- 1Horticulture Section, School of Integrative Plant Science, Urban Horticulture Institute, Cornell University, Ithaca, NY, United States
- 2Department of Biology, Boston University, Boston, MA, United States
- 3Department of Ecology and Evolutionary Biology, Cornell University, Ithaca, NY, United States
- 4Department of Biological and Environmental Engineering, Cornell University, Ithaca, NY, United States
- 5Soil and Crop Sciences Section, School of Integrative Plant Science, Cornell University, Ithaca, NY, United States
Intensive agriculture represents a recent extension of green roof technology. Perceived ecosystem services provided by rooftop farming include stormwater management and the production of affordable and nutritious vegetables for local consumption. However, intensive agriculture can increase nutrient loads to surface water, yet there is little empirical data from full-scale operational rooftop farms. This study reports the N balance and N management efficiency of the Brooklyn Grange Navy Yard Farm, a 0.61-ha farm atop an 11-story building in New York City USA. We monitored atmospheric N deposition, soil N concentration, N output by harvest, N leaching from soil, and drainage N output, in addition to estimating net N mineralization and the N load to sewers during the combined sewer overflows. We found that the annual drainage N output was 1,100% of the atmospheric bulk N deposition, and was 540% of the estimated total atmospheric N deposition, which makes the Brooklyn Grange a net N source in the urban environment. Annual N leaching from soil was 97% of fertilizer N input, and the efficiency of N management can be lower than in conventional vegetable production. For the Brooklyn Grange to integrate stormwater management and intensive agriculture, it will be important to use soil with greater water holding capacity within the range of readily available water, and to recycle drainage. This case study shows how the intensification of agriculture on rooftops should be managed for both the yield and quality of crops and to reduce N loss to storm drains, which affects aquatic ecosystems and water quality.
Introduction
As hotspots for biogeochemical cycles, cities offer various opportunities for developing novel ecosystems to enhance sustainability (Palmer et al., 2004; Kaye et al., 2006; Grimm et al., 2008; Pataki et al., 2011; Lundholm, 2015). These opportunities include green roofs to manage stormwater runoff, save energy, reduce air pollution, and preserve biodiversity (Mentens et al., 2006; Oberndorfer et al., 2007; Berndtsson, 2010; Rowe, 2011). More recently, rooftop farming has been explored as a way to achieve these goals and to produce food for local communities (Ackerman et al., 2013; Thomaier et al., 2015; Whittinghill and Starry, 2016; Harada et al., 2017). Ideally, these diverse ecosystem services could be integrated into the limited space available in urban environments and to offer social-cultural benefits, such as environmental education, eco-justice, and building more cohesive communities (Lovell, 2010; Lovell and Taylor, 2013; McPhearson et al., 2013; Specht et al., 2014). However, it can be challenging to integrate ecosystem services, if each requires different management strategies. For example, intensive vegetable production and stormwater management are among the ecosystem services emphasized in the studies of rooftop farming (Ackerman et al., 2013; Specht et al., 2014; Thomaier et al., 2015; Whittinghill et al., 2015; Goldstein et al., 2016). Intensive agriculture can require higher nutrient inputs to maintain crop yield and quality, while stormwater management aims to reduce nutrient loads to surface water by managing vegetation with limited supplemental nutrients. Drainage output of nutrients from intensive agriculture, such as nitrogen (N) from application of high amounts of fertilizer, has led to multiple negative consequences, including contamination of groundwater and development of “dead zones,” such as the Chesapeake Bay, Gulf of Mexico, and Long Island Sound (Howarth et al., 2000; Rabalais et al., 2002; Kemp et al., 2005; Howarth, 2008). These nutrient loadings into waterways are regulated by the Clean Water Act through the total maximum daily load and best management practices (Wainger, 2012). Some estuaries are more sensitive to nutrient loads than others. For instance, the longer mean residence time of water in Long Island Sound (1,100 days) compared to Delaware Bay (60 days) and Chesapeake Bay (250 days) makes N management particularly important to New York City, which is a major source of N for Long Island Sound (Nixon et al., 1996; Howarth et al., 2006). Nutrient loadings from urban land uses including urban agriculture are often managed by sewer systems. For example, 60% of NYC's sewer systems manage stormwater and sanitary sewage together in the same sewers (NYC EDC, 2013), and these combined sewers can bypass treatment plants during storm events, discharging untreated effluent to surface water (US EPA, 2004). The Clean Water Act requires that cities using combined sewers must implement best management practices (Carter and Fowler, 2008). In the infancy of rooftop farming practices, there are opportunities to establish and implement best management practices to limit the nutrient loads from urban farms to surface waterbodies.
Many studies have reported rates of N output from agricultural, forested, and urban land uses. Whittinghill et al. (2016) reports the drainage loss of N from a rooftop farm in NYC to be about three times greater than the maximum N output from urban watersheds, and toward the upper end of the range leaving N-saturated forests (Fenn et al., 1998; Howarth et al., 2002; Campbell et al., 2004; Groffman et al., 2004; Berndtsson et al., 2006; Gregoire and Clausen, 2011; Likens, 2013; Whittinghill et al., 2016). The rate of N loss from rooftop farms falls within the range of in-ground agriculture, in-ground organic farming, in-ground farming using BMPs for no-tillage system with legume cover crops, and the low end of in-ground intensive vegetable production (Goulding et al., 2000; Eriksen et al., 2004; Jayasundara et al., 2007; Zhao et al., 2010; Min et al., 2012; Pärn et al., 2012; Syswerda et al., 2012; Cameron et al., 2013; Whittinghill et al., 2016; Zhang et al., 2017). These observations suggest that relatively low drainage N output from rooftop farming could be accomplished, but the best approaches to achieve this goal have not been determined.
There are many potential management practices urban farmers could use to reduce drainage output of nutrients. For example, reducing drainage volume can reduce drainage N output from soil-plant systems on roofs (Di and Cameron, 2002; Hartz, 2006; Cameron et al., 2013). However, this can be difficult when mineral fractions like expanded shale, clay, and slate are used as base materials in rooftop farm soils because they hold less water and nutrients than field soils, increasing the potential for drainage output (Emilsson et al., 2007; Ampim et al., 2010; Best et al., 2015; Harada et al., 2018). Even in non-production green roofs where plant yield and quality are arguably less important, fertilizer and irrigation inputs during the initial establishment period can increase drainage output of nutrients (Emilsson et al., 2007; Berndtsson, 2010; Rowe, 2011; Driscoll et al., 2015). In comparison to vegetables, non-crop species, such as Sedum spp. require less water and nutrients, so subsidies can be reduced or terminated after establishment (see Emilsson et al., 2007; Shock and Wang, 2011; Congreves and Van Eerd, 2015; Van Mechelen et al., 2015). In rooftop farms, however, economic viability relies on the yield and quality of diverse vegetables with high market value, such as salad greens, tomatoes, peppers, and eggplants, which have high requirements for water and nutrients (Shock and Wang, 2011; Ackerman et al., 2013; Congreves and Van Eerd, 2015). Since not all nutrient supplements are taken up by these vegetables, greater rates of fertilizer application can lead to greater drainage output of nutrients (Cameron et al., 2013).
Potentially mineralizable N (PMN) can be measured by incubating soil samples to determine the potential for microbes to convert soil organic N to NO3-N and NH4-N (Drinkwater et al., 1996). Kong et al. (2015) report that PMN for a rooftop farm soil falls within the ranges observed in rural forests, agricultural sites, and urban green space (Drinkwater et al., 1995; Benedetti and Sebastiani, 1996; Poudel et al., 2002; Scharenbroch et al., 2005; Szlavecz et al., 2006; Kong et al., 2015). Mineralization of organic N can increase drainage N output from both intensive agriculture and non-production green roofs (Drinkwater et al., 1996; Di and Cameron, 2002; Cameron et al., 2013; Buffam et al., 2016). The mineral components used in soils for rooftop farms and green roofs are amended with compost, peat, coconut coir, and other forms of soil organic matter (SOM) (Ampim et al., 2010; Eksi et al., 2015; Buffam et al., 2016; Whittinghill et al., 2016). In comparison to soils specifically designed for rooftops, potting mixes have much higher organic matter content (Harada et al., 2017). More organic matter may enhance soil water holding capacity, thus reducing drainage output of water and N, but enhanced N mineralization rates of organic nitrogen may increase drainage N from rooftop farms. This magnitude of N loss from rooftop farms is currently unknown.
Despite the potential for rooftop farming to induce drainage N output from roofs, the emergent practice of rooftop farming (see Ackerman et al., 2013; Specht et al., 2014; Thomaier et al., 2015) provide an opportunity to evaluate and determine the best management practices to minimize drainage output of nutrients while maintaining crop yield and quality. Compared to traditional agricultural practices, rooftop farming provides a relatively simpler and more compartmentalized system with centralized drainage that allows for easier quantification of nutrient sources, cycling, and outputs. These novel urban ecosystems permit precise observation and manipulation over time, making an ideal model for improving management practices for sustainable intensification of urban agriculture.
Although multiple studies have documented rates of soil mineralization and drainage N output from rooftop farms, we are not aware of any study that reports the total N balance. The objective of this paper is to quantify the N balance of a full-scale rooftop farm in New York City, USA. We pose the following a priori questions:
1. What is the impact of drainage N output from rooftop farming on urban watershed planning?
2. What is the contribution of atmospheric deposition to total N inputs of rooftop farms?
3. What are the main drivers for N leaching?
4. What lessons can we learn from creating a total ecosystem N budget for writing best management practices for rooftop farming?
Materials and Methods
Site Description
The study was located at the Brooklyn Grange Navy Yard farm (the Grange hereafter), atop an 11-story building in the former Brooklyn Navy Yard, NYC. The entire site area (6,122 m2) is divided into 8 catchments (Figure 1), each with a drain connected to the municipal combined sewer system. Of the entire site area, 33% (2,040 m2) of the surface is impervious and 67% (4,082 m2) is covered with soil underlain by a drainage layer (Figure 1).
