- 1Departament de Biologia Evolutiva, Ecologia i Ciències Ambientals, Institut de Recerca de la Biodiversitat, Universitat de Barcelona, Barcelona, Spain
- 2MIVEGEC Research Unit, UMR 5290, Centre IRD, Centre National de la Recherche Scientifique-IRD-University of Montpellier, Montpellier, France
- 3Estación Biológica de Doñana, Consejo Superior de Investigaciones Científicas, Sevilla, Spain
- 4Zoological Institute, Russian Academy of Sciences, Saint Petersburg, Russia
Feather mites are useful models for studying speciation due to their high diversity and strong degree of host specialization. However, studies to date have focused on the evolution of higher-level mite taxa while much hidden diversity likely occurs at the level of host genera and species. In this study, we examined the diversity and evolution of feather mites infesting six sympatric seabird species from six genera, breeding in the Cape Verde archipelago. We report 32 feather mite morphospecies categorized into 10 genera and three families, of which nine correspond to new, undescribed species. Molecular data corroborated morphological species descriptions, except for two morphologically-cryptic, but genetically distinct mite lineages related to Zachvatkinia oceanodromae and Laminalloptes simplex. Using these communities, we then applied a co-structure approach to test the contribution of ectosymbiont and host factors in driving feather mite evolution. Most seabird species hosted specific and unique feather mite species, even under sympatric conditions, and in general, feather mite species exhibited strong host-driven genetic structure. However, patterns of genetic differentiation were variable. That is, some mite species are more generalist than others and mite lineages/haplotypes can be shared by related seabird species. Interestingly, host-specific mites (e.g., Zachvatkinia spp.) tend to display much higher intra-specific diversity compared to more generalist mites (e.g., Microspalax and Plicatalloptes spp.). We discuss ectosymbiont and host life-history traits that might generate these patterns, such as host dispersal and breeding behavior and/or mite spatial and trophic specialization. Our findings highlight both the vast and largely unrecognized diversity of avian feather mites on seabirds, and the intrinsic complexity of the ecological processes underlying the evolution of these ectosymbionts.
Introduction
By definition, parasites and symbionts depend almost entirely on their host for survival and transmission, and thus have evolved intricate adaptations to optimize their life cycle (de Meeûs et al., 1998; Criscione et al., 2005). The degree of host dependency is thought to determine the specificity of the interaction, and we expect to find higher species diversity and stronger patterns of host specialization with increasingly intimate interactions (Poulin and Morand, 2000; Mouillot et al., 2006). This specificity may be due to limited opportunities for dispersal and colonization of new hosts (Clayton et al., 1992; Johnson et al., 2002; Whiteman et al., 2004) or may be determined by specific adaptations to host structures (e.g., feather, hair, immune responses), such that parasites/symbionts are incapable of establishing, surviving and/or reproducing on foreign hosts (Tompkins and Clayton, 1999; Reed et al., 2000).
Comparative studies of parasite co-structures have been shown to be useful in elucidating the proximal factors underlying variation in host-specificity and patterns of parasite/symbiont genetic structure (Johnson et al., 2002; Criscione, 2008; Mazé-Guilmo et al., 2016). This approach consists in comparing population demographic or genetic structures between two or more interacting species: a parasite/symbiont inhabiting multiple host species, or multiple parasite/symbiont species on a single host. Studies of this type have previously been used to improve our understanding of avian ectoparasite diversity (Johnson et al., 2002). However, multiple host and parasite/symbiont life-history traits can influence the specificity of an interaction and the resulting population structure of the parasite/symbiont (i.e., dispersal and drift, host ecology and behavior, symbiont life-cycle complexity and demography) (Blouin et al., 1995; McCoy et al., 2003; Criscione and Blouin, 2004; Bruyndonckx et al., 2009; Mazé-Guilmo et al., 2016). This can lead to conflicting predictions on patterns of structure. In this context, communities of ecologically similar and geographically overlapping host species provide an ideal framework to investigate the proximate factors involved in the divergent evolution of their parasites and symbionts. This scenario also offers the opportunity to test parasite/symbiont structure at hierarchical spatial scales (among host individuals, populations and communities), and make predictions on selection and adaptation processes (McCoy et al., 2001). Not surprisingly, few studies have used this multi-species and multi-scale approach (but see Johnson et al., 2002; Rivera-Parra et al., 2015).
Feather mites (Acari: Astigmata: Analgoidea and Pterolichoidea) are particularly useful models to study divergence and speciation processes. They are common obligate ectosymbionts of birds, with approximately 2,500 species described from extant avian orders (Gaud and Atyeo, 1996; Proctor, 2003; Mironov and Proctor, 2008). Feather mites cannot survive in the off-host environment, and have evolved diverse habitat and trophic specializations to the host environment (Dabert and Mironov, 1999; Doña et al., 2015a; Stefan et al., 2015). This strong dependency has led to the “paradigm” that feather mites are highly host-specific, and co-evolved with their bird hosts. However, previous studies reported varying degrees of co-phylogenetic patterns among mite groups, calling this paradigm into question (Gaud and Atyeo, 1996; Proctor, 2003; Doña et al., 2017a,b). That is, some feather mite species appear restricted to one or a few closely-related host species, others are associated with a wide range of hosts from different genera, and there have even been a couple of records of mite species infesting hosts from different avian families and orders (Doña et al., 2017b). One of the main difficulties in evaluating specificity in feather mite-host associations is the potential presence of cryptic diversity. Indeed, the morphological identification of feather mites is difficult. Immature stages of many taxa and females of closely-related species are often indistinguishable, while male morphological characterization is a laborious task even for experienced taxonomists (Doña et al., 2015b). Molecular studies hold much promise for documenting and understanding diversity in this group, but are still relatively scarce (but see Dabert et al., 2001, 2015; Klimov and OConnor, 2008; Knowles and Klimov, 2011; Štefka et al., 2011; Doña et al., 2015b).
In feather mites, divergence might be affected by either host-related factors; feather structure and dynamics (i.e., moult), defenses (i.e., preening), and/or ectosymbiont life-history traits; dispersal abilities, population size, generation time (Table 1, Proctor, 2003; Pap et al., 2005; Jovani et al., 2006; Clayton et al., 2010; Fernández-González et al., 2015). Due to the dependency of feather mites on host, as their main environment, and the assumed predominant role of vertical transmission, host factors could act as important selective forces promoting the specialization of mite traits. For example, in our previous study, we showed that competition has likely led to within-host spatial and trophic niche partitioning in two co-occurring seabird mite species (M. brevipes and Z. ovata), which in turn correlates with mite-specific morphological traits (Stefan et al., 2015). These adaptations have been hypothesized to shape mite genetic structure and divergence (Gaud and Atyeo, 1996; Dabert and Mironov, 1999; Proctor and Owens, 2000; Proctor, 2003). However, comparative studies examining multiple feather mite species and considering various host and spatial factors are currently lacking.
In the present study, we explore the diversity and genetic structure of feather mite species inhabiting the community of procellariform seabirds of the Cape Verde Islands. These colonial seabirds represent excellent hosts for investigating divergence processes in feather mites. Seabirds are highly pelagic birds that breed in large colonies on remote oceanic islands. These colonies represent spatially discrete and hierarchical habitats, with extremely high host densities. Most seabird species show strong interannual fidelity and natal philopatry to their breeding sites, features that can favor parasite specialization and host specificity (Brooke, 2004; McCoy et al., 2013). However, seabirds are also long distance migrants and frequently breed sympatrically in mixed species colonies, which in turn can promote dispersal and host switching in their ectosymbionts.
The specific aims of our study were: (1) to characterize the diversity of feather mites inhabiting the community of seabirds breeding in the Cape Verde Archipelago; (2) to compare genetic structure among feather mite species using different hosts in sympatric and allopatric breeding conditions, and (3) to evaluate the role of different host (breeding and nesting behavior) and mite (dispersal ability and within host habitat use) factors in generating these patterns.
