi: 10.1038/srep33216
Hill, J. M., and Weissburg, M. J. (2013). Predator biomass determines the magnitude of non-consumptive effects (NCEs) in both laboratory and field environments. Oecologia 172, 79–91. doi: 10.1007/s00442-012-2488-4
Ilyina, T., Zeebe, R. E., and Brewer, P. G. (2010). Future ocean increasingly transparent to low-frequency sound owing to carbon dioxide emissions. Nat. Geosci. 3, 18–22. doi: 10.1038/ngeo719
IPCC (2014). “Climate change 2014: synthesis report,”? in Contribution of Working Groups I, II and III to the Fifth Assessment Report of the Intergovernmental Panel on Climate Change, eds Core Writing Team, R. K. Pachauri, and L. A. Meyer (Geneva: IPCC).
Jarrold, M. D., Humphrey, C., McCormick, M. I., and Munday, P. L. (2017). Diel CO2 cycles reduce severity of behavioural abnormalities in coral reef fish under ocean acidification. Sci. Rep. 7:10153. doi: 10.1038/s41598-017-10378-y
Jarrold, M. D., and Munday, P. L. (2018). Elevated temperature does not substantially modify the interactive effects between elevated CO2 and diel CO2 cycles on the survival, growth and behavior of a coral reef fish. Front. Mar. Sci. 5:458. doi: 10.3389/fmars.2018.00458
Jellison, B. M., Ninokawa, A. T., Hill, T. M., Sanford, E., and Gaylord, B. (2016). Ocean acidification alters the response of intertidal snails to a key sea star predator. Proc. R. Soc. B Biol. Sci. 283:20160890. doi: 10.1098/rspb.2016.0890
Jiahuan, R., Wenhao, S., Xiaofan, G., Wei, S., Shanjie, Z., Maolong, H., et al. (2018). Ocean acidification impairs foraging behavior by interfering with olfactory neural signal transduction in Black Sea Bream, Acanthopagrus schlegelii. Front. Physiol. 9:1592. doi: 10.3389/fphys.2018.01592
Jutfelt, F., Bresolin de Souza, K., Vuylsteke, A., and Sturve, J. (2013). Behavioural disturbances in a temperate fish exposed to sustained high-CO2 levels. PLoS ONE. 8:e65825. doi: 10.1371/journal.pone.0065825
Jutfelt, F., and Hedgärde, M. (2013). Atlantic cod actively avoid CO2 and predator odour, even after long-term CO2 exposure. Front. Zool. 10:81. doi: 10.1186/1742-9994-10-81
Kats, L. B., and Dill, L. M. (1998). The scent of death: chemosensory assessment of predation risk by prey animals. Ecoscience 5, 361–394.
Kidawa, A., Potocka, M., and Janecki, T. (2010). The effects of temperature on the behaviour of the Antarctic sea star Odontaster validus. Pol. Polar Res. 31, 273–284. doi: 10.2478/v10183-010-0003-3
Kim, T. W., Taylor, J., Lovera, C., and Barry, J. P. (2016). CO 2 -driven decrease in pH disrupts olfactory behaviour and increases individual variation in deep-sea hermit crabs. ICES J. Mar. Sci. J. Cons. 73, 613–619. doi: 10.1093/icesjms/fsv019
Kroeker, K. J., Kordas, R. L., Crim, R., Hendriks, I. E., Ramajo, L., Singh, G. S., et al. (2013). Impacts of ocean acidification on marine organisms: quantifying sensitivities and interaction with warming. Glob. Change Biol. 19, 1884–1896. doi: 10.1111/gcb.12179
Kruse, P. D., Toft, S., and Sunderland, K. D. (2008). Temperature and prey capture: opposite relationships in two predator taxa. Ecol. Entomol. 33, 305–312. doi: 10.1111/j.1365-2311.2007.00978.x
Ladich, F. (2000). Acoustic communication and the evolution of hearing in fishes. Philos. Trans. R. Soc. B Biol. Sci. 355, 1285–1288. doi: 10.1098/rstb.2000.0685