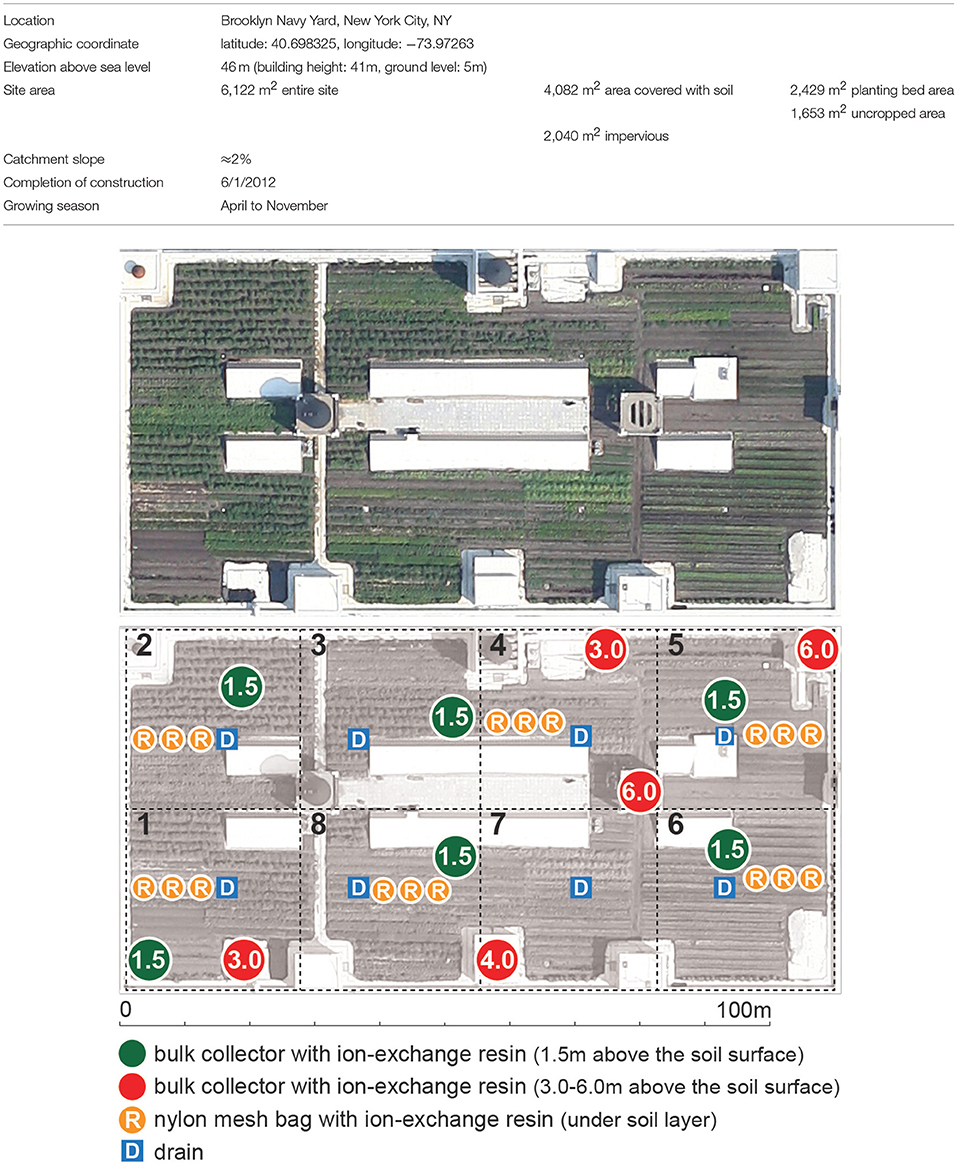
Figure 1. Site information for the Brooklyn Grange Navy Yard farm in New York City, NY. The top figure shows aerial photo of the roof from ©google 2014. In the bottom figure, dotted lines indicate the eight catchments on the roof, and circled numbers indicate the height in meters of atmospheric deposition collectors above the soil surface.
Fertilizer is applied to only the planting bed area, which is 60% of the total area covered with soil (Figure 2). Farmers of the Brooklyn Grange determined the selection and application rates of fertilizer products, which were not controlled by this study. Since the construction of the farm in 2012, farmers have tested various management scenarios to optimize yield because the best management practices specific to rooftop farming had not been established. Over the 3-years study, annual fertilizer N input increased 2-fold (Figure 3). Synthetic N (nitrate, ammoniacal, and urea N) was applied only during the 2015 growing season and accounted for only 5% of the total fertilizer N input during this period. Among non-synthetic N sources summarized in Figure 3, the mined NaNO3 was the only N source that was nearly 100% mineral N. All other N sources are organic N derived from either plant, animal, or seafood by-products, which contained NH4-N <3% of the total N mass. Over the 2014 and 2015 growing seasons, 11 organic N fertilizer products were used, which were narrowed down to only Nature Safe 13-0-0® and Nature Safe 10-2-8® (Darling Ingredients Inc., Irving, TX) in the 2016 growing season, and both were made of animal by-products (Figure 3). Biological N fixation was unlikely to have major impact on N supply, because pea (Pisum sativum) and bush bean (Phaseolus vulgaris) were the only legume crops grown, and occupied <3% (90 m2) of the planting bed area (Benjamin Flanner, personal communication), roughly equivalent to 1 kg N y−1 of biological N fixation based on the general rate for legume crops (Herridge et al., 2008).
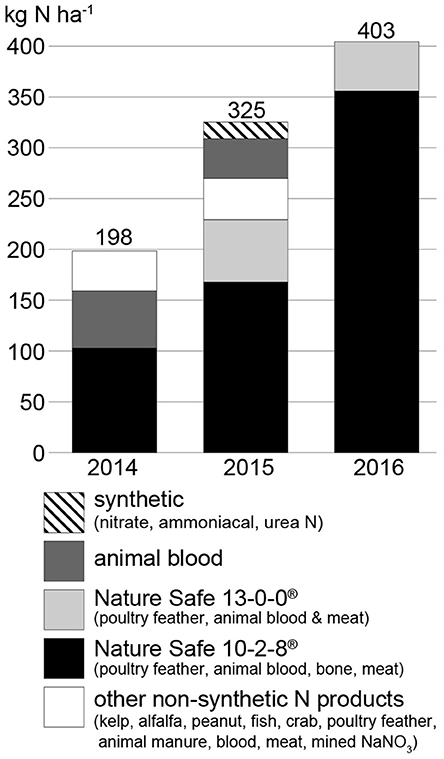
Figure 3. N input by fertilizer application and N sources at the Brooklyn Grange Navy Yard farm, NYC. All values are expressed in terms of the area of the planting beds only.
Soil is Rooflite® Intensive ag (Skyland USA LLC, Avondale, PA), a commercial soil blend of expanded shale, animal manure, and re-used mushroom substrate (Kong et al., 2015; Skyland USA LLC, 2015). The drainage layer consists of filter fabric covering gravel 10–15 cm in depth (Figure 2). Within each catchment, the lateral export of stormwater runoff from impervious surfaces and the leachate from soil enters the drainage layer, then flows laterally to the drain (Figure 2). The Grange grows over 60 crops, with leaf lettuce (Lactuca sativa), mustard greens (Brassica juncea), arugula (Eruca sativa), kale (Brassica oleracea), pepper (Capsicum annuum), and tomato (Solanum lycopersicum) accounting for over 60% of the planting bed area and fresh weight.
Atmospheric N Deposition
Like most rooftops, our site lacks trees, so we measured only bulk deposition. This was compared to total N deposition measured by the National Atmospheric Deposition Program (NADP; nearest site 20 km away) and estimated for our site using the Community Multiscale Air Quality (CMAQ) model (Schwede and Lear, 2014; NADP Total Deposition Science Committee, 2017). Bulk deposition was measured at 11 locations on the roof between 6/2014 and 11/2016. Six collectors were 1.5 m above the soil surface and 5 were placed 3–6 m above the roof plane on rooftop structures (Figure 1). Each bulk collector consisted of a 9 cm polypropylene Büchner funnel inserted into a disposable 20 ml chromatography column (BioRad, Hercules, CA) filled with a mixed bed ion-exchange resin (Dowex Amberlite IRN150). These resin columns were replaced every 6 weeks and extracted and shaken with 150 ml 2 M KCl solution. Nitrate () and ammonium () concentrations were analyzed using a colorimetric microplate reader (VersaMax, Molecular Devices, Sunnyvale, CA) following the method described by Sims et al. (1995) and Doane and Horwáth (2003). Concentrations in sample solutions were converted to fluxes by accounting for the total volume of KCl used to extract samples (150 ml), the surface area of the top of the funnel (9 cm diameter), and the amount of time the columns were left in the field.
N Leaching From Soil
N leaching from soil is the vertical export of N from soil to the drainage layer (Figure 2). Between 6/2014 and 11/2016, nylon mesh bags filled with 100 ml of ion-exchange resin (Dowex Amberlite IRN150) were used to monitor N leaching from soil. Three bags were placed in six planting beds (Figure 1). Bags were clamped in a circular 10 cm plastic frames to ensure a uniform area through which vertical flow could occur, and buried under the soil layer at a depth of 25 cm, atop the filter fabric covering the drainage layer. Ion exchange resin bags were replaced every 6 weeks and 20 ml of the resin from each bag was extracted with 150 ml 2 M KCl and analyzed in the same fashion as bulk deposition samples described above. Concentrations in resin extracts were converted to fluxes by accounting for the total volume of KCl used to extract samples (150 ml), the cross-sectional area of the plastic frame (10 cm diameter), and the amount of time the resin bags were in the field.
