- 1Department of Epidemiology of Microbial Diseases, Yale University School of Public Health, New Haven, CT, United States
- 2W. Harry Feinstone Department of Molecular Microbiology and Immunology, The Johns Hopkins Bloomberg School of Public Health, Baltimore, MD, United States
- 3Geography Department and Emerging Pathogens Institute, University of Florida, Gainesville, FL, United States
Two Old World rodents, house mice (Mus musculus) and Norway rats (Rattus norvegicus), were introduced into and established populations on every continent, save Antarctica. With their travels, they concomitantly introduced several zoonotic agents capable of causing human diseases. Two viruses—Lymphocytic choriomeningitis virus (LCMV; genus Arenavirus with mice) and Seoul virus (SEOV; genus Hantavirus with rats)—can cause chronic infections within their respective rodent hosts, resulting in persistent or life-long sporadic shedding of virus through secreta and excreta. Although the prevalence of infection within their wild rodent hosts can exceed 25% among mice infected with LCMV and 50% among rats infected with SEOV, acute human disease resulting from direct transmission from wild rodents is rarely reported even though both species live in close coexistence with humans. The usual “classic” zoonotic cycle of transmission from wild rodent reservoirs to humans now includes multiple unusual/unexpected routes. The largest described outbreaks of human disease caused by these viruses are linked to pet rodents. A novel reservoir host, the golden hamster, has supplanted house mice as the major source of LCMV infection, and SEOV outbreaks are linked to fancy rats kept as pets. Following LCMV, and to a lesser extent SEOV, outbreaks or infections associated with lab animals and/or cultured tissues derived from mice and hamsters have led to hundreds of cases of LCMV among laboratory workers, and SEOV has been detected among cell-cultured tissues. Additionally, LCMV is now a recognized source of severe congenital disease and is the unexpected source of severe and often fatal disease among solid organ recipients. Although the extensive usual and unusual routes of LCMV infection are exceptional there are many parallels with SEOV emergence.
Introduction
The critical role of zoonoses in the emergence of new infectious diseases impacting humans and/or domestic animals has reached a level of near doctrine. Associated with this perspective has been an in-depth effort to study the dynamics of pathogens in reservoir populations with less focus on the significant role of the dynamics of the target (human or domestic animal) population in changing patterns of transmission. Previously, one of the authors emphasized the roles of adaptation and changes in social/behavioral activities that could qualitatively change risk patterns (Childs, 2004). However, relatively little focus continues to be paid to these factors.
As an example of what we believe is a critically understudied aspect of emerging diseases, we review the evolution of risk patterns from two viral rodent-borne zoonoses during the past 80 years. These agents, lymphocytic choriomeningitis virus (LCMV) and Seoul virus (SEOV), are among the earliest and best studied agents. Historically, the house mouse (Mus musculus) and the Norway rat (Rattus norvegicus) have been recognized as their reservoirs. Close contact with wild populations of these rodents has been viewed as the major risk factor for humans. During the past several decades, however, as research has progressed, a more nuanced understanding of how human activities have altered human risk has developed.
In this paper we outline the history of the discoveries of these agents, the diseases they cause, why they persist in their reservoir populations and how human activities and unforeseen events widened our understanding of the epidemiology of these viruses and the spectrum of people at risk for infection.
History of Lymphocytic Choriomeningitis (LCM) and LCM Virus (LCMV)
Lymphocytic choriomeningitis (LCM) was first described as a cause of aseptic meningitis among hospitalized patients in St. Louis, Missouri, USA, in the early 1930s (Armstrong and Lillie, 1934; Rivers and Scott, 1935). Although rarely reported as of 2018, LCMV was once a significant cause of aseptic meningitis. Hospital-based studies conducted from 1953 to 1958 identified LCM among 8% of cases of encephalitis among 713 hospitalized patients (Meyer et al., 1960). Infection, however does not always cause disease among humans, and clinical cases appear rare among the general population.
Lymphocytic choriomeningitis virus (LCMV) is a bi-segmented, negative sense RNA virus (Bishop and Auperin, 1987). The two gene segments are labeled L (7.5 kb) and S (3.5 kb). LCMV is the type species of the family Arenaviridae which includes Lassa, Junin, Machupo, and Sabia—the latter four viruses are closely related arenaviruses causing hemorrhagic disease among humans. Over the years, significant genetic heterogeneity and phenotype of clinical course have been shown to vary with different LCMV isolates. However, numerous LCMV variants can cause severe disease among humans (Amman et al., 2007; Emonet et al., 2007; Palacios et al., 2008) and significant genetic variation occurs among isolates (Albariño et al., 2010). LCMV has served as a model system for understanding the immunobiology of virus infections (Zhou et al., 2012), and in 1996 Nobel prizes were awarded to two individuals (Peter Doherty and Rolf Zinkernagel) who used LCMV as a model to enumerate the immunobiology of virus persistence and differential immune responses based on modes of transmission.
In humans acute disease is marked by fever, headache, myalgia and other non-specific signs and symptoms typical of many viral infections (Farmer and Janeway, 1942; Biggar et al., 1975a,b; Folk et al., 2011). LCM presents without the lymphopenia and thrombocytopenia characteristic of severe disease caused by closely related arenaviruses associated with hemorrhagic fever. In LCM cerebral fluid pleocytosis, choriomeningitis and other neurological signs and symptoms develop among some patients after an apparent remission of acute symptoms.
Acute LCM is not a mandated reportable disease and few cases are identified as of 2018. Without any doubt this infection and disease is significantly underestimated, in part because of limited facilities offering diagnostic testing (Barton et al., 1995; Barton and Hyndman, 2000).
Diagnosis of LCM
The most commonly used tests for identifying LCMV infections among humans and animals are serological assays that detect antibodies to the virus in serum and cerebral spinal fluid. The ELISA (enzyme-linked immunosorbent antibody test) is cheap to perform, and may be followed by a confirmatory immuno-fluorescent antibody test (IFA) or plaque reduction neutralization assay (PRNT), which is the gold standard of serological tests that distinguishes among different arenaviruses (Armstrong and Lillie, 1934; Rivers and Scott, 1935; Traub, 1936a; Wooley et al., 1939; Fischer et al., 2006; Becker et al., 2007; Takimoto et al., 2008; Knust et al., 2011). Currently, RT-PCR detection is available at specialty labs.
Antibody assays can detect both IgM (recent or ongoing infection) and IgG (infection at some time); a four-fold increase in IgG antibody titer between two sampling dates is diagnostic of recent infection. However, serum and intrathecal levels (measured in cerebral spinal fluid—CSF) of LCMV antibody are always low but in suspected cases, even a low titer of LCMV antibody aids the diagnosis (Sukthana, 2006). The neutralization test requires live virus and is available only in specialty labs because of the obvious risk of human exposure and infection among unsuitably protected lab personnel.
The incidence of infection among humans is unknown (Centers for Disease Control and Prevention, 2006). On-site diagnostic testing for LCMV infection is limited among laboratories serving acute care hospitals, as exemplified by a survey in which none of 30 such facilities contacted in Connecticut, United States, had the in-house means of testing. In the same survey most infectious disease doctors (28 responses out of 35 sent) would consider LCM in a differential diagnosis if there was a history of contact with wild mice or a healthy or sick pet rodent; only six would consider LCMV as a potential cause of unexplained fever in immunocompromised patients without an exposure history (Centers for Disease Control and Prevention, 2006).
Epidemiology of LCM
The routes of LMCV transmission to humans are believed to include fine particle aerosols arising from infected secreta and excreta (saliva and urine most commonly), droplets, fomites (e.g., contaminated bedding of laboratory, commercial colonies or pet rodents), bites and contact with rodent blood. Exact routes of transmission of LCMV from mice to humans have not been evaluated—in part because large house mouse-associated outbreaks do not occur—but aerosol transmission is most probable.
LCMV infection acquired from pet rodents, notably the Syrian golden hamster (see sections below), provide significant evidence of aerosol, droplet, or fomite transmission of LCMV. In some of these occurrences multiple family members have become infected, some of whom reported no physical contact with the animal (Biggar et al., 1975a,b, 1977). Many laboratory infections of humans appear to be airborne (Armstrong et al., 1969; Vanzee et al., 1975; Hotchin et al., 1977). Sentinel guinea pigs housed in the same room but separated from infected mice show a high susceptibility to airborne infection and experimental studies have demonstrated high susceptibility of mice and guinea pigs to airborne exposure with LCMV (Benda, 1964).
LCMV presents little risk of infection to general populations. Various serologic tests indicate a prevalence of antibody of 1–5% of persons (Childs et al., 1991; Stephensen, 1992; Childs and Wilson, 1994; Knust et al., 2011), although it is unclear how many of these persons suffered from acute disease. It is likely that the prevalence of antibody would be greater among certain populations if surveyed. In some locations severe mouse infestations, and many LCMV-infected mice, are present in low-socioeconomic status (SES) urban locations containing substandard housing with inadequate sanitation and waste removal. The same features provide an excellent habitat for brown rats (R. norvegicus see sections on Seoul virus below: Figure 1A) (Childs et al., 1991, 1992). Early studies linking house mice to LCM often described heavy mouse infestations.
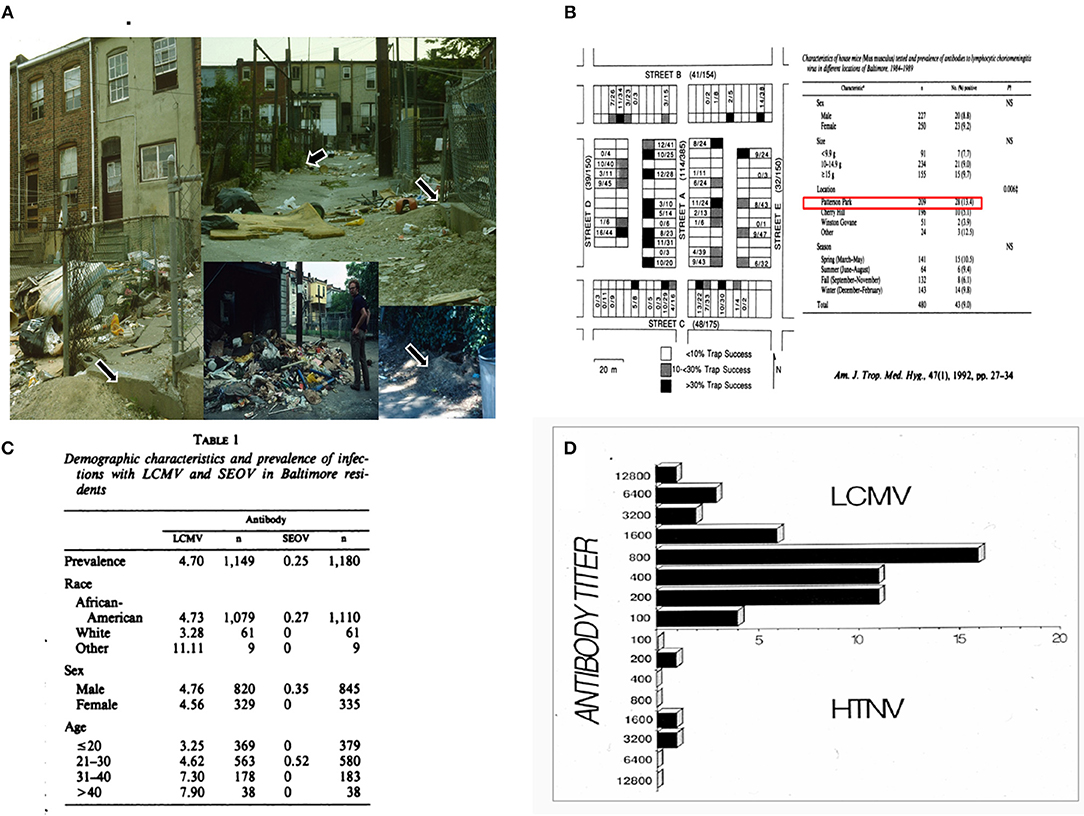
Figure 1. (A) Multiple scenes from alleys in Baltimore, MD, from which rats were captured to elucidate the natural history of SEOV (dark arrows indicate sites of extensive rat burrows). House mice were captured from row houses fronting on the alleys to study the epidemiology of LCMV. Mice were never captured in alleys and few rats were captured within residences. (B) Map on left shows the layout of one square block of row houses surrounding an alley shown in (A). The shading indicates mouse-trap success, an index of population density, obtained over a three-year period. The table to the left shows the prevalence of antibodies to LCMV among house mice captured among several study sites, indicating clustering of infection within blocks. (C) The prevalence of antibodies among individuals visiting an STD clinic in Baltimore, of which many resided in low-SES neighborhoods likely to harbor extensive Norway rat populations. The prevalence of LCMV antibodies increases with age, but the lack of sufficient data on SEOV precluded any similar conclusions. (D) Endpoint titers of antibody-positive sera to LCMV or SEOV.
Immunocompromised individuals or children born with an in utero infection acquired from an acutely infected mother are at the highest risk of developing severe and fatal disease (see below).
Enter the House Mouse (Mus musculus)
Shortly after the discovery of the disease, evidence rapidly accumulated implicating the house mouse as the reservoir host of LCMV transmitted to humans (Armstrong and Sweet, 1939) (see Figures 2A,B for an abbreviated timeline of some significant observations marking the history of LCMV). A year prior to the discovery of LCMV in wild house mice, laboratory colonies of albino mice were found infected. Additional research on these colonies elucidated the different phenology of vertically acquired (in utero) and horizontal intra-specific infection with regard to immunity, duration of virus persistence and shedding, and the pathological consequences of infection (the significance of vertical transmission is discussed below) (Traub, 1936a, 1938, 1939).