Lecchini, D., Dixson, D. L., Lecellier, G., Roux, N., Frédérich, B., Besson, M., et al. (2017). Habitat selection by marine larvae in changing chemical environments. Mar. Pollut. Bull. 114, 210–217. doi: 10.1016/j.marpolbul.2016.08.083
Leduc, A. O. H. C., Roh, E., Macnaughton, C. J., Benz, F., Rosenfeld, J., and Brown, G. E. (2010). Ambient pH and the response to chemical alarm cues in Juvenile Atlantic Salmon: mechanisms of reduced behavioral responses. Trans. Am. Fish. Soc. 139, 117–128. doi: 10.1577/T09-024.1
Leonard, G. H., Levine, J. M., Schmidt, P. R., and Bertness, M. D. (1998). Flow-driven variation in intertidal community structure in a Maine estuary. Ecology 79, 1395–1411. doi: 10.1890/0012-9658(1998)079[[1395:FDVIIC]]2.0.CO;2
Li, L., Lu, W., Sui, Y., Wang, Y., Gul, Y., and Dupont, S. (2015). Conflicting effects of predator cue and ocean acidification on the mussel Mytilus coruscus byssus production. J. Shellf. Res. 34, 393–400. doi: 10.2983/035.034.0222
Lönnstedt, O. M., McCormick, M. I., and Chivers, D. P. (2013a). Degraded environments alter prey risk assessment. Ecol. Evol. 3, 38–47. doi: 10.1002/ece3.388
Lönnstedt, O. M., Munday, P. L., McCormick, M. I., Ferrari, M. C. O., and Chivers, D. P. (2013b). Ocean acidification and responses to predators: can sensory redundancy reduce the apparent impacts of elevated CO2 on fish? Ecol. Evol. 3, 3565–3575. doi: 10.1002/ece3.684
Lunt, J., and Smee, D. L. (2015). Turbidity interferes with foraging success of visual but not chemosensory predators. PeerJ. 3:e1212. doi: 10.7717/peerj.1212
Luo, J., Koselj, K., Zsebok, S., Siemers, B. M., and Goerlitz, H. R. (2013). Global warming alters sound transmission: differential impact on the prey detection ability of echolocating bats. J. R. Soc. Interface 11, 20130961–20130961. doi: 10.1098/rsif.2013.0961
Majeed, S., Hill, S. R., and Ignell, R. (2014). Impact of elevated CO2 background levels on the host-seeking behaviour of Aedes aegypti. J. Exp. Biol. 217, 598–604. doi: 10.1242/jeb.092718
Manciocco, A., Toni, M., Tedesco, A., Malavasi, S., Alleva, E., and Cioni, C. (2015). The acclimation of European Sea Bass (Dicentrarchus labrax) to temperature: behavioural and neurochemical responses. Ethology 121, 68–83. doi: 10.1111/eth.12315
Manríquez, P., Elisa Jara, M., Mardones, M., Torres, R., Navarro, J., Lardies, M., et al. (2014). Ocean acidification affects predator avoidance behaviour but not prey detection in the early ontogeny of a keystone species. Mar. Ecol. Prog. Ser. 502, 157–167. doi: 10.3354/meps10703
McCormick, M. I., Barry, R. P., and Allan, B. J. M. (2017). Algae associated with coral degradation affects risk assessment in coral reef fishes. Sci. Rep. 7:16937. doi: 10.1038/s41598-017-17197-1
McCormick, M. I., and Lönnstedt, O. M. (2013). Degrading habitats and the effect of topographic complexity on risk assessment. Ecol. Evol. 3, 4221–4229. doi: 10.1002/ece3.793
McCormick, M. I., Watson, S.-A., Simpson, S. D., and Allan, B. J. M. (2018). Effect of elevated CO 2 and small boat noise on the kinematics of predator–prey interactions. Proc. R. Soc. B Biol. Sci. 285:20172650. doi: 10.1098/rspb.2017.2650