N in Vegetables
Over the 2014, 2015, and 2016 growing seasons, leaves of the greens mix (leaf lettuce and mustard greens) and arugula were collected from the same 6 planting beds where the resin bags were located (Figure 1), and total nitrogen content of 10–15 mg dried samples were analyzed by Vario El Cube CHNOS Elemental Analyzer at the Cornell Nutrient Analysis Laboratory. The ratio of dry:fresh weight was measured by oven drying the samples at 60°C for 48 h. The mass of N leaving the farm in harvested vegetables were calculated as dry-weight N concentration multiplied by the dry:fresh weight ratio, and the fresh weight from each harvest record (B. Flanner, personal communication). The mass of N of all other crops were estimated as fresh weight from the same harvest record multiplied by the standard fresh-weight N concentrations from National Nutrient Database for Standard Reference by the U.S. Department of Agriculture (USDA, 2016). For each year, the possible error of this estimation was calculated as the estimated N in the non-measured crops, multiplied by the difference between the measured crops' average N concentration and their standard values found in USDA database. Vegetable sampling, the harvest record, and the information retrieved from USDA database focused on the edible portion of each crop which leaves the Grange for sale, while all remaining parts of the crops are composted at the Grange and used to amend the planting beds.
Soil N
Soil samples were collected from the same 6 planting beds where the resin bags were located. Between 8/2014 and 11/2016, soil samples were collected from the total depth of soil layer (25 cm), before and after each growing season, with two additional samples collected in 8/2014 and 8/2015. Total nitrogen content was analyzed by the same method as the aforementioned vegetable sample. Soil N pool was calculated by Equation (1).
where Nsoil = soil N (kg N ha−1)
Nconcentration = soil N concentration (kg N kg soil−1)
Sdensity = average soil bulk density (625 kg m−3)
Sdepth = planting bed depth (0.25 m)
Potential N Mineralization
Between 12/2014 and 11/2016, soil samples to the total depth of soil layer (25 cm) were collected every 6 weeks from each of the 6 beds where the resin bags were located, and each sample was sieved (2 mm mesh) and divided into 6 subsamples. Ten grams from three sub-samples each were extracted with 40 ml 2 M KCl within 24 h of collection and the remaining three soil samples were extracted after a 28 day aerobic incubation at 22–25°C (Drinkwater et al., 1996; Robertson, 1999). Net N mineralization was calculated as the extracted total inorganic N (TIN = NO3-N + NH4-N) on day 28 minus the extracted TIN on day 0, divided by the number of days soils incubated in the lab. TIN concentrations of extracts were analyzed by the colorimetric microplate. Concentrations were converted to mass of N mineralized per unit dry soil after determining gravimetric soil moisture content. Soil moisture was determined by oven drying samples at 60°C for 24 h.
Drainage Output of N
Lateral flow through the drainage layer contains leachate from soil layer and surface runoff from impervious area, and is lost to drains (Figure 2). This drainage N output is the N load to municipal sewer system and surface water, including the East River and Long Island Sound. Drainage samples were collected from all 8 drains, at least once in each sampling period for atmospheric N deposition and N leaching from soil between 2/2015 and 11/2016. Unfiltered drainage samples were analyzed by the colorimetric microplate method. N loss to drainage for each sampling period was calculated as the average concentration multiplied by cumulative drainage volume estimated from direct measurement from a V-notch weir in catchment 8 (see Harada et al., 2018 for details). The average concentration for each sampling period was the daily average concentration averaged over each sampling period.
N drainage output during combined sewer overflows was modeled as a function of water input depth calibrated by the results from brief monitoring campaigns during the 2016 growing season. This monitoring campaigns lasted 14 h following a 5.4 mm irrigation on 6/22, 20.5 mm rainfall on 7/4-5, and 36.1 mm rainfall on 7/29, respectively. Each of these water input depths and drainage N outputs were used as daily water input and drainage N output respectively for the calibration equation using JMP Pro 13.1.0. Drainage samples were taken hourly from 2 drains (catchments 2 and 8) on 6/22, and from all 8 drains on 7/4-5 and 29. Hourly drainage N output was estimated as hourly drainage N concentration multiplied by hourly drainage depth. Drainage sample analysis and drainage depth estimation were conducted with the same method used for annual drainage N output.
N Balance and Efficiency of N Management
Fertilizer N input, N output via vegetable harvest, and N leaching from soil are fluxes occurring in the planting bed area only, whereas atmospheric N deposition and the drainage area for the roof applies to the entire roof, including impervious areas. To allow for comparison between these N fluxes, each flux was expressed both in terms of the area of the planting beds only (the mass of N divided by time and the planting bed area), and in terms of the entire site (the mass of N divided by time and the entire site area) in the annual N balance. For the efficiency of N management, we compared the 2015 and 2016 growing seasons, and fluxes were expressed in terms of the area of the planting beds only, with N leaching from soil treated as the outflow N loss from the production system.
Results
N Balance
Drainage N output was 11X the cumulative bulk deposition (Figure 4), and was 5.4X the estimated total deposition (Table 1; Schwede and Lear, 2014; NADP Total Deposition Science Committee, 2017). This makes the Grange a net N source in the urban environment. The major inputs were fertilizer and atmospheric deposition, but these inputs were offset by N lost via harvest of vegetables and drainage output, and the annual N balance of the entire rooftop was −26 kg N ha−1 y−1 (Figure 4). The annual N balance of the soil layer suggests that equivalent to 83% of N output via harvest was lost from the total N pool of the soil layer over time, including both growing and non-growing seasons. The annual N balance of the drainage layer suggests that 32% of N leaching from soil to the drainage layer was retained in the drainage layer instead of draining from the system. This net effect of the drainage layer combines denitrification, microbial immobilization, and the exchangeable nutrient pool of the drainage layer.
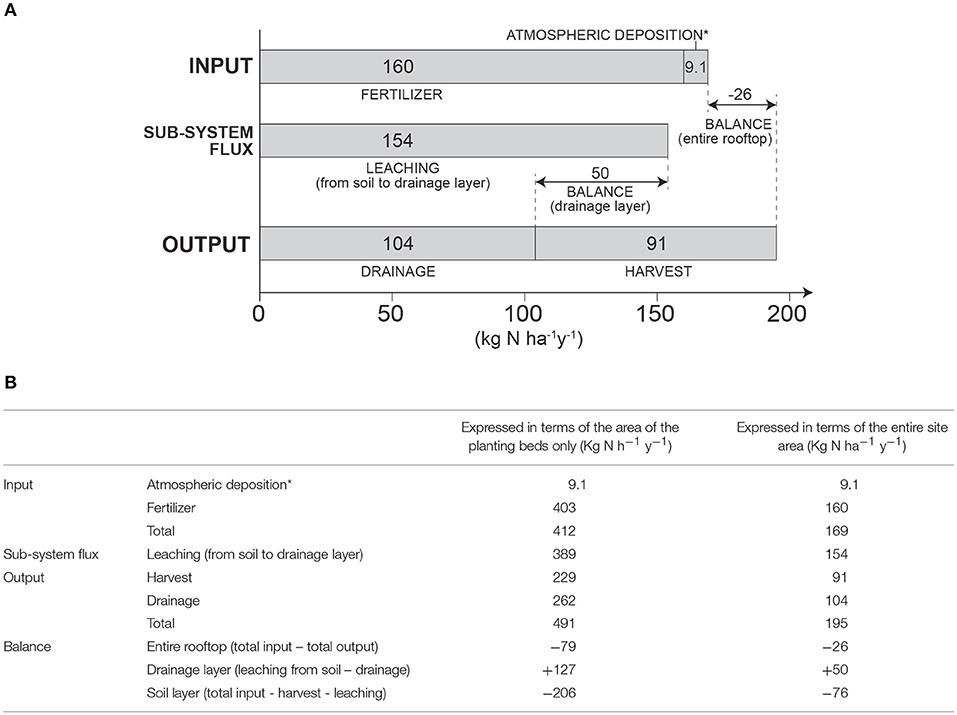
Figure 4. Annual N balance of the Brooklyn Grange Navy Yard farm, NYC, between 11/12/2015 and 11/12/2016. The top figure (A) compares N fluxes expressed in terms of the entire site area. *Bulk collector 1.5 m above the soil surface. The bottom figure (B) shows each flux expressed both in terms of the area of the planting beds only, and in terms of the entire site area.
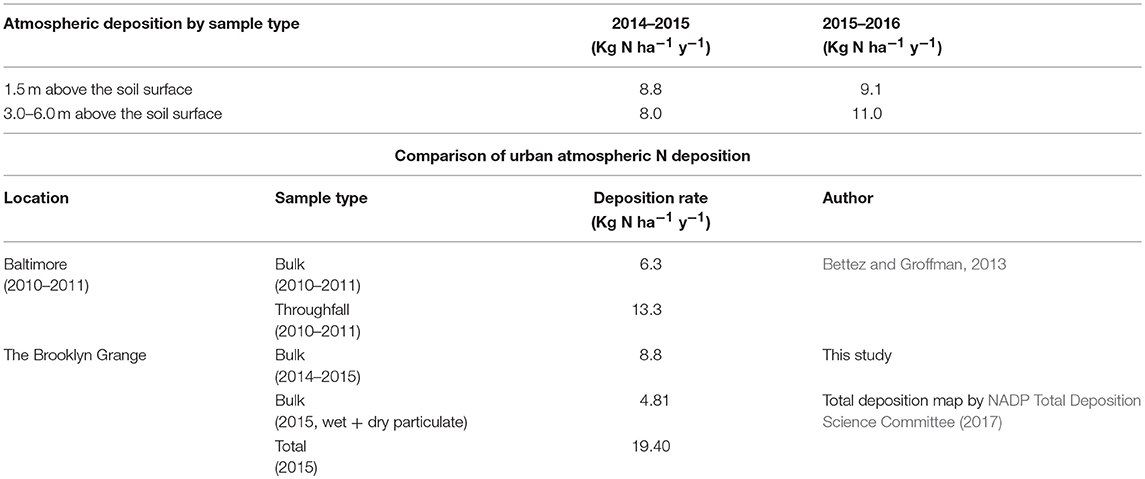
Table 1. Atmospheric N deposition at the Brooklyn Grange Navy Yard farm, NYC, between 11/12/2014 and 11/12/2015, and between 11/12/2015 and 11/12/2016, compared with other studies.