Materials and Methods
Study Species and Feather Mite Sampling
The Cape Verde Archipelago is located in the mid-Atlantic Ocean (16°N 24°W) approximately 570 km off the west coast of Africa. Mite sampling was performed on six seabird species, five from the order Procellariiformes (Cape Verde shearwater, Calonectris edwardsii; Boyd's shearwater, Puffinus boydi; Bulwer's petrel, Bulweria bulwerii; band-rumped storm petrel, Hydrobates castro; Cape Verde petrel, Pterodroma feae) and one from the order Phaethontiformes (the red-billed tropicbird, Phaethon aethereus), breeding on five islands and islets of the archipelago: Raso, Ilhéu Cima, Ilhéu Grande, Curral Velho and Fogo (Figure 1). All sampled islands harbor multiple seabird species, except Fogo, which is inhabited by a single procellariiform species. From 2004 to 2012, feather mites were collected from captured adult birds using the dust-ruffling method (Walther and Clayton, 1997) and stored in absolute ethanol for subsequent morphological identification and molecular analyses. A total of 453 birds (C. edwardsii: 46, P. boydi: 60, B. bulwerii: 83, P. feae: 76, H. castro: 173 and P. aethereus: 15) were sampled; details on the geographic locations and number of individuals sampled per locality and host species are shown in Figure 1. Main life-history characteristics of the feather mite genera and seabird hosts are detailed in Table 2.
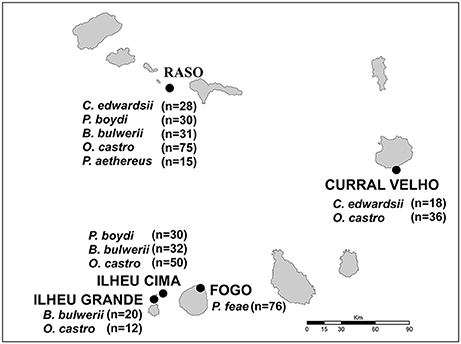
Figure 1. Map of the Cape Verde Archipelago showing the five islands where feather mites were sampled. The name of seabird species and the number of dust-ruffling samples examined per host species and locality are shown in boxes.
Morphological Identifications
Initial screening of feather mites used morphological criteria to characterize the feather mite community composition on a host. Mites were cleared in lactic acid for 24 h and mounted on microscope slides in PVA medium (BioQuip Products, Rancho Dominguez, California). The slides were cured on a slidewarmer at 40°C for 4 days and then examined using a Leica DM 5000B light microscope with differential interference contrast (DIC) illumination. Mites were identified using the identification keys for bonnetellines (Mironov, 1989a,b, 2000), xolalgids (Dabert and Ehrnsberger, 1991; Mironov and Palma, 2006) and alloptids (Peterson and Atyeo, 1968; Peterson, 1971; Atyeo and Gaud, 1991; Mironov, 1996; Mironov and Stefan, 2016). Feather mite specimens of each identified morphospecies are deposited in the Zoological Institute of the Russian Academy of Sciences, Saint Petersburg, Russia (ZISP) and in the Museum of the Faculty of Biology (Centro de Recursos de Biodiversidad Animal), University of Barcelona, Spain.
DNA Isolation, Amplification and Sequencing
When possible, five specimens of each feather mite morphospecies per seabird host and island and from different host individuals were selected for the molecular analyses. Total numbers of mites were: 50 specimens from the genus Zachvatkinia (five species), 37 from the genus Microspalax (four species), 66 from the genus Brephosceles (nine species), 19 from the genus Plicatalloptes (one species), 13 from the genus Laminalloptes (three species) and 5 specimens from Onychalloptes (one species) (Table S1). The mite genera: Rhinozachvatkinia, Promegninia, Ingrassia and Opetiopoda, were not included in the molecular analyses due either to low sample size and/or failure to obtain DNA sequences.
DNA extractions were performed on each individual mite using a DNeasy Tissue Kit (Qiagen, Valencia, CA, USA) and the non-destructive method described by Dabert et al. (2008). After DNA extraction, mite exoskeletons were recovered, mounted on microslides, re-identified morphologically and kept as reference vouchers. Partial sequences of two mitochondrial genes, 12S rRNA (12S) and 16S rRNA (16S), were amplified for each feather mite using the following primers: SR-J-14199 (5′-TACTATGTTACGACTTAT-3′) and SR-N-14594 (5′-AAACTAGGATTAGATACCC-3′) for the 12S gene (Kambhampati and Smith, 1995); and 16SA2 (5′-TTTAATTGGTTACTTGTATGAATG-3′) and 16C2 (5′-CGCTGTTATCCCTAGAGTAT-3′) for the 16S gene (Dabert et al., 2001). Amplifications were carried out in a total volume of 25 μl containing 2.5 μl 10x reaction buffer with 15 mM MgCl2 (Roche Diagnostics), 2 mM MgCl2, 0.2 mM of each dNTP, 0.4 μM of each primer, 1.25 U Taq DNA polymerase (Roche Diagnostics) and 2 μl of DNA template. Amplification conditions for the 12S rRNA gene consisted of an initial step of 2 min at 94°C, followed by 10 cycles of denaturation at 94°C for 30 sec, annealing at 40°C for 30 sec, and extension at 68°C for 1 min, and 35 cycles of denaturation at 94°C for 30 sec, annealing at 43°C for 30 sec, and extension at 68°C for 1 min, with a final step of 5 min at 72°C. For the 16S rRNA gene, the PCR conditions followed Black and Piesman (1994). Amplification products were separated by electrophoresis in a 2% agarose gel and visualized under UV light. Successful amplifications were sent for sequencing to Beckman Coulter Genomics (France). Only mite specimens with complete sequence information for the two mitochondrial genes were included in the analyses. DNA sequences were checked and edited using Bioedit version 7.0.5.3 (Hall, 1999) and all variable sites were confirmed by visual inspection of the chromatograms. Sequences were aligned for each gene independently using MAFFT version 7, with default parameters.
Molecular Diversity and Species Delimitation
We tested for neutrality for each gene of each mite genus using Tajima's D test included in DNASP v.5 (Librado and Rozas, 2009). Genetic statistics (number of polymorphic sites, number of haplotypes, nucleotide and haplotype diversity) for each gene and morphospecies/genetic lineage were assessed using DNASP v.5. Mean genetic distances within and between morphospecies/genetic lineages were calculated with MEGA 4.1 using Kimura's 2-parameter (K2P) distance model (Tamura et al., 2007).
Following the initial morphological characterization of all mites and to control for the potential presence of cryptic species, we conducted two different species delimitation analyses on the molecular data. First, we applied the general mixed Yule-coalescent (GMYC) method (Pons et al., 2006). This method infers an ultrametric tree and, based on this topology, attempts to detect transitions in the tree where branching patterns switch from being attributed to speciation (one lineage per species) to being attributed to an intra-species coalescent process (multiple lineages per species). The GMYC analysis was conducted using the “splits” package (Species Limits by Threshold Statistics) in R version 3.0.3. The single threshold method was applied in order to find the Maximum Likelihood solution of the GMYC model. The ultrametric tree was generated using BEAST v.1.6.2 (Drummond and Rambaut, 2007) after removing identical sequences. In order to choose the most appropriate tree prior, we considered different parameter combinations: Yule vs. coalescent tree priors which refers to the model used to express the expected branching pattern on the tree, and strict clock vs. relaxed uncorrelated lognormal clock, which is compared to determine the model of molecular evolution. Secondly, for each mite genus, we inferred haplotype networks using the concatenated sequences of the two mitochondrial genes to which we applied the statistical parsimony algorithm implemented in TCS version 1.21 (Clement et al., 2000). This method partitions the data into independent haplotype sub-networks connected by changes that are non-homoplastic with a 95% probability (Templeton, 2001). Although this threshold does not necessarily correspond to species boundaries, the algorithm has been shown to be useful in separating independent lineages, which in most cases correspond to good species (Hart and Sunday, 2007).
Genetic Structure
To examine the genetic structure of the mites, we first mapped host and geographic information onto the mitochondrial haplotype network of each mite species. In general, each host species harbored its own set of feather mite species, except for three mite species (M. brevipes, B. puffini, and Plicatalloptes sp.1) shared by the Cape Verde and Boyd's shearwaters (see results). For these mite species, we evaluated the partitioning of genetic variation within and between feather mite populations (in this case at host species and host individual levels), using one-way analyses of molecular variance (AMOVA) in ARLEQUIN version 3.5 and determined significance using 10,000 permutations. We also calculated overall and pairwise estimates of FST in ARLEQUIN (Excoffier and Lischer, 2010) and then examined genetic structure over geographic space. We ran AMOVA analyses, partitioning genetic variation among islands and among individuals within islands for each mite species that occurred on at least two islands.
