- 1Division of Geology, Department of Geography and Geology, University of Turku, Turku, Finland
- 2Industrial Environments and Recycling Unit, Geological Survey of Finland, Kuopio, Finland
Both natural and anthropogenic changes in boreal lakes have been studied utilizing paleolimnological methods, but the spatial variation in the natural conditions of lakes and its connection to geological factors has drawn less attention. Our aims were to examine the spatial distribution of naturally eutrophic lakes on the previously glaciated terrain of central-eastern Finland and the relationship between pre-human disturbance water quality and geological factors related to the basins and their catchments. Furthermore, we studied the pre- to post-human disturbance changes in the diatom assemblages and water quality of 48 lakes (51 sampling sites) across the pre-disturbance phosphorus gradient by using the top-bottom sampling approach and multivariate statistics. According to our results, naturally eutrophic boreal lakes are more common than previously thought, occurring on fine-grained and organic Quaternary landforms, including fine-grained till. Our study emphasizes the importance of the previously overlooked matter of till grain-size variation as a driver behind the spatial variation in the natural trophic states of boreal lakes. The location of a lake in the hydrologic landscape and basin morphology appear to be important factors as well. Shallow, naturally eutrophic lakes with short water residence times and high catchment area to lake area and volume ratios have been particularly sensitive to anthropogenic forcing. Our results indicate that cultural eutrophication is not the only water protection challenge for the relatively remote and dilute boreal lakes, but salinization and alkalinization are also serious threats that should be taken into account. Therefore, it is crucial to consider the notable variation in the natural conditions of boreal lakes in addition to mitigating the effects of anthropogenic forcing, such as nutrient loading, catchment erosion, salt pollution, and climate change, in order to achieve efficient water protection.
Introduction
Cultural eutrophication of surface waters is a widely acknowledged but still largely unresolved environmental problem (Smith and Schindler, 2009; Khan and Mohammad, 2014). Excessive nutrient supplies into lakes due to anthropogenic activities, such as agriculture, can lead to increased primary production and algal blooms, decreased biodiversity, fish kills, decreased water transparency, and oxygen depletion (Callisto et al., 2014). These consequences of eutrophication affect the societal, commercial, and recreational use of the waters (Khan and Mohammad, 2014). Lowland lakes, in particular, may have been affected by humans for hundreds or thousands of years, and since the 1850s, cultural eutrophication and bottom water anoxia have further intensified (Battarbee et al., 2011; Jenny et al., 2016; Saulnier-Talbot, 2016; Dubois et al., 2017).
In addition to anthropogenic forcing, natural factors, such as the direct and indirect effects of climate on lakes and their catchment processes, affect the nutrient levels of lakes leading to natural variation in their background conditions (Fritz and Anderson, 2013; Dubois et al., 2017). In the boreal regions, climate has shifted from the relatively cool and humid conditions of the early Holocene to the drier Holocene Thermal Maximum (8,200–4,200 BP) and again to cooler and more humid conditions in the late Holocene (Heikkilä and Seppä, 2003; Heikkilä et al., 2010). Primary succession resulted in long-term physical, chemical, and biological changes in boreal lakes as the newly-formed catchments stabilized during the Holocene following the retreat of the continental ice sheet (Engström et al., 2000; Fritz and Anderson, 2013). The development of vegetation and soil-forming processes, for example, have led to the long-term acidification and oligotrophication of some boreal lakes (Engström et al., 2000; Gottschalk, 2011; Väliranta et al., 2011; Fritz and Anderson, 2013). Water level, water residence time, and flow direction changes related to uneven glacio-isostatic land uplift have also altered lake basins and their catchments and nutrient concentrations during the Holocene (Tikkanen, 2002a). Furthermore, glaciodynamic conditions during the deglaciation controlled the deposition of different glacial landforms and sediment types in different regions (Lintinen, 1995; Punkari, 1997; Putkinen et al., 2017). These geological landforms have varying hydrogeological landscapes and groundwater connectivity leading to fine-textured landforms often having higher phosphorus concentrations than coarse-textured landforms (Plach et al., 2016).
Lake sediment records and quantitative paleolimnological and paleoecological methods provide an excellent tool for studying both natural and anthropogenic environmental changes in lakes. The top-bottom (before-after) approach, in particular, allows an effective assessment of regional environmental change between the present-day and pre-human disturbance conditions of many lakes (Smol, 2008; Anderson, 2014; Ginn et al., 2015). However, paleolimnological studies have often concentrated on the temporal examination of anthropogenic or natural disturbances in certain key sites, whereas spatial studies between lakes and their catchments have been less frequent (Anderson, 2014). For instance, there is a lack of regional studies examining the connection between surficial catchment geology and natural (background) nutrient levels of lakes. Naturally eutrophic, boreal lakes could be more common than previously thought, although they have not been studied extensively (Miettinen et al., 2005; Räsänen et al., 2006). Naturally eutrophic, boreal lakes have been found in the fertile, clayey lowland catchments of southern Finland (Räsänen et al., 2006), but the till-dominated inland area in North Savo, central-eastern Finland, also appears to be a naturally nutrient-rich region (Kauppila et al., 2012; Tammelin et al., 2017). Naturally eutrophic lakes are heterogenous even in their natural state (Räsänen et al., 2006) and, thus, it is crucial to know their baseline or pre-human disturbance conditions in order to set realistic management goals (Räsänen et al., 2006; Ginn et al., 2015; Saulnier-Talbot, 2016).