Soil N and N Output in Harvested Vegetables
Over the 3-years study, average soil total N concentration was 7.9 g N kg soil−1, which translates into the total farm N pool of 12,000 kg N ha−1 expressed in terms of the area of the planting beds only, or 4,900 kg N ha−1 expressed in terms of the entire site area. On average, extractable TIN was 48 ± 47 mg N kg−1, or only 0.6% of the total N pool, and PMN was 1.9 mg N kg−1d−1. Over the three growing seasons, a total of 126 kg N was exported from the Grange through the harvest of 35 Mg vegetables in fresh weight (Figure 5). Annual harvest in fresh weight was 11 Mg in 2014 and 2015, respectively, and increased to 13 Mg in 2016. Annual harvest N output was 35 kg N in 2014 and 2015, respectively, and increased to 56 kg N in 2016 (Figure 5). The possible overestimation was 4 ± 5 kg N in 2015, and the possible underestimations were 1 ± 4 and 6 ± 2 kg N in 2014 and 2016, respectively.
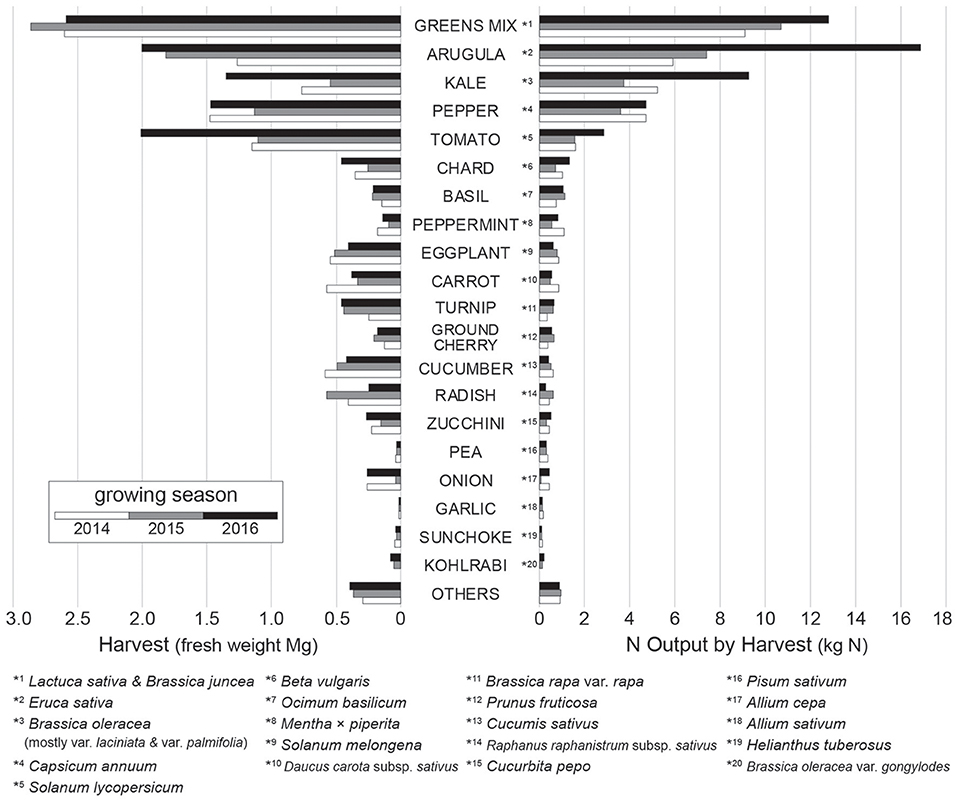
Figure 5. Fresh weight and N content of the harvested vegetables over the 2014, 2015, and 2016 growing seasons at the Brooklyn Grange Navy Yard farm, NYC. Crops are listed in order of the N contents over three growing seasons combined.
Efficiency of N Management
For the production of leafy vegetables during the 226-day growing seasons, the major N supplies were fertilizer, atmospheric deposition, and the extractable soil N, but these N supplies were offset by the harvest and N leaching from soil. The total N balance ranged between +1 kg N ha−1 and −160 kg N ha−1 (Table 2). The total N supply over the 2016 growing season was 1.2X the 2015 growing season, whereas leaching from soil was 1.1X the 2015 growing season. Thus, N supply increased more than N leaching loss. As a result, N supply efficiency increased from 28 to 34%.
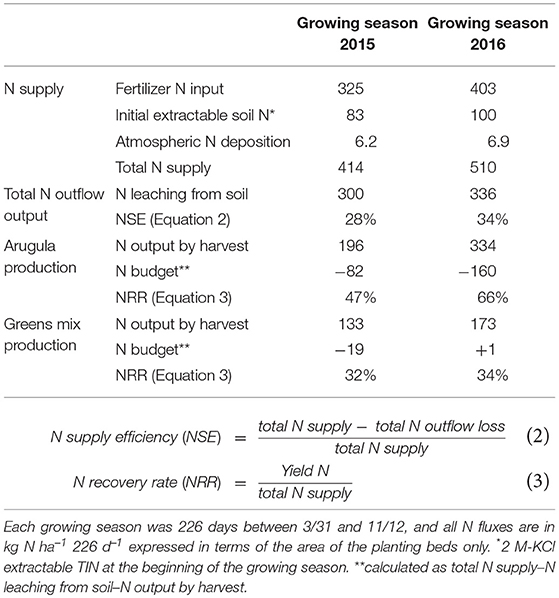
Table 2. Efficiency of N management for leafy vegetable production at the Brooklyn Grange Navy Yard farm, NYC, in the 2015 and 2016 growing seasons.
From the 2015 to 2016 growing seasons, N recovery rate of arugula increased from 47 to 66%, while N recovery rate of greens mix increased only from 32 to 34%, respectively (Table 2). However, fresh-weight yield per unit area over the 2016 growing season was 83 and 97% of the 2015 growing season for arugula and greens mix, respectively. This was due to the dry-weight N concentrations, which increased from 4.5 to 6.4% for arugula, and from 3.5 to 6.2% for greens mix, from the 2015 to 2016 growing seasons, respectively.
Drainage N Output During Combined Sewer Overflows
Daily drainage N output was expressed as a function of daily water input, and calibrated (RMSE = 0.06) by the results from the hourly monitoring campaigns in the 2016 growing season (Figures 6A–I). The model is given by Equation (4).
where Nload = daily drainage N output (kg N ha−1 d−1) expressed in terms of the entire site area; and W = daily total water input (rainfall + irrigation) (mm d−1) expressed in terms of the entire site area. During the 2016 growing season (226 days between 3/31 and 11/12), the cumulative drainage N output estimated by the model (79 kg N ha−1) exceeded the measured N load (77 kg N ha−1) by 3%, and root mean square error (RMSE) between the measured N load and model estimate in each sampling period was 9.6. This result suggests that the model could express the general trend of the Grange's drainage N output over the 2016 growing season.
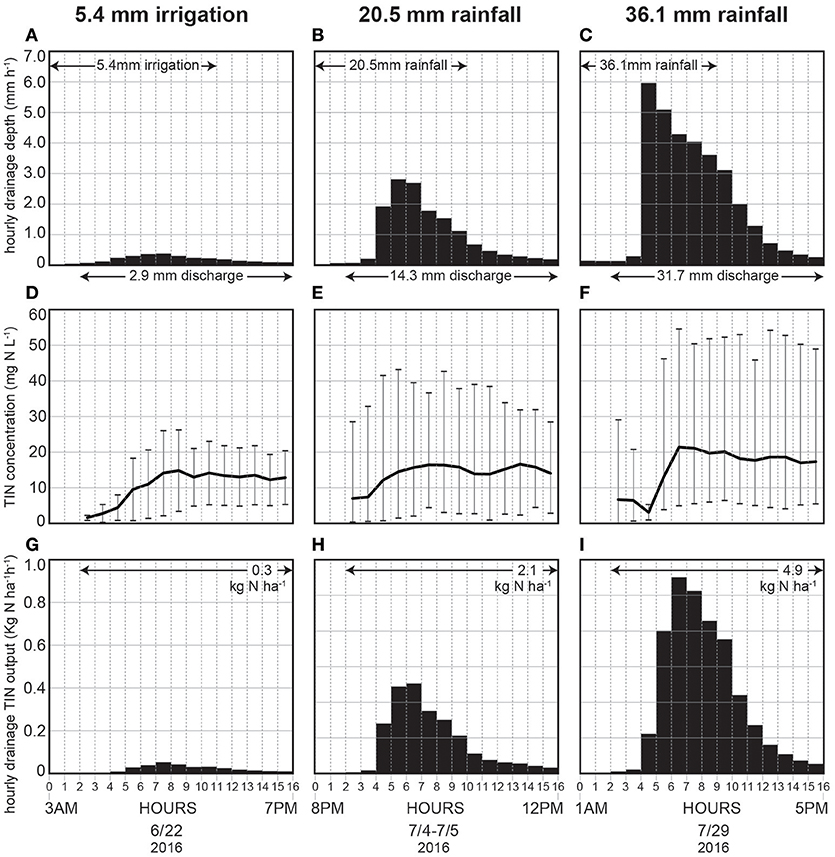
Figure 6. Hourly drainage depth (A–C), TIN concentration (D–F), and drainage TIN output (G–I) during the brief monitoring campaigns on 6/22, 7/4-5, 7/29 in 2016.