McMahon, S., Donelson, J., and Munday, P. (2018). Food ration does not influence the effect of elevated CO2 on antipredator behaviour of a reef fish. Mar. Ecol. Progr. Ser. 586, 155–165. doi: 10.3354/meps12397
Meager, J. J., Solbakken, T., Utne-Palm, A. C., and Oen, T. (2005). Effects of turbidity on the reactive distance, search time, and foraging success of juvenile Atlantic cod (Gadus morhua). Can. J. Fish. Aquat. Sci. 62, 1978–1984. doi: 10.1139/f05-104
Miles, R. N., Robert, D., and Hoy, R. R. (1995). Mechanically coupled ears for directional hearing in the parasitoid fly Ormia ochracea. J. Acoust. Soc. Am. 98, 3059–3070. doi: 10.1121/1.413830
Miller, L. P., Matassa, C. M., and Trussell, G. C. (2014). Climate change enhances the negative effects of predation risk on an intermediate consumer. Glob. Change Biol. 20, 3834–3844. doi: 10.1111/gcb.12639
Moir, F., and Weissburg, M. J. (2009). Cautious cannibals: behavioral responses of juvenile and adult blue crabs to the odor of injured conspecifics. J. Exp. Mar. Biol. Ecol. 369, 87–92. doi: 10.1016/j.jembe.2008.10.026
Munday, P. L., Dixson, D. L., Donelson, J. M., Jones, G. P., Pratchett, M. S., Devitsina, G. V., et al. (2009). Ocean acidification impairs olfactory discrimination and homing ability of a marine fish. Proc. Natl. Acad. Sci. U.S.A. 106, 1848–1852. doi: 10.1073/pnas.0809996106
Munday, P. L., Dixson, D. L., McCormick, M. I., Meekan, M., Ferrari, M. C. O., and Chivers, D. P. (2010). Replenishment of fish populations is threatened by ocean acidification. Proc. Natl. Acad. Sci. U.S.A. 107, 12930–12934. doi: 10.1073/pnas.1004519107
Munday, P. L., Pratchett, M. S., Dixson, D. L., Donelson, J. M., Endo, G. G. K., Reynolds, A. D., et al. (2013). Elevated CO2 affects the behavior of an ecologically and economically important coral reef fish. Mar. Biol. 160, 2137–2144. doi: 10.1007/s00227-012-2111-6
Munday, P. L., Welch, M. J., Allan, B. J. M., Watson, S.-A., McMahon, S. J., and McCormick, M. I. (2016). Effects of elevated CO2 on predator avoidance behaviour by reef fishes is not altered by experimental test water. PeerJ. 4:e2501. doi: 10.7717/peerj.2501
Munk, W. H., and Forbes, A. M. G. (1989). Global Ocean Warming: An Acoustic Measure? J. Phys. Oceanogr. 19, 1765–1778. doi: 10.1175/1520-0485(1989)019<1765:GOWAAM>2.0.CO;2
Näslund, J., Lindström, E., Lai, F., and Jutfelt, F. (2015). Behavioural responses to simulated bird attacks in marine three-spined sticklebacks after exposure to high CO2 levels. Mar. Freshw. Res. 66, 877–885. doi: 10.1071/MF14144
Natt, M., Lönnstedt, O. M., and McCormick, M. I. (2017). Coral reef fish predator maintains olfactory acuity in degraded coral habitats. PLoS ONE. 12:e0179300. doi: 10.1371/journal.pone.0179300
Nilsson, G. E., Dixson, D. L., Domenici, P., McCormick, M. I., Sorensen, C., Watson, S. A., et al. (2012). Near-future carbon dioxide levels alter fish behaviour by interfering with neurotransmitter function. Nat. Clim. Change 2, 201–204. doi: 10.1038/Nclimate1352
O'Connor, J. J., Lecchini, D., Beck, H. J., Cadiou, G., Lecellier, G., Booth, D. J., et al. (2016). Sediment pollution impacts sensory ability and performance of settling coral-reef fish. Oecologia 180, 11–21. doi: 10.1007/s00442-015-3367-6