In this study, we examined the relationship between the natural total phosphorus (TP) levels of lakes in North Savo, central-eastern Finland, and the geological settings of the lakes (surficial catchment geology in particular) using top-bottom sampling, diatom analysis, and multivariate statistics. Our aims were to investigate (1) the spatial distribution of naturally eutrophic lakes in central-eastern Finland and to find out (2) which geological drivers are important in explaining the high natural nutrient levels of these lakes. Furthermore, we studied (3) how anthropogenic disturbance has changed the diatom assemblages and water quality of the naturally eutrophic lakes in comparison to nutrient-poorer lakes within the same study area.
Materials and Methods
Site Description
This study comprises 48 lakes (51 sampling sites) located in North Savo, central-eastern Finland between latitudes 62°44′N−63°52′N, longitudes 26°18′E−28°04′E, and altitudes 82–160 m.a.s.l. (Figure 1, Table 1). Their selection process, modern water quality, and basin characteristics were described by Tammelin et al. (2017). The lakes are linked together via rivers and streams forming watercourses (chains of lakes). The watercourses consist of small headwater lakes and larger central basins that collect the waters from the headwaters. Roughly half of the studied lakes belong to the Iisalmi Route watercourse, while the rest are located in three adjacent watercourses (the Nilsiä Route, the Rautalampi Route, and the Kallavesi-Sorsavesi area). These watercourses form the headwaters of the River Vuoksi and Kymijoki drainage systems that eventually flow into the Baltic Sea. The Iisalmi Route has currently lower ecological quality than the adjacent watercourses based on the classification of the Finnish environmental administration (Kauppila et al., 2012; Vallinkoski et al., 2016). The classification emphasizes the state of freshwater biota but also takes into account water quality as well as the hydrological and morphological characteristics of the lakes (Vallinkoski et al., 2016).
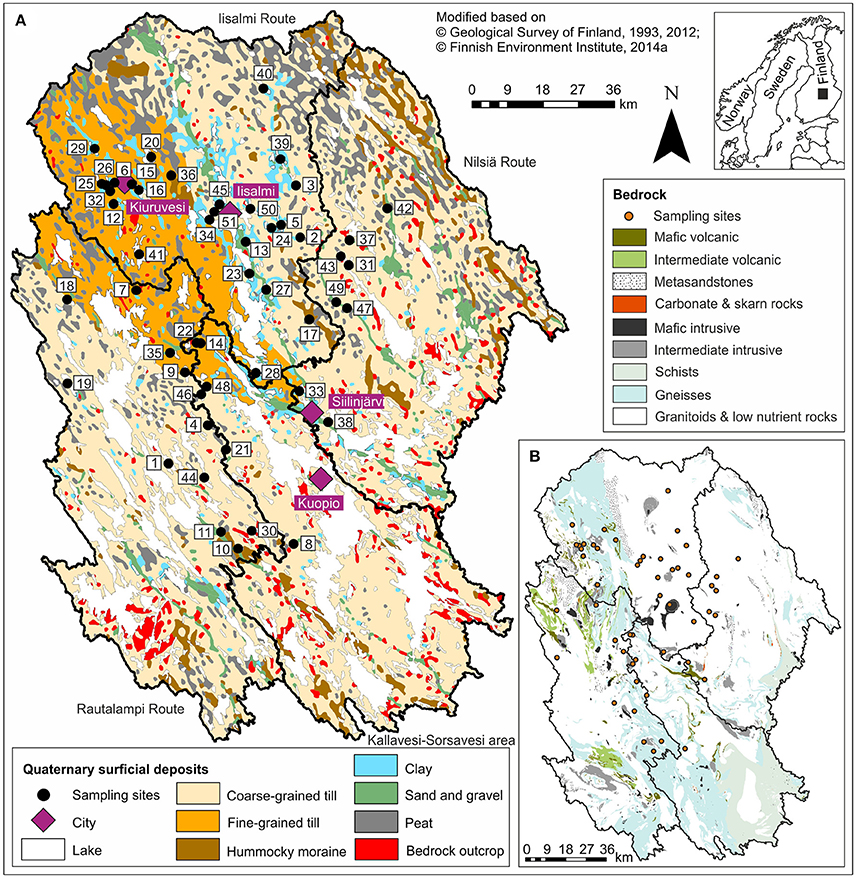
Figure 1. A map showing the location (inset), (A) the surficial geology, and (B) the crystalline bedrock of the study area together with the sampling sites and the catchments of the four watercourses. See Table 1 for the names and locations of the lakes.