A minimum of 0.4 inch (10 mm) daily rainfall can trigger combined sewer overflows from the watershed of Red Hook wastewater treatment plant, which receives N load from the Grange while discharging untreated sewage to the East River and Long Island Sound during combined sewer overflows (ODMHED NYC, 2011; NYC DEP, 2015, 2016). Daily rainfall depth was 10 mm or deeper for 9% (20 days) of the 2016 growing season. For these 20 days, the cumulative daily N load estimated by the model was 67% (51 kg N ha−1) of the cumulative drainage N output over the 2016 growing season, which was likely to be discharged from the Grange to the East River and Long Island Sound during combined sewer overflows.
Discussion
Drainage N Output
The annual drainage output of N (104 kg N ha−1 y−1) was 3.1X that reported Whittinghill et al. (2016), perhaps because fertilizer N input exceeded application rates by 7.3X reported by these authors. For direct comparison with other agricultural systems, drainage N output must be expressed in terms of the area of the planting beds only (262 kg N ha−1y−1), which falls within the range of in-ground intensive vegetable production (Zhao et al., 2010; Min et al., 2012; Zhang et al., 2017).
In non-production green roofs, drainage N output can decrease over time because unused soil mixes imported during construction can have the largest N pool prone to drainage output (Emilsson et al., 2007). However, the effect of site age is inconsistent among the studies of non-production green roofs due to mineralization of organic N and the lack of multi-year observations (Monterusso et al., 2002; Berndtsson et al., 2006; Hathaway et al., 2008; Buffam and Mitchell, 2015; Buffam et al., 2016). Our study site was constructed in 2012, and N leaching continuously increased from 1.2 to 1.8 kg N ha−1 d−1 from the 2014 to 2016 growing seasons, respectively. This could reflect the 2-fold increase of annual fertilizer N input from 2014 to 2016.
Drainage from the Grange is collected by Red Hook wastewater treatment plant, which discharges effluent to the East River and Long Island Sound (NYC DEP, 2007; ODMHED NYC, 2011). In 2016, the Grange's N load to the sewer (0.064 Mg N y−1) was only 0.01% of the N load from the Red Hook wastewater treatment plant (634 Mg N y−1; US EPA, 2017a). While this contribution is miniscule, New York City reportedly has 8599 ha of flat rooftop surface, of which 14% is reported to be suitable for farming (Acks, 2006; Ackerman et al., 2013). If all suitable roofs were used for farms performing like the Grange, the citywide N load from these farms would be 129 Mg N y−1. Even this amounts to only 0.6% of the total citywide N load from all 14 wastewater treatment plants combined (NYC DEP, 2007; US EPA, 2017a).
Urban Atmospheric N Deposition
Between 11/2014 and 11/2015, atmospheric N deposition was only 45% of the estimated total N deposition from the Total Deposition Map (Schwede and Lear, 2014; NADP Total Deposition Science Committee, 2017), perhaps because bulk collectors undersampled cloud and gaseous N deposition. In Baltimore, Bettez and Groffman (2013) report that bulk N deposition was only 47% of throughfall N deposition, which is used as a surrogate for total N deposition in many studies. These findings suggest that cloud and gaseous N deposition could account for over the half of total N deposition in urban environments. In our study, possible N gas emission sources include the Brooklyn Navy Yard Cogeneration Facility (a 286-megawatt gas-fired power plant 300 m west of the site), which released over 32 Mg NH3-N gas annually between 2014 and 2016 (EPA, 2017). Also, Brooklyn/Queens Expressway is 250 m south from the site, and had annual average daily traffic (AADT) of 123,631 cars day−1 in 2015 (NYS DOT, 2015). Historically, atmospheric deposition monitoring stations in the US have been deliberately located away from the urban and industrial emission sources, but there is a growing body of work in urban areas reporting deposition values between 11 and 14.8 kg N ha−1y−1 (Lohse et al., 2008; Bettez and Groffman, 2013; Rao et al., 2014).
N Mineralization
Over the 2016 growing season, TIN represented only 2% of fertilizer N inputs, suggesting that mineralization of organic N contributed much of TIN leaching loss. In the mass balance over the 2016 growing season (Figure 7), the estimated rates of net mineralization were 527 kg N ha−1 226 d−1. Total non-mineralized TIN supply was 116 kg N ha−1, which equals 35% of the 336 kg ha−1 leached from soil. This would indicate that at least 65% of TIN leached from soil came from mineralization during the 2016 growing season. However, the mass balance also shows that over the half of the total TIN supply (non-mineralized TIN + net mineralized N = 644 kg N ha−1) was lost by leaching from soil, suggesting that both the drainage volume and soil N mineralization made important contributions to the N load in leachate. Sources of organic N mineralized over the 2016 growing season could include the ingredients of soil during construction, which was greater than the levels of annual fertilizer N input by two orders of magnitude. However, N leaching increased over the 3 growing seasons, and mineralization of the organic N in fertilizer was more likely to contribute to the N load in the leachate.
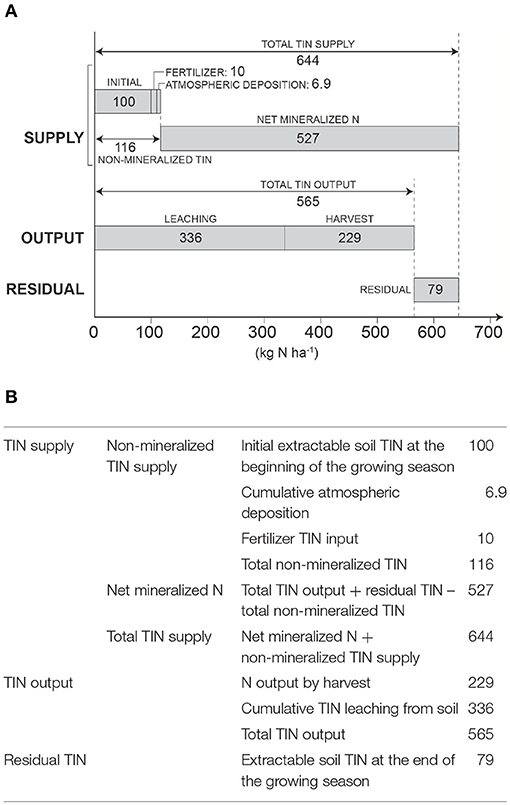
Figure 7. Estimation of net mineralized N over the 2016 growing season (226 days between 3/31 and 11/12). The top figure (A) compares the N values used for the estimation. All values are expressed in terms of the area of the planting beds only. All fluxes are in kg N ha−1 226 d−1, and extractable soil TIN values are in kg N ha−1. The bottom figure (B) summarizes N values used in the estimation.
Implications of Drainage N Concentrations for Environmental Planning
For the Eastern coastal plain including New York City, the U.S. Environmental Protection Agency has set the ambient water quality criteria for total nitrogen concentrations in rivers and streams at 0.71 mg N L−1 and for lakes and reservoirs at 0.32 mg N L−1, but not for salt water (US EPA, 2000, 2001). Because salt water ecosystems can be more N-limited than fresh water ecosystems (see Howarth, 2008b), those criteria could be too permissive for salt water systems like Long Island Sound, yet both the annual average (12.0 ± 6.5 mg NO3-N L−1) and annual flow-weighted average (10.7 mg NO3-N L−1) concentration of the Grange exceed the ambient water quality criteria by an order of magnitude. While N drainage concentrations in rainwater, combined sewer overflows, surface water, and surface runoff from urban environments are equal to or less than the ambient water quality criteria, effluent from wastewater treatment plants and agriculture, including rooftop farms, can exceed these criteria by an order of magnitude or more (Gold et al., 1990; Randall et al., 1997; Steuer et al., 1997; Goulding et al., 2000; Pimentel et al., 2005; Gundersen et al., 2006; Benotti et al., 2007; Bakhsh et al., 2010; Zhao et al., 2010; Likens, 2013; Whittinghill et al., 2016; NADP, 2017; US EPA, 2017a; US NPS, 2017).
The WHO (2004), US EPA (2017b), and the NYS DEC (2017) have set guideline levels for drinking water at 10 mg NO3-N L−1. Because drinking water below this guideline level can still pose human health risks (see Townsend et al., 2003), this guideline level could be too lenient, yet is exceeded by in-ground agriculture and this study. In short, rooftop farming could be similar to in-ground agriculture and wastewater with respect to environmental planning based on drainage N concentrations.
Efficiency of N Management
The N concentrations of leafy vegetables were around or above the target range for optimum yield and quality (arugula: 2.86–3.97%, mustard greens: 2.97–3.85%) reported by Mills and Jones (1996). Also, fertilizer N input, total N supply, N supply efficiency, and N recovery rate increased from the 2015 to 2016 growing seasons for those leafy vegetables, while their fresh-weight yield per unit area decreased (Table 2). These observations suggest that the production of leafy vegetables was not necessarily N-limited in the 2016 growing season, and that increasing just N fertilizer in the future may not effectively increase fresh-weight yield.
Between 11/2015 and 11/2016, the annual N leaching from the planting beds (389 kg N ha−1y−1) was 97% of the N input by fertilizer application (403 kg N ha−1y−1), which suggests a great opportunity for improving the efficiency of N management. Among the studies of in-ground intensive vegetable production, Min et al. (2012) report that while fertilizer N input exceeded application rates at the Grange by 2.7 X, only 25% of this fertilizer N was lost to leaching. This is perhaps because annual depth of drainage water (234.7 mm y−1) was only 25% of the drainage from the Grange (954.0 mm y−1). Another study of in-ground intensive vegetable production by Zhao et al. (2010) report that the fertilizer N input (1480 kg N ha−1y−1) exceeded application rates at the Grange by 3.7X, yet only 24% of this fertilizer N was lost by leaching. Zhao et al. (2010) report annual drainage loss was only 39% of the annual total water input whereas annual drainage depth was 62% of the annual total water input at the Grange. These findings by others suggest that excessive irrigation at the Grange increased the drainage volume and N leaching from soil.