O'Connor, N. E., Grabowski, J. H., Ladwig, L. M., and Bruno, J. F. (2008). Simulated predator extinctions: Predator identity affects survival and recruitment of oysters. Ecology 89, 428–438. doi: 10.1890/06-2029.1
Ode, P. J., Johnson, S. N., and Moore, B. D. (2014). Atmospheric change and induced plant secondary metabolites - are we reshaping the building blocks of multi-trophic interactions? Curr. Opin. Insect Sci. 5, 57–65. doi: 10.1016/j.cois.2014.09.006
Orr, J. C., Fabry, V. J., Aumont, O., Bopp, L., Doney, S. C., Feely, R. A., et al. (2005). Anthropogenic ocean acidification over the twenty-first century and its impact on calcifying organisms. Nature 437, 681–686. doi: 10.1038/nature04095
Ou, M., Hamilton, T. J., Eom, J., Lyall, E. M., Gallup, J., Jiang, A., et al. (2015). Responses of pink salmon to CO2-induced aquatic acidification. Nat. Clim. Change 5:950. doi: 10.1038/nclimate2694
Paerl, H. W., and Huisman, J. (2009). Climate change: a catalyst for global expansion of harmful cyanobacterial blooms. Environ. Microbiol. Rep. 1, 27–37. doi: 10.1111/j.1758-2229.2008.00004.x
Paganini, A. W., Miller, N. A., and Stillman, J. H. (2014). Temperature and acidification variability reduce physiological performance in the intertidal zone porcelain crab Petrolisthes cinctipes. J. Exp. Biol. 217, 3974–3980. doi: 10.1242/jeb.109801
Paine, R. T. (1966). Food web complexity and species diversity. Am. Nat. 100, 65–75. doi: 10.1086/282400
Peñuelas, J., and Staudt, M. (2010). BVOCs and global change. Trends Plant Sci. 15, 133–144. doi: 10.1016/j.tplants.2009.12.005
Pistevos, J. C., Nagelkerken, I., Rossi, T., Olmos, M., and Connell, S. D. (2015). Ocean acidification and global warming impair shark hunting behaviour and growth. Sci. Rep. 5:16293. doi: 10.1038/srep16293
Pistevos, J. C. A., Nagelkerken, I., Rossi, T., and Connell, S. D. (2017). Antagonistic effects of ocean acidification and warming on hunting sharks. Oikos 126, 241–247. doi: 10.1111/oik.03182
Porteus, C. S., Hubbard, P. C., Webster, T. M. U., Aerle, R., van, Canário, A. V. M., Santos, E. M., et al. (2018). Near-future CO 2 levels impair the olfactory system of a marine fish. Nat. Climate Change 8, 737–743. doi: 10.1038/s41558-018-0224-8
Pörtner, H. O., and Farrell, A. P. (2008). Physiology and Climate Change. Science 322, 690–692. doi: 10.1126/science.1163156
Poulin, R. X., Lavoie, S., Siegel, K., Gaul, D. A., Weissburg, M. J., and Kubanek, J. (2018). Chemical encoding of risk perception and predator detection among estuarine invertebrates. Proc. Natl. Acad. Sci. U.S.A. 115, 662–667. doi: 10.1073/pnas.1713901115
Pruett, J. L., and Weissburg, M. J. (2018). Hydrodynamics affect predator controls through physical and sensory stressors. Oecologia, 186, 1079–1089. doi: 10.1007/s00442-018-4092-8
Queirós, A. M., Fernandes, J. A., Faulwetter, S., Nunes, J., Rastrick, S. P. S., Mieszkowska, N., et al. (2015). Scaling up experimental ocean acidification and warming research: from individuals to the ecosystem. Glob. Change Biol. 21, 130–143. doi: 10.1111/gcb.12675
Ripple, W. J., Larsen, E. J., Renkin, R. A., and Smith, D. W. (2001). Trophic cascades among wolves, elk and aspen on Yellowstone National Park's northern range. Biol. Conserv. 102, 227–234. doi: 10.1016/S0006-3207(01)00107-0
Rittschof, D. (1990). Peptide-mediated behaviors in marine organisms Evidence for a common theme. J. Chem. Ecol. 16, 261–272. doi: 10.1007/BF01021283