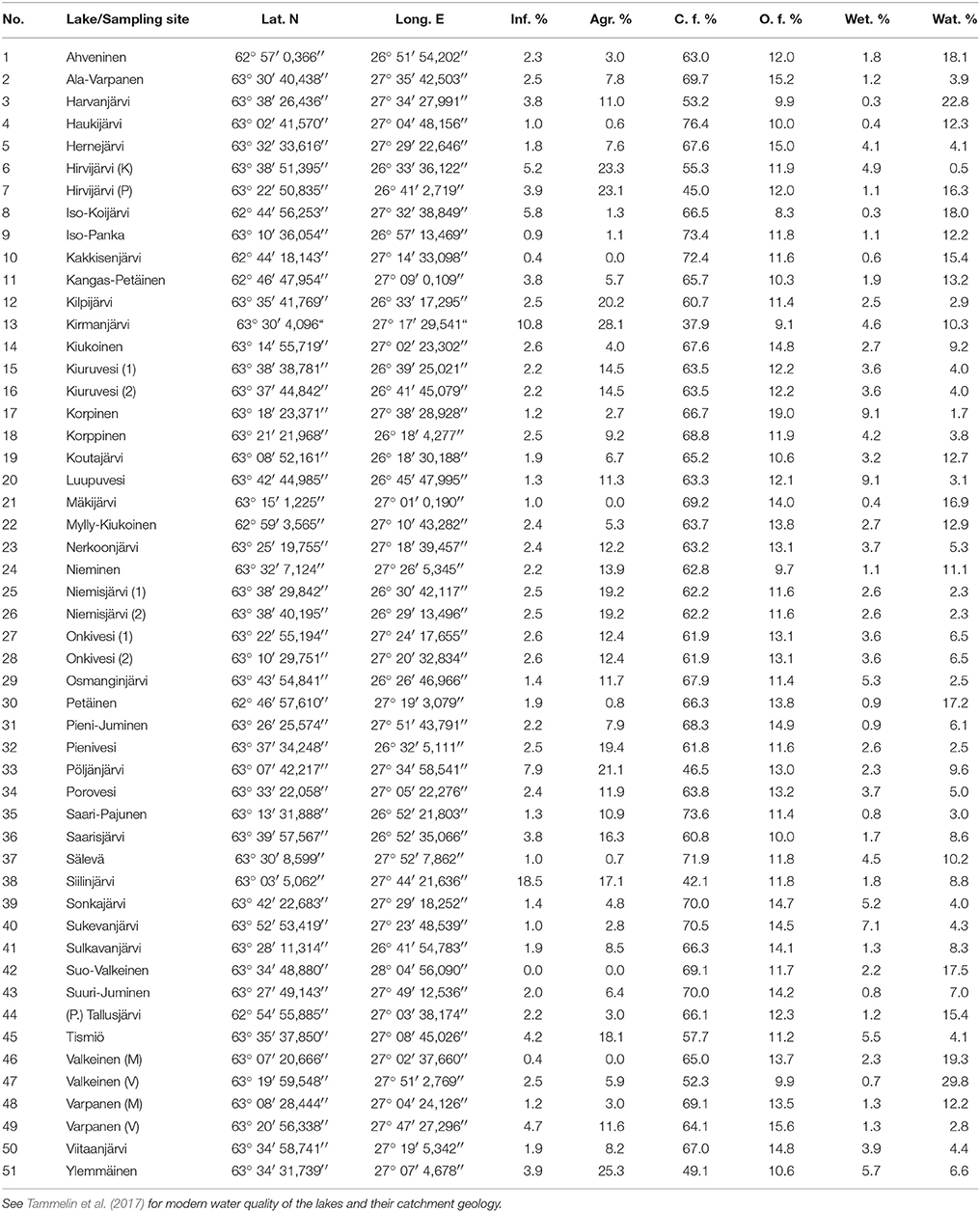
Table 1. Coordinates for the sampling sites and modern land use of the lake catchments (Finnish Environment Institute, 2014b) including infrastructure, agriculture, closed and open forests, wetlands, and water.
The lakes included in this study represent the following water quality gradients: TP 5–120 μg l−1, total nitrogen (TN) 310–1,750 μg l−1, color 15–340 mg Ptl−1, pH 6.3–7.8, electrical conductivity (EC) 1.9–12 mS m−1, and Secchi depth 0.4–3.5 m. The lakes are mainly small (surface area median 2.0 km2) and shallow (average depth median 2.8 m, maximum depth median 9.6 m), as is typical for boreal lakes in glaciated landscapes (Simola and Arvola, 2005). However, the ranges are 0.004–114 km2, 0.7–6.9 m, and 1.0–37 m, respectively, due to three larger central basins (Lake Porovesi #34, Lake Nerkoonjärvi #23, and Lake Onkivesi #27-28). The water residence times range from four to over 1,000 days based on the VEMALA nutrient loading model of the Finnish Environmental Administration (Huttunen et al., 2016). The catchment areas range from 0.2 to 5,564 km2.
Lakes in North Savo formed after the last Scandinavian deglaciation ca. 10,200–9,500 BP mainly by isolating from the Yoldia Sea or Ancylus Lake phases of the Baltic Sea (Saarnisto, 2000; Tikkanen, 2002a). The surficial geology of their catchments is also largely a result of this deglaciation phase. The catchments are covered by basal till with varying grain sizes, glaciogenic hummocky moraines, glaciofluvial eskers, and landforms that deposited after the deglaciation, including littoral deposits, varved Yoldia Sea clays and silts, later homogenous clays and silts, and peat deposits (Figure 1A; Tikkanen, 2002b). Bedrock outcrops are scarce in most catchments, but lakes and rivers often follow bedrock fracture zones (Tikkanen, 2002b). The bedrock in the northeastern part of the study area belongs to the 3.2–2.6 Ga Archean Iisalmi complex (including granitoids, migmatites, and high-grade gneisses) and in the southwestern part to the 1.89–1.86 Ga Proterozoic granitoid complex of central Finland with the 1.92–1.88 Ga sheared Savo Belt between these two complexes (Figure 1B; Vaasjoki et al., 2005; Geological Survey of Finland, 2012). The Savo Belt is an intricate volcanic belt deformed by
several tails of a shear system and characterized by metamorphic mica gneisses with interlayers of mafic to intermediate volcanic rocks, schists, and carbonate rocks as well as gabbro intrusions (Vaasjoki et al., 2005).