Results
Morphological Diversity of Feather Mites
Thirty-two feather mite species belonging to ten genera and three families were found on the six studied seabird species based only on morphological criteria: Zachvatkinia, Rhinozachvatkinia, Promegninia (Avenzoariidae), Microspalax, Brephosceles, Plicatalloptes, Laminalloptes, Onychalloptes (Alloptidae), Ingrassia, and Opetiopoda (Xolalgidae) (Table 3). Many of these genera, such as Zachvatkinia, Microspalax, Brephosceles and Ingrassia, co-occurred in multiple seabird species in the Cape Verde Islands, whereas the genera Rhinozachvatkinia, Promegninia, Opetiopoda, Plicatalloptes, Laminalloptes, and Onychalloptes were restricted to one or two host species (Table 3). The procellariiform seabirds harbored distinct acarofauna compared to the phaethontiform species, being Ingrassia the only mite genus shared by the two avian orders.
Nine of the recorded mite species corresponded to new undescribed species, and six were recently described species (Rhinozachvatkinia calonectris, Promegninia calonectris, Promegninia bulweriae, Opetiopoda bulweriae, Ingrassia calonectris, and Ingrassia micronota) (Stefan et al., 2013, 2014; Mironov et al., 2015). The Cape Verde shearwater and Bulwer's petrel hosted the greatest number of feather mite species (eight each) with the highest number of new species (three for the Cape Verde shearwater and four for Bulwer's petrel), whereas the Cape Verde petrel harbored the lowest mite richness with only three species found. Except for three feather mite species (M. brevipes, B. puffini, and Plicatalloptes sp.1) which were shared by Cape Verde and Boyd's shearwaters, each host species harbored its own feather mite assembly (Table 3).
Molecular Diversity and Structure of Feather Mites
In total, 190 sequences for each of the two mitochondrial genes were obtained for 23 out of the 32 morphologically distinct species (Table S1).
The number of haplotypes per mite species ranged from 1 to 11 for the 12S gene and from 1 to 6 for the 16S gene. Haplotype diversity ranged from 0 to 1 for both genes, while nucleotide diversity ranged from 0 to 0.0051 once cryptic lineages were accounted for (Table S2). No deviation from neutrality was found for any mite genus or gene (12S and 16S) (all P > 0.05), except for the 12S data of Plicatalloptes sp.1, which may indicate purifying selection or population expansion (Tajima's D = −1.87; P < 0.05). A Tajima's test could not be computed for the 16S data of Onychalloptes due to a lack of polymorphism.
Sequence divergence between feather mite morphospecies within each genus are shown in Table S3. Mean divergences ranged from 3.7% (Brephosceles) to 24.9% (Laminalloptes) for the 12S, and from 1.9% (Brephosceles) to 32.2% (Laminalloptes) for the 16S gene (Table S3). Mean genetic distances between mite individuals within morphospecies ranged from 0 to 0.7% for all species from all genera and for both genes, except for Z. oceanodromae (1%, SE = 0.002 for 12S gene and 1.5%, SE = 0.006 for 16S gene; bootstrap 1,000 replicates) and L. simplex (2.8%, SE = 0.005 for 12S gene and 4.4%, SE = 0.010 for 16S gene, respectively; bootstrap 1,000 replicates), indicating a high degree of intra-specific diversity and the presence of putative distinct genetic lineages within these two morphospecies (Table S2). Sequence divergence between the genetic lineages of Z. oceanodromae A and Z. oceanodromae B was 6.6% for the 12S gene and 7% for the 16S gene, whereas between L. simplex A and L. simplex B it was 11.2 and 16.4%, respectively for the two genes.
The GMYC analysis suggested the presence of 26 putative mite species within Cape Verde Islands when considering the Yule-strict clock combination [NGMYC = 29 (CI 28–30), LGMYC = 732.49; where N = number of species including the 3 outgroups and L = likelihood of GMYC model]. For the coalescent strict clock, the GMYC analysis resulted in 25 putative mite species [NGMYC = 28 (CI 28–30], LGMYC = 738.21) (Figure S1). For both models (Yule and coalescent), the GMYC method indicated that there are two species within Z. oceanodromae and two within L. simplex. The only disagreement between the two models used to delimit species concerned M. brevipes, which was partitioned into two distinct species corresponding to different host species (Cape Verde and Boyd's shearwaters) for the Yule-strict clock combination. Neither the coalescent strict clock nor the TCS network analysis (see below) recognized these as separate lineages. In favor of this split, the Bayes factor of the Yule prior with a strict clock gave a slightly better fit than a coalescent prior (Yule: −5755.31, coalescent: −5741.33).
The mtDNA networks revealed high levels of haplotypic diversity, with 102 distinct haplotypes, based on the concatenated 12S and 16S sequences. These sequences grouped into 25 different networks, which agrees with the 25 genetic lineages identified by the GMYC approach. Furthermore, we a high degree of correspondence between morphological and molecular data (Figures 2A–F). Again, the only exceptions were Z. oceanodromae from band-rumped storm petrels and L. simplex from red-billed tropicbirds for which two different sub-networks were found (Z. oceanodromae A and B; Figure 2A and L. simplex A and B; Figure 2E).
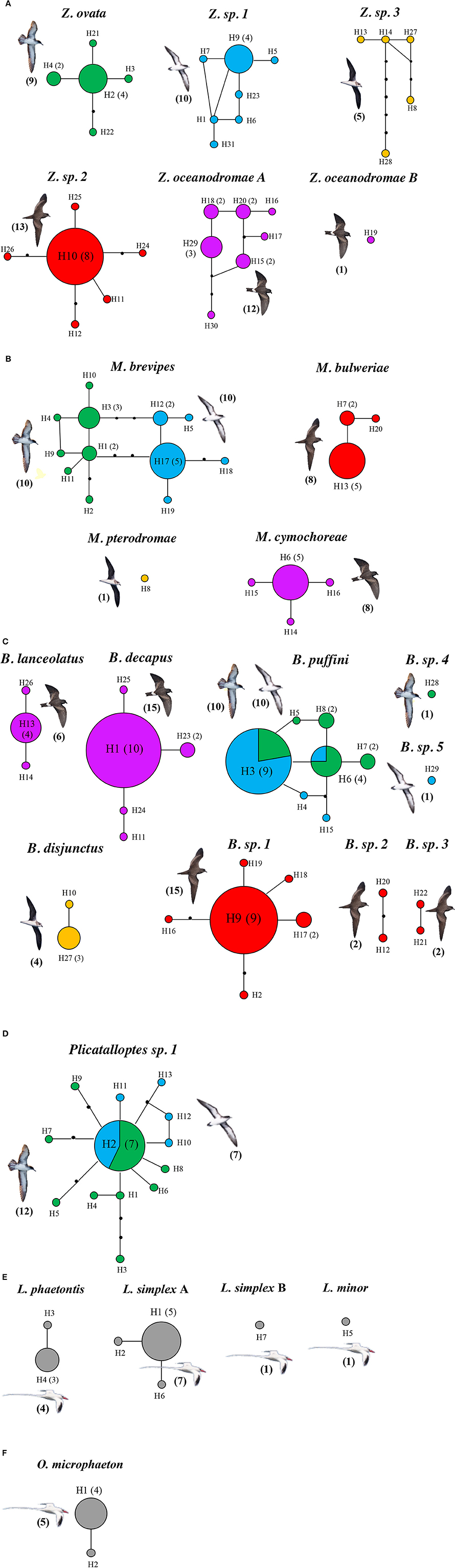
Figure 2. Statistical parsimony haplotype networks for seabird feather mites Zachvatkinia (A), Microspalax (B), Brephosceles (C), Plicatalloptes (D), Laminalloptes (E), and Onychalloptes (F) from the Cape Verde Islands based on the concatenation of two mitochondrial genes (12S and 16S). Haplotypes are colored according to the hosts harboring the mites (green – C. edwardsii, blue – P. boydi, red – B. bulwerii, purple – O. castro, orange – P. feae, and gray – P. aethereus). Circle sizes are proportional to the number of individuals possessing each haplotype, indicated in brackets; when no number is indicated, only one mite individual presented this haplotype. Black dots represent mutational steps. The number of mite individuals analyzed per each bird species is shown in brackets.