The efficiency of N management could be enhanced by using soil with greater water holding capacity within the range of readily available water (10–100 kPa; Harada et al., 2018). For example, shallow (<15 cm) potting mixes using coconut coir or other organic materials as base materials, could increase water in the effective root zone, while reducing loss to drainage (Harada et al., 2017, 2018). Studies of types, amounts, and timings of fertilizer application and organic amendments could improve N management efficiency of soil-based approach, which could be combined with a system to recycle drainage water, with appropriate measures to control salt accumulation and pathogens. Further empirical studies are needed to determine the cost-benefit relationship of such system vs. hydroponic and controlled-environment systems.
Conclusions
This study reports the N balance and efficiency of N management of the Brooklyn Grange, a large rooftop farm in NYC, USA. We found that the drainage N output to the combined sewer was 11X greater than atmospheric bulk deposition and 5.4X the estimated total deposition, which makes the Grange a net N source in the urban environment. Both the drainage volume and soil N mineralization were the important factors increasing N discharge, because fertilizer N input was dominantly organic N, and much of the mineralized N was lost to drains. In particular, excessive irrigation and the use of soil with low water holding capacity increased the overall drainage N output and drainage N output during combined sewer overflows, while reducing N management efficiency to levels lower than typically found in soil-based vegetable farms. Recycling drainage and using the soil with enhanced water holding capacity could reduce N discharge while maintaining satisfactory yield and quality. Rooftop farming is in its infancy, and there are unprecedented opportunities to develop best management practices in advance to the large-scale implementation.
Author Contributions
YH experimental design, field and laboratory experiments, data analysis, manuscript preparation. TW and RH experimental design, data analysis, manuscript preparation. PT laboratory analysis, data analysis, manuscript preparation. MW, JR-A, and NB experimental design, data analysis.
Conflict of Interest Statement
The authors declare that the research was conducted in the absence of any commercial or financial relationships that could be construed as a potential conflict of interest.
Acknowledgments
This study was supported through the United States Department of Agriculture (USDA) Hatch Grant (1001972), Sustainable Agriculture Research and Education (SARE) Partnership Grant Projects (ONE16-276), and the Toward Sustainability Foundation. YH wishes to express his thanks for stipend and tuition support from the Section of Horticulture, School of Integrative Plant Sciences, Cornell University. We also greatly appreciate the collaborative effort of all members of the Brooklyn Grange and Brooklyn Navy Yard Development Corporation.
References
Ackerman, K., Dahlgren, E., and Xu, X. (2013). Sustainable Urban Agriculture: Confirming Viable Scenarios for Production, Final Report No. 13-07.
Acks, K. (2006). A Framework for Cost-Benefit Analysis of Green Roofs: Initial Estimates. Green Roofs in the Metropolitan Region. Research Report, Columbia University Center for Climate Systems Research.
Ampim, P. A., Sloan, J. J., Cabrera, R. I., Harp, D. A., and Jaber, F. H. (2010). Green roof growing substrates: types, ingredients, composition and properties. J. Environ. Hortic. 28, 244–252. doi: 10.24266/0738-2898-28.4.244 (Accessed June 01, 2016).
Bakhsh, A., Kanwar, R. S., and Baker, J. (2010). N-application methods and precipitation pattern effects on subsurface drainage nitrate losses and crop yields. Water Air Soil Pollut. 212, 65–76. doi: 10.1007/s11270-010-0322-3
Benedetti, A., and Sebastiani, G. (1996). Determination of potentially mineralizable nitrogen in agricultural soil. Biol. Fertil. Soils 21, 114–120. doi: 10.1007/BF00336002
Benotti, M. J., Abbene, M., and Terracciano, S. (2007). Nitrogen Loading in Jamaica Bay, Long Island, New York: Predevelopment to 2005. Scientific Investigations Report 2007–5051, U.S. Department of the Interior and U.S. Geological Survey.
Berndtsson, J. C. (2010). Green roof performance towards management of runoff water quantity and quality: a review. Ecol. Eng. 36, 351–360. doi: 10.1016/j.ecoleng.2009.12.014
Berndtsson, J. C., Emilsson, T., and Bengtsson, L. (2006). The influence of extensive vegetated roofs on runoff water quality. Sci. Total Environ. 355, 48–63. doi: 10.1016/j.scitotenv.2005.02.035
Best, B. B., Swadek, K. R., and Burgess, L. T. (2015). “Soil-based green roofs,” in Green Roof Ecosystems, ed. K. R. Sutton (Cham: Springer International Publishing), 139–174.
Bettez, N. D., and Groffman, P. M. (2013). Nitrogen deposition in and near an urban ecosystem. Environ. Sci. Technol. 47, 6047–6051. doi: 10.1021/es400664b
Buffam, A. P. D. I., and Mitchell, M. E. (2015). “Nutrient cycling in green roof ecosystems,” in Green Roof Ecosystems (Cham: Springer), 107–137.
Buffam, I., Mitchell, M. E., and Durtsche, R. D. (2016). Environmental drivers of seasonal variation in green roof runoff water quality. Ecol. Eng. 91, 506–514. doi: 10.1016/j.ecoleng.2016.02.044
Cameron, K., Di, H., and Moir, J. (2013). Nitrogen losses from the soil/plant system: a review. Ann. Appl. Biol. 162, 145–173. doi: 10.1111/aab.12014
Campbell, J. L., Hornbeck, J. W., Mitchell, M. J., Adams, M. B., Castro, M. S., Driscoll, C. T., et al. (2004). Input-output budgets of inorganic nitrogen for 24 forest watersheds in the northeastern United States: a review. Water Air Soil Pollut. 151, 373–396. doi: 10.1023/B:WATE.0000009908.94219.04
Carter, T., and Fowler, L. (2008). Establishing green roof infrastructure through environmental policy instruments. Environ. Manage. 42, 151–164. doi: 10.1007/s00267-008-9095-5
Congreves, K., and Van Eerd, L. (2015). Nitrogen cycling and management in intensive horticultural systems. Nutr. Cycling Agroecosyst. 102, 299–318. doi: 10.1007/s10705-015-9704-7
Di, H., and Cameron, K. (2002). Nitrate leaching in temperate agroecosystems: sources, factors and mitigating strategies. Nutr. Cycling Agroecosyst. 64, 237–256. doi: 10.1023/A:1021471531188
Doane, T. A., and Horwáth, W. R. (2003). Spectrophotometric determination of nitrate with a single reagent. Anal. Lett. 36, 2713–2722. doi: 10.1081/AL-120024647
Drinkwater, L., Letourneau, D., Workneh, F., Van Bruggen, A., and Shennan, C. (1995). Fundamental differences between conventional and organic tomato agroecosystems in California. Ecol. Appl. 5, 1098–1112. doi: 10.2307/2269357
Drinkwater, L. E., Cambardella, C. A., Reeder, J. D., and Rice, C. W. (1996). “Potentially mineralizable nitrogen as an indicator of biologically active soil nitrogen,” in Methods for Assessing Soil Quality, SSSA Spec. Publ. Vol. 49, eds J. W. Doran and A. J. Jones (Madison, WI: SSSA), 217–229.
Driscoll, C. T., Eger, C. G., Chandler, D. G., Davidson, C. I., Roodsari, B. K., Flynn, C. D., et al. (2015). Green Infrastructure: Lessons from Science and Practice. A publication of the Science Policy Exchange. 32. Available online at: https://science-policy-exchange.org/resources/reports/green-infrastructure-lessons-science-and-practice (Accessed June 01, 2018).
Eksi, M., Rowe, D. B., Fernández-Ca-ero, R., and Cregg, B. M. (2015). Effect of substrate compost percentage on green roof vegetable production. Urban Forest. Urban Green. 14, 315–322. doi: 10.1016/j.ufug.2015.03.006
Emilsson, T., Berndtsson, J. C., Mattsson, J. E., and Rolf, K. (2007). Effect of using conventional and controlled release fertiliser on nutrient runoff from various vegetated roof systems. Ecol. Eng. 29, 260–271. doi: 10.1016/j.ecoleng.2006.01.001
EPA, U. (2017). The Toxics Release Inventory (TRI) Search System. Available online at: https://www.epa.gov/enviro/tri-search (Accessed December 03, 2017).
Eriksen, J., Askegaard, M., and Kristensen, K. (2004). Nitrate leaching from an organic dairy crop rotation: the effects of manure type, nitrogen input and improved crop rotation. Soil Use Manage. 20, 48–54. doi: 10.1111/j.1475-2743.2004.tb00336.x
Fenn, M. E., Poth, M. A., Aber, J. D., Baron, J. S., Bormann, B. T., Johnson, D. W., et al. (1998). Nitrogen excess in North American ecosystems: predisposing factors, ecosystem responses, and management strategies. Ecol. Appl. 8, 706–733. doi: 10.1890/1051-0761(1998)008[0706:NEINAE]2.0.CO;2
Gold, A. J., DeRagon, W. R., Sullivan, W. M., and Lemunyon, J. L. (1990). Nitrate-nitrogen losses to groundwater from rural and suburban land uses. J. Soil Water Conserv. 45, 305–310.