In the four meteorological stations within or next to our study area, annual mean temperature varies between 2.3–3.4 °C and annual mean precipitation between 612 and 720 mm (Pirinen et al., 2012). The study area mainly belongs to the coniferous southern boreal vegetation zone (Ahti et al., 1968). The first permanent settlers utilized forests for slash-and-burn cultivation since the sixteenth century until the end of the nineteenth century when modern agriculture and forestry with industrial fertilizers and field, forest, and peatland drainage began (Soininen, 1961, 1974). Land use and nutrient loading further intensified from the 1950s to the 1980s, whereas sewage treatment and industrial processes improved since the 1970s (Simola and Arvola, 2005). Currently, the most intensive agriculture and highest nutrient loading (> 20 kg P km−2 a−1, > 400 kg N km−2 a−1) is found in the Iisalmi and Nilsiä Route catchments where several lakes suffer from eutrophication and oxygen depletion (Table 1; Vallinkoski et al., 2016). Water quality restoration targets ranging 18–55 μg P l−1 and 500–930 μg N l−1 have been set for some of the lakes in our study area (Vallinkoski et al., 2016).
Sampling and Data Acquisition
A rod-operated piston corer was used for obtaining long sediment cores from 42 sampling sites in late winter 2010 and from the remaining nine sites in late winter 2012 (through ice). Two 1-cm bottom samples, 10 cm apart, were taken from each sediment core for diatom analysis, except for Lake Ylemmäinen (#51) from which only one bottom sample was taken. The depths of the bottom (i.e., pre-disturbance) samples were selected based on the magnetic susceptibility profiles of the sediment cores. Magnetic susceptibility was measured at 1-cm intervals with a Bartington MS2E susceptibility meter and a MS2C loop sensor. A rise in the magnetic susceptibility values in the top part of the cores was assumed to represent increased catchment erosion due to human disturbance (Thompson et al., 1975; Sandgren and Snowball, 2001). The upper bottom samples were taken ~20 cm and the lower samples ~30 cm below the onset of this recent rise in the magnetic susceptibility values. These are the pre-disturbance samples. The top (post-disturbance) diatom samples (0–1 cm; Tammelin et al., 2017) were obtained in late winter 2010 from the same sampling sites as the bottom samples but with a Limnos® gravity corer (Kansanen et al., 1991) that is particularly suitable for taking undisturbed surface sediment samples.
The diatom slides were prepared and identified from the bottom and top sediment samples using standard procedures described by Battarbee et al. (2001). A minimum of 300 diatom valves were identified to the species level from each bottom sample with a phase-contrast light microscope (1000x final magnification) and using the taxonomic references of Krammer and Lange-Bertalot (1986, 1988, 1991a,b), Krammer (1992), Lange-Bertalot and Moser (1994), and Houk (2003). The top samples were identified otherwise similarly but with a minimum count of 400 (Tammelin et al., 2017) in order to improve the statistical reliability of the transfer function training set consisting of the top samples (Battarbee et al., 2001). Nomenclature was updated based on Porter (2008), Spaulding et al. (2010), and Guiry and Guiry (2018). In addition to diatoms, chrysophyte cysts were counted from the same microscope views as diatoms and expressed as cyst to diatom (CD) ratio in order to serve as a proxy for eutrophication (Werner and Smol, 2005).
In addition to the diatom data, environmental data was collected for each lake from open data repositories. These environmental data include surficial catchment geology (Geological Survey of Finland, 1993) from the Hakku service, new catchment divisions of Finnish watersheds (Finnish Environment Institute, 2014a) and Corine Land Cover 2012 dataset (Finnish Environment Institute, 2014b) from the VALUE catchment tool, and modern water quality of the water quality monitoring sites at or near the sediment coring sites (Finnish Environment Institute, 2016) from the Hertta service. Furthermore, we gathered lake morphologies and water residence times from the VEMALA national-scale nutrient loading model for Finnish watersheds (Huttunen et al., 2016).
Numerical Analyses
Ordination and regression analyses of the diatom and environmental data were made with the Canoco 4.5 for Windows (ter Braak and Šmilauer, 2002) and C2 version 1.7.7 (Juggins, 2007) software. As in Tammelin et al. (2017), the relative abundance diatom data was square root transformed prior to numerical analysis in order to stabilize the variance (ter Braak and Šmilauer, 2002) and the water quality data was log-transformed, except for pH that is already on log-scale. We reconstructed past TP concentrations for each bottom sample by using the training set comprising the top samples and the diatom-TP transfer function developed by Tammelin et al. (2017). The two-component transfer function is based on weighted averaging partial least squares regression (WA-PLS; ter Braak and Juggins, 1993) and it has a jack-knifed coefficient of determination () of 0.72 and root mean squared error of prediction (RMSEP) of 0.191 log μg l−1. The reconstructed TPs were compared with modern water quality monitoring data in order to examine the phosphorus level change of the lakes during human disturbance. The reliability of the reconstructions was evaluated using three simple percentage measures suggested by Juggins and Birks (2012) as the more complex sample-specific RMSEP, goodness-of-fit, and analog measures are difficult to interpret. The measures included (i) the percentage of fossil sample taxa absent from or (ii) poorly represented (<10 occurrences) in the surface sample training set and (iii) taxa with higher maximum abundances in the bottom than top samples (representing possible no modern analog situations).