As outlined above, clear patterns of host-associated genetic structure were apparent in most of the feather mite genera analyzed. Twenty out of the 23 mite species examined showed a one-host one-mite species pattern (Figure 2). M. brevipes, B. puffini, and Plicatalloptes sp.1 were shared by two related hosts, Cape Verde and Boyd's shearwaters (Figure 2). The two shearwater hosts share breeding habitats, but differ in their breeding phenology (Table 2). In accordance with this temporal isolation, AMOVA results showed significant host-associated genetic differentiation for M. brevipes (Φ = 0.669, P = 0) and B. puffini (Φ = 0.225, P = 0.010) (Table 4). This was not the case for Plicatalloptes sp.1 (Φ = 0.048, P = 0.069), in which genetic structure between host species was not statistically significant. In contrast, when testing for the effect of geography, the TCS parsimony networks showed only weak spatial genetic structure in the feather mite species that occurred on more than one island population (Figures 3A–D). Indeed, almost all mite species shared haplotypes among islands. Furthermore, the AMOVA analyses with island as a grouping factor supported this observation, with only Zachvatkinia sp.1 and M. brevipes showing a tendency for island-related genetic structure (Table S4).
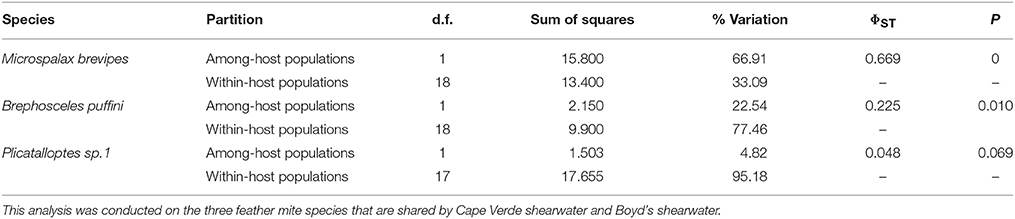
Table 4. Analysis of molecular variance (AMOVA) on mitochondrial haplotypes partitioned by seabird host.
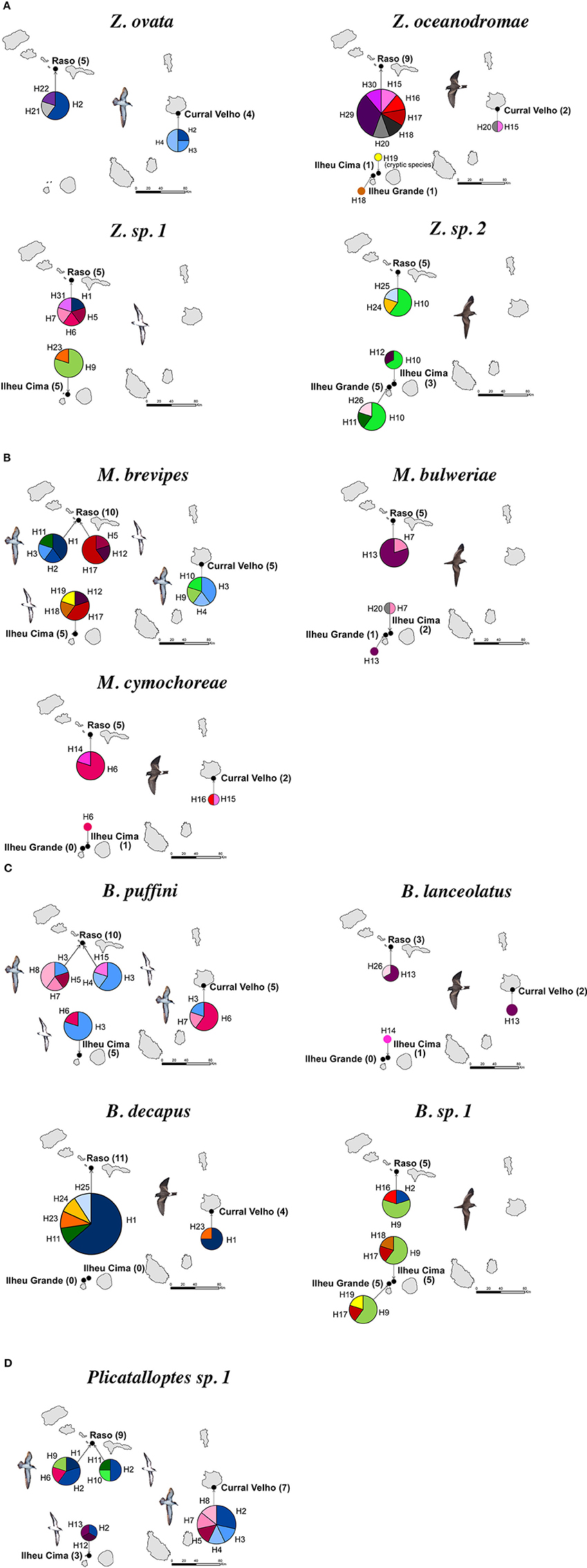
Figure 3. Geographic distribution of haplotypes for seabird feather mites Zachvatkinia (A), Microspalax (B), Brephosceles (C), and Plicatalloptes (D) from the Cape Verde Islands based on the concatenation of two mitochondrial genes (12S and 16S). Each color represents a different haplotype and the size of each haplotype is proportional to the number of individuals having that haplotype. The number of mite individuals analyzed in each locality is shown in brackets.
Discussion
In studying the diversity of feather mites on procellariiform and phaenthontiform seabirds from Cape Verde, we uncovered a unique mite fauna composed of 32 morphologically distinct species belonging to ten genera, of which nine are new, undescribed species. All seabird species examined in this study hosted at least three feather mite species, with Cape Verde shearwaters and Bulwer's petrels presenting the richest mite communities (eight species each). This number is in line with mite richness documented in other groups of birds, including seabirds (Fitzpatrick and Threlfall, 1977; Doña et al., 2016). For instance, other procellariiform seabirds are known to harbor more than one mite species: Puffinus gravis (nine), Oceanodroma leucorhoa (eight), and Puffinus tenuirostris (seven) (Bourgeois and Threlfall, 1979; Doña et al., 2016).
The host-feather mite associations found in the present study are largely in agreement with previous records in the literature (Peterson, 1971; Mironov, 1989a; Atyeo and Gaud, 1991; Stefan et al., 2013; Mironov and Stefan, 2016), although some inconsistencies were detected. First, Z. ovata was previously reported from Calonectris diomedea borealis and different species of Puffinus shearwaters (Mironov, 1989a). Our study clearly shows that these two host genera harbor distinct species of Zachvatkinia. Another disagreement concerns Brephosceles puffini, previously known only from Puffinus hosts (Peterson, 1971); the present study revealed that B. puffini also inhabits Calonectris shearwaters. This does not seem to be a spurious result given that B. puffini specimens were found in various localities in which the two seabird genera (Calonectris and Puffinus) are not sympatric.
One of the difficulties in measuring diversity and in studying host-parasite associations is the presence of cryptic species. Cryptic speciation appears to be common in ectosymbionts, such as lice (Malenke et al., 2009) and mites (Miller et al., 2013; Doña et al., 2015b). The strong host-associated adaptive pressures on these ectosymbionts can promote convergence in morphology, rendering traditional morphological methods insufficient for species identification (Doña et al., 2015b). To address this problem, we integrated morphological and molecular data. We found that, in general, morphospecies correlated well with genetic lineages, with the exception of two species (Zachvatkinia oceanodromae, Laminalloptes simplex). Similar examples of cases of hidden diversity were found in the literature in avian skin (Whiteman et al., 2006), nasal and feather mites (Doña et al., 2015b). In the case of Z. oceanodromae, some small morphological differences could be detected on secondary examination (i.e., slightly shorter incision in the interlobar membrane; S. Mironov personal observation). Therefore, in order to confirm the existence of the two cryptic species found in the present study, more specimens belonging to each species should be collected and redescribed using multilocus genetic data and more quantitative morphological methods (e.g., geometric morphometrics).