Goldstein, B., Hauschild, M., Fernández, J., and Birkved, M. (2016). Urban versus conventional agriculture, taxonomy of resource profiles: a review. Agronom. Sustain. Dev. 36, 1–19. doi: 10.1007/s13593-015-0348-4
Goulding, K. W. T., Poulton, P. R., Webster, C. P., and Howe, M. T. (2000). Nitrate leaching from the Broadbalk Wheat Experiment, Rothamsted, UK, as influenced by fertilizer and manure inputs and the weather. Soil Use Manage. 16, 244–250. doi: 10.1111/j.1475-2743.2000.tb00203.x
Gregoire, B. G., and Clausen, J. C. (2011). Effect of a modular extensive green roof on stormwater runoff and water quality. Ecol. Eng. 37, 963–969. doi: 10.1016/j.ecoleng.2011.02.004
Grimm, N. B., Faeth, S. H., Golubiewski, N. E., Redman, C. L., Wu, J., Bai, X., et al. (2008). Global change and the ecology of cities. science 319, 756–760. doi: 10.1126/science.1150195
Groffman, P. M., Law, N. L., Belt, K. T., Band, L. E., and Fisher, G. T. (2004). Nitrogen fluxes and retention in urban watershed ecosystems. Ecosystems 7, 393–403. doi: 10.1007/s10021-003-0039-x
Gundersen, P., Schmidt, I. K., and Raulund-Rasmussen, K. (2006). Leaching of nitrate from temperate forests effects of air pollution and forest management. Environ. Rev. 14, 1–57. doi: 10.1139/a05-015
Harada, Y., Whitlow, T. H., Bassuk, N. L., and Russell-Anelli, J. (2017). “Biogeochemistry of Rooftop Farm Soils,” in Urban Soils, eds. R. Lal, B.A. Stewart (Portland, OR: Taylor & Francis Group).
Harada, Y., Whitlow, T. H., Todd Walter, M., Bassuk, N. L., Russell-Anelli, J., and Schindelbeck, R. R. (2018). Hydrology of the Brooklyn Grange, an urban rooftop farm. Urban Ecosyst. doi: 10.1007/s11252-018-0749-7
Hartz, T. (2006). Vegetable production best management practices to minimize nutrient loss. HortTechnol. 16, 398–403. Available online at: http://horttech.ashspublications.org/content/16/3/398.full.pdf+html (Accessed September 24, 2016).
Hathaway, A. M., Hunt, W. F., and Jennings, G. D. (2008). A field study of green roof hydrologic and water quality performance. Transac. ASABE 51, 37–44. doi: 10.13031/2013.24225
Herridge, D. F., Peoples, M. B., and Boddey, R. M. (2008). Global inputs of biological nitrogen fixation in agricultural systems. Plant Soil 311, 1–18. doi: 10.1007/s11104-008-9668-3
Howarth, R. W. (2008). Coastal nitrogen pollution: a review of sources and trends globally and regionally. Harmful Algae 8, 14–20. doi: 10.1016/j.hal.2008.08.015
Howarth, R. W., Anderson, D., Cloern, J., Elfring, C., Hopkinson, C., Lapointe, B., et al. (2000). Nutrient pollution of coastal rivers, bays and seas. Issues Ecol. 7, 1–15. Available online at: https://www.esa.org/esa/wp-content/uploads/2013/03/issue7.pdf (Accessed June 11, 2018).
Howarth, R. W., Marino, R., Swaney, D., and Boyer, E. (2006). “Wastewater and watershed influences on primary productivity and oxygen dynamics in the lower hudson river estuary,” in The Hudson River Estuary, eds J. Levinton and J. Waldman (Cambridge: Cambridge University Press), 121–139. doi: 10.1017/CBO9780511550539.012
Howarth, R. W., Sharpley, A., and Walker, D. (2002). Sources of nutrient pollution to coastal waters in the United States: implications for achieving coastal water quality goals. Estuaries 25, 656–676. doi: 10.1007/BF02804898
Jayasundara, S., Wagner-Riddle, C., Parkin, G., von Bertoldi, P., Warland, J., Kay, B., et al. (2007). Minimizing nitrogen losses from a corn–soybean–winter wheat rotation with best management practices. Nutr. Cycling Agroecosyst. 79, 141–159. doi: 10.1007/s10705-007-9103-9
Kaye, J. P., Groffman, P. M., Grimm, N. B., Baker, L. A., and Pouyat, R. V. (2006). A distinct urban biogeochemistry? Trends Ecol. Evol. 21, 192–199. doi: 10.1016/j.tree.2005.12.006
Kemp, W. M., Boynton, W. R., Adolf, J. E., Boesch, D. F., Boicourt, W. C., Brush, G., et al. (2005). Eutrophication of Chesapeake Bay: historical trends and ecological interactions. Mar. Ecol. Prog. Ser. 303, 1–29. doi: 10.3354/meps303001
Kong, A. Y., Rosenzweig, C., and Arky, J. (2015). Nitrogen dynamics associated with organic and inorganic inputs to substrate commonly used on rooftop farms. HortScience 50, 806–813.
Lohse, K. A., Hope, D., Sponseller, R., Allen, J. O., and Grimm, N. B. (2008). Atmospheric deposition of carbon and nutrients across an arid metropolitan area. Sci. Total Environ. 402, 95–105. doi: 10.1016/j.scitotenv.2008.04.044
Lovell, S. T. (2010). Multifunctional urban agriculture for sustainable land use planning in the United States. Sustainability 2, 2499–2522. doi: 10.3390/su2082499
Lovell, S. T., and Taylor, J. R. (2013). Supplying urban ecosystem services through multifunctional green infrastructure in the United States. Landsc. Ecol. 28, 1447–1463. doi: 10.1007/s10980-013-9912-y
Lundholm, J. T. (2015). The ecology and evolution of constructed ecosystems as green infrastructure. Front. Ecol. Evol. 3:106. doi: 10.3389/fevo.2015.00106
McPhearson, T., Kremer, P., and Hamstead, Z. A. (2013). Mapping ecosystem services in New York City: applying a social–ecological approach in urban vacant land. Ecosyst. Serv. 5, 11–26. doi: 10.1016/j.ecoser.2013.06.005
Mentens, J., Raes, D., and Hermy, M. (2006). Green roofs as a tool for solving the rainwater runoff problem in the urbanized 21st century? Landsc. Urban Plan. 77, 217–226. doi: 10.1016/j.landurbplan.2005.02.010
Mills, H. A., and Jones, J. B. (1996). Plant Analysis Handbook II: A Practical Sampling, Preparation, Analysis, and Interpretation Guide. Athens, GA: Micro-Macro Publishing.
Min, J., Zhang, H., and Shi, W. (2012). Optimizing nitrogen input to reduce nitrate leaching loss in greenhouse vegetable production. Agri. Water Manage. 111, 53–59. doi: 10.1016/j.agwat.2012.05.003
Monterusso, M., Rowe, D., Rugh, C., and Russell, D. (2002). “Runoff water quantity and quality from green roof systems,” in XXVI International Horticultural Congress: Expanding Roles for Horticulture in Improving Human Well-Being and Life Quality 639. Toronto, ON, 369–376.
NADP (2017). National Trend Network Online Database. Available online at: http://nadp.sws.uiuc.edu/data/NTN/ (Accessed August 21, 2017).
NADP Total Deposition Science Committee (2017). Total Deposition Maps. Available online at: http://nadp.sws.uiuc.edu/committees/tdep/tdepmaps/ (Accessed August 21, 2017).
Nixon, S., Ammerman, J., Atkinson, L., Berounsky, V., Billen, G., Boicourt, W., et al. (1996). The fate of nitrogen and phosphorus at the land-sea margin of the North Atlantic Ocean. Biogeochemistry 35, 141–180. doi: 10.1007/BF02179826
NYC DEP (2007). New York City's Wastewater Treatment System. Available online at: http://www.nyc.gov/html/dep/pdf/wwsystem.pdf (Accessed April 01, 2016).
NYC DEP (2015). Combined Sewer Overflow Long Term Control Plan for Gowanus Canal. Capital Project No. WP-169 Long Term Control Plan 2. Available online at: http://www.nyc.gov/html/dep/pdf/cso_long_term_control_plan/gowanus-canal-ltcp-201506.pdf (Accessed August 21, 2016).
NYC DEP (2016). New York City CSO Advisory Web Page. Available online at: http://www.nyc.gov/html/dep/pdf/harbor/cso_waterbody_advisories_about.pdf (Accessed August 21, 2016).
NYC EDC (2013). A Stronger, More Resilient New York: Water and Wastewater. Available online at: https://www1.nyc.gov/assets/sirr/downloads/pdf/Ch_12_WaterWaste_FINAL_singles.pdf (Accessed July 23, 2018).
NYS DEC (2017). 6 CRR-NY 703.5 Title 6. Chapter X Subchapter A. General Article 2 Part 703. Surface Water and Groundwater Quality Standards and Groundwater Effluent Limitations. Available online at: http://www.dec.ny.gov/chemical/23853.html (Accessed August 01, 2017).
NYS DOT (2015). New York State Department of Transportation Traffic Data Viewer. Available online at: https://gis3.dot.ny.gov/html5viewer/?viewer=tdv (Accessed August 01, 2017).
Oberndorfer, E., Lundholm, J., Bass, B., Coffman, R. R., Doshi, H., Dunnett, N., et al. (2007). Green roofs as urban ecosystems: ecological structures, functions, and services. Bioscience 57, 823–833. doi: 10.1641/B571005
ODMHED NYC (2011). Water and Sewer Infrastructure Admirals Row Plaza Environmental Impact Statement CEQR No. 11DME001K. Available online at: https://a002-ceqraccess.nyc.gov/ceqr/ (Accessed August 21, 2016).