To study the diatom species assemblage change between pre- and post-disturbance conditions, we calculated the squared chord distances (SCDs) between the bottom and top samples (Simpson, 2012) with the Python programming language and the species richness (rarefaction at the total count of 298 valves; Hurlbert, 1971) of the bottom and top samples with PAST 3.16 software (Hammer et al., 2001). In addition, we used multivariate ordination methods and Canoco 4.5 for Windows software (ter Braak and Šmilauer, 2002) to visualize the diatom assemblages in relation to environmental factors. The species gradient length of the bottom samples, calculated with detrended correspondence analysis (DCA; Hill and Gauch, 1980), allowed the use of unimodal canonical correspondence analysis (CCA; ter Braak, 1986). The bottom samples were plotted passively on top of a CCA ordination of the top samples (with downweighting of rare species) and modern water quality to see the trajectories of the bottom to top diatom assemblage changes in relation to the environmental gradients. In addition, basin characteristics (morphology) and the modern land use of the catchments were added as supplementary environmental variables to the analysis. Another CCA was run on averaged bottom samples together with the surficial geology of the catchments. Averaged samples were used because the surficial catchment geology is the same for both bottom samples of an individual lake but the diatom assemblages vary to some extent. Furthermore, we calculated Spearman rank correlations (Spearman, 1904) between the environmental variables to recognize drivers for the pre- and post-disturbance TP concentrations.
Results
Sediment Properties
The cored sediments were mainly brown organic-rich mud (gyttja) with sulfide-stained black layers in the top parts of many cores. The lengths of the cores ranged from 113 to 228 cm (mean 176 cm). Apart from a few exceptions, the magnetic susceptibility profiles of these sediment cores showed clearly elevated values in the upper parts of the cores (Figure 2) with the highest values located, on average, at the depth of 14 cm (range 0–51 cm). The bottom diatom sample depths, selected 20 and 30 cm below the onset of the increasing magnetic susceptibility values, ranged from 45 cm below the sediment surface down to 145 cm (mean 74 cm).
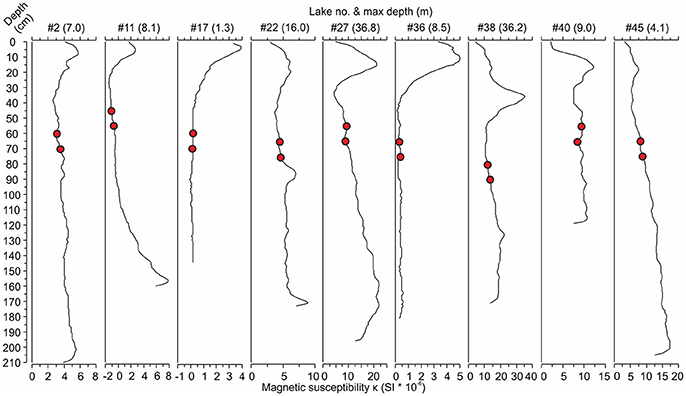
Figure 2. Examples of magnetic susceptibility profiles from nine lakes [#lake number (max lake depth)] showing the increases in magnetic susceptibility toward the present day (core top). The red circles represent the bottom samples taken at 20 and 30 cm below the rise in magnetic susceptibility indicating the start of anthropogenic catchment erosion. See Table 1 for the names and locations of the lakes.
Diatom Assemblages
The bottom (pre-disturbance) diatom samples were dominated by planktonic taxa together with small benthic fragilarioids, such as Staurosira construens var. venter (Ehrenberg) Hamilton, Staurosirella pinnata (Ehrenberg) Williams and Round, Stauroforma exiguiformis (Lange-Bertalot) Flower, Jones and Round, and Pseudostaurosira brevistriata (Grunow) Williams & Round (Figure 3). A variety of Aulacoseira taxa in addition to Lindavia rossii (Håkansson) Nakov et al., Asterionella formosa Hassall, and Tabellaria flocculosa (Roth) Kützing were the most common planktonic taxa. Small Fragilaria, Navicula, and Achnanthes taxa (sensu lato) as well as Eunotia spp. were typical among the benthic taxa of the bottom samples. Lake Suo-Valkeinen (#42) differed from the other samples with the lack of planktonic taxa and high abundances of Pinnularia mesolepta (Ehrenberg) Smith and Stauroneis anceps Ehrenberg. The lakes along the Iisalmi Route had generally higher abundances of meso-eutrophic taxa, such as Aulacoseira subarctica (Müller) Haworth, Aulacoseira ambigua (Grunow) Simonsen, Aulacoseira islandica (Müller) Simonsen, Aulacoseira granulata (Ehrenberg) Simonsen, and S. construens var. venter, than the lakes in the adjacent watercourses. In contrast, these adjacent watercourses had higher relative abundances of oligo-mesotrophic T. flocculosa, L. rossii, Aulacoseira nivalis (Smith) English & Potapova, and Frustulia rhomboides (Ehrenberg) De Toni. Correspondingly, the species richness (rarefaction; Figure 4A) of the bottom sample diatom assemblages and CD ratios (Figure 4B) were generally lower in the Iisalmi Route than in the other watercourses.