Roggatz, C. C., Lorch, M., Hardege, J. D., and Benoit, D. M. (2016). Ocean acidification affects marine chemical communication by changing structure and function of peptide signalling molecules. Glob. Change Biol. 22, 3914–3926. doi: 10.1111/gcb.13354
Rosenblatt, A. E., and Schmitz, O. J. (2016). Climate change, nutrition, and bottom-up and top-down food web processes. Trends Ecol. Evol. 31, 965–975. doi: 10.1016/j.tree.2016.09.009
Rossi, T., Connell, S. D., and Nagelkerken, I. (2016a). Silent oceans: ocean acidification impoverishes natural soundscapes by altering sound production of the world's noisiest marine invertebrate. Proc. R. Soc. B Biol. Sci. 283:20153046. doi: 10.1098/rspb.2015.3046
Rossi, T., Nagelkerken, I., Pistevos, J. C., and Connell, S. D. (2016b). Lost at sea: ocean acidification undermines larval fish orientation via altered hearing and marine soundscape modification. Biol. Lett. 12:20150937. doi: 10.1098/rsbl.2015.0937
Rossi, T., Nagelkerken, I., Simpson, S. D., Pistevos, J. C. A., Watson, S.-A., Merillet, L., et al. (2015). Ocean acidification boosts larval fish development but reduces the window of opportunity for successful settlement. Proc. R. Soc. B Biol. Sci. 282:20151954. doi: 10.1098/rspb.2015.1954
Scherer, A. E., Lunt, J., Draper, A. M., and Smee, D. L. (2016). Phenotypic plasticity in oysters (Crassostrea virginica) mediated by chemical signals from predators and injured prey. Invertebr. Biol. 135, 97–107. doi: 10.1111/ivb.12120
Scherer, A. E., and Smee, D. L. (2016). A review of predator diet effects on prey defensive responses. Chemoecology 26, 83–100. doi: 10.1007/s00049-016-0208-y
Schmitz, O. J. (2008). Effects of predator hunting mode on grassland ecosystem function. Science 319, 952–954. doi: 10.1126/science.1152355
Schoeppner, N. M., and Relyea, R. A. (2005). Damage, digestion, and defence: the roles of alarm cues and kairomones for inducing prey defences. Ecol. Lett. 8, 505–512. doi: 10.1111/j.1461-0248.2005.00744.x
Shears, N. T., Babcock, R. C., and Salomon, A. K. (2008). Context-dependent effects of fishing: variation in trophic cascades across environmental gradients. Ecol. Appl. 18, 1860–1873. doi: 10.1890/07-1776.1
Silliman, B. R., and Bertness, M. D. (2002). A trophic cascade regulates salt marsh primary production. Proc. Natl. Acad. Sci. U.S.A. 99, 10500–10505. doi: 10.1073/pnas.162366599
Simpson, S. D., Munday, P. L., Wittenrich, M. L., Manassa, R., Dixson, D. L., Gagliano, M., et al. (2011). Ocean acidification erodes crucial auditory behaviour in a marine fish. Biol. Lett. 7, 917–920. doi: 10.1098/rsbl.2011.0293
Slabbekoorn, H., and Smith, T. B. (2002). Habitat-dependent song divergence in the little greenbul: an analysis of environmental selection pressures on acoustic signals. Evolution 56, 1849–1858. doi: 10.1111/j.0014-3820.2002.tb00199.x
Smee, D. L., Ferner, M. C., and Weissburg, M. J. (2010). Hydrodynamic sensory stressors produce nonlinear predation patterns. Ecology 91, 1391–1400. doi: 10.1890/09-0017.1
Spady, B. L., Watson, S.-A., Chase, T. J., and Munday, P. L. (2014). Projected near-future CO2 levels increase activity and alter defensive behaviours in the tropical squid Idiosepius pygmaeus. Biology Open 3, 1063–1070. doi: 10.1242/bio.20149894