Hosts are the most important driving force generating diversity in symbionts due to the selective pressure imposed by the host environment, i.e. immune and behavioral responses (Magalhães et al., 2007). This selection can result in host adaptation, which in turn, can promote specialization and diversification (Gandon and Michalakis, 2002; Lajeunesse and Forbes, 2002; Malenke et al., 2009). In agreement with the hypothesis of host-driven diversification, this study revealed that most seabird species hosted specific and unique mite species, even under sympatry. When mite species were shared, it was between phylogenetically related seabird host taxa (“clade-limited switches”) (Krasnov et al., 2010; Poulin, 2010; Poulin et al., 2011; Braga et al., 2015). For example, Zachvatkinia, Microspalax, Brephosceles and Plicatalloptes, all occurred on multiple host species, but with evident host-associated genetic structure that conforms with the high degree of trophic, spatial and morphological specialization reported in previous studies (Dabert and Mironov, 1999; Dabert et al., 2015; Stefan et al., 2015). Our findings are also in agreement with previous co-phylogenetic studies reporting that host switching is infrequent and likely plays a relatively minor role in the diversification of these ectosymbionts (Doña et al., 2016, 2017b).
Despite the high level of host-specificity, we observed differences in host-driven feather mite genetic structuring. That is, Zachvatkinia appear highly genetically structured by the host. In contrast, M. brevipes and B. puffini occur in two related shearwater species but with low host-associated genetic divergence. More extreme is the case of Plicatalloptes sp.1 which showed no genetic differentiation among mites inhabiting each of the two host species. Accumulating evidence suggests that multiple parasite and host life-history traits are important in determining the adaptative dynamics and population structure of parasites (Mazé-Guilmo et al., 2016). In this context, studying multiple host-symbiont/parasite associations, where hosts and symbionts/parasites differ in key characteristics such as dispersal and behavior, can provide insight on the factors that affect their microevolutionary dynamics. In lice, differences in specific habitat use within the host body has been shown to alter patterns of among-host dispersal, with body lice having reduced dispersal potential compared to wing lice (Johnson et al., 2002). In the case of feather mites, ecological characteristics linked to differential dispersal abilities between wing and body mites are not congruent with host-associated patterns of structure. Zachvatkinia and Microspalax are large vane-dwelling wing mites with heavily sclerotized bodies that occupy exposed areas along the ventral surfaces of flight feathers (Stefan et al., 2015), features that should favor dispersal. In contrast, Brephosceles and Plicatalloptes are small bodied mites with weakly sclerotized bodies that occupy more protected areas, wing coverts and soft body feathers, and are less mobile (Peterson, 1971; Bourgeois and Threlfall, 1979; Dabert et al., 2015; L. Stefan personal observations). These incoherencies suggest that successful host-switching in this group may depend more on host behavior and ecology than mite life histories per se.
Due to high host-specificity, host dispersal is generally assumed to drive parasite dispersal, such that their genetic structure is expected to conform to that of their hosts (McCoy et al., 2003; Barrett et al., 2008). However, empirical studies show contrasting results in relation to the degree of co-structure; some parasites mirroring host spatial genetic structuring whereas others appear completely undifferentiated (Mazé-Guilmo et al., 2016). Dispersal opportunities in otherwise highly host-specific mite lineages may arise by the fact that phylogenetically related seabird host species breed sympatrically and may even share nest sites, promoting host switching (i.e., Cape Verde and Boyd's shearwaters). However, in the case of the shearwaters in Cape Verde, although they share habitat, they are often temporally isolated during the breeding season. Temporal segregation should “a priori” prevent host-switching given that feather mites are not able to live for long periods outside of the host body (Dubinin, 1951; Proctor, 2003; Doña et al., 2017c). It could be that feather mites survive in molted feathers in the nest substrate for relatively long periods of time, but this explanation seems unlikely given the escape behavior of feather mites during molting (Jovani and Serrano, 2001). That is, it has been shown that feather mites tend to avoid the feathers destined to be molted and leave these feathers shortly before they are dropped (Pap et al., 2005).
In contrast to strong host-associated structure, we found little geographic differentiation between mites of different islands. Direct physical contact among hosts of the same species could occur during mating and chick rearing throughout the breeding period. Mite dispersal between host populations could also be favored by juvenile birds that prospect among breeding colonies, or adult birds that change their breeding locality (Boulinier et al., 2016), or even by phoresy (Harbison et al., 2009). This is supported by previous population genetic studies of the seabird hosts that show weak genetic structuring at local geographic scales and high between island population gene flow (Gómez-Díaz et al., 2006, 2009; Friesen et al., 2007). Regardless of the specific mechanism of dispersal, the weak structure found at relatively large geographic scales suggest that mite dispersal regularly occurs among host populations and occasionally between certain seabird host species, i.e., host switch. This supports the hypothesis that host-associated selection, rather than simple isolation, is likely responsible for the strong patterns of host-associated divergence in these ectosymbionts. Variation among some mite species in genetic diversity and geographic structure may then arise through interspecific differences in generation times and population sizes that lead, in turn, to a variable impact of genetic drift (Johnson et al., 2003). Regular population bottlenecks have been proposed to explain strong structure in contact-transmitted wing mites of social bats (Bruyndonckx et al., 2009). Unfortunately, with the genetic markers at hand and the limited knowledge on the biology of seabird mites, the drift hypothesis is difficult to test in our system. At present, we only have mite abundance data on individual hosts for two species, M. brevipes and Z. ovata, and both species show similar infestation levels (Stefan et al., 2015). Mitochondrial DNA is typically useful to resolve taxonomic uncertainties, but records few traces of contemporary events. Future studies employing more powerful SNP or microsatellite markers, combined with temporal samples on the same bird host will be needed to explore these alternatives more in-depth.
Overall, this study highlights the rich and diverse fauna of feather mites exploiting seabirds. Our morphological and molecular data have revealed the presence of nine new species and suggest the existence of at least two putative cryptic species. Our findings also reinforce the hypothesis that host specialization is a major driver of parasite/symbiont diversification with infrequent host-switching between related seabird host species. Different degrees of host-associated genetic structure among mite species may reflect different transmission probabilities and/or genetic drift effects linked to mite life-history traits and population dynamics. Detailed examination of specific parasite/symbiont traits associated with host specificity combined with field-transplantation experiments, and temporal population genetic studies are now called for to test the relative roles of isolation and adaptation in generating mite diversity.
Availability of Data and Materials
Sequence data is available at GenBank: Accession nos. KX372354-KX372371; MH077590-MH077951
Ethics Statement
Bird captures and mite sampling were performed in accordance with good animal practices as defined by current European legislation and under permission from the government authorities of Cape Verde.
Author Contributions
EG-D, LS, JG-S, and KM conceived and designed the study. EG-D and JG-S collected data. LS performed the laboratory work. LS performed data analysis with input from EG-D, SM, and KM. EG-D, JG-S, and KM contributed to the interpretation of the results. EG-D, LS, and KM wrote the manuscript with input from JG-S and SM. EG-D, JG-S, and KM supervised the study. KM and JG-S provided infrastructures and funds to conduct the study. All the authors revised the manuscript and approved the final version.
Conflict of Interest Statement
The authors declare that the research was conducted in the absence of any commercial or financial relationships that could be construed as a potential conflict of interest.
Acknowledgments
We thank T. Militão, R. Ramos, P. Rodrigues, J.M. de los Reyes, P. Lopez., L. F. López-Jurado, E. Vendrell, S. Martins, A. Rendall, M. Brooke and H. Dinis for their help during fieldwork at different stages and mite sample collection and Valérie Noël for molecular assistance. Permits to capture and examine live procellariiform birds were issued by Direcção Geral do Ambiente and the Instituto Nacional de Investigação e Desenvolvimento Agrário from Cape Verde. The present investigation was performed during the postgraduate project of LMS supported by the University of Barcelona. EG-D was supported by JdC and RyC contracts from the MINECO, Spain. Financial support was provided by CGL2009-11278/BOS from the Ministerio de Ciencia e Innovación (Spain) and Fondos FEDER for LMS, by French ANR project ESPEVEC (ANR-13-BSV7-0018) and the CNRS, France for KM and by a Marie Curie Reintegration Grant to EG-D (ERG-2010-276838).
Supplementary Material
The Supplementary Material for this article can be found online at: https://www.frontiersin.org/articles/10.3389/fevo.2018.00097/full#supplementary-material
References
Atyeo, W. T., and Gaud, J. (1991). Microspalacinae, a new subfamily of the feather mite family Alloptidae Gaud (Acarina, Analgoidea). Folia Parasitol. 38, 327–343.