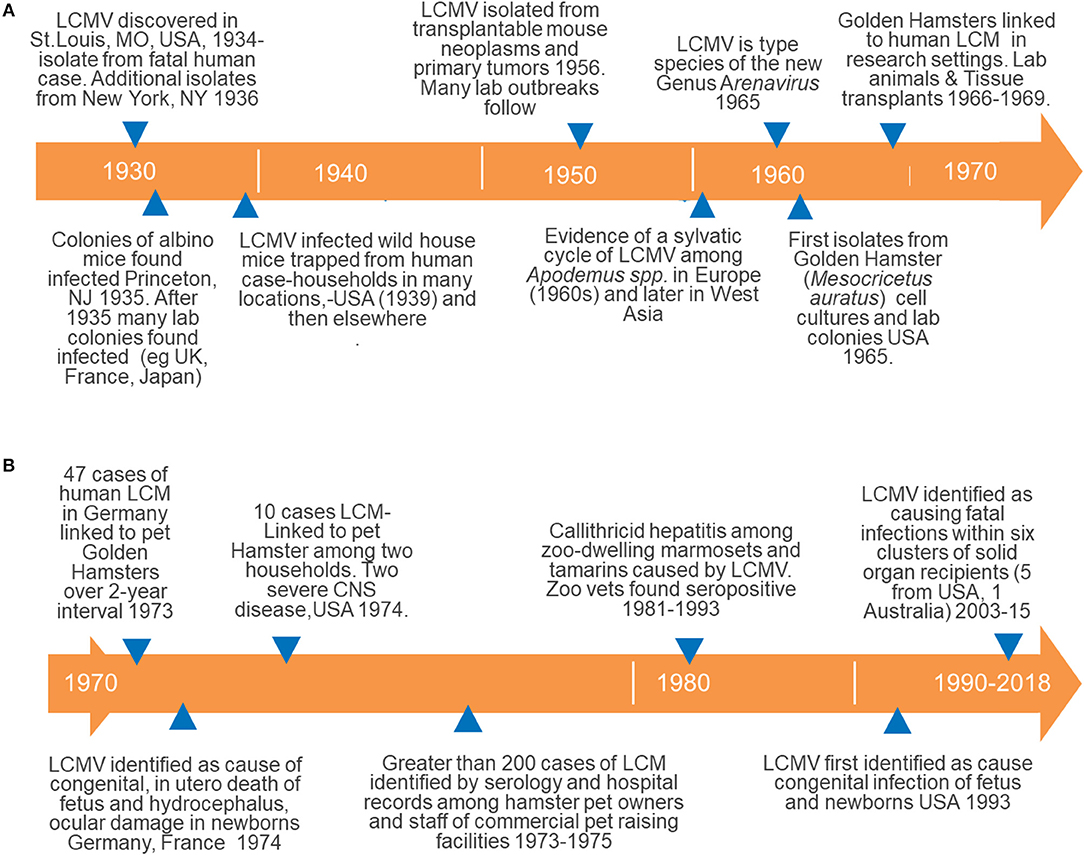
Figure 2. Timelines documenting the history of major events in our understanding of the epidemiology maintenance and transmission of LCMV to humans; (A) from the discovery of LCMV (1930s) and its association with wild house mice through the discovery of human-to-human transmission causing severe congenital disease; (B) details of the emergence of a transcendent host for LCMV, the golden hamster, through the recognition of LCMV among recipients of solid organ transplants. These figures illustrate the development of both usual and unusual sources of LCMV infection among humans as discussed in detail in the text.
The widespread introduction and global colonization by the house mice (Long, 2003) suggest that LCMV could enjoy a cosmopolitan distribution, and some authors suggest this (Charrel and de Lamballerie, 2010). However, most studies indicate that the distribution of infected mice is patchy and tends to cluster as discussed below. There are four subspecies of the genus Mus but herein we refer to them collectively as house mice or M. musculus.
Most available LCMV isolates come from the United States and West-Central Europe (Albariño et al., 2010), but reports of infection and isolation of LCMV are confirmed from Argentina (Sabattini et al., 1970, 1974), Japan (Morita et al., 1991), and variants of LCMV based on RNA sequencing have been reported from Australia (Dandenong virus) and Africa (Kudoko virus) (Lecompte et al., 2007; Palacios et al., 2008). As of 2009, no clinical cases of LCM had been described from Southeast Asia (Kim et al., 2009).
Describing/discovering a zoonotic reservoir for agents pathogenic to humans and transmitted by inter-species contact is old. The association between rabies, dog bite, and human disease dates to at least the year 500 B.C. (Steele et al., 1991). However, the observation that laboratory mice, in addition to wild mice, were infected with LCMV was significant as disease outbreaks occurred among research personnel working or in contact with lab mice (Figure 2A). Additionally, these findings necessitated reconsideration of published scientific reports based on mouse models (potentially contaminated by LCMV) and raised serious concerns over the health and sanitation of lab mouse colonies and the risk of infection for research staff and suppliers. Even short periods, such as 30 min, of contact between infected and susceptible mice kept within the same cage was sufficient for transmission to occur (Skinner and Knight, 1973). The “natural” history of LCMV maintenance and transmission to humans included only the normal suspects, albino, and wild house mice at this juncture in what developed into a far more complicated and unusual history (Figure 2A).
Of Mice and Monkeys
Humans are not the only primates in which mice derived from breeding stock of commercial vendors were the source of infection and severe disease. In 1981, two outbreaks of infectious hepatitis of unknown origin were reported from zoos in the United Kingdom and United States affecting marmosets and tamarins (Family: Callithricidae)—New World primates of which several of the affected spp. are considered endangered (Montali et al., 1989). Between 1981 and 1989, 12 outbreaks were reported from different zoos in the USA and this new, unique and fatal disease was named Callithrichid hepatitis (Montali et al., 1989).
Follow-up studies showed that inoculations of liver homogenates from an ill monkey into three common marmosets produced severe disease within 10 days; all monkeys either required euthanasia or died from infection. Known viruses capable of causing hepatitis in non-human primates were ruled out and the etiologic agent was unidentified (Montali et al., 1989). In 1991, the causative agent was identified as LCMV (Stephensen et al., 1991) and one outbreak was linked to a point source involving perinatal mice (“pinkies”) fed to these monkeys as a supplemental source of animal protein (Montali et al., 1993). In the same study, two veterinarians who autopsied infected monkeys and one individual bitten by an infected monkey had seroconverted to LCMV.
Epidemiology of LCMV among House Mice
The prevalence of LCMV infection among wild mouse populations has been reported to range from 0 to 25% (Figures 1C,D) (Stephensen, 1992; Childs and Peters, 1993; Childs and Wilson, 1994; Becker et al., 2007; Knust et al., 2011; Williams et al., 2018). LCMV infection of house mice can be highly focal at both large and small scales (see Childs and Peters, 1993). In Baltimore, Maryland, United States, house mouse densities and LCMV infection varied extensively among connected row houses studied in a two-block area (Figure 1B). Prevalence of LCMV infection varied between 50 and ~100% among mice in some houses while neighboring residences had few or no infected mice—observations also obtained from other studies (Childs et al., 1992; Emonet et al., 2007). There was a significant association between mouse density (trap success) and prevalence of LCMV at different sampling sites, which could reflect density-dependent horizontal transmission or clustering of chronically infected mice and vertical transmission (Figure 1B) (Childs et al., 1992).
At regional scales the distribution of LCMV is patchy. From 1960 to 1962, in possibly the most intensive effort to map LCMV distribution among wild house mice, 1795 house mice were collected from 376 evenly spaced trapping sites in the Federal Republic of Germany. Although the overall prevalence of infection was 3%, all 65 LCMV-positive mice came from a subset of 44 trapping sites (Ackermann et al., 1964).
Due to this patchiness of LCMV infections among house mice, negative findings are important and further illuminate the spotty distribution of this virus. In New York City, New York, United States, highly sensitive genetic methods failed to identify any LCMV among 395 mice captured from several sites (Williams et al., 2018). However, human LCM occurs within the city (Asnis et al., 2010).
The focality of LCMV infections among mice in some settings may be due to the combination of congenital transmission LCMV among mice (Lehmann-Grube, 1963, 1971) and the demic structure of house mouse populations (e.g., Petras, 1967; Singleton, 1983). Urban mice have an extremely limited dispersal distance when occupying human structures, such as row houses (Baltimore) and apartments (New York) (it is greater among mice inhabiting agricultural settings) (Pocock et al., 2005). Genetic studies of house mice populations found that mice inhabiting adjacent houses were closely related but mice sampled from houses on different blocks were genetically distinguishable (Murphy et al., 2005).
Persistent chronic infection of mice results in in utero vertical transmission of LCMV to fetuses and surviving offspring so that infected mice never clear the virus. Infection prevalence among such progeny can approach 100% (Traub, 1936c; Lehmann-Grube, 1963, 1971). Most importantly for virus transmission to humans, persistently infected mice shed LCMV in their saliva and urine throughout their lives (Traub, 1936a,b; Barber et al., 2006), generating material that can lead to aerosol infection within infested households. Infected laboratory mice appear to suffer no ill effects from their infection. However, tolerance of persistent LCMV infection is not absolute as deposits of antibody-virus complexes in the kidneys of older lab mice can lead to severe glomerulonephritis as antigen-antibody complexes accumulate in kidneys (see Buchmeier et al., 1980). This occurrence has not been shown among wild house mice, which probably do not live long enough to suffer from this late-onset complication.
House mice born from an uninfected dam can be infected later in life by horizontal transmission routes. These individuals develop antibodies, clear their infections and are believed not to become chronic shedders of LCMV, although demonstration of this phenomenon has not been confirmed by longitudinal study of wild mice.
Although viruses have evolved multiple mechanisms to escape the host immune system, of relevance to this review are the mechanisms viruses use to suppress antiviral defenses. The most well-established model system is LCMV infection of house mice. The Armstrong strain of LCMV is readily cleared from immunocompetent adult mice; conversely, LCMV Clone 13 differs from Armstrong (i.e., the parental strain) by 2 amino acid positions and causes persistent infection in adult mice (Salvato et al., 1988, 1991; Ahmed et al., 1991). Persistence of LCMV Clone 13 is associated with functional impairment (often referred to as “exhaustion”) and deletion of virus-specific CD8+ T cells, increased production of IL-10, and induction of programmed death (PD)-1 activity (Moskophidis et al., 1993; Barber et al., 2006; Ejrnaes et al., 2006). Blocking the IL-10R or PD-1/PD-L1 pathways promotes LCMV clearance (Barber et al., 2006; Brooks et al., 2006; Ejrnaes et al., 2006; Maris et al., 2007). The exhaustion phenotype of CD8+ T cells during persistent LCMV infection is mediated by Treg cells because depletion of Treg cells increases the expansion of LCMV-specific CD8+ T cells, but has no effect on virus persistence (Penaloza-MacMaster et al., 2014).
Other Wild Rodent Hosts
Other sylvatic rodents have been shown to be infected with LCMV. Species of Apodemus infected with LCMV in Europe were identified as early as the 1950s and continue to be found to the present (Figure 2A; for descriptions of early findings see (Lehmann-Grube, 1971, 1984). Exposure to Apodemus mice and cases of LCM among humans have not been conclusively identified.
Back to the Research Laboratory
The next chapter in the unusual history of LVMC began in the 1940s−1950s, when scientific investigators identified LCMV among transplantable mouse tumors and laboratory cell lines—including a line used for growing rabies virus for vaccines (Figure 2A: see Hotchin, 1971; Hotchin et al., 1977 for review). Clearly this unanticipated route of human exposure was not one of the usual routes of transmission thought previously to be restricted to contact with living rodents (Figure 1A). Additionally, in 1992 LCMV laboratory personnel working with nude mice were shown to be LCMV-positive by serology (Dykewicz et al., 1992).
Within a year, other observations indicated that infected and infectious cell cultures derived from an additional species, the Syrian or golden hamster (Mesocricetus auratus), were responsible for human laboratory infections (Figure 2A) (Lewis et al., 1965; Hotchin, 1971). But not only cell cultures were incriminated, as over the course of 2 years, 1971–1973, 48 staff members of the University of Rochester Medical Center were sickened by LCM acquired by working directly with hamsters (Figure 2A, unusual because the first infections arising from hamsters came from cultured bits rather than the whole animal (Vanzee et al., 1975; Hotchin et al., 1977).
These findings were alarming enough but the public health problem was quickly shown to extend beyond sporadic reports of infection/disease in laboratorians. Reports of pet hamster-associated LCM were identified in the early 1970s when 47 cases from pet hamsters were reported over 2 years in Germany (Figure 2B) (Ackermann et al., 1972). Within 3 years, 1973–1975, serological and clinical studies identified pet hamster-associated LCM among 181 persons living in 12 states in the United States. Many of these cases were identified through a national alert issued by the CDC after investigating an initial cluster of cases among pet owners (summarized within Gregg, 1975). Other reports continued to surface over years from a number of countries.
These findings heralded in the highly unusual emergence of a secondary host for LCMV that was associated closely with humans as a laboratory animal and household pet (Skinner et al., 1976). Most significantly, the size of outbreaks associated with hamsters dwarfs outbreaks and sporadic occurrences of LCM associated with mice by many fold. A novel reservoir host had supplanted the natural house mouse reservoir as the major link to human infection and disease, transforming itself from its status of “not the usual suspect” to one of “the usual suspects.”
A Brief Natural History of Hamsters
Hamsters are native to much of Europe and Asia (Wilson and Reeder, 2005). Of the over 20 spp. of hamsters, only five are kept as pets with the most popular being the golden hamster. As with the house mouse they are introduced species to the New World, although their introduction was intentional.
The first lab colony of golden hamsters was derived from wild-caught animals in ~1930 and housed at Hebrew University, Jerusalem. From this initial colony these readily domesticated animals made their way into many research settings, including the United States, after the first established commercial breeding facilities appeared in the 1940s (Adler, 1948). Almost simultaneously, hamsters were marketed as adorable household pets. Based on a survey conducted by the American Veterinary Association in 2012, the number of households in the United States owning a pet hamster was estimated at 877,000, with 1,146,000 individuals owned (American Veterinary Medical Association, 2012).
Enter Human-to-Human Transmission
Of major significance to human health are two other twists in LCMV's mechanisms of transmission to humans associated with severe and fatal disease. Unlike many of the arenaviruses causing hemorrhagic fevers, LCMV had not been shown to be transmitted from human to human. Acquired LCMV infection from contact with a reservoir, including cultured cells, rarely causes human severe disease or fatalities; however, the discovery of in utero infection of the fetus from Europe beginning in 1974 changed this perception (Figure 2B) (Ackermann et al., 1972). This was a clear and unusual departure from previously described transmission routes (Figure 2A). When acute infection of pregnancy occurs during the first trimester of pregnancy, symptomatic in utero and/or perinatal infection results in significant neurological disease, such as hydrocephalus, microcephaly, hydrops fetalis, and choriomeningitis. Surviving young suffer from permanent sequelae. This significant development in the changing epidemiology of LCM was not described until almost 20 years later in 1990s in the United States (van den Pol, 2006; Bonthius and Perlman, 2007; Kang and McGavern, 2008; Meritet et al., 2009).
Pregnant women rarely show any indication of acute LCM. Presumably, their immunosuppressed state predisposes them to acute infection which can then be transmitted to the fetus. Support for this observation comes from reports of infection and severe and often fatal disease among other immunosuppressed persons, such as organ recipients (see below) (Table 1). That this virus is an important, but underestimated, cause of fetal infection is likely accurate as diagnostic testing is restricted. However, only 58+ incidents of congenital LCM have been reported up to 2010, and the TORCH agents are ascribed to most cases.