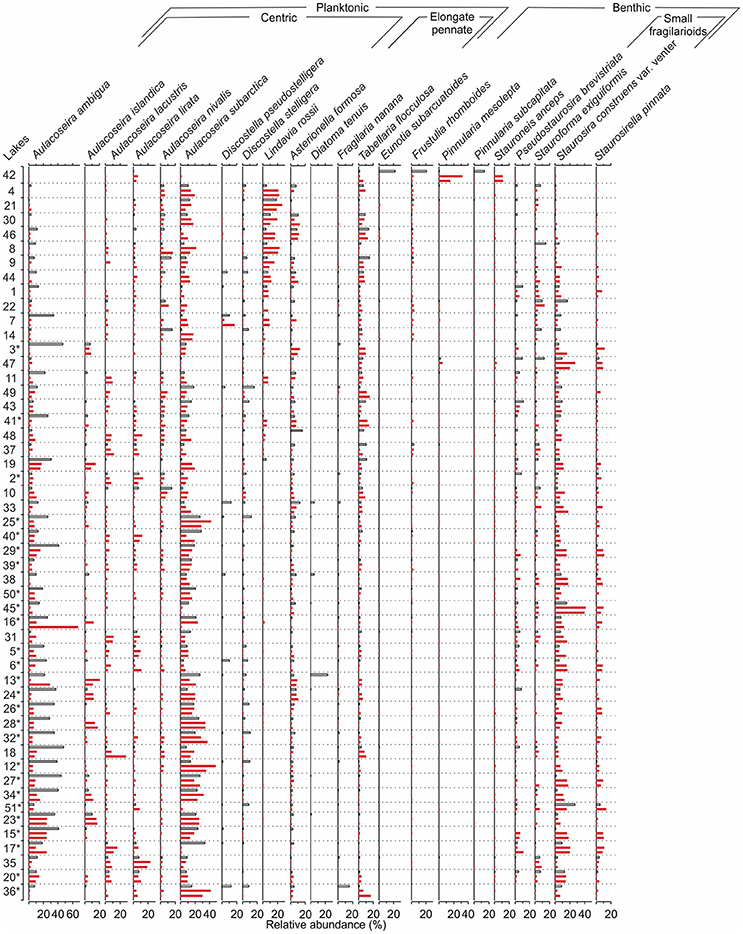
Figure 3. A comparison between the bottom sample (red bars) and top sample (white bars) diatom assemblages for each sampling site. See Table 1 for the names and locations of the sites and Tammelin et al. (2017) for the description of the top sample data. The sampling sites are organized according to increasing bottom sample total phosphorus reconstructions. The Iisalmi Route sampling sites are marked with an asterisk. Taxa with a maximum relative abundance over 10% are included in the diagram.
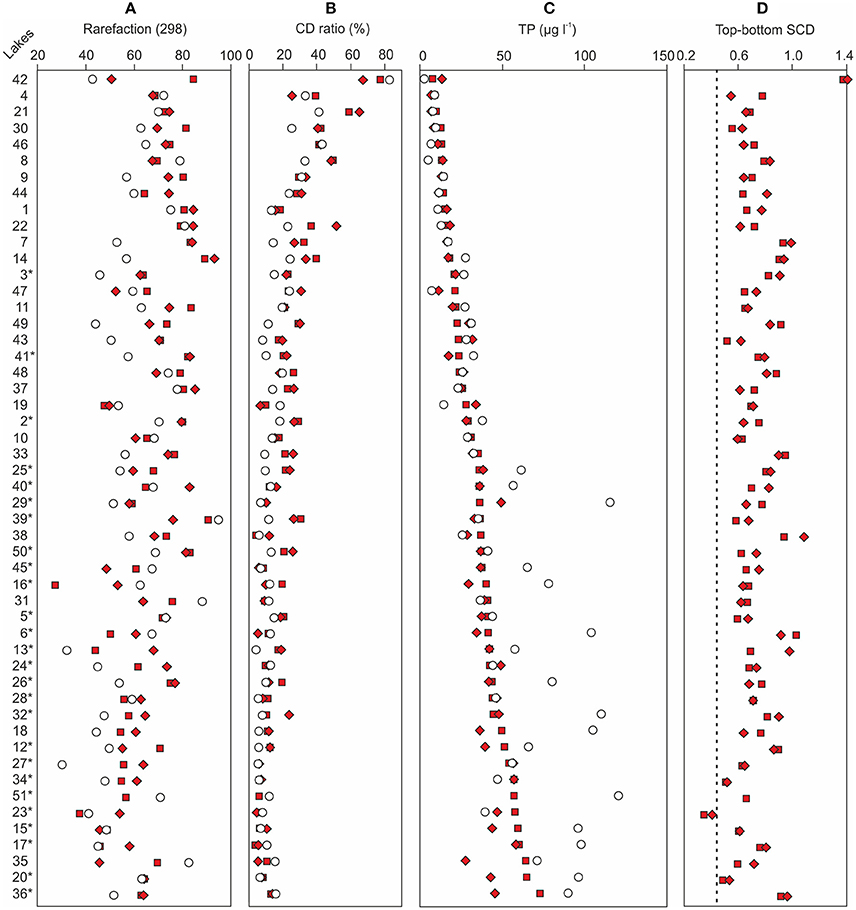
Figure 4. A comparison between (A) the reconstructed bottom sample total phosphorus (TP) concentrations and the monitored modern TP concentrations of each sampling site, (B) bottom and top sample chrysophyte cyst to diatom (CD) ratios and (C) diatom species richness, and (D) bottom sample squared chord distances (SCDs) from the top samples. The filled squares denote the lower bottom samples, the filled diamonds denote the upper bottom samples, and the empty circles represent modern TP in (A) and top samples in (B–D). The dashed vertical line in (D) represents the median SCD between the two bottom samples of each lake. The sampling sites are organized according to increasing bottom sample total phosphorus reconstructions. The Iisalmi Route sampling sites are marked with an asterisk. See Table 1 for the names and locations of the sampling sites and Tammelin et al. (2017) for the description of the top sample data.