Barrett, L. G., Thrall, P. H., Burdon, J. J., and Linde, C. C. (2008). Life history determines genetic structure and evolutionary potential of host-parasite interactions. Trends Ecol. Evol. 23, 678–685. doi: 10.1016/j.tree.2008.06.017
Black, IV. W. C., and Piesman, J. (1994). Phylogeny of hard- andsoft-tick taxa (Acari: Ixodida) based on mitochondrial 16S rDNA sequences. Proc. Natl. Acad. Sci. 91, 10034–10038. doi: 10.1073/pnas.91.21.10034
Blouin, M. S., Yowell, C. A., Courtney, C. H., and Dame, J. B. (1995). Host movement and the genetic structure of populations of parasitic nematodes. Genetics 141, 1007–1014.
Boulinier, T., Kada, S., Ponchon, A., Dupraz, M., Dietrich, M., Gamble, A., et al. (2016). Migration, prospecting, dispersal? What host movement matters for infectious agent circulation? Integr. Comp. Biol. 56, 330–342. doi: 10.1093/icb/icw015
Bourgeois, C. E., and Threlfall, W. (1979). Parasites of the greater shearwater (Puffinus gravis) from Newfoundland, Canada. Can. J. Zool. 57, 1355–1357. doi: 10.1139/z79-176
Braga, M. P., Razzolini, E., and Boeger, W. A. (2015). Drivers of parasite sharing among Neotropical freshwater fishes. J. Anim. Ecol. 84, 487–497. doi: 10.1111/1365-2656.12298
Bruyndonckx, N., Dubey, S., Ruedi, M., and Christe, P. (2009). Molecular cophylogenetic relationships between European bats and their ectoparasitic mites (Acari, Spinturnicidae). Mol. Phylogenet. Evol. 51, 227–237. doi: 10.1016/j.ympev.2009.02.005
Clayton, D. H., Gregory, R. D., and Price, R. D. (1992). Comparative ecology of Neotropical bird lice (Insecta: Phthiraptera). J. Anim. Ecol. 61, 195–206. doi: 10.2307/5631
Clayton, D. H., Koop, J. A. H., Harbison, C. W., Moyer, B. R., and Bush, S. E. (2010). How birds combat ectoparasites. Open Ornithol. J. 3, 41–71. doi: 10.2174/1874453201003010041
Clement, M., Posada, D., and Crandall, K. A. (2000). TCS: a computer program to estimate gene genealogies. Mol. Ecol. 9, 1657–1660. doi: 10.1046/j.1365-294x.2000.01020.x
Criscione, C. D. (2008). Parasite co-structure: broad and local scale approaches. Parasite 15, 439–443. doi: 10.1051/parasite/2008153439
Criscione, C. D., and Blouin, M. S. (2004). Life cycles shape parasite evolution: comparative population genetics of salmon trematodes. Evolution 58, 198–202. doi: 10.1111/j.0014-3820.2004.tb01587.x
Criscione, C. D., Poulin, R., and Blouin, M. S. (2005). Molecular ecology of parasites: elucidating ecological and microevolutionary processes. Mol. Ecol. 14, 2247–2257. doi: 10.1111/j.1365-294X.2005.02587.x
Dabert, J., Dabert, M., and Mironov, S. V. (2001). Phylogeny of feather mite subfamily Avenzoariinae (Acari: Analgoidea: Avenzoariidae) inferred from combined analyses of molecular and morphological data. Mol. Phylogenet. Evol. 20, 124–135. doi: 10.1006/mpev.2001.0948
Dabert, J., and Ehrnsberger, R. (1991). Zwei neue Federmilben-Arten aus der Gattung Ingrassia Oudemans, 1905 (Analgoidea; Xolalgidae, Ingrassiinae). Osnabrücker Naturw. Mitt. 17, 127–142.
Dabert, J., Ehrnsberger, R., and Dabert, M. (2008). Glaucalges tytonis sp. n. (Analgoidea, Xolalgidae) from the barn owl Tyto alba (Strigiformes, Tytonidae): compiling morphology with DNA barcode data for taxon descriptions in mites (Acari). Zootaxa 1719, 41–52.
Dabert, J., and Mironov, S. V. (1999). Origin and evolution of feather mites (Astigmata). Exp. Appl. Acarol. 23, 437–454. doi: 10.1023/A:1006180705101
Dabert, M., Coulson, S. J., Gwiazdowicz, D. J., Moe, B., Are Hanssen, S., Biersma, E. M., et al. (2015). Differences in speciation progress in feather mites (Analgoidea) inhabiting the same host: the case of Zachvatkinia and Alloptes living on arctic and long-tailed skuas. Exp. Appl. Acarol. 65, 163–179. doi: 10.1007/s10493-014-9856-1
de Meeûs, T., Michalakis, Y., and Renaud, F. (1998). Santa Rosalia revisited: or why are there so many kinds of parasites in “the garden of earthly delights”? Parasitol. Today 14, 10–13.
Doña, J., Diaz-Real, J., Mironov, S., Bazaga, P., Serrano, D., and Jovani, R. (2015b). DNA barcoding and minibarcoding as a powerful tool for feather mite studies. Mol. Ecol. Resour. 5, 1216–1225. doi: 10.1111/1755-0998.12384
Doña, J., Moreno-García, M., Criscione, C. D., Serrano, D., and Jovani, R. (2015a). Species mtDNA genetic diversity explained by infrapopulation size in a host-symbiont system. Ecol. Evol. 5, 5801–5809. doi: 10.1002/ece3.1842
Doña, J., Potti, J., de la Hera, I., Blanco, G., Frías, O., and Jovani, R. (2017c). Vertical transmission in feather mites: insights into its adaptive value. Ecol. Entomol. 42, 492–499. doi: 10.1111/een.12408
Doña, J., Proctor, H., Mironov, S., Serrano, D., and Jovani, R. (2016). Global associations between birds and vane-dwelling feather mites. Ecology 97, 3242. doi: 10.1002/ecy.1528
Doña, J., Proctor, H., Mironov, S., Serrano, D., and Jovani, R. (2017b). Host specificity, infrequent major host switching and the diversification of highly host-specific symbionts: The case of vane-dwelling feather mites. Global Ecol. Biogeogr. 27, 188–198. doi: 10.1111/geb.12680
Doña, J., Sweet, A. D., Johnson, K. P., Serrano, D., Mironov, S., and Jovani, R. (2017a). Cophylogenetic analyses reveal extensive host-shift speciation in a highly specialized and host-specific symbiont system. Mol. Phylogenet. Evol. 115, 190–196. doi: 10.1016/j.ympev.2017.08.005
Drummond, A., and Rambaut, A. (2007). BEAST: Bayesian evolutionary analysis by sampling trees. BMC Evol. Biol. 7:214. doi: 10.1186/1471-2148-7-214
Dubinin, V. B. (1951). Feather mites (Analgesoidea). Part I. Introduction to their study. Fauna USSR 6, 1–363.
Excoffier, L., and Lischer, H. E. L. (2010). Arlequin suite ver 3.5: a new series of programs to perform population genetics analyses under Linux and Windows. Mol. Ecol. Resour. 10, 564–567. doi: 10.1111/j.1755-0998.2010.02847.x
Fernández-González, S., Pérez-Rodríguez, A., de la Hera, I., Proctor, H. C., and Pérez-Tris, J. (2015). Different space preferences and within-host competition promote niche partitioning between symbiotic feather mite species. Int. J. Parasitol. 45, 655–662. doi: 10.1016/j.ijpara.2015.04.003
Fitzpatrick, C., and Threlfall, W. (1977). The ectoparasites of three species of seabirds from Newfoundland, Canada. Can. J. Zool. 55, 1205–1209. doi: 10.1139/z77-158
Friesen, V. L., Smith, A. L., Gómez-Díaz, E., Bolton, M., Furness, R. W., González Solís, J., et al. (2007). Sympatric speciation by allochrony in a seabird. Proc. Natl. Acad. Sci. U.S.A. 104, 18589–18594. doi: 10.1073/pnas.0700446104
Furness, R. W., and Monteiro, L. R. (1995). Red-billed tropic bird Phaethon aethereus in the Azores: first breeding record for Europe. Bull. Br. Orn. Club. 115, 6–8.
Gandon, S., and Michalakis, Y. (2002). Local adaptation, evolutionary potentialand host parasite coevolution: interactions between migration, mutation, population size and generation time. J. Evol. Biol. 15, 451–462. doi: 10.1046/j.1420-9101.2002.00402.x
Gaud, J., and Atyeo, W. T. (1996). Feather mites of the World (Acarina, Astigmata): the supraspecific taxa. Ann. Zool. Wet. 277:1–193 (Part I. Text), 1–436 (Part II. Illustrations).