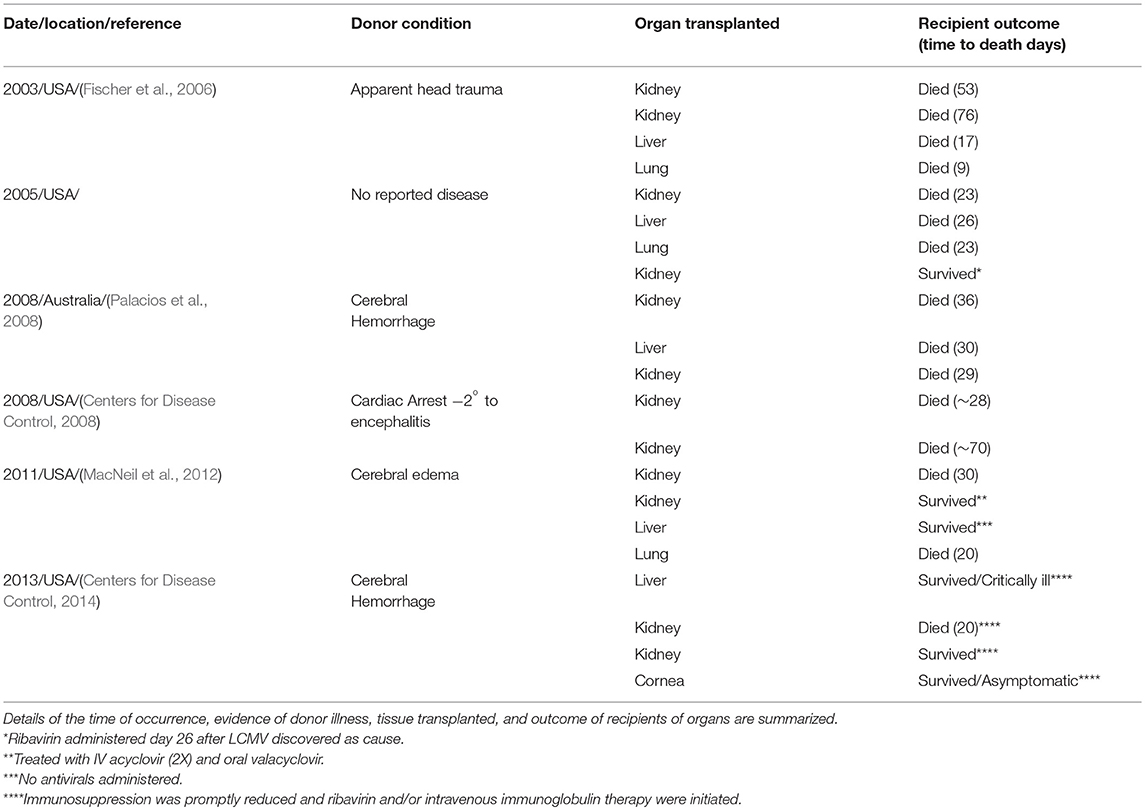
Table 1. Six clusters of solid organ-associated LCMV infection; five from the United States and one from Australia.
Many authors now refer to LCM as an “emerging disease” or “re-emerging disease” (Armstrong and Sweet, 1939; Barton et al., 2002; Fischer et al., 2006; Jamieson et al., 2006; Asnis et al., 2010; Barton, 2010). In the case of LCMV, most of the emerging was due to recognition of congenital disease rather than an increase in infection/disease among the general public.
Another Novel and Disturbing Development
In 2003 and again in 2005, the medical community was taken by surprise by the identification of severe and most often fatal LCM among transplant patients (Table 1; Figure 1B) [see Perspective (Peters, 2006) for additional comments about the evolution of LCMV transmission to humans]. Not only was this heretofore undiscovered transmission route of epidemiologic importance, it was a clear demonstration that LCM goes undiagnosed in acute disease or infection and is certainly significantly underestimated. Donors had to be acutely infected as, unlike in mice, the virus does not persist. Thus, the organ donor became a new transmission source and a very unusual suspect.
In total, five clusters of LCM derived from donors in the United States have resulted in recipients with 21 cases and 15 deaths (Table 1). One survivor suffered severe neurological sequelae (Mathur et al., 2017). An additional cluster of donor-derived LCM was discovered in Australia in 2008 when three solid organ recipients died of LCM (Table 1). The LCMV typed by genetic analyses in Australia was a variant previously undocumented. Australia had been reported clear of LCMV up until this discovery (Palacios et al., 2008). How most organ donors acquired their LCMV infection is undetermined. However, in 2005, LCM in four recipients (two died) was traced to transmission from an organ donor who kept golden hamsters as pets. These animals were purchased from a major supplier of hamsters. Traceback studies identified infected hamsters within the store from which the animal originated as well as the distribution and commercial breeding location.
How rare is organ transplant-associated LCM?
In 2017 there were 19,484 kidney, 8,082 liver, and 2,449 lung transplants performed in the USA—the solid organs responsible for LCMV infections resulting from infected donors (Table 1) (https://www.organdonor.gov/statistics-stories/statistics.html).
Risk of LCMV among organ recipients is low. As individual hospitals and organizations procure and provide donor organs, there are an unstandardized variety of tests performed on tissues. Recommendations for live donor pathogen screening by the Organ Procurement and Transplantation Network do not include testing for LCMV (Rosen and Ison, 2017).
These alarming occurrences further confirm that the once usual and originally described source of LCMV transmission to humans through contact with the introduced house mouse has been supplanted by contact with another non-native rodent species, the hamster.
Control of LCMV and Prevention of LCM
Against the usual suspect, the house mouse, the most effective methods to reduce the risk of human infection with LCMV are to employ traditional and, less rarely implemented but most effective, integrated pest management, to control and prevent transmission from the usual suspects. These combine rodent elimination through trapping and poisoning, sound construction procedures or remediation as needed, and reduced access to human-generated food resources through sanitary efforts (Childs and Wilson, 1994; Centers for Disease Control and Prevention, 2005), and are essentially the same measures for reducing SEOV exposure and disease (see sections below).
Controlling exposure to LCMV from laboratory animals and derived cell cultures requires barrier breeding, periodic testing of rodent colonies and cultures of derived tissues, prevention of wild mouse ingression and culling of any colony shown to be infected, as the role of wild mice in infecting laboratory colonies has been well-established for decades (Figure 2B) (see Skinner et al., 1977 for review) (https://www.criver.com/sites/default/files/resources/LymphocyticChoriomeningitisVirusTechnicalSheet.pdf).
In addition to testing samples derived from colonies of mice, sampling of rodent bedding has been suggested as a means of surveillance. However, in one significant case, testing of material failed to identify LCMV contamination of lab mice, which was revealed only after embryo transplants, which also demonstrated a failure of caesarian delivery to control spread (Ike et al., 2007).
Control of LCMV among hamsters requires radically different approaches when animals are destined for the pet trade. Studies have shown control to be problematic even when recommendations (sealing buildings from wild mice entry, regular veterinary inspections, monitoring for zoonotic infections, culling of animals, etc.) to reduce infection among breeding and distribution centers exist—although they are not always strictly adhered to or followed (Amman et al., 2007). As of 2018, the risk of human infection with LCMV among persons working in breeder facilities is still substantial. In one study, 32% (N = 97) of workers had antibodies to LCMV and LCM was diagnosed among four persons (Knust et al., 2014). Of over 1,800 mice tested, the prevalence of LCMV infection was >20% and RT-PCR identified currently infected mice. Complete culling of colonies was implemented.
Although a rare phenomenon, the problems associated with preventing LCMV transmission through solid organ transplant are formidable. The organizations providing organs have different operational standards for screening donors and none screen for LCMV infection (MacNeil et al., 2012). Additionally, screening is unlikely as it is not considered cost effective. New occurrences of transplant-associated LCM will continue to occur, although rarely (this also applies to other viruses shown to cause solid organ transplant-associated encephalitis, including West Nile virus and rabies virus).
Some suggestions for screening donors for history of contact with wild or pet rodents have been made, but given the backlog of waiting recipients and pressure to obtain organs, the effectiveness of such efforts will be difficult to assess (MacNeil et al., 2012). Screening of possibly rejected donors for LCMV infection would be a necessary component to such a measure, and in past investigations (Fischer et al., 2006; Fischer, 2008) most donors showed no evidence of acute LCMV infection and were only demonstrated to be infected after the fact.
LCM Conclusions
The original concept of the zoonotic cycle leading to human LCM was through contact with wild mice and inhalation of their aerosolized excreta/secreta. From the 1930s onward, it was well-recognized that LCMV was maintained among mice by vertical and horizontal transmission among mouse populations (Figure 2A). The first twist in this traditional cycle was when LCM outbreaks were linked to lab colonies of mice maintained in research institutes. Almost concurrently, it was shown that the whole mouse was not required for the maintenance of LCMV, but contact with cell cultures—particularly those of transplantable tumors—was sufficient to result in substantial outbreaks among scientists and other personnel working in research settings.
Within a few years, LCMV infection derived from lab and pet hamsters dwarfed the number of traditional recognized infections acquired from wild mice. The hamster, once not a usual suspect, supplanted the mouse as the major link to human infection and disease—transforming itself into “the usual suspect.” Through natural maintenance among wild mice, the ongoing risk of laboratory infections, LCMV's association with hamsters—laboratory and pet—and the ongoing phenomenon of human-to-human transmission, the public health significance of LCM has an assured future.
Seoul virus (SEOV) in Rattus norvegicus
There are numerous parallels in the history of Seoul virus (SEOV) recognition and discovery of host and disease consequences with that described for LCMV. The disease caused by SEOV is similar to, but less severe than and more rarely identified as, that caused by other members of the genus Hantavirus. The characterization of hantaviral disease as a unique clinical syndrome across Eurasia preceded the identification and culture of the agent by several decades.
The earliest modern clinical descriptions of hantaviral disease were from the undeclared Soviet-Japanese border conflict (Battles of Khalkhyn Gol) in 1939 (Ishii, 1942; Kitano, 1944a,b; Smorodintsev et al., 1944, 1959) (Figure 3). Epidemiologic investigations of outbreaks among combatants led both sides to conclude that a zoonotic virus was responsible. Although neither group isolated the agent, the Soviets identified the likely reservoir as a species of the genus Myodes, while the Japanese indicated that Apodemus was the likely source (Smorodintsev et al., 1959; Traub and Wisseman, 1978). The “rediscovery” of the syndrome occurred during the United Nations action in Korea during the early 1950s, when a disease with a matching presentation was reported among several thousand troops (Traub and Wisseman, 1978). As in prior studies, epidemiologic investigations suggested a rodent-borne zoonotic virus as the likely cause of what was referred to as “Korean hemorrhagic fever” (KHF). The means of virus transmission were not known at this time and some investigations centered around identifying a possible arthropod vector.
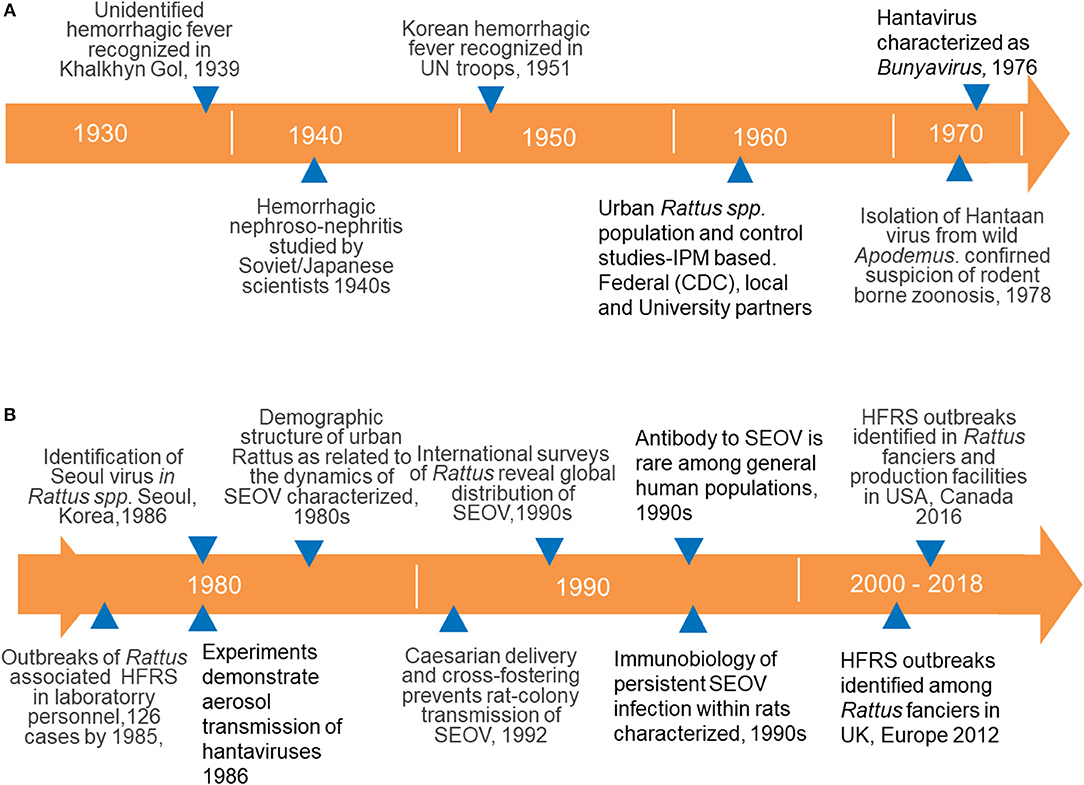
Figure 3. (A) Time lines documenting the history of major events in our understanding of Old World hantaviruses causing HFRS, focusing on SEOV isolated from Rattus spp.; from the discovery of a new clinical entity (HFRS) to the identification of virus maintenance among rodents and the taxonomic designation of hantaviruses with the family Bunyaviridae; (B) details of our understanding of the epidemiology, maintenance, immunobiology, and transmission of SEOV to humans and the increase in recognized SEOV cases associated with ownership of pet rats.
Roughly two decades later, Lee and colleagues (Lee et al., 1978) demonstrated viral antigen in the lungs of Apodemus agrarius using convalescent-stage human sera in an indirect immunofluorescent antibody assay (IFA). The subsequently isolated virus was named Hantaan virus after a river in South Korea, in keeping with place designations for proximate locations where zoonotic and arboviruses are first isolated.
Shortly after Lee's development of serological tests he identified a case of disease in an urban maintenance worker whose history was notable for having killed a rat (genus Rattus). Apodemus spp. are largely absent from most city environments and Lee, and colleagues focused on R. norvegicus and the black rat (Rattus rattus) and isolated a second Hantavirus, SEOV, from these species (Lee et al., 1982). This led to international concern that SEOV might have become or would become globally disseminated along with the introduced and cosmopolitan synanthropic Rattus spp. Studies to address these possibilities revealed the worst-case scenario; Norway rats infected with SEOV were found on nearly every continent (LeDuc et al., 1986). Spillover infections to other rodents were not observed and the genus Rattus was presumed to be the reservoir host (Korch et al., 1989). Hereafter we mainly focus on the Norway rat's role in the maintenance and transmission of SEOV.
Characteristics of Hantaviruses
SEOV is a negative-sense single-stranded RNA virus that is tri-segmented (McAllister and Jonsson, 2014). The three gene segments are identified as L, M, and S. The S segment codes for the nucleocapsid protein, while the M segment codes for two glycoproteins (Gn and Gc). The L segment encodes the RNA-dependent RNA polymerase (McAllister and Jonsson, 2014). Hantaan virus represents the type species of the family Bunyaviridae while SEOV is likely among the most widely distributed member of the group. For an extended period of time, hantaviruses were presumed restricted to three major rodent groups. The exception was the description of amplification of Thottapalayam virus using RT-PCR against a targeted region in the S-segment of other hantaviruses (Arthur et al., 1992). The virus was isolated from the Asian house shrew (Suncus murinus)—an insectivore. More recently, identification of other hantaviruses from additional insectivore species and bats suggests a more complex and diverse characterization of this group (Klempa, 2018).