Palmer, M., Bernhardt, E., Chornesky, E., Collins, S., Dobson, A., Duke, C., et al. (2004). Ecology for a crowded planet. Science 304, 1251–1252. doi: 10.1126/science.1095780
Pärn, J., Pinay, G., and Mander, Ü. (2012). Indicators of nutrients transport from agricultural catchments under temperate climate: a review. Ecol. Indic. 22, 4–15. doi: 10.1016/j.ecolind.2011.10.002
Pataki, D. E., Carreiro, M. M., Cherrier, J., Grulke, N. E., Jennings, V., Pincetl, S., et al. (2011). Coupling biogeochemical cycles in urban environments: ecosystem services, green solutions, and misconceptions. Front. Ecol. Environ. 9:220. doi: 10.1890/090220
Pimentel, D., Hepperly, P., Hanson, J., Douds, D., and Seidel, R. (2005). Environmental, energetic, and economic comparisons of organic and conventional farming systems. Bioscience 55, 573–582. doi: 10.1641/0006-3568(2005)055[0573:EEAECO]2.0.CO;2
Poudel, D., Horwath, W., Lanini, W., Temple, S., and Van Bruggen, A. (2002). Comparison of soil N availability and leaching potential, crop yields and weeds in organic, low-input and conventional farming systems in northern California. Agric. Ecosyst. Environ. 90, 125–137. doi: 10.1016/S0167-8809(01)00196-7
Rabalais, N. N., Turner, R. E., and Wiseman, W. J. Jr. (2002). Gulf of Mexico hypoxia, AKA “The dead zone.” Annu. Rev. Ecol. Syst. 235–263. doi: 10.1146/annurev.ecolsys.33.010802.150513
Randall, G., Huggins, D., Russelle, M., Fuchs, D., Nelson, W., and Anderson, J. (1997). Nitrate losses through subsurface tile drainage in conservation reserve program, alfalfa, and row crop systems. J. Environ. Qual. 26, 1240–1247. doi: 10.2134/jeq1997.00472425002600050007x
Rao, P., Hutyra, L. R., Raciti, S. M., and Templer, P. H. (2014). Atmospheric nitrogen inputs and losses along an urbanization gradient from Boston to Harvard Forest, MA. Biogeochemistry 121, 229–245. doi: 10.1007/s10533-013-9861-1
Robertson, G. P. (1999). Standard Soil Methods for Long-Term Ecological Research. New York, NY: Oxford University Press.
Rowe, D. B. (2011). Green roofs as a means of pollution abatement. Environ. Pollut. 159, 2100–2110. doi: 10.1016/j.envpol.2010.10.029
Scharenbroch, B. C., Lloyd, J. E., and Johnson-Maynard, J. L. (2005). Distinguishing urban soils with physical, chemical, and biological properties. Pedobiologia 49, 283–296. doi: 10.1016/j.pedobi.2004.12.002
Schwede, D. B., and Lear, G. G. (2014). A novel hybrid approach for estimating total deposition in the United States. Atmos. Environ. 92, 207–220. doi: 10.1016/j.atmosenv.2014.04.008
Shock, C. C., and Wang, F. X. (2011). Soil water tension, a powerful measurement for productivity and stewardship. HortScience 46, 178–185. Available online at: http://hortsci.ashspublications.org/content/46/2/178.full (Accessed September 24, 2016).
Sims, G., Ellsworth, T., and Mulvaney, R. (1995). Microscale determination of inorganic nitrogen in water and soil extracts. Commun. Soil Sci. Plant Anal. 26, 303–316. doi: 10.1080/00103629509369298
Skyland USA LLC (2015). Rooflite Intensive Ag Product Specification. Available online at: http://www.rooflitesoil.com/products/intensive-ag (Accessed June 01, 2016).
Specht, K., Siebert, R., Hartmann, I., Freisinger, U. B., Sawicka, M., Werner, A., et al. (2014). Urban agriculture of the future: an overview of sustainability aspects of food production in and on buildings. Agric. Human Values 31, 33–51. doi: 10.1007/s10460-013-9448-4
Steuer, J., Selbig, W., Hornewer, N., and Prey, J. (1997). “Sources of Contamination in an Urban Basin in Marquette, Michigan and an Analysis of Concentrations, Loads, and Data Quality. Marquette, MI: US Geological Survey.
Syswerda, S., Basso, B., Hamilton, S., Tausig, J., and Robertson, G. (2012). Long-term nitrate loss along an agricultural intensity gradient in the Upper Midwest USA. Agric. Ecosyst. Environ. 149, 10–19. doi: 10.1016/j.agee.2011.12.007
Szlavecz, K., Placella, S. A., Pouyat, R. V., Groffman, P. M., Csuzdi, C., and Yesilonis, I. (2006). Invasive earthworm species and nitrogen cycling in remnant forest patches. Appl. Soil Ecol. 32, 54–62. doi: 10.1016/j.apsoil.2005.01.006
Thomaier, S., Specht, K., Henckel, D., Dierich, A., Siebert, R., Freisinger, U. B., et al. (2015). Farming in and on urban buildings: present practice and specific novelties of Zero-Acreage Farming (ZFarming). Renew. Agric. Food Syst. 30, 43–54. doi: 10.1017/S1742170514000143
Townsend, A. R., Howarth, R. W., Bazzaz, F. A., Booth, M. S., Cleveland, C. C., Collinge, S. K., et al. (2003). Human health effects of a changing global nitrogen cycle. Front. Ecol. Environ. 1, 240–246. doi: 10.1890/1540-9295(2003)001[0240:HHEOAC]2.0.CO;2
US EPA (2000). Ambient Water Quality Criteria Recommendations for Rivers and Streams in Nutrient Ecoregion XIV, Office of Water 4304, EPA 822-B-00-022. Available online at: https://www.epa.gov/sites/production/files/documents/rivers14.pdf (Accessed March 20, 2018).
US EPA (2001). Ambient Water Quality Criteria Recommendations for Lakes and Reservoirs in Nutrient Ecoregion XIV, Office of Water 4304, EPA 822-B-01-011. Available online at: https://www.epa.gov/sites/production/files/documents/lakes14.pdf (Accessed March 20, 2018).
US EPA (2004). Report to Congress on Impacts and Control of Combined Sewer Overflows and Sanitary Sewer Overflows (full report). Available online at: https://www.epa.gov/sites/production/files/2015-10/documents/csossortc2004_full.pdf (Accessed July 23, 2018).
US EPA (2017a). Enforcement and Compliance History Online Website. Available online at: https://echo.epa.gov/ (Accessed December 6, 2017).
US EPA (2017b). National Primary Drinking Water Regulations. US EPA. Available online at: https://www.epa.gov/ground-water-and-drinking-water/national-primary-drinking-water-regulations (Accessed August 01, 2016).
US NPS (2017). Jamaica Bay Water Quality Data from The NPS-USGS Water Quality Partnership Program. Available online at: https://www.epa.gov/waterdata/water-quality-data-wqx (Water Quality Data Warehouse, Accessed December 6, 2017).
USDA (2016). National Nutrient Database for Standard Reference. Available online at: https://ndb.nal.usda.gov/ndb/ (Accessed August 01, 2016).
Van Mechelen, C., Dutoit, T., and Hermy, M. (2015). Adapting green roof irrigation practices for a sustainable future: a review. Sustain. Cities Soc. 19, 74–90. doi: 10.1016/j.scs.2015.07.007
Wainger, L. A. (2012). Opportunities for reducing total maximum daily load (TMDL) compliance costs: lessons from the Chesapeake Bay. Environ. Sci. Technol. 46, 9256–9265. doi: 10.1021/es300540k
Whittinghill, L., and Starry, O. (2016). “Up on the roof: considerations for food production on rooftops.” in Sowing Seeds in the City: Ecosystem and Municipal Services, eds. S. Brown, K. McIvor and E. Hodges Snyder (Dordrecht: Springer Netherlands), 325–338.
Whittinghill, L. J., Hsueh, D., Culligan, P., and Plunz, R. (2016). Stormwater performance of a full scale rooftop farm: runoff water quality. Ecol. Eng. 91, 195–206. doi: 10.1016/j.ecoleng.2016.01.047
Whittinghill, L. J., Rowe, D. B., Andresen, J. A., and Cregg, B. M. (2015). Comparison of stormwater runoff from sedum, native prairie, and vegetable producing green roofs. Urban Ecosyst. 18, 13–29. doi: 10.1007/s11252-014-0386-8
WHO (2004). Guidelines for Drinking-Water Quality: Recommendations. Geneva: World Health Organization.
Zhang, B., Li, Q., Cao, J., Zhang, C., Song, Z., Zhang, F., et al. (2017). Reducing nitrogen leaching in a subtropical vegetable system. Agric. Ecosyst. Environ. 241, 133–141. doi: 10.1016/j.agee.2017.03.006
Keywords: ecosystem services, rooftop farming, stormwater management, nutrient runoff, atmospheric deposition, green roof, urban sustainability, urban agriculture
Citation: Harada Y, Whitlow TH, Templer PH, Howarth RW, Walter MT, Bassuk NL and Russell-Anelli J (2018) Nitrogen Biogeochemistry of an Urban Rooftop Farm. Front. Ecol. Evol. 6:153. doi: 10.3389/fevo.2018.00153
Received: 17 June 2018; Accepted: 13 September 2018;
Published: 11 October 2018.
Edited by:
Joseph P. McFadden, University of California, Santa Barbara, United StatesReviewed by:
Rüdiger Grote, Karlsruher Institut für Technologie (KIT), GermanyKatalin Szlavecz, Johns Hopkins University, United States
Copyright © 2018 Harada, Whitlow, Templer, Howarth, Walter, Bassuk and Russell-Anelli. This is an open-access article distributed under the terms of the Creative Commons Attribution License (CC BY). The use, distribution or reproduction in other forums is permitted, provided the original author(s) and the copyright owner(s) are credited and that the original publication in this journal is cited, in accordance with accepted academic practice. No use, distribution or reproduction is permitted which does not comply with these terms.
*Correspondence: Yoshiki Harada, eWg1MzVAY29ybmVsbC5lZHU=