Strod, T., Izhaki, I., Arad, Z., and Katzir, G. (2008). Prey detection by great cormorant (Phalacrocorax carbo sinensis) in clear and in turbid water. Journal of Experimental Biology 211, 866–872. doi: 10.1242/jeb.014324
Sui, Y., Hu, M., Huang, X., Wang, Y., and Lu, W. (2015). Anti-predatory responses of the thick shell mussel Mytilus coruscus exposed to seawater acidification and hypoxia. Mar. Environ. Res. 109, 159–167. doi: 10.1016/j.marenvres.2015.07.008
Sundin, J., Amcoff, M., Mateos-González, F., Raby, G. D., Jutfelt, F., and Clark, T. D. (2017). Long-term exposure to elevated carbon dioxide does not alter activity levels of a coral reef fish in response to predator chemical cues. Behav. Ecol. Sociobiol. 71:108. doi: 10.1007/s00265-017-2337-x
Sundin, J., and Jutfelt, F. (2016). 9–28 d of exposure to elevated p CO 2 reduces avoidance of predator odour but had no effect on behavioural lateralization or swimming activity in a temperate wrasse (Ctenolabrus rupestris). ICES J. Mar. Sci. J. Cons. 73, 620–632. doi: 10.1093/icesjms/fsv101
Swanbrow Becker, L. J., and Gabor, C. R. (2012). Effects of Turbidity and Visual vs. Chemical Cues on Anti-Predator Response in the Endangered Fountain Darter (Etheostoma fonticola). Ethology 118, 994–1000. doi: 10.1111/eth.12002
Sweka, J. A., and Hartman, K. J. (2003). Reduction of reactive distance and foraging success in smallmouth bass, Micropterus dolomieu, exposed to elevated turbidity levels. Environ. Biol. Fishes 67, 341–347. doi: 10.1023/A:1025835031366
Symonds, M. R. E., and Elgar, M. A. (2008). The evolution of pheromone diversity. Trends Ecol. Evol. 23, 220–228. doi: 10.1016/j.tree.2007.11.009
Tix, J. A., Hasler, C. T., Sullivan, C., Jeffrey, J. D., and Suski, C. D. (2017). Elevated carbon dioxide has the potential to impact alarm cue responses in some freshwater fishes. Aquat. Ecol. 51, 59–72. doi: 10.1007/s10452-016-9598-8
Turner, A. M. (2008). Predator diet and prey behaviour: freshwater snails discriminate among closely related prey in a predator's diet. Anim. Behav. 76, 1211–1217. doi: 10.1016/j.anbehav.2008.06.005
Van de Meutter, F., Meester, L. D., and Stoks, R. (2005). Water turbidity affects predator–prey interactions in a fish–damselfly system. Oecologia 144, 327–336. doi: 10.1007/s00442-005-0050-3
Wang, X., Song, L., Chen, Y., Ran, H., and Song, J. (2017). Impact of ocean acidification on the early development and escape behavior of marine medaka (Oryzias melastigma). Mar. Environ. Res. 131, 10–18. doi: 10.1016/j.marenvres.2017.09.001
Wang, Y., Hu, M., Wu, F., Storch, D., and Pörtner, H.-O. (2018). Elevated pCO2 Affects feeding behavior and acute physiological response of the brown crab cancer pagurus. Front. Physiol. 9:1164. doi: 10.3389/fphys.2018.01164
Wang, Y., Li, L., Hu, M., and Lu, W. (2015). Physiological energetics of the thick shell mussel Mytilus coruscus exposed to seawater acidification and thermal stress. Sci. Total Environ. 514, 261–272. doi: 10.1016/j.scitotenv.2015.01.092
Warren, D. T., Donelson, J. M., and McCormick, M. I. (2017). Extended exposure to elevated temperature affects escape response behaviour in coral reef fishes. PeerJ. 5:e3652. doi: 10.7717/peerj.3652