Human Infection and Disease
SEOV infection in humans presents as hemorrhagic nephroso-nephritis or hemorrhagic fever with renal syndrome (HFRS; Smorodintsev et al., 1944). As with the other Old World hantaviruses, the name emphasized the hemorrhagic and renal aspects of infection, rather than the cardiac and pulmonary components of New World viruses, described in the early 1990s with the discovery of Sin Nombre virus (SNV) in the Southwestern United States (Nichol et al., 1993).
The course of severe HFRS has been divided into four stages; a prodromal with non-specific characteristics of fever, aches, chills, and often accompanied by headache; a hypotensive phase marked by thrombocytopenia and hypoxemia occurring 3–7 days after initial onset; a hypertensive stage with renal dysregulation and pronounced oliguria or anuria; and a convalescence phase with spontaneous return of renal function, often associated with polyuria and hypotension. Survivors often experience a protracted convalescence of weeks/months. Most deaths occur during phase three (Smadel, 1953; Traub and Wisseman, 1978).
Diagnosis of SEOV
Human immune responses to infection are marked (as with rats) with IgM and IgG antibody responses usually beginning 1–2 weeks after infection (Lee and Johnson, 1982). The presence of both IgM and IgG antibodies or a four-fold rise in IgG antibody titers are considered diagnostic of acute infection (LeDuc et al., 1990; Mattar et al., 2015). Detectable IgG persists for extended times (Mattar et al., 2015) so that IgG in the absence of IgM is considered evidence of previous infection.
Many of the early efforts for discovery and diagnosis of hantaviral infections used thin sections of fixed infected tissues (often lung) obtained from naturally infected wild rodents or from intentionally infected lab animals as the antigen for screening sera for antibodies. Application of a fluorescent-tagged secondary antibody resulted in a highly specific and sensitive test—the indirect immunofluorescent antibody assay, or IFA. These methods were used to diagnose human infections and to document seroconversion and antibody dynamics in experimentally infected rats and other species (Lee et al., 1978, 1982). With the successful isolation of Hantaan virus and its adaptation to tissue culture, infected Vero E-6 cells fixed to slides became the standard substrate for IFA tests (Lee et al., 1978). Later enzyme-linked immunosorbent assays (ELISA) and Western blots (and variants) were developed and used for screening and identifying important immunologically active proteins (Schmaljohn et al., 1990).
However, plaque reduction neutralization tests (PRNT) are considered the gold standard of serological tests as they measure the function rather than simply the binding of antibodies. PRNT also allows differentiation of various hantaviruses potentially causing the infection through comparison of end-point titers.
In the 1990s, reverse transcriptase polymerase chain reaction (RT-PCR) was first applied to the S segment of hantaviruses and demonstrated the utility of the technique for virus detection and differentiation by amplicon size (Arthur et al., 1992). This development allowed direct identification of SEOV and other hantaviral RNA in tissues. With the advent of sequencing technology with RT-PCR, it was demonstrated that amplicon sequences varied sufficiently among humans and rodent reservoir hosts that fine scale heterogeneities could be used to determine geographic sites of infection. This ability was dramatically highlighted by studies elucidating the cause and epidemiology of hantavirus pulmonary syndrome (HPS) caused by Peromyscus-associated SNV in the Southwestern United States in 1993 (Nichol et al., 1993).
Epidemiology of SEOV Associated with Wild Rats
In humans, infection with SEOV reflects the extent to which individuals contact infectious rats, although transmission from wild rats is rare. This includes urban locations where high-density populations of humans living in low SES neighborhoods, lacking amenities such as routine garbage removal (Figures 1A,C,D), are frequently exposed to rats and the prevalence of SEOV infection among adult animals often exceeds 50% (see section below). As with other hantaviruses, risk of exposure and infection is defined by the characteristics of the human population, the environment features conducive to supporting rat populations and interactions capturing them in time and space. The usual route of SEOV transmission from wild rats to humans is presumed by aerosols of dried excreta/secreta.
Researchers speculate on drivers contributing to the rarity of diagnosed cases, but few studies have attempted to untangle the many possible factors. Although biological and/or environmental barriers presumably preclude ready transmission and infection of humans with SEOV, physician awareness of this rare disease is negligible and public health surveillance nonexistent. Acute illness caused by SEOV, like LCMV, does not have a unique presentation and signs and symptoms are consistent with a wide range of diseases (Glass et al., 1994). As with LCM, specialized testing laboratories are unavailable at most point-of-entry hospitals and ascribing etiologic cause to agents like these is a rarity.
The rarity of infection is exemplified by a serosurvey of more than 1,100 individuals visiting a STD clinic in inner city Baltimore, Maryland, United States, where only four individuals had antibodies to SEOV (diagnosed by IFA and confirmed by PRNT; Figure 1D; Childs and Glass, 1988; Childs et al., 1988a, 1991). During interviews, all four individuals (prior to knowing their exposure status) volunteered that part of their daily home maintenance was to sweep rat feces from the paved areas of their properties. Cleaning activities involving sweeping up rodent feces are a common risk factor for acquiring hantaviral infection, presumably due to large or small particle aerosols.
When focusing longitudinal serosurveys of special populations exhibiting signs suggestive of possible current or past SEOV infection (e.g., renal dysfunction measured by high levels of proteinuria or requiring dialysis), three acutely infected individuals were identified based on a four-fold rise in PRNT titers. Two of the three had primary symptoms consistent with HFRS, including striking renal insufficiency and shock (Glass et al., 1994). This suggests there is some background level of ongoing transmission by the usual routes to human populations from wild rats even in the absence of recognized threat.
SEOV Prevalence, Maintenance, and Transmission among Norway Rats
Surveys for evidence of SEOV in wild Norway rats show substantial variation in prevalence. Even in the earliest studies (LeDuc et al., 1986), there was striking geographic variation. These surveys showed prevalence ranging from single digits, in various African cities, to 20–40% in Asian and Australian cities. In North America, most urban areas showed prevalence between these ranges, except for cities in California (United States), where infection was not reported, and Baltimore, where it reached the global extreme. This led to a series of studies in that city to better characterize the maintenance of the virus in the rodent host and suggested additional reasons why prevalence could appear to vary widely in wild rat populations.
One of the first observations was the non-monotonic change in antibody prevalence with age (size) in the rats. An initially high, but rapidly decreasing, prevalence of anti-SEOV antibody among the smallest wild-caught Norway rats was identified as due to maternal antibody (Figure 4). As maternally-derived antibodies waned, reaching a nadir just prior to the onset of sexual maturity, juvenile and sub-adult rats (based on sexual maturity and body mass determinations) were infected by SEOV through horizontal transmission routes (detailed below), producing a characteristic “J-shaped” distribution from cross-sectional sampling (Figure 3; Childs et al., 1987a, 1988b).
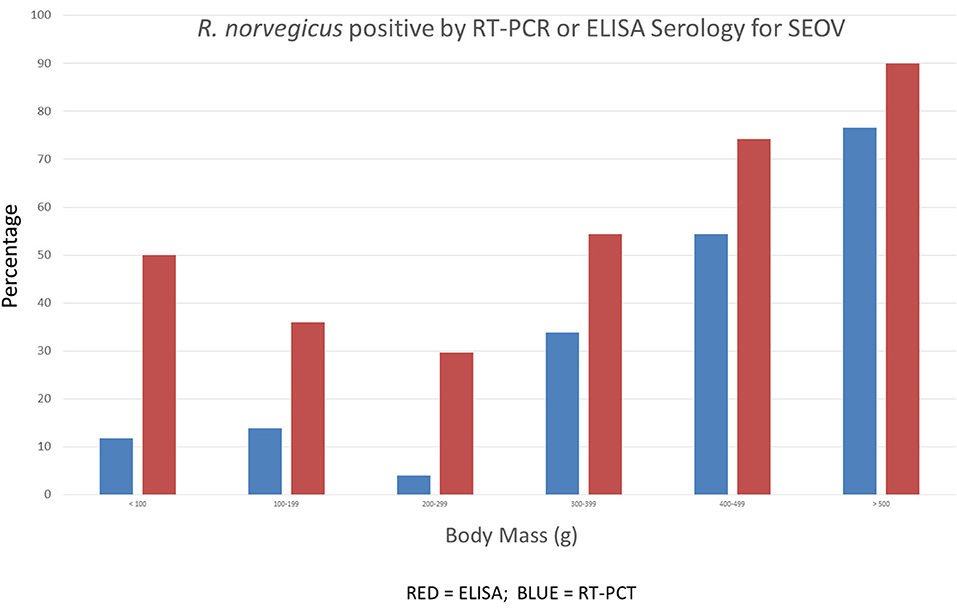
Figure 4. Cross-sectional patterns of antibody and RT-PCR prevalence to SEOV among 485 wild Norway rats sampled from Baltimore, MD. The prevalence of antibody titers and RT-PCR prevalence decline among juvenile rats before rising steadily among adult rats (details are discussed in the text).
This phenomenon of maternal antibody-derived protection of neonates challenged with SEOV is reproducible in lab-reared rats, where immunity lasts for 4–6 weeks (Zhang et al., 1988). An additional finding corroborating the protective effects of maternally-derived IgG is the near absence of viral RNA detected by RT-PCR in rats (Figure 4). In larger/older rats, the prevalence of infection, as measured both by viral RNA detected by RT-PCR and serology, can increase until most rats show evidence of infection—~80% in Baltimore, which appears to be a hyper-endemic area (LeDuc et al., 1986; Childs et al., 1987b; Glass et al., 1988).
SEOV infection in rats results in persistent infection, even in the presence of high levels of IFA and neutralizing antibodies. Initial isolation efforts are most often successful by targeting individuals with high antibody titers (Childs et al., 1987b) as opposed to LCMV, where horizontally infected mice clear the virus and seroconvert (see LCMV sections above). SEOV infection among wild rats causes no obvious overt disease and survivorship, fecundity, and fertility are unaffected among wild R. norvegicus (Lee et al., 1986; Childs et al., 1989).
In experimental systems, however, neonates born to uninfected dams and then infected with SEOV prior to seven days of age develop a progressive severe clinical course characterized by weight loss, ruffled fur, ataxia, limb paralysis, convulsions, and death, most often within 35 days (Zhang et al., 1988). These observations stand in stark contrast to the protective effect of maternal antibody in protecting neonates from pathogenic effects (Zhang et al., 1988; Dohmae and Nishimune, 1995).
Immunobiology of SEOV Persistence in Norway Rats
The existing dogma states that hantaviral infection alone did not cause overt disease in rats (or other reservoir hosts for different hantaviruses) except under experimental conditions described above (Yanagihara et al., 1985; Lee et al., 1986; Hutchinson et al., 1998; Botten et al., 2000; Wahl-Jensen et al., 2007; Eyzaguirre et al., 2008). How rats and other rodents infected with hantaviruses avoid the deleterious effects are not well understood. Recently, advances have been made in elucidating the basis for the persistence of SEOV within rats in the face of robust humoral immune responses.
Persistence is driven by the upregulation of regulatory responses and downregulation of proinflammatory responses. SEOV preferentially replicates in rat lungs (Khaiboullina and St. Jeor, 2002), where the virus is found both in alveolar macrophages (AMs) and endothelial cells. This may polarize CD4+ T cell differentiation toward a regulatory T (Treg) cell phenotype. In vitro, viral infection increases immunological tolerance by promoting transforming growth factor beta (Tgfβ) mRNA in AMs and programmed cell death 1 ligand 1 (PD-L1) in lung microvascular endothelial cells (LMVECs). SEOV-infected LMVECs, but not AMs, induce increased expression of Foxp3 expression (the transcriptional factor in Treg cells) and Treg cell frequency in allogeneic CD4+ T cells (Li and Klein, 2012). In vivo, elevated regulatory responses include TGF-β1 and numbers of CD4+CD25+FoxP3+ Treg cells in the lungs. This is associated with persistence of SEOV (Easterbrook et al., 2007a; Schountz et al., 2007; Easterbrook and Klein, 2008a).
In addition to the regulatory responses, activity along the type I interferon (IFN) pathway and proinflammatory cytokines (e.g., IL-1β, IL-6, TNF-α, and IFN-γ) remain at or below baseline throughout SEOV infection in the lungs of male, but not female, Norway rats (Klein et al., 2004a; Easterbrook and Klein, 2008a; Hannah et al., 2008). Conversely, in the spleen, proinflammatory, and antiviral responses are elevated during acute SEOV infection in both sexes (Easterbrook and Klein, 2008a). Infection of bone marrow-derived macrophages (BMDMs) with SEOV suppresses NF-κB-mediated inflammatory responses, including TNF-α, IL-6, and IL-10, and surface marker expression (i.e., MHCII, CD80, and CD86), suggesting SEOV infection suppresses the innate immune response in antigen-presenting cells (Au et al., 2010).
These pathways suggest a reason for the observed sex difference in infection prevalence, with males having a higher likelihood of infection than females (Glass et al., 1998; Bernshtein et al., 1999; Hinson et al., 2004; Klein et al., 2004b); (Douglass et al., 2007). Males have more SEOV RNA and antigen in target organs and saliva (i.e., vehicle for virus shedding) than do females (Klein et al., 2001; Hannah et al., 2008). In the laboratory, removal of the testes in males to reduce androgen levels also reduces SEOV loads, whereas removal of the ovaries in females increases SEOV RNA loads (Hannah et al., 2008). The expression of innate antiviral (e.g., Tlr7, Myd88, Rig-I, Visa, Ifnβ, and Mx2) and proinflammatory (e.g., Tnfα and Ccl5) factors is higher in the lungs of gonad intact females rats than in males (Klein et al., 2002, 2004a; Easterbrook and Klein, 2008b; Hannah et al., 2008), suggesting that this contributes to reduced viral loads in females compared to males. Conversely, the expression and production of regulatory factors, including Foxp3 and TGF-β, are elevated in the lungs of males compared to females (Easterbrook and Klein, 2008b). This suggests that sex differences in immune responses during hantavirus infection may be driven by estradiol in females and testosterone in males, as gonadectomy reverses these differences (Klein et al., 2004a; Hannah et al., 2008). It is plausible that reduced innate and proinflammatory defenses and elevated regulatory responses combined with an increased propensity to engage in aggression may contribute to increased maintenance and transmission of hantaviruses among male as opposed to among female rodents.
The Usual and Unusual Routes of Human Exposure to SEOV
Human exposure to SEOV requires contact with infectious materials from R. norvegicus. As the virus is excreted in feces, urine and secreted in saliva (Klein et al., 2000), direct contact with the animal itself is not needed. The first documentation of hantavirus transmission by the indirect aerosol route was described among Soviet laboratory workers, facility cleaners and secretaries who were infected, often without any near contact with a colony of A. agrarius (Nuzum et al., 1988).
The low prevalence of antibodies among urban residents with putatively high levels of exposure to infected rats indicates there is a significant barrier to cross-species transmission. Whether this barrier(s) is driven by cultural, social, biological, or environmental factors (or more likely a combination of these) is not known.
Enter Laboratory and Pet Rats
A twist in the risk of SEOV exposure involved a category of interactions with R. norvegicus where the intent of humans is to interact with the animals themselves (Figure 3B). As with LCMV, these at-risk groups are exposed to potentially infectious animals through occupation (research settings) or avocation (pet owners). These interactions with rats are outside the realm of usual interactions with wild Norway rats.