The relative abundances of certain diatom taxa clearly increased from bottom to top samples, while others decreased (Figure 3). A. ambigua and Discostella stelligera (Cleve & Grunow) Houk & Klee, for example, increased in most sampling sites regardless of the watercourse, whereas Discostella pseudostelligera (Hustedt) Houk & Klee and Diatoma tenuis Agardh showed clear increases only in a few lakes. Other Aulacoseira taxa, L. rossii, S. construens var. venter, and S. pinnata generally decreased from bottom to top. Many lakes showed a decrease in diatom species richness (Figure 4A). The CD ratio was typically lower in the recent samples than in the bottom samples and the decrease from bottom to top was generally more pronounced in the less eutrophic watercourses than in the Iisalmi Route (Figure 4B). Lake Suo-Valkeinen (#42) differed from the other lakes based on its diatom assemblage change as its P. mesolepta and S. anceps-dominated bottom sample assemblage changed into a community dominated by Eunotia subarcuatoides Alles, Nörpel & Lange-Bertalot, F. rhomboides, and Pinnularia subcapitata Gregory.
Diatom-Inferred Total Phosphorus
The background diatom-inferred total phosphorus (DI-TP) concentrations (8–60 μg l−1, median 35 μg l−1) were higher in the Iisalmi Route than in the other watercourses apart from a few exceptions (Figure 4C). The two bottom diatom samples of each sampling site gave relatively similar DI-TP values except for the eutrophic Lakes Luupuvesi (#20), Saari-Pajunen (#35), and Saarisjärvi (#36). The DI-TPs were notably lower than the monitored modern TPs in many eutrophic lakes with background DI-TPs over 35 μg l−1, while the DI-TPs and modern TPs were quite similar in the less eutrophic lakes. However, the latter situation was also seen in some of the eutrophic lakes. These lakes mainly included the largest central basins of the Iisalmi Route as well as other deep lakes (maximum depth > 10 m) in the easternmost branch of the Iisalmi Route and in the Nilsiä Route.
The reliability of the DI-TP reconstructions was evaluated by using three simple percentage measures. The relative abundance of bottom sample taxa absent from the top sample training set (Figure 5A) ranged between 2.5 and 30.4% (median 15.8%), excluding Lake Suo-Valkeinen (#42, ca. 50%), being slightly lower in the Iisalmi Route than in the less eutrophic lakes. The median percentage of fossil taxa poorly represented in the modern training set (Figure 5B) was 3.7% (range 0–13.7%) being highest in Lake Saari-Pajunen (#35). For comparison, the top sample percentages of taxa absent from the training set ranged from 2.4 to 22.7% (median 9.0%) and poorly represented taxa from 0 to 9.5% (median 1.5%). The median relative abundance of the taxa that had higher fossil abundances than their training set maximum value (Figure 5C) was 16.4% (range 0–66.6%). In most cases, however, these higher abundances of fossil taxa were close (within 6%) to the maximum training set value.
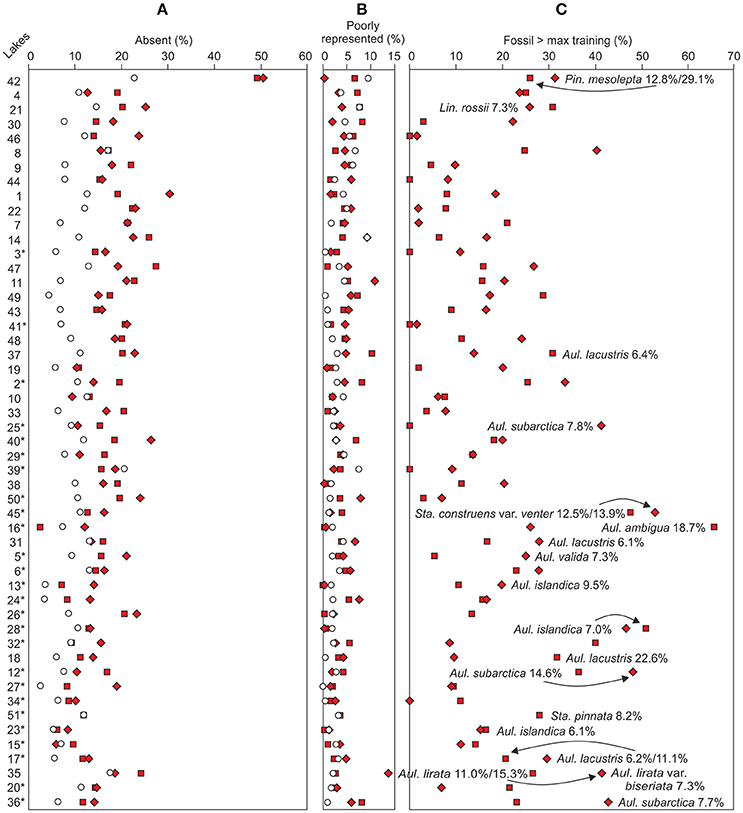
Figure 5. Similarity between the top (empty circles) and bottom (filled squares lower bottom sample and diamonds upper bottom sample) samples based on the percentages of fossil taxa (A) absent from or (B) poorly represented in the modern training set. (C) Taxa with higher fossil abundances than the training set maximum abundance. Taxa with fossil abundances over 5% higher than the training set maximum values are marked in the figure. The sampling sites (see Table 1 for names and locations) are organized according to increasing bottom sample total phosphorus reconstructions. The Iisalmi Route sampling sites are marked with an asterisk.