González-Solís, J., Felicísimo, A., Fox, J. W., Afanasyev, V., Kolbeinsson, Y., and Muñoz, J. (2009). Influence of sea surface winds on shearwater migration detours. Mar. Ecol. Prog. Ser. 391, 221–230. doi: 10.3354/meps08128
Gómez-Díaz, E., González-Solís, J., and Peinado, M. A. (2009). Population structure in a highly pelagic seabird, the Cory's shearwater Calonectris diomedea: an examination of genetics, morphology and ecology. Mar. Ecol. Prog. Ser. 382, 197–209. doi: 10.3354/meps07974
Gómez-Díaz, E., González-Solís, J., Peinado, M. A., and Page, R. D. M. (2006). Phylogeography of the Calonectris shearwaters using molecular and morphometric data. Mol. Phylogenet. Evol. 41, 322–332. doi: 10.1016/j.ympev.2006.05.006
Hall, T. A. (1999). BioEdit: a user-friendly biological sequence alignment editor and analysis program for Windows 95/98/NT. Nucleic Acids Symp. Ser. 41, 95–98.
Harbison, C. W., Jacobsen, M. V., and Clayton, D. H. (2009). A hitchhiker's guide to parasite transmission: the phoretic behaviour of feather lice. Int. J. Parasitol. 39, 569–575. doi: 10.1016/j.ijpara.2008.09.014
Hart, M. W., and Sunday, J. (2007). Things fall apart: biological species form unconnected parsimony networks. Biol. Lett. 3, 509–512. doi: 10.1098/rsbl.2007.0307
Štefka, J., Hoeck, P. E. A., Keller, L. F., and Smith, V. S. (2011). A hitchhikers guide to the Galápagos: co-phylogeography of Galápagos mockingbirds and their parasites. BMC Evol. Biol. 11:284. doi: 10.1186/1471-2148-11-284
Johnson, K. P., Adams, R. J., Page, R. D., and Clayton, D. H. (2003). When do parasites fail to speciate in response to host speciation? Syst. Biol. 52, 37–47. doi: 10.1080/10635150390132704
Johnson, K. P., Williams, B. L., Drown, D. M., Adams, R. J., and Clayton, D. H. (2002). The population genetics of host specificity: genetic differentiation in dove lice (Insecta: Phthiraptera). Mol. Ecol. 11, 25–38. doi: 10.1046/j.0962-1083.2001.01412.x
Jovani, R., and Serrano, D. (2001). Feather mites (Astigmata) avoid moulting wing feathers of passerine birds. Anim. Behav. 62, 723–727. doi: 10.1006/anbe.2001.1814
Jovani, R., Serrano, D., Frías, Ó., and Blanco, G. (2006). Shift in feather mite distribution during the molt of passerines: the case of barn swallows (Hirundo rustica). Can. J. Zool. 84, 729–735. doi: 10.1139/z06-042
Kambhampati, S., and Smith, P. T. (1995). PCR primers for the amplification of four insect mitochondrial gene fragments. Insect Mol. Biol. 4, 233–236. doi: 10.1111/j.1365-2583.1995.tb00028.x
Klimov, P. B., and OConnor, B. M. (2008). Origin and higher-level relationships of psoroptidian mites (Acari: Astigmata: Psoroptidia): Evidence from three nuclear genes. Mol. Phylogenet. Evol. 47, 1135–1156. doi: 10.1016/j.ympev.2007.12.025
Knowles, L. L., and Klimov, P. B. (2011). Estimating phylogenetic relationships despite discordant gene trees across loci: the species tree of a diverse species group of feather mites (Acari: Proctophyllodidae). Parasitology 138, 1750–1759. doi: 10.1017/S003118201100031X
Krasnov, B. R., Mouillot, D., Shenbrot, G. I., Khokhlova, I. S., Vinarski, M. V., Korallo-Vinarskaya, N. P., et al. (2010). Similarity in ectoparasite faunas of Palaearctic rodents as a function of host phylogenetic, geographic or environmental distances: which matters the most? Int. J. Parasitol. 40, 807–817. doi: 10.1016/j.ijpara.2009.12.002
Lajeunesse, M. J., and Forbes, M. R. (2002). Host range and local parasite adaptation. Proc. R. Soc. Lond. B. Biol. Sci. 269, 703–710. doi: 10.1098/rspb.2001.1943
Librado, P., and Rozas, J. (2009). DnaSP v5: a software for comprehensive analysis of DNA polymorphism data. Bioinformatics 25, 1451–1452. doi: 10.1093/bioinformatics/btp187
Magalhães, S., Forbes, M. R., Skoracka, A., Osakabe, M., Chevillon, C., and McCoy, K. D. (2007). Host race formation in the Acari. Exp. Appl. Acarol. 42, 225–238. doi: 10.1007/s10493-007-9091-0
Malenke, J. R., Johnson, K. P., and Clayton, D. H. (2009). Host specialization differentiates cryptic species of feather-feeding lice. Evolution 63, 1427–1438. doi: 10.1111/j.1558-5646.2009.00642.x
Mazé-Guilmo, E., Blanchet, S., McCoy, K. D., and Loot, G. (2016). Host dispersal as the driver of parasite genetic structure: a paradigm lost? Ecol. Lett. 19, 336–347. doi: 10.1111/ele.12564
McCoy, K. D., Boulinier, T., Tirard, C., and Michalakis, Y. (2001). Host specificity of a generalist parasite: genetic evidence of sympatric host races in the seabird tick Ixodes uriae. J. Evol. Biol. 14, 395–405. doi: 10.1046/j.1420-9101.2001.00290.x
McCoy, K. D., Boulinier, T., Tirard, C., and Michalakis, Y. (2003). Host-dependent genetic structure of parasite populations: differential dispersal of seabird tick host races. Evolution 57, 288–296. doi: 10.1111/j.0014-3820.2003.tb00263.x
McCoy, K. D., Léger, E., and Dietrich, M. (2013). Host specialization in ticks and transmission of tick-borne diseases: a review. Front. Cell. Infect. Microbiol. 3:57. doi: 10.3389/fcimb.2013.00057
Militão, T., Dinis, H. A., Zango, L., Calabuig, P., Stefan, L. M., and González-Solís, J. (2017). Population size, breeding biology and on-land threats of Cape Verde petrel (Pterodroma feae) in Fogo Island, Cape Verde. PLoS ONE 12:e0174803. doi: 10.1371/journal.pone.0174803
Miller, A. D., Skoracka, A., Navia, D., de Mendonca, R. S., Szydło, W., Schultz, M. B., et al. (2013). Phylogenetic analyses reveal extensive cryptic speciation and host specialization in an economically important mite taxon. Mol. Phylogenet. Evol. 66, 928–940. doi: 10.1016/j.ympev.2012.11.021
Mironov, S. V. (1989a). A brief review of the feather mites of the genus Zachvatkinia in the USSR (Analgoidea, Avenzoariidae). Parazitol. Sb. 36, 91–115.
Mironov, S. V. (1989b). A new subgenus and three new species of the feather mite genus Zachvatkinia from Procellariiformes. Parazitologia. 23, 309–319.
Mironov, S. V. (1996). On a validity of the genus Plicatalloptes (Acarina: Analgoidea: Alloptidae). Parasitologiia 30, 216–222.
Mironov, S. V. (2000). Seasonal dynamics of the feather mite Monojoubertia microphylla (Astigmata: Analgoidea: Proctophyllodidae) on the chaffinch Fringilla coelebs. Parazitologia. 34, 457–469.
Mironov, S. V., and Palma, R. L. (2006). Two new feather mite species (Acari: Analgoidea) from the Tuamotu Sandpiper Aechmorhynchus parvirostris (Charadriiformes: Scolopacidae). Tuhinga 17, 49–59.
Mironov, S. V., and Proctor, H. C. (2008). The probable association of feather mites of the genus Ingrassia Oudemans, 1905 (Analgoidea: Xolalgidae) with the blue penguin Eudyptula minor (Aves: Sphenisciformes) in Australia. J. Parasitol. 94, 1243–1248. doi: 10.1645/GE-1579.1
Mironov, S. V., and Stefan, L. M. (2013). Redescription of the feather mite species, Zachvatkinia puffini (Buchholz, 1869) (Acariformes: Avenzoariidae), from its type host, the grey petrel Procellaria cinerea (Procellariiformes: Procellariidae). Acarina 21, 27–37.