The risk of human exposure and infection among laboratory researchers and individuals working in breeding facilities with Norway rats was foreshadowed by the outbreaks among research labs housing Apodemus spp. (detailed above). HFRS outbreaks associated with SEOV first occurred among personnel working with lab rats in Korea and Japan (of note, these occurrences also provided some additional evidence that an arthropod vector was not necessary for SEOV transmission) (Umenai et al., 1979; Lee and Johnson, 1982; Kawamata et al., 1987). Subsequent outbreaks of SEOV-associated disease among laboratory staff in Europe (Desmyter et al., 1983; Lloyd et al., 1984) confirmed that unapparent infections among laboratory stocks of Rattus were not isolated events and posed a significant health threat, and remedial actions were instituted. One important step to prevent maintenance of SEOV in the laboratory was to adopt caesarian delivery and cross-fostering of pups to known uninfected dams (Figure 3B) (McKenna et al., 1992).
In parallel with the unfolding history of LCMV, SEOV was also identified in hybridomas of rat tissues in research laboratories (Figures 2A, 3A). Once again, it became apparent that the intact reservoir rodent was not required for viral persistence. Upon retrospective analyses, cell lines were shown to remain persistently infected in excess of 8–10 years, indicating the past and ongoing health risk to laboratory personnel and the specter of contamination of other cell lines, as occurred when LCMV was present in cell cultures producing rabies vaccine (see LCMV sections above) (Lloyd and Jones, 1986). Once serological diagnostic tests became commonplace, extensive infection among cell lines was eliminated by destroying contaminated cell lines and culling infected rats in research and commercial settings to reduce recurrent reinfection.
Although contamination and productive infection by SEOV, LCMV and other zoonotic viruses (e.g., SV40) is now a well characterized and largely controllable phenomenon among lab animals and cell lines, the next twist to SEOV transmission to humans was unanticipated and posed new challenges to control. The first reports that owners and breeders of fancy rats were infected were published in 2013 from Europe and several localized outbreaks were identified (Figure 3B) (Jameson et al., 2013; Lundkvist et al., 2013; McElhinney et al., 2017; Reynes et al., 2017). Similar findings from the USA followed (Figure 3B).
The association between pet rats and human SEOV was only recognized by the occurrence of HFRS in humans rather than by any screening of rats destined for the pet trade. Serological and virological surveys during investigations of human cases found levels of SEOV to be between 80 and 100% among breeding colonies—a figure higher than the prevalence found among wild rat populations! As these colonies were identified after the human cases this does not, presumably, reflect the typical prevalence among breeding colonies more generally. However, the extent of human infection dwarfed the previous number of acute SEOV cases resulting from exposures to wild or laboratory animals. Surveys of more than 800 people involved in Rattus work, either professionally or for avocation, found evidence of SEOV infection in more than a third of this population (Duggan et al., 2017). The highest prevalence was among persons maintaining rats as pets, with substantially lower rates in those involved with professional activities in laboratories. Considering that the prevalence of SEOV antibodies is <0.5% among populations of urban residents living in conditions where dense populations of Norway rats exist and where a large proportion are infected by SEOV (Figures 1C,D), the results reported from surveys of rat owners were staggering. When it was considered as dogma to accept that SEOV transmission to humans was rare, these results indicated that in the right settings, transmission was actually common.
Most recently, a sizable outbreak of SEOV was identified among pet rat owners in North America (Kerins et al., 2018). The disease was identified in two individuals and follow-up studies showed that more than 10% of people tested were antibody-positive to SEOV. Investigations of more than 100 facilities found evidence of infection either in people or Rattus at more than 30% of sites. These findings—shifting attention from the usual (albeit rare) epidemiologic cycle of SEOV transmission from wild rat to human, or from the more unusual laboratory rat or cell line to human—demonstrated that ownership and interactions with pet rats changed our whole notion about risks for infection.
Two major findings emerged from these outbreaks among pet owners. Of public health concern was the number of SEOV cases and severity of disease among rat owners. The numbers of humans involved eclipsed the few sporadic cases of disease/infection reported among the general public interacting with the wild reservoir of SEOV—an eerie parallel to the emergence of LCMV among owners of hamsters. Secondly, the introduction of SEOV into rat breeding colonies, aimed for the fancy rat admirer or simply persons wanting a plain rat pet, was geographically extensive and was likely to be found beyond Europe and North America. An immediate consequence of these reports was that no adequate surveillance and control policies were in place to protect the public, as breeding of rats destined for the pet trade does not require a dedicated facility but can be a household or cottage industry; control will be challenging and incomplete at best. This represents déjà vu for persons previously following LCMV's peregrinations.
The likely reason for these high rates of infection among rat fanciers has been explored in Europe (Robin et al., 2017). In semi-structured surveys it was concluded that owners re-framed the perception of their rats to “pets” away from the perspective of synanthropic wild animals, so that the fancy rat became qualitatively distinct and was presumed “clean”—in essence a “new” species divorced from pestilential baggage carried by the wild ancestor. In the absence of overt clinical disease in the pet rat and lack of awareness of how persistent SEOV infection can perpetuate infection from generation to generation, there was simply a misperception among owners of the potential risk of rat-to-human transmission. This was one proposed reason for why levels of SEOV infection among owners of fancy rats were elevated nearly 20-fold above that of commercial breeders (Duggan et al., 2017).
Controlling SEOV Transmission
The traditional approach to reduce SEOV exposure and transmission from wild Norway rats involves rat population control in urban areas. Population reduction is achievable but eradication of rats even from intensely targeted locations is rarely achieved, and populations typically rebound over months even when reductions may exceed 50% (Gardner-Santana et al., 2009; Glass et al., 2016). Control efforts have typically relied heavily on the application of rodenticide, and still do in most urban settings, but the implementation of integrative pest management (IPM) has achieved longer dampening of populations as they can alter the carrying capacity of targeted locations (Keiner, 2005).
Early attempts to mitigate populations through IPM involved both ecological and environmental interventions to reduce carrying capacity by reducing food and shelter resources, with complementary population reduction by trapping or chemical means (see Davis, 1953). The IPM approach was embraced by CDC initiatives in the 1970s directed at improving conditions to reduce rat infestation levels through a block-by-block intervention protocol carried out within targeted cities (including Baltimore), which was effective but not sustainable (Centers for Disease Control and Prevention, 1981, 1982a,b).
As a rare case in point, Baltimore reinstated these protocols in the mid-1990s in response to rat infestations in several city locations that proved resistant to rodenticide applications. A combination of targeted rodenticide-baiting, trash collection, public education, reduction in nesting sites and other habitat modifications were applied in two neighborhoods of ~1,700 households (Lambpropolous et al., 1999). One of the neighborhoods was heavily infested (21% households with rats in adjoining alleys or within homes), while in the other rat infestation was only 4%. After a 3-month intervention, infestations had been reduced to 8 and 0.3%, respectively. At 6 months post-intervention, infestation levels in the heavily infested neighborhood had returned to 20% while rat populations were undetectable in the less intensively infested area. Based on the limited sample size, the conclusion was that there may be some level of infestation above which ongoing IPM control efforts will fail to solve effectively in the long term.
The cause of this IPM failure was the basis for further studies. As observed repeatedly (Abelkrim et al., 2005; Gardner-Santana et al., 2009; Combs et al., 2018), Norway rats show highly structured populations with substantial population/genetic isolation by distance over hundreds of meters. Examination of maternity/paternity dyads in wild rats showed, however, distinct mate-seeking behavior compared to normal movements. While most non-sexual activities among individual rats occur within 100 m (Glass et al., 1989, 2016), mates often came from more distant alleys (likely beyond the geographic range of the IPM studies), essentially expanding the potential pool of mates and buffering local populations from perturbations.
Consequently, new strategies are needed to target interconnected populations. Population genetic studies that trace and link rat-infested locations by determining levels of gene flow should help to inform intervention efforts by defining and targeting an Eradication Unit rather than relying on block-by-block or simple community-based control (Robertson and Gemmell, 2004).
Although the outcomes of single or limited interventions to control targeted rat populations may be unconvincing, the conclusion that sustained IPM strategies are critical is unchanged. We have no other actionable options that simultaneously address human and rat behavior at the community level, mitigate environmental conditions that provide key resources for rat populations and improve sanitation (garbage and major refuse removal) measures provided by local government agencies. However, examples of long-term community and political commitment to sustain efforts are few and rarely achieved (Childs and Glass, 1988; Lambpropolous et al., 1999).
Reduction of SEOV infection of lab colonies of rats has been achieved in part by placing the burden of screening and culling on commercial operations maintaining breeding stock. However, ongoing surveillance of such colonies is required as ongoing reintroduction of SEOV (and other pathogens) from wild rats remains a threat (Easterbrook et al., 2007b; Reynes et al., 2017).
Individuals who place themselves at risk of SEOV infection by intentionally adopting rats as pets pose a unique and challenging group for intervention. As noted by Robin et al. (2017), this group has a perception that their domesticated rats pose little or no risk. Prevention for this group is more direct but has practical challenges influenced by the rat–virus interactions that produce persistent, unapparent infections in the reservoir. Historically, most pest control strategies focused on the inadvertent exposure population rather than this group. In the absence of routinely scheduled diagnostic testing of breeding colonies, which range from commercial enterprises to cottage industries, the lack of apparent disease among pet rats will permit persistent infection among closely housed individuals and across generations.
Summary and Conclusions
LCMV and SEOV are rodent-borne zoonotic pathogens that enjoy a global or near global distribution. The murid rodents, M. musculus and R. norvegicus, were introduced with ship-borne commerce and colonization and are the primary reservoir hosts for LCMV and SEOV, respectively.
The original concept of the zoonotic cycle linking transmission of LCMV and SEOV to humans through contact with their wild rodent hosts was established within a year after the discovery of LCMV as a cause of aseptic meningitis but required several decades to definitively link wild rodents as the source of viruses causing HFRS among humans (Figures 2, 3). A few years after the isolation of HTNV, the closely related SEOV was isolated from urban Rattus spp. and was shown to be responsible for HFRS among residents of Seoul, Korea. In this way, the natural or usual zoonotic transmission cycle was established linking human disease to wild rodent contact or proximity.
Within years, subsequent reports of laboratory-acquired infections caused by both viruses indicated that breeding stocks of rodents were contaminated. Patterns of infection and disease implicated aerosol transmission of LCMV and SEOV shed within rodent secreta/excreta, indicating that physical contact with the reservoir host was not required, adding an important element into the simple zoonotic cycle. It was also the first indication that simple zoonoses of wild animals had slipped unrecognized into human enterprises on a broader scale than had been previously appreciated. The realization that breeding stocks of rodents were infected with both LCMV and SEOV and were the source of human disease was not anticipated and raised questions about health security among lab personnel, commercial breeders and, importantly, the reliability of scientific studies using potentially infected rodents as the basis for experimental outcomes.
Almost concurrently it was shown that the whole mouse or rat was not required for the maintenance and transmission of LCMV or SEOV, but contact with virus-contaminated cell cultures, particularly those of transplantable tumors or hybridomas, was sufficient to produce substantial outbreaks among scientists and other personnel working in research settings. The usual and traditional zoonotic cycle of virus maintenance among wild rodents and transmission to humans via aerosolization of, or contact with, infectious secreta/excreta was far too simple. The transmission from inadvertently infected conspecific laboratory animals was deemed unusual but understandable, but infection from cell cultures, often repeatedly passed, was out of the ordinary and an unusual twist to be added within the expanding concept of the zoonotic cycle. Of particular note, outbreaks of LCMV- and SEOV-related infection/disease were more extensive than reported in natural settings, where only the simplest cycle existed.
Within years of the discovery of lab mice and mouse-derived cell cultures as the source of human disease, a nearly identical pattern of discoveries unfolded—but in these instances the source of LCMV was from hamsters (most notably the golden hamster), a different rodent species in a taxonomically removed family. In short order, infections were being diagnosed within research settings and among residents keeping golden hamsters as pets. Outbreaks caused by LCMV among hamster owners exceeded by several orders of magnitude the total number of acute cases ascribed to contact with wild house mice—the original and only species in the initial scheme of the zoonotic cycle. Not only had a distantly related rodent become involved in what had become the most “routine” transmission of LCMV to humans, its public health significance as the most important intermediary in the zoonotic cycle of transmission to humans established this species as the new reigning reservoir host. This unusual twist completely rearranged our thinking on zoonotic cycling of LCMV.
In a manner that partially mirrors events driving our new understanding of LCMV epidemiology, with SEOV, which rarely causes clinically diagnosed infection among the general population that has high levels of exposures to infected wild Norway rat populations, there has emerged disease outbreaks among persons owning fancy rats as pets. Like owning pet hamsters, these are inadvertent but intentional induced exposures from animals assumed to be virus free. Controlling these unusual routes of transmission are daunting and requires efforts entirely different from routine IPM measures aimed at controlling wild rodent populations. In both LCMV and SEOV, the immunobiology of rodent–virus interactions produces occult infections that require substantial diagnostic efforts to eliminate the threat.
At this juncture, the different, unusual, and unpredicted routes of LCMV transmission diverge from that of SEOV. Beginning with the demonstration that human-to-human transmission from acutely infected women during pregnancy resulted in severe, often fatal consequences to the fetus and permanent sequelae among surviving offspring (Figure 2B), a new twist, again unanticipated, was identified. Human-to-human transmission of LCMV to solid organ recipients caused severe, and most often fatal, disease (Table 1). In some cases, infection among donors was linked to ownership of pet hamsters while in most cases the source of infection remained undetermined. Traceback studies showed that infected breeding stocks distributed to wholesale suppliers were the source of infection to pet owners. Of note, these rare occurrences highlighted how often LCMV infection goes unsuspected and undiagnosed.
The evolution of transmission routes for human infection by LCMV and SEOV have unfolded the highly complex interactions, deviating dramatically from the usual or classically defined zoonotic cycle of one reservoir species transmitting these viruses directly or indirectly to humans. A bewildering transmission web has evolved, linking the interaction of multiple variables to human infection, as humans have found new ways to interact with these rodents.
One fact is the maintenance of both viruses within wild rodents will continue to provide renewed sources for virus spillover to other rodent hosts owned as pets. These viruses have surprised us on numerous occasions, and whether no new string will be added to the complex web of transmission is uncertain. However, the epidemiology of these viruses' urban infections promotes that possibility. The global percentage of humans living in cities has surpassed 50% and continues to grow. Inevitably, many residents of burgeoning cities will live in conditions highly conducive to significant human–rodent interactions and these will be locations for examining increased incidence/prevalence of human infection and disease. Whatever twists remain, these viruses are here to stay and their significance in causing substantial public health challenges, perhaps limited today, is likely to increase.
Author Contributions
All authors listed have made a substantial, direct and intellectual contribution to the work, and approved it for publication.
Funding
This work was supported by U.S. federal agencies, including the U.S. Department of Defense, the U.S. National Institutes of Health, and the U.S. National Science Foundation supported much of the field and laboratory research discussed in this overview during the time interval 1984–2014.
Conflict of Interest Statement
The authors declare that the research was conducted in the absence of any commercial or financial relationships that could be construed as a potential conflict of interest.