Weissburg, M., Atkins, L., Berkenkamp, K., and Mankin, D. (2012). Dine or dash? Turbulence inhibits blue crab navigation in attractive-aversive odor plumes by altering signal structure encoded by the olfactory pathway. J. Exp. Biol. 215, 4175–4182. doi: 10.1242/jeb.077255
Weissburg, M., and Beauvais, J. (2015). The smell of success: the amount of prey consumed by predators determines the strength and range of cascading non-consumptive effects. PeerJ. 3:e1426. doi: 10.7717/peerj.1426
Weissburg, M., Smee, D. L., and Ferner, M. C. (2014). The sensory ecology of nonconsumptive predator effects. Am. Nat. 184, 141–157. doi: 10.1086/676644
Weissburg, M. J., Ferner, M. C., Pisut, D. P., and Smee, D. L. (2002). Ecological consequences of chemically mediated prey perception. J. Chem. Ecol. 28, 1953–1970. doi: 10.1023/A:1020741710060
Weissburg, M. J., and Zimmerfaust, R. K. (1994). Odor plumes and how blue crabs use them in finding prey. J. Exp. Biol. 197, 349–375.
Welch, M. J., Watson, S.-A., Welsh, J. Q., McCormick, M. I., and Munday, P. L. (2014). Effects of elevated CO2 on fish behaviour undiminished by transgenerational acclimation. Nat. Clim. Change 4:1086–1089. doi: 10.1038/nclimate2400
Werner, E. E., and Peacor, S. D. (2003). A review of trait-mediated indirect interactions in ecological communities. Ecology 84, 1083–1100. doi: 10.1890/0012-9658(2003)084[[1083:Arotii]]2.0.Co;2
Williams, C. R., Dittman, A. H., McElhany, P., Busch, D. S., Maher, M. T., Bammler, T. K., et al. (2018). Elevated CO2 impairs olfactory-mediated neural and behavioral responses and gene expression in ocean-phase coho salmon (Oncorhynchus kisutch). Glob. Change Biol. 25, 963–977. doi: 10.1111/gcb.14532
Keywords: climate change, global warming, carbon dioxide, sensory ecology, behavior, predator-prey interactions
Citation: Draper AM and Weissburg MJ (2019) Impacts of Global Warming and Elevated CO2 on Sensory Behavior in Predator-Prey Interactions: A Review and Synthesis. Front. Ecol. Evol. 7:72. doi: 10.3389/fevo.2019.00072
Received: 01 October 2018; Accepted: 25 February 2019;
Published: 20 March 2019.
Edited by:
Evan Preisser, University of Rhode Island, United StatesReviewed by:
Youji Wang, Shanghai Ocean University, ChinaCopyright © 2019 Draper and Weissburg. This is an open-access article distributed under the terms of the Creative Commons Attribution License (CC BY). The use, distribution or reproduction in other forums is permitted, provided the original author(s) and the copyright owner(s) are credited and that the original publication in this journal is cited, in accordance with accepted academic practice. No use, distribution or reproduction is permitted which does not comply with these terms.
*Correspondence: Marc J. Weissburg, bWFyYy53ZWlzc2J1cmdAYmlvbG9neS5nYXRlY2guZWR1
Disclaimer: All claims expressed in this article are solely those of the authors and do not necessarily represent those of their affiliated organizations, or those of the publisher, the editors and the reviewers. Any product that may be evaluated in this article or claim that may be made by its manufacturer is not guaranteed or endorsed by the publisher.
Research integrity at Frontiers
Learn more about the work of our research integrity team to safeguard the quality of each article we publish.