Mironov, S. V., and Stefan, L. M. (2016). On identification of species in the feather mite genus Laminalloptes Dubinin, 1955 (Acari: Alloptidae). Acarina 24, 77–85. doi: 10.21684/0132-8077.2016.24.1.77.85
Mironov, S. V., Stefan, L. M., and González-Solís, J. (2015). New species of the feather mite genus Promegninia Gaud and Atyeo (Acari: Avenzoariidae) from petrels and shearwaters (Procellariiformes: Procellariidae). Syst. Parasitol. 90, 91–103. doi: 10.1007/s11230-014-9532-1
Monteiro, L. R., and Furness, R. W. (1998). Speciation through temporal segregation of Madeiran storm petrel (Oceanodroma castro) populations in the Azores? Phil. Trans. R. Soc. Lond. B 353, 945–953. doi: 10.1098/rstb.1998.0259
Mouillot, D., Krasnov, B. R., Shenbrot, G. I., Gaston, K. J., and Poulin, R. (2006). Conservatism of host specificity in parasites. Ecography 29, 596–602. doi: 10.1111/j.0906-7590.2006.04507.x
Pap, P. L., Szép, T., Tökölyi, J., and Piper, S. (2005). Habitat preference, escape behavior, and cues used by feather mites to avoid molting wing feathers. Behav. Ecol. 17, 277–284. doi: 10.1093/beheco/arj026
Peterson, P. C. (1971). A revision of the feather mite genus Brephosceles (Proctophyllodidae: Alloptinae). Bull. Univ. Nebr. State Mus. 9, 89–172.
Peterson, P. C., and Atyeo, W. T. (1968). New genera related to the genus Brephosceles Hull, 1934 (Acarina: Proctophyllodidae). Bull. Univ. Nebr. State Mus. 8, 217–236.
Pons, J., Barraclough, T. G., Gomez-Zurita, J., Cardoso, A., Duran, D. P., Hazell, S., et al. (2006). Sequence-based species delimitation for the DNA taxonomy of undescribed insects. Syst. Biol. 55, 595–609. doi: 10.1080/10635150600852011
Poulin, R. (2010). Decay of similarity with host phylogenetic distance in parasite faunas. Parasitology 137, 733–741. doi: 10.1017/S0031182009991491
Poulin, R., Krasnov, B. R., and Mouillot, D. (2011). Host specificity in phylogenetic and geographic space. Trends Parasitol. 27, 355–361. doi: 10.1016/j.pt.2011.05.003
Poulin, R., and Morand, S. (2000). The diversity of parasites. Q. Rev. Biol. 75, 277–293. doi: 10.1086/393500
Proctor, H. C. (2003). Feather mites (Acari: Astigmata): ecology, behavior and evolution. Annu. Rev. Entomol. 48, 185–209. doi: 10.1146/annurev.ento.48.091801.112725
Proctor, H., and Owens, I. (2000). Mites and birds: diversity, parasitism and coevolution. Trends Ecol. Evol. (Amst). 15, 358–364. doi: 10.1016/S0169-5347(00)01924-8
Ramos, R., Ramírez, I., Paiva, V. H., Militão, T., Biscoito, M., Menezes, D., et al. (2016). Global spatial ecology of three closely-related gadfly petrels. Sci. Rep. 6:23447. doi: 10.1038/srep23447
Ramos, R., Sanz, V., Militão, T., Bried, J., Neves, V. C., Biscoito, M., et al. (2015). Leapfrog migration and habitat preferences of a small oceanic seabird, Bulwer's petrel (Bulweria bulwerii). J. Biogeogr. 42, 1651–1664. doi: 10.1111/jbi.12541
Reed, D. L., Allen, S. K., and Hafner, M. S. (2000). Mammal hair diameter as a possible mechanism for host specialization in chewing lice. J. Mammal. 81, 999–1007. doi: 10.1644/1545-1542(2000)081<0999:MHDAAP>2.0.CO;2
Rivera-Parra, J. L., Levin, I. I., Johnson, K. P., and Parker, P. G. (2015). Lineage sorting in multihost parasites: Eidmanniella albescens and Fregatiella aurifasciata on seabirds from the Galapagos Islands. Ecol. Evol. 5, 3264–3271. doi: 10.1002/ece3.1587
Stefan, L. M., Gómez-Díaz, E., Elguero, E., Proctor, H. C., McCoy, K. D., and González-Solís, J. (2015). Niche partitioning of feather mites within a seabird host, Calonectris borealis. PLoS ONE 10:e0144728. doi: 10.1371/journal.pone.0144728
Stefan, L. M., Gómez-Díaz, E., and Mironov, S. V. (2013). Three new species of the feather mite subfamily Ingrassiinae (Acariformes: Xolalgidae) from shearwaters and petrels (Procellariiformes: Procellariidae). Zootaxa 3682, 105–120. doi: 10.11646/zootaxa.3682.1.4
Stefan, L. M., McCoy, K. D., and Mironov, S. V. (2014). A new species of the feather mite genus Rhinozachvatkinia (Acari: Avenzoariidae) from Calonectris shearwaters (Procellariiformes: Procellariidae): integrating morphological descriptions with DNA barcode data. Folia Parasitol. 61, 90–96. doi: 10.14411/fp.2014.009
Tamura, K., Dudley, J., Nei, M., and Kumar, S. (2007). MEGA4: molecular evolutionary genetics analysis (MEGA) software version 4.0. Mol. Biol. Evol. 24, 1596–1599. doi: 10.1093/molbev/msm092
Templeton, A. R. (2001). Using phylogeographic analyses of gene trees to test species status and processes. Mol. Ecol. 10, 779–791. doi: 10.1046/j.1365-294x.2001.01199.x
Tompkins, D. M., and Clayton, D. H. (1999). Host resources govern the specificity of swiftlet lice: size matters. J. Anim. Ecol. 68, 489–500. doi: 10.1046/j.1365-2656.1999.00297.x
Walther, B. A., and Clayton, D. H. (1997). Dust-ruffling: a simple method for quantifying ectoparasite loads of live birds. J. Field. Ornithol. 68, 509–518.
Whiteman, N. K., Sánchez, P., Merkel, J., Klompen, H., and Parker, P. G. (2006). Cryptic host specificity of an avian skin mite (Epidermoptidae) vectored by louseflies (Hippoboscidae) associated with two endemic Galápagos bird species. J. Parasitol. 92, 1218–1228. doi: 10.1645/GE-918R.1
Whiteman, N. K., Santiago-Alarcon, D., Johnson, K. P., and Parker, P. G. (2004). Differences in straggling rates between two genera of dove lice (Insecta: Phthiraptera) reinforce population genetic and cophylogenetic patterns. Int. J. Parasitol. 34, 1113–1119. doi: 10.1016/j.ijpara.2004.06.003
Keywords: host-symbiont systems, cryptic species, co-structure, host-specialization, adaptation, Procellariiformes, morphology, Analgoidea
Citation: Stefan LM, Gómez-Díaz E, Mironov SV, González-Solís J and McCoy KD (2018) “More Than Meets the Eye”: Cryptic Diversity and Contrasting Patterns of Host-Specificity in Feather Mites Inhabiting Seabirds. Front. Ecol. Evol. 6:97. doi: 10.3389/fevo.2018.00097
Received: 17 April 2018; Accepted: 20 June 2018;
Published: 16 July 2018.
Edited by:
Jingchun Li, University of Colorado Boulder, United StatesReviewed by:
Lucia Pita, GEOMAR Helmholtz-Zentrum für Ozeanforschung Kiel, GermanyGillian H. Gile, Arizona State University, United States
Copyright © 2018 Stefan, Gómez-Díaz, Mironov, González-Solís and McCoy. This is an open-access article distributed under the terms of the Creative Commons Attribution License (CC BY). The use, distribution or reproduction in other forums is permitted, provided the original author(s) and the copyright owner(s) are credited and that the original publication in this journal is cited, in accordance with accepted academic practice. No use, distribution or reproduction is permitted which does not comply with these terms.
*Correspondence: Elena Gómez-Díaz, ZWxlbmEuZ29tZXpAY3NpYy5lcw==
†These authors have contributed equally to this work.