References
Abelkrim, J., Pascal, M., Calmet, C., and Samadi, S. (2005). Importance of assessing population genetic structure before eradication of invasive species: examples from insular Norway rat populations. Conserv. Biol. 19, 1509–1518. doi: 10.1111/j.1523-1739.2005.00206.x
Ackermann, R., Bloedhorn, H., Küpper, B., Winkens, I., and Scheid, W. (1964). Über die verbreitung des virus der lymphocytären choriomeningitis unter den Mäusen in Westdeutschland. Zen. Bakter. Parasit. Infekt. Hyg. 194, 407–430.
Ackermann, R., Stille, W., Blumenthal, W., Helm, E. B., Keller, K., and Baldus, O. (1972). Syrische goldhamster als uberträger von lymphozytärer choriomeningitis. Deut. Med. Wochen. 97, 1725–1731. doi: 10.1055/s-0028-1107638
Adler, S. (1948). Origin of the golden hamster cricetus auratus as a laboratory animal. Nature 162:256. doi: 10.1038/162256b0
Ahmed, R., Hahn, C. S., Somasundaram, T., Villarete, L., Matloubian, M., and Strauss, J. H. (1991). Molecular basis of organ-specific selection of viral variants during chronic infection. J. Virol. 65, 4242–4247.
Albariño, C., Palacios, G., Khristova, G., Erickson, M. L., Carroll, B. R., Comer, S. A., et al. (2010). High diversity and ancient common ancestry of Lymphocytic Choriomeningitis virus. Emerg. Infect. Dis. 16, 1093–1100. doi: 10.3201/eid1607.091902
American Veterinary Medical Association (2012). U.S. Pet Ownership & Demographics Sourcebook. Schaumburg, IL.
Amman, B. R., Pavlin, B. I., Albarino, C. G., Comer, J. A., Erickson, B. R., Oliver, J. B., et al. (2007). Pet rodents and fatal lymphocytic choriomeningitis in transplant patients. Emerg. Infect. Dis. 13, 719–725. doi: 10.3201/eid1305.061269
Armstrong, C., and Sweet, L. K. (1939). Lymphocytic choriomeningitis. Public Health Rep. 54, 673–684. doi: 10.2307/4582864
Armstrong, C. L., and Lillie, R. D. (1934). Experimental Lymphocytic Choriomeningitis of monkeys and mice produced by a virus encountered in studies of the 1933 St. Louis Encephalitis Epidemic. Public Health Rep. 49, 1019–1020. doi: 10.2307/4581290
Armstrong, D. F., Fortner, J. G., Rowe, W. P., and Parker, J. C. (1969). Meningitis due to Lymphocytic Choriomeningitis virus endemic in a hamster colony. JAMA 209, 465–467. doi: 10.1001/jama.1969.03160150051019
Arthur, R. R., Lofts, R., Gomez, J., Glass, G. E., LeDuc, J. W., and Childs, J. E. (1992). Grouping of hantaviruses by S genome segment PCR and amplification of viral RNA from wild-caught rats. Am. J. Trop. Med. Hyg. 46, 410–424.
Asnis, D. S., Muana, O., Kim, D. G., Garcia, M., Rollin, P. E., and Slavinski, S. (2010). Lymphocytic Choriomeningitis virus meningitis, New York, NY, USA, 2009. Emerg. Infect. Dis. 16, 328–330. doi: 10.3201/eid1602.091347
Au, R. Y., Jedlicka, A. E., Li, W., Pekosz, A., and Klein, S. L. (2010). Seoul virus suppresses NF-kappaB-mediated inflammatory responses of antigen presenting cells from Norway rats. Virology 400, 115–127 doi: 10.1016/j.virol.2010.01.027
Barber, D. L., Wherry, E. J., Masopust, D., Zhu, B., Allison, J. P., and Sharpe, A. H. (2006). Restoring function in exhausted CD8 T cells during chronic viral infection. Nature 439, 682–687. doi: 10.1038/nature04444
Barton, L. L. (2010). Human infection with Lymphocytic Choriomeningitis virus. Emerg. Infect. Dis. 16, 1046–1047. doi: 10.3201/eid1606.100250
Barton, L. L., and Hyndman, N. J. (2000). Lymphocytic choriomeningitis virus: reemerging central nervous system pathogen. Pediatrics 105:E35. doi: 10.1542/peds.105.3.e35
Barton, L. L., Mets, M. B., and Beauchamp, C. L. (2002). Lymphocytic choriomeningitis virus: emerging fetal teratogen. Am. J. Obstet. Gynecol. 187, 1715–1716. doi: 10.1067/mob.2002.126297
Barton, L. L., Peters, C. J., and Ksiazek, T. G. (1995). Lymphocytic choriomeningitis virus: an unrecognized teratogenic pathogen. Emerg. Infect. Dis. 1, 152–153. doi: 10.3201/eid0104.950410
Becker, S. D., Bennett, M., Stewart, J. P., and Hurst, J. L. (2007). Serological survey of virus infection among wild house mice (Mus domesticus) in the UK. Lab. Anim. 41, 229–238. doi: 10.1258/002367707780378203
Benda, R. (1964). Passive immunoprophylaxis and immunotherapy of inhalation lymphocytic choriomeningitis in guinea-pigs. J. Hyg. Epidemiol. Microbiol. Immunol. 8, 243–251.
Bernshtein, A. D., Apekina, N. S., Mikhailova, T. V., Myasnikov, Y. A., Khlyap, L. A., Korotkov, Y. S., et al. (1999). Dynamics of Puumala hantavirus infection in naturally infected bank voles (Clethrinomys glareolus). Arch. Virol. 144, 2415–2428. doi: 10.1007/s007050050654
Biggar, R. J., Douglas, R. G., and Hotchin, J. (1975a). Letter: Lymphocytic choriomeningitis associated with hamsters. Lancet 1, 856–857. doi: 10.1016/S0140-6736(75)93027-5
Biggar, R. J., Schmidt, T. J., and Woodall, J. P. (1977). Lymphocytic choriomeningitis in laboratory personnel exposed to hamsters inadvertently infected with LCM virus. J. Am. Vet. Med. Assoc. 171, 829–832.
Biggar, R. J., Woodall, J. P., Walter, P. D., and Haughie, G. E. (1975b). Lymphocytic choriomeningitis outbreak associated with pet hamsters: fifty-seven cases from New York state. JAMA 232, 494–500. doi: 10.1001/jama.1975.03250050016009
Bishop, D. H., and Auperin, D. D. (1987). Arenavirus gene structure and organization. Curr. Top. Microbiol. Immunol. 133, 5–17. doi: 10.1007/978-3-642-71683-6_2
Bonthius, D. J., and Perlman, S. (2007). Congenital viral infections of the brain: lessons learned from lymphocytic choriomeningitis virus in the neonatal rat. PLoS Pathog. 3:e149. doi: 10.1371/journal.ppat.0030149
Botten, J., Mirowsky, K., Kusewitt, D., Bharadwaj, M., Yee, J., Ricci, R., et al. (2000). Experimental infection model for Sin Nombre hantavirus in the deer mouse (Peromyscus maniculatus). Proc. Natl. Acad. Sci. U.S.A. 97, 10578–10583. doi: 10.1073/pnas.180197197
Brooks, D. G., Trifilo, M. J., Edelmann, K. H., Teyton, L., McGavern, D. B., and Oldstone, M. B. (2006). Interleukin-10 determines viral clearance or persistence in vivo. Nat. Med. 12, 1301–1309. doi: 10.1038/nm1492
Buchmeier, M. J., Welsh, R. M., Dutko, F. J., and Oldstone, M. B. A. (1980). “The virology and immunobiology of Lymphocytic Choriomeningitis Virus infection,” in Advances in Immunology, eds F. J. Dixon and H. G. Kunkel (Boston, MA: Academic Press), 275–331.
Centers for Disease Control and Prevention (1981). Urban rat control–United States. MMWR Morb. Mortal. Wkly. Rep. 30:205.
Centers for Disease Control and Prevention (1982a). Urban rat control–United States, second quarter, fiscal year 1982. MMWR Morb. Mortal. Wkly Rep. 31:602.
Centers for Disease Control and Prevention (1982b). Urban rat control–United States. MMWR Morb. Mortal. Wkly. Rep. 31:259.
Centers for Disease Control and Prevention (2006). Survey of lymphocytic choriomeningitis virus diagnosis and testing–Connecticut, 2005. MMWR Morb. Mortal. Wkly. Rep. 55:398.
Centers for Disease Control and Prevention (2008). Brief report: lymphocytic choriomeningitis virus transmitted through solid organ transplantation–Massachusetts, 2008. MMWR Morb. Mortal. Wkly. 57, 799–801.
Centers for Disease Control and Prevention (2014). A cluster of lymphocytic choriomeningitis virus infections transmitted through organ transplantation-Iowa, 2013. MMWR Morb. Mortal. Wkly. 63:249.
Centers for Disease Control and Prevention (2005). Lymphocytic choriomeningitis virus infection in organ transplant recipients–Massachusetts, Rhode Island, 2005. MMWR Morb. Mortal. Wkly. Rep. 54, 537–539.
Charrel, R. N., and de Lamballerie, X. (2010). Zoonotic aspects of arenavirus infections. Vet. Microbiol. 140, 213–220. doi: 10.1016/j.vetmic.2009.08.027
Childs, J. E. (2004). Zoonotic viruses of wildlife: hither from yon. Arch. Virol. Suppl. 18, 1–11. doi: 10.1007/978-3-7091-0572-6_1
Childs, J. E., and Glass, G. E. (1988). Epidemiology of Hantavirus Infections in Baltimore. USMRDC Contract No. DAMD17-87-C-7101.
Childs, J. E., Glass, G. E., Korch, G. W., Arthur, R. R., Shah, K. V., LeDuc, J. W., et al. (1988a). Evidence of human infection with a rat associated Hantavirus in Baltimore, Maryland. Am. J. Epidemiol. 127, 875–878. doi: 10.1093/oxfordjournals.aje.a114871
Childs, J. E., Glass, G. E., Korch, G. W., Ksiazek, T. G., and LeDuc, J. W. (1992). Lymphocytic choriomeningitis virus infection and house mouse (Mus musculus) distribution in urban Baltimore. Am. J. Trop. Med. Hyg. 47, 27–34. doi: 10.4269/ajtmh.1992.47.27
Childs, J. E., Glass, G. E., Korch, G. W., and LeDuc, J. W. (1987a). Prospective seroepidemiology of hantaviruses and population dynamics of small mammal communities of Baltimore, Maryland. Am. J. Trop. Med. Hyg. 37, 648–662. doi: 10.4269/ajtmh.1987.37.648
Childs, J. E., Glass, G. E., Korch, G. W., and LeDuc, J. W. (1988b). The ecology and epizootiology of hantaviral infections in small mammal communities of Baltimore: a review and synthesis. Bull. Soc. Vector Ecol. 13, 1–9.
Childs, J. E., Glass, G. E., Ksiazek, T. G., Rossi, C. A., Barrera Oro, J. G., and LeDuc, J. W. (1991). Human-rodent contact and infection with lymphocytic choriomeningitis and Seoul viruses in an inner-city population of Baltimore. Am. J. Trop. Med. Hyg. 44:117121. doi: 10.4269/ajtmh.1991.44.117
Childs, J. E., Glass, G. E. G. W., Korch, G. W., and LeDuc, J. W. (1989). Effects of hantaviral infection on survival, growth and fertility in wild rat (Rattus norvegicus) populations of Baltimore, Maryland. J. Wldlf. Dis. 25, 469–476. doi: 10.7589/0090-3558-25.4.469
Childs, J. E., Korch, G. W., Glass, G. E., Shah, K. V., and LeDuc, J. W. (1987b). Epizootiology of Hantavirus infections in Baltimore: isolation of a virus from wild rats, and characteristics of infected rat populations. Am. J. Epidemiol. 126, 55–68. doi: 10.1093/oxfordjournals.aje.a114662
Childs, J. E., and Peters, C. J. (1993). “Ecology and epidemiology of arenaviruses and their hosts,” in The Arenaviridae, ed M. S. Salvato (New York, NY: Plenum Press), 331–384. doi: 10.1007/978-1-4615-3028-2_19
Childs, J. E., and Wilson, L. J. (1994). “Lymphocytic choriomeningitis,” in Handbook of Zoonoses: Viral, ed G. W. Beran (Boca Raton, FL: CRC Press), 483–491.
Combs, M., Puckett, E. E., Richardson, J., Mims, D., and Munshi-South, J. (2018). Spatial population genomics of the brown rat (Rattus norvegicus) in New York City. Mol. Ecol. 27, 83–98. doi: 10.1111/mec.14437
Davis, D. E. (1953). The characteristics of rat populations. Quart. Rev. Biol. 28, 373–401. doi: 10.1086/399860
Desmyter, J., LeDuc, J. W., Johnson, K. M., Brasseur, F., Deckers, C., and van Ypersele, S. C. (1983). Laboratory rat associated outbreak of haemorrhagic fever with renal syndrome due to Hantaan-like virus in Belgium. Lancet 2, 1445–1448. doi: 10.1016/S0140-6736(83)90797-3
Dohmae, K., and Nishimune, Y. (1995). Protection against hantavirus infection by dam's immunity transferred vertically to neonates. Arch. Virol. 140, 165–172.
Douglass, R. J., Calisher, C. H., Wagoner, K. D., and Mills, J. N. (2007). Sin Nombre virus infection of deer mice in Montana: characteristics of newly infected mice, incidence, and temporal pattern of infection. J. Wildl. Dis. 43, 12–22. doi: 10.7589/0090-3558-43.1.12
Duggan, J. M., Close, R., McCann, L., Wright, D., Keys, M., McCarthy, N., et al. (2017). A seroprevalence study to determine the frequency of Hantavirus infection in people exposed to wild and pet fancy rats in England. Epidemiol. Infect. 145, 2458–2465. doi: 10.1017/S0950268817001480
Dykewicz, C. A., Dato, V. M., Fisher-Hoch, S. P., Howarth, M. V., Perez-Oronoz, G. I., Ostroff, S. M., et al. (1992). Lymphocytic choriomeningitis outbreak associated with nude mice in a research institute. JAMA 267, 1349–1353. doi: 10.1001/jama.1992.03480100055030
Easterbrook, J. D., Kaplan, J. B., Vanasco, N. B., Reeves, W. K., Purcell, R. H., Kosoy, M. Y., et al. (2007b). A survey of zoonotic pathogens carried by Norway rats in Baltimore, Maryland, USA. Epidemiol. Infect. 135, 1192–1199. doi: 10.1017/S0950268806007746
Easterbrook, J. D., and Klein, S. L. (2008a). Seoul virus enhances regulatory and reduces proinflammatory responses in male Norway rats. J. Med. Virol. 80, 1308–1318. doi: 10.1002/jmv.21213
Easterbrook, J. D., and Klein, S. L. (2008b). Corticosteroids modulate Seoul virus infection, regulatory T-cell responses and matrix metalloprotease 9 expression in male, but not female, Norway rats. J. Gen. Virol. 89(Pt 11), 2723–2730. doi: 10.1099/vir.0.2008/03715-0
Easterbrook, J. D., Zink, M. C., and Klein, S. L. (2007a). Regulatory T cells enhance persistence of the zoonotic pathogen Seoul virus in its reservoir host. Proc. Natl. Acad. Sci. U.S.A. 104, 15502–15507. doi: 10.1073/pnas.0707453104
Ejrnaes, M., Filippi, C. M., Martinic, M. M., Ling, E. M., Togher, L. M., Crotty, S., et al. (2006). Resolution of a chronic viral infection after interleukin-10 receptor blockade. J. Exp. Med. 203, 2461–2472. doi: 10.1084/jem.20061462
Emonet, S., Retornaz, K., Gonzalez, J. P., de Lamballerie, X., and Charrel, R. N. (2007). Mouse-to-human transmission of variant lymphocytic choriomeningitis virus. Emerg. Infect. Dis. 13, 472–475. doi: 10.3201/eid1303.061141
Eyzaguirre, E. J., Milazzo, M. L., Koster, F. T., and Fulhorst, C. F. (2008). Choclo virus infection in the Syrian golden hamster. Am. J. Trop. Med. Hyg. 78, 669–674. doi: 10.4269/ajtmh.2008.78.669
Farmer, T. W., and Janeway, C. A. (1942). Infections with the virus of Lymphocytic Choriomeningitis. Medicine 21, 65–94. doi: 10.1097/00005792-194202000-00001
Fischer, S. A. (2008). Emerging viruses in transplantation: there is more to infection after transplant than CMV and EBV. Transplantation 86, 1327–1339. doi: 10.1097/TP.0b013e31818b6548
Fischer, S. A., Graham, M. B., Kuehnert, M. J., Kotton, C. N., Srinivasan, A., Marty, F. M., et al. (2006). Transmission of lymphocytic choriomeningitis virus by organ transplantation. N. Engl. J. Med. 354, 2235–2249. doi: 10.1056/NEJMoa053240
Folk, S., Steinbecker, S., Windmeyer, J., MacNeil, A., Campbell, S., and Rollin, P. E. (2011). Lymphocytic Choriomeningitis with severe manifestations, Missouri, USA. Emerg. Infect. Dis. 17, 1973–1974. doi: 10.3201/eid1710.110911
Gardner-Santana, L. C., Norris, D. E., Fornadel, C. M., Hinson, E. R., Klein, S. L., and Glass, G. E. (2009). Commensal ecology, urban landscapes, and their influence on the genetic characteristics of city-dwelling Norway rats (Rattus norvegicus). Mol. Ecol. 13, 2766–2778 doi: 10.1111/j.1365-294X.2009.04232.x
Glass, G. E., Childs, J. E., Korch, G. W., and LeDuc, J. W. (1988). Association of intraspecific aggression and hantavirus infection in wild rats (Rattus norvegicus). Epidemiol. Infect. 101, 459–472. doi: 10.1017/S0950268800054418
Glass, G. E., Childs, J. E., Korch, G. W., and LeDuc, J. W. (1989). Comparative Ecology and Social Interactions of Norway Rats Rattus norvegicus, in Baltimore, Maryland. Vol 130 Occasional papers of the Museum of Natural History. The University of Kansas, 1–33.
Glass, G. E., Klein, S. L., Norris, D. E., and Gardner, L. C. (2016). Multiple paternity in urban Norway rats: extended ranging for mates. Vector-borne Zoon. Dis. 16, 342–348. doi: 10.1089/vbz.2015.1816
Glass, G. E., Livingstone, W., Mills, J. N., Hlady, W. G., Fine, J. B., Biggler, W., et al. (1998). Black Creek Canal Virus infection in Sigmodon hispidus in southern Florida. Am. J. Trop. Med. Hyg. 59, 699–703. doi: 10.4269/ajtmh.1998.59.699
Glass, G. E., Watson, A. J., LeDuc, J. W., and Childs, J. E. (1994). Domestic cases of hemorrhagic fever with renal syndrome in the United States. Nephron 68, 48–51. doi: 10.1159/000188086
Gregg, M. B. (1975). Recent outbreaks of lymphocytic choriomeningitis in the United States of America. Bull.World Health. Org. 52, 549–554.
Hannah, M. F., Bajic, V. B., and Klein, S. L. (2008). Sex differences in the recognition of and innate antiviral responses to Seoul virus in Norway rats. Brain Behav. Immun. 22, 503–516. doi: 10.1016/j.bbi.2007.10.005
Hinson, E. R., Shone, S. M., Zink, M. C., Glass, G. E., and Klein, S. L. (2004). Wounding: the primary mode of Seoul virus transmission among male Norway rats. Am. J. Trop. Med. Hyg. 70, 310–317 doi: 10.4269/ajtmh.2004.70.310
Hotchin, J. (1971). The contamination of laboratory animals with lymphocytic choriomeningitis virus. Am. J. Pathol. 64, 747–769.
Hotchin, J., Sikora, E., Kinch, W., Hinman, A., and Woodall, J. (1977). Lymphocytic Choriomeningitis in a hamster colony causes infection of hospital personnel. Science 185, 1173–1174. doi: 10.1126/science.185.4157.1173
Hutchinson, K. L., Rollin, P. E., and Peters, C. J. (1998). Pathogenesis of a North American hantavirus, Black Creek Canal virus, in experimentally infected Sigmodon hispidus. Am. J. Trop. Med. Hyg. 59, 58–65. doi: 10.4269/ajtmh.1998.59.58
Ike, F., Bourgade, F., Ohsawa, K., Sato, H., Morikawa, S., Saijo, M., et al. (2007). Lymphocytic Choriomeningitis infection undetected by dirty-bedding sentinel monitoring and revealed after embryo transfer of an inbred strain derived from wild mice. Comp. Med. 57, 272–281.
Jameson, L. J., Taori, S. K., Atkinson, B., Levick, P., Featherstone, C. A., van der Burgt, G., et al. (2013). Pet rats as a source of Hantavirus in England and Wales, 2013. Euro Surveill. 18:20415. doi: 10.2807/ese.18.09.20415-en
Jamieson, D. J., Kourtis, A. P., Bell, M., and Rasmussen, S. A. (2006). Lymphocytic choriomeningitis virus: an emerging obstetric pathogen? Am. J. Obstet.Gynecol. 194, 1532–1536. doi: 10.1016/j.ajog.2005.11.040
Kang, S. S., and McGavern, D. B. (2008). Lymphocytic choriomeningitis infection of the central nervous system. Front. Biosci. 13, 4529–4543. doi: 10.2741/3021
Kawamata, J., Yamanouchi, T., Dohmae, K., Miyamoto, H., Takahaski, M., Yamanishi, K., et al. (1987). Control of laboratory acquired hemorrhagic fever with renal syndrome (HFRS) in Japan. Lab. Anim. Sci. 37, 431–436.
Keiner, C. (2005). Wartime rat control, rodent ecology and the rise and fall of chemical rodenticides. Endeavour 29, 119–125. doi: 10.1016/j.endeavour.2005.07.004
Kerins, J. L., Koske, S. E., Kazmierczak, J., Austin, C., Gowdy, K., Dibernardo, A., et al. (2018). Outbreak of Seoul virus among rats and rat owners – United States and Canada, 2017. MMWR Morb. Mortal. Wkly. Rep. 67, 131–134. doi: 10.15585/mmwr.mm6704a5
Khaiboullina, S. F., and St. Jeor, S. C. (2002). Hantavirus immunology. Viral Immunol. 15, 609–625 doi: 10.1089/088282402320914548
Kim, B., Vincent, H., Heikki, H., Darouny, P., Jean-Pierre, H., and Philippe, B. (2009). Rodent-borne zoonotic viruses in Southeast Asia. J. Nat. Sci. 43, 94–105.
Kitano, M. (1944b). Studies concerning epidemic hemorrhagic fever. J. Jpn. Infect. Dis. Soc. 18, 303–317.
Klein, S. L., Bird, B. H., and Glass, G. E. (2000). Sex differences in Seoul virus infection are not related to adult sex steroid concentrations in Norway rats. J. Virol. 74, 8213–8217. doi: 10.1128/JVI.74.17.8213-8217.2000
Klein, S. L., Bird, B. H., and Glass, G. E. (2001). Sex differences in immune responses and viral shedding following Seoul virus infection in Norway rats. Am. J. Trop. Med. Hyg. 65, 57–63. doi: 10.4269/ajtmh.2001.65.57
Klein, S. L., Cernetich, A., Hilmer, S., Hoffman, E. P., Scott, A. L., and Glass, G. E. (2004a). Differential expression of immunoregulatory genes in male and female Norway rats following infection with Seoul virus. J. Med. Virol. 74, 180–190. doi: 10.1002/jmv.20163
Klein, S. L., Marson, A. L., Scott, A. L., Ketner, G., and Glass, G. E. (2002). Neonatal sex steroids affect responses to Seoul virus infection in male but not female Norway rats. Brain Behav. Immun. 16, 736–746. doi: 10.1016/S0889-1591(02)00026-0
Klein, S. L., Zink, M. C., and Glass, G. E. (2004b). Seoul virus infection increases aggressive behaviour in male Norway rats. Anim. Behav. 67, 421–429 doi: 10.1016/j.anbehav.2003.03.022
Klempa, B. (2018). Reassortment events in the evolution of hantaviruses. Virus Genes 54, 638–646. doi: 10.1007/s11262-018-1590-z
Knust, B., MacNeil, A., Wong, S. J., Backenson, P. B., Gibbons, A., Rollin, P. E., et al. (2011). Exposure to Lymphocytic Choriomeningitis Virus, New York, USA. Emerg. Infect. Dis. 17, 1324–1325. doi: 10.3201/eid1707.101349
Knust, B., Ströher, U., Edison, L., Albariño, C. G., Lovejoy, J., Armeanu, E., et al. (2014). Lymphocytic Choriomeningitis Virus in employees and mice at multipremises feeder-rodent operation, United States, 2012. Emerg. Infect. Dis. 20, 240–247. doi: 10.3201/eid2002.130860
Korch, G. W., Childs, J. E., Glass, G. E., and LeDuc, J. W. (1989). Spatial and temporal analyses and host range of hantaviral infections within small mammal communities of Baltimore, Maryland USA. Am. J. Trop. Med. Hyg. 41, 230–240. doi: 10.4269/ajtmh.1989.41.230
Lambpropolous, S., Fine, J. B., Perbeck, A., Torres, D., Glass, G. E., McHugh, P., et al. (1999). Rodent control in urban areas: an interdisciplinary approach. J. Environ. Health 61, 12–17.
Lecompte, E., ter Meulen, J., Emonet, S., Daffis, S., and Charrel, R. N. (2007). Genetic identification of Kodoko virus, a novel arenavirus of the African pigmy mouse (Mus nannomys minutoides) in West Africa. Virology 364, 178–183. doi: 10.1016/j.virol.2007.02.008
LeDuc, J. W., Ksiazek, T. G., Rossi, C. A., and Dalrymple, J. M. (1990). A retrospective analysis of sera collected by the Hemorrhagic Fever Commission during the Korean conflict. J. Infect. Dis. 162, 1182–1184. doi: 10.1093/infdis/162.5.1182
LeDuc, J. W., Smith, G. A., Childs, J. E., Pinheiro, F. P., Maiztegui, J. I., Niklasson, B., et al. (1986). Global survey of antibody to Hantaan-related viruses among peridomestic rodents. Bull. WHO 64, 139–144.
Lee, H. W., Baek, L. J., and Johnson, K. M. (1982). Isolation of Hantaan virus, the etiologic agent of Korean hemorrhagic-fever, from wild urban rats. J. Infect. Dis. 146, 638–644. doi: 10.1093/infdis/146.5.638
Lee, H. W., and Johnson, K. M. (1982). Laboratory-acquired infections with Hantaan virus, the etiologic agent of Korean hemorrhagic-fever. J. Infect. Dis. 146, 645–651. doi: 10.1093/infdis/146.5.645
Lee, H. W., Lee, P. W., and Johnson, K. M. (1978). Isolation of etiologic agent of Korean hemorrhagic-fever. J. Infect. Dis. 137, 298–308.
Lee, P. W., Yanagihara, R., Gibbs, C. J. Jr., and Gajdusek, D. C. (1986). Pathogenesis of experimental Hantaan virus infection in laboratory rats. Arch. Virol. 88, 57–66. doi: 10.1007/BF01310890
Lehmann-Grube, F. (1963). Lymphocytic choriomeningitis in the mouse. II. Establishment of carrier colonies. Archiv. Virusforsch. 14, 353–357.
Lehmann-Grube, F. (1971). “Lymphocytic Choriomeningitis virus,”. in Virology Monographs 10, Handbook of Virus Research, eds S. Gard Hallauer and C. K. F. Meyer (New York, NY: Springer-Verlag), 339–339. doi: 10.1007/978-3-7091-8276-5
Lehmann-Grube, F. (1984). Portrait of viruses: arenaviruses. Intervirology 22, 121–145. doi: 10.1159/000149543
Lewis, A. M., Rowe, W. P., Turner, H. C., and Huebner, R. J. (1965). Lymphocytic-Choriomeningitis virus in hamster tumor: spread to hamsters and humans. Science 150, 363–364. doi: 10.1126/science.150.3694.363
Li, W., and Klein, S. L. (2012). Seoul virus-infected rat lung endothelial cells and alveolar macrophages differ in their ability to support virus replication and induce regulatory T cell phenotypes. J. Virol. 86, 11845–11855 doi: 10.1128/JVI.01233-12
Lloyd, G., Bowen, E. T. W., Jones, N., and Pendry, A. (1984). HFRS outbreak associated with laboratory rats in UK. Lancet 1, 1175–1176. doi: 10.1016/S0140-6736(84)91413-2
Lloyd, G., and Jones, N. (1986) Infection of laboratory workers with hantavirus acquired from immunocytomas propagated in laboratory rats. J. Infect. 12, 117–125. doi: 10.1016/S0163-4453(86)93533-4
Long, J. L. (2003). Introduced Mammals of the World. Collingwood, VIC: CSIRO Publishing. doi: 10.1071/9780643090156
Lundkvist, A., Verner-Carlsson, J., Plyusnina, A., Forslund, L., Feinstein, R., and Plyusnin, A. (2013). Pet rat harbouring Seoul Hantavirus in Sweden, June 2013. Euro Surveill.18:20521. doi: 10.2807/1560-7917.ES2013.18.27.20521
MacNeil, A., Ströher, U., Farnon, E., Campbell, S., Cannon, D., Paddock, C. D., et al. (2012). Solid organ transplant–associated Lymphocytic Choriomeningitis, United States, 2011. Emerg. Infect. Dis. 18, 1256–1262. doi: 10.3201/eid1808.120212
Maris, C. H., Chappell, C. P., and Jacob, J. (2007). Interleukin-10 plays an early role in generating virus-specific T cell anergy. BMC Immunol. 8:8. doi: 10.1186/1471-2172-8-8
Mathur, G., Yadav, K., Ford, B., Schafer, I. J., Basavaraju, S. V., Knust, B., et al. (2017). High clinical suspicion of donor-derived disease leads to timely recognition and early intervention to treat solid organ transplant-transmitted lymphocytic choriomeningitis virus. Transpl. Infect. Dis. 19. doi: 10.1111/tid.12707
Mattar, S., Guzman, C., and Figueiredo, A. T. (2015). Diagnosis of hantavirus infection in humans Expert Rev. Anti-Infect. Ther. 13, 939–946. doi: 10.1586/14787210.2015.1047825
McAllister, R. C., and Jonsson, C. B. (2014). Hantaviruses: past, present and future. Future Virol. 9, 87–99. doi: 10.2217/fvl.13.113
McElhinney, L. M., Marston, D. A., Pounder, K. C., Goharriz, H., Wise, E. L., Verner-Carlsson, J., et al. (2017). High prevalence of Seoul hantavirus in a breeding colony of pet rats. Epidemiol. Infect.145, 3115–3124. doi: 10.1017/S0950268817001819
McKenna, P., van der Groen, G., Hoofd, G., Beelaert, G., Leirs, H., et al. (1992). Eradication of hantavirus infection among laboratory rats by application of caesarian section and a foster mother technique. J. Infect. 25, 181–188. doi: 10.1016/0163-4453(92)94035-V
Meritet, J. F., Krivine, A., Lewin, F., Poissonnier, M. H., Poizat, R., Loget, P., et al. (2009). A case of congenital lymphocytic choriomeningitis virus (LCMV) infection revealed by hydrops fetalis. Prenat. Diagn. 29, 626–627. doi: 10.1002/pd.2240
Meyer, H. M. Jr., Johnson, R. T., Crawford, I. P., Dascomb, H. E., and Rogers, N. G. (1960). Central nervous system syndromes of viral etiology. Am. J. Med. 29, 334–347. doi: 10.1016/0002-9343(60)90029-2
Montali, R. J., Ramsay, E. C., Stephensen, C. B., Worley, M., Davis, J. A., and Holmes, K. V. (1989). A new transmissible viral hepatitis of marmosets and tamarins. J. Infect. Dis. 160, 759–765. doi: 10.1093/infdis/160.5.759
Montali, R. J., Scanga, C. A., Pernikoff, D., Wessner, D. R., Ward, R., and Holmes, K. V. (1993). A common-source outbreak of callitrichid hepatitis in captive tamarins and marmosets. J. Infect. Dis. 167, 946–950. doi: 10.1093/infdis/167.4.946
Morita, C., Matsuura, Y., Fujji, H., Joh, K., Baba, K., Kato, M., et al. (1991). Isolation of lymphocytic choriomeningitis virus from wild house mice (Mus musculus) in Osaka Port, Japan. J. Vet. Med. Sci. 53, 889–892. doi: 10.1292/jvms.53.889
Moskophidis, D., Lechner, F., Pircher, H., and Zinkernagel, R. M. (1993). Virus persistence in acutely infected immunocompetent mice by exhaustion of antiviral cytotoxic effector T cells. Nature 362, 758–761. doi: 10.1038/362758a0
Murphy, R. G., and Hide, G. (2005). “Population biology of the urban house mouse (Mus musculus) in the U.K.,” in Fifth International Conference of Urban Pests, eds C. Y. Lee and R. W. (Singapore).
Nichol, S. T., Spiropoulou, C. F., Morzunov, S., Rollin, P. E., Ksiazek, T. G., et al. (1993). Genetic identification of a hantavirus associated with an outbreak of acute respiratory illness. Science 262, 914–917. doi: 10.1126/science.8235615
Nuzum, E. O., Rossi, C. A., Stephenson, E. H., and LeDuc, J. W. (1988). Aerosol transmission of Hantaan and related viruses to laboratory rats. Am. J. Trop. Med. Hyg. 38, 636–640. doi: 10.4269/ajtmh.1988.38.636
Palacios, G., Druce, J., Du, L., Tran, T., Birch, C., Briese, T., et al. (2008). A new arenavirus in a cluster of fatal transplant-associated diseases. N. Eng. J. Med. 358, 991–998. doi: 10.1056/NEJMoa073785
Penaloza-MacMaster, P., Kamphorst, A. O., Wieland, A., Araki, K., Iyer, S. S., West, E. E., et al. (2014). Interplay between regulatory T cells and PD-1 in modulating T cell exhaustion and viral control during chronic LCMV infection. J. Exp. Med. 211, 1905–1918. doi: 10.1084/jem.20132577
Peters, C. J. (2006). Lymphocytic Choriomeningitis virus — an old enemy up to new tricks. N. Engl. J. Med. 354, 2208–2211. doi: 10.1056/NEJMp068021
Petras, M. L. (1967). Studides of natural populations of Mus. I. Biochemical poly morphisms and their bearing on breeding structure. Evolution 21, 259–274.
Pocock, M. J. O., Hauffe, H. C., and Searle, J. B. (2005). Dispersal in house mice. Biol. J. Linn. Soc. 84, 565–583. doi: 10.1111/j.1095-8312.2005.00455.x
Reynes, J.-M., Carli, D., Bour, J.-B., Boudjeltia, S., Dewilde, A., Gerbier, G., et al. (2017). Seoul virus infection in humans, France, 2014-2016. Emerg. Infect. Dis. 23, 973–977. doi: 10.3201/eid2306.160927
Rivers, T. M., and Scott, T. F. M. (1935). Meinigitis in man caused by a filterable virus. Science 109, 439–440. doi: 10.1126/science.81.2105.439-a
Robertson, B. C., and Gemmell, N. J. (2004). Defining eradication units to control invasive pests. J. Appl. Ecol. 41, 1042–1048. doi: 10.1111/j.0021-8901.2004.00984.x
Robin, C., Perkins, E., Watkins, F., and Christley, R. (2017). Pets, purity and pollution: why conventional models of disease transmission do not work for pet rat owners. Int. J. Environ. Res. Public Health 14:E1526. doi: 10.3390/ijerph14121526
Rosen, A., and Ison, M. G. (2017). Screening of living organ donors for endemic infections: understanding the challenges and benefits of enhanced screening. Transpl. Infect. Dis. 19:e12633. doi: 10.1111/tid.12633
Sabattini, M. S., Barrera Oro, J. G., Maiztegui, J. I., Fernandez, D., Contigiani, M. S., and Diaz, G. E. (1970). Aislamiento de un Arenavirus relacionado con el de la coriomeningitis linfocitica (LCM) a partir de un Mus musculus capturado en zona endemica de fiebre hemorragica Argentina (FHA). Rev. Soc. Argen. Microbiol. 11, 182–184.
Sabattini, M. S., Barrera Oro, J. G., Maiztegui, J. I., and Ferradas, B. R. (1974). Actividad del virus de la Coriomeningitis linfocitica en el area endemica de Fiebre Hemorragica Argentina. II. Aislamiento a partir de un Mus musculus campestre capturado en el sudeste de Cordoba. Medicina 34, 313–313.
Salvato, M., Borrow, P., Shimomaye, E., and Oldstone, M. B. (1991). Molecular basis of viral persistence: a single amino acid change in the glycoprotein of lymphocytic choriomeningitis virus is associated with suppression of the antiviral cytotoxic T-lymphocyte response and establishment of persistence. J. Virol. 65, 1863–1869.
Salvato, M., Shimomaye, E., Southern, P., and Oldstone, M. B. (1988). Virus-lymphocyte interactions. IV. Molecular characterization of LCMV Armstrong (CTL+) small genomic segment and that of its variant, Clone 13 (CTL-). Virology 164, 517–522. doi: 10.1016/0042-6822(88)90566-1
Schmaljohn, C. S., Chu, Y. K., Schmaljohn, A. L., and Dalrymple, J. M. (1990). Antigenic subunits of Hantaan virus expressed by baculovirus and vaccinia virus recombinants. J. Virol. 64, 3162–3170
Schountz, T., Prescott, J., Cogswell, A. C., Oko, L., Mirowsky-Garcia, K., and Hjelle, A. P. (2007). Regulatory T cell-like responses in deer mice persistently infected with Sin Nombre virus. Proc. Natl. Acad. Sci. U.S.A. 104, 15496–15501. doi: 10.1073/pnas.0707454104
Singleton, G. (1983). The social and genetic structure of a natural colony of house mice, mus musculus, at healesville wildlifesanctuary. Austral. J. Zool. 31, 155–166. doi: 10.1071/ZO9830155
Skinner, H. H., and Knight, E. H. (1973). Natural routes for post-natal transmission of murine lymphocytic choriomeningitis. Lab. Anim. 7, 171–184. doi: 10.1258/002367773781008597
Skinner, H. H., Knight, E. H., and Buckley, L. S. (1976). The hamster as a secondary reservoir host of lymphocytic choriomeningitis virus. J. Hyg. Camb. 76, 299–306. doi: 10.1017/S0022172400055194
Skinner, H. H., Knight, E. H., and Grove, R. (1977). Murine lymphocytic choriomeningitis: the history of a natural cross-infection from wild to laboratory mice. Lab. Anim. 11, 219–222. doi: 10.1258/002367777780936422
Smadel, J. E. (1953). Epidemic hemorrhagic fever. Am. J. Public Health 43, 1327–1330. doi: 10.2105/AJPH.43.10.1327
Smorodintsev, A. A., Al'tshuler, I. S., Dunaevskii, M. L., Kokhredze, K. A., Neustroev, V. D., and Churilov, A. V. (1944). Etiology and clinical observations of hemorrhagic nephroso-nephritis. Medgiz Moscow 26–47.
Smorodintsev, A. A., Chudakov, V. G., and Churilov, A. V. (1959). Haemorrhagic Nephroso-Nephritis. London: Pergamon Press.
Steele, J. H., Fernandez, P. J., and Baer, G. M. (1991). The Natural History of Rabies. Boca Raton, FL: CRC Press.
Stephensen, C. B. (1992). Prevalence of serum antibodies against Lymphocytic Choriomeningitis virus in selected populations from 2 United States cities. J. Med. Virol. 38, 27–31. doi: 10.1002/jmv.1890380107
Stephensen, C. B., Jacob, J. R., Montali, R. J., Holmes, K. V., Muchmore, E., Compans, R. W., et al. (1991). Isolation of an arenavirus from a marmoset with callitrichid hepatitis and its serologic association with disease. J. Virol. 65, 3995–4000.
Sukthana, Y. (2006). Toxoplasmosis: beyond animals to humans. Trends Parasitol. 22, 137–142. doi: 10.1016/j.pt.2006.01.007
Takimoto, K., Taharaguchi, M., Morikawa, S., Ike, F., and Yamada, Y. K (2008). Detection of the antibody to Lymphocytic Choriomeningitis virus in sera of laboratory rodents infected with viruses of laboratory and newly isolated strains by ELISA using purified recombinant nucleoprotein. Exp. Anim. 57, 357–365. doi: 10.1538/expanim.57.357
Traub, E. (1936a). An epidemic in a mouse colony due to the virus of acute lymphocytic choriomeningitis. J. Exp. Med. 63, 533–547. doi: 10.1084/jem.63.4.533
Traub, E. (1936b). The epidemiology of Lymphocytic Choriomeningitis in white mice. J. Exp. Med. 64, 183–200. doi: 10.1084/jem.64.2.183
Traub, E. (1936c). Persistence of Lymphocytic Choriomeningitis virus in immune animals and its relation to immunity. J. Exp. Med. 63, 847–861. doi: 10.1084/jem.63.6.847
Traub, E. (1938). Factors influencing the persistence of choriomenigitis virus in the blood of mice after clinical recovery. J. Exp. Med. 68, 229–250. doi: 10.1084/jem.68.2.229
Traub, E. (1939). Epidemiology of lymphocytic choriomeningitis in a mouse stock observed for four years. J. Exp. Med. 69, 801–817. doi: 10.1084/jem.69.6.801
Traub, R., and Wisseman, C. L. Jr. (1978). Korean hemorrhagic fever. J. Infect. Dis. 138, 267–272. doi: 10.1093/infdis/138.2.267
Umenai, T., Lee, P. W., Toyoda, T., Yoshinaga, K., Horiuchi, T., Lee, H. W., et al. (1979). Korean haemorrhagic fever in staff in an animal laboratory. Lancet 313, 1314–1316. doi: 10.1016/S0140-6736(79)91948-2
van den Pol, A. N. (2006). Viral infections in the developing and mature brain. Trends Neurosci. 29, 398–406. doi: 10.1016/j.tins.2006.06.002
Vanzee, B. E., Douglas, R. G. Jr., Betts, R. F., Bauman, A. W., Fraser, D. W., and Hinman, A. R. (1975). Lymphocytic choriomeningitis in University Hospital personnel. Am. J. Med. 58, 803–809. doi: 10.1016/0002-9343(75)90635-X
Wahl-Jensen, V., Chapman, J., Asher, L., Fisher, R., Zimmerman, M., et al. (2007). Temporal analysis of Andes virus and Sin Nombre virus infections of Syrian hamsters. J. Virol. 81, 7449–7462. doi: 10.1128/JVI.00238-07
Williams, S. H., Che, X., Garcia, J. A., Klena, J. D., Lee, B., Muller, D., et al. (2018). Viral diversity of house mice in New York City. mBio 9:01354-17. doi: 10.1128/mBio.01354-17
Wilson, D. E., and Reeder, D. M. (2005). Mammal Species of the World, 2nd Edn. Baltimore, MD: Johns Hopkins University Press.
Wooley, J. G., Stimpert, F. D., Kessel, J. F., and Armstrong, C. (1939). A Study of human sera antibodies capable of neutralizing the virus of Lymphocytic Choriomeningitis. Public Health Rep. 54, 938–944. doi: 10.2307/4582902
Yanagihara, R., Amyx, H. L., and Gajdusek, D. C. (1985). Experimental infection with Puumala virus, the etiologic agent of nephropathia epidemica, in bank voles (Clethrionomys glareolus). J. Virol. 55, 34–38.
Zhang, X.-K., Takashima, I., and Hashimoto, N. (1988). Role of maternal antibody in protection from hemorrhagic fever with renal syndrome virus infection in rats. Arch. Virol. 103, 253–265. doi: 10.1007/BF01311097
Keywords: lymphocytic choriomeningitis virus, Seoul virus, Rattus norvegicus, Mus musculus, zoonotic disease
Citation: Childs JE, Klein SL and Glass GE (2019) A Case Study of Two Rodent-Borne Viruses: Not Always the Same Old Suspects. Front. Ecol. Evol. 7:35. doi: 10.3389/fevo.2019.00035
Received: 27 October 2018; Accepted: 29 January 2019;
Published: 21 March 2019.
Edited by:
Mathew Samuel Crowther, University of Sydney, AustraliaReviewed by:
Armanda Bastos, University of Pretoria, South AfricaChris Whitehouse, United States Food and Drug Administration, United States
Copyright © 2019 Childs, Klein and Glass. This is an open-access article distributed under the terms of the Creative Commons Attribution License (CC BY). The use, distribution or reproduction in other forums is permitted, provided the original author(s) and the copyright owner(s) are credited and that the original publication in this journal is cited, in accordance with accepted academic practice. No use, distribution or reproduction is permitted which does not comply with these terms.
*Correspondence: Gregory E. Glass, Z2dsYXNzQHVmbC5lZHU=