Spearman Rank Correlations
Spearman rank correlations between variables were studied to find the drivers for both the pre-disturbance and modern water quality and to estimate the covariation between the environmental variables. The DI-TP of the upper and lower bottom samples correlated negatively (p ≤ 0.001) with the CD ratio (r from −0.73 to −0.83), water residence time (r from −0.51 to −0.55), and rarefaction (r from −0.38 [p = 0.007] to −0.61). In contrast, the DI-TP correlated positively with the drainage basin area/lake volume (DBA/LV) ratio (r = 0.47–0.53, p < 0.001), where drainage basin area is catchment area − lake area, and drainage basin area/lake area (DBA/LA) ratio (r = 0.40–0.42, p = 0.004–0.002). From the surficial geology, the DI-TP had the strongest positive correlation with clay (r = 0.51–0.55, p < 0.001). The DI-TP also correlated (p > 0.001) positively with fine-grained till and negatively with the average and maximum depths of the lakes and coarse-grained till. However, these correlations were even stronger (p < 0.001) with the relative bottom to top TP change [(TP−DI–TPav)/DI-TPav] being 0.48, −0.52, −0.45, and −0.49, respectively. The correlation between the DI-TP and relative TP change was 0.41–0.51 (p ≤ 0.003).
The modern nutrient concentrations showed statistically significant (p < 0.001) positive correlation with the catchment percentages of agricultural land (TP r = 0.59, TN r = 0.65) and wetlands (TP r = 0.55, TN r = 0.52). In contrast, both TP (r = −0.75) and TN (r = −0.73) correlated negatively (p < 0.001) with the percentage of water in the catchments. EC, in turn, correlated positively (p < 0.001) with agriculture (r = 0.74) and infrastructure (r = 0.51), while closed forests (r = −0.57) and water (r = −0.42, p = 0.002) had negative correlation coefficients. Agriculture (r = 0.48, p < 0.001), closed forests (r = −0.42, p = 0.002), and infrastructure (r = 0.39, p = 0.004) showed statistically significant correlations with pH as well. Secchi depth and TN/TP ratio correlated (p < 0.001) with water (rSecchi = 0.66, rTN/TP = 0.66), wetlands (rSecchi = −0.47, rTN/TP = −0.45), and agriculture (rSecchi = −0.48, rTN/TP = −0.46), while color only correlated with water (r = −0.63, p < 0.001) and wetlands (r = −0.36, p = 0.009).
Besides correlating with pre-disturbance and modern water quality, the environmental variables showed marked covariation. The catchment percentage of agriculture correlated positively with catchment clay percentage (r = 0.70, p < 0.001) and fine-grained till (r = 0.41, p = 0.003) and negatively with closed forests (r = −0.77, p < 0.001) and water (r = −0.51, p < 0.001). Infrastructure, on the other hand, showed only weaker correlations with clay (r = 0.38, p = 0.006) and hummocky moraine (r = −0.29, p = 0.036). However, the correlations were strong between infrastructure and agriculture (r = 0.71, p < 0.001) and closed forests (r = −0.76, p < 0.001), as well as agriculture and closed forests (r = −0.77, p < 0.001).
Squared Chord Distances Between Diatom Samples
Floristic variability within, and changes between, the sets of samples were studied with SCDs. Slightly higher variability was found for the bottom samples compared to the modern samples. The SCDs between all bottom samples ranged from 0.21 to 1.64 (median 0.75) and between all top samples from 0.17 to 1.38 (median 0.72), excluding Lake Suo-Valkeinen (#42) that had anomalous diatom assemblages compared to the other lakes. For Lake Suo-Valkeinen, the SCDs to the other bottom samples ranged between 1.11 and 1.77 and to the other top samples between 1.44 and 1.87. The temporal changes between the background and modern samples (bottom-to-top) were, on average, somewhat higher than those between the background sample pairs. The SCDs between the bottom and top sample pairs of each sampling site (Figure 4D) ranged from 0.34 to 1.41 (median 0.71). The SCDs between the two bottom samples of each sampling site, on the other hand, only ranged between 0.21 and 0.87 (median 0.44; dashed line in Figure 4D). When the SCDs between all bottom samples, all top samples, and bottom-top sample pairs were examined in three groups (<20 μg l−1, 20–40 μg l−1, and > 40 μg l−1) along the bottom sample DI-TP gradient (Lake Suo-Valkeinen excluded), the floristic variability was lowest in the highest DI-TP class (Table 2).

Table 2. Minimum (Min), median (Md), average (Av), and maximum (Max) values for squared chord distances (SCDs) between all bottom diatom samples, all top samples, and the bottom-top sample pairs in three groups along the natural phosphorus gradient (pre-human disturbance diatom-inferred total phosphorus, DI-TP; Lake Suo-Valkeinen #42 excluded).
Ordinations
The DCA-based species gradient length of 2.98 standard deviation (SD) units for the bottom diatom samples allowed the use of the unimodal CCA method for further analysis (ter Braak and Prentice, 1988). The bottom sample species gradient was shorter than the corresponding gradient of the top diatom samples (3.87 SD units). However, the anomalous Lake Suo-Valkeinen (#42) lengthened particularly the top sample species gradient. If Lake Suo-Valkeinen was excluded from the gradient length analysis, the bottom and top sample lengths reduced to 2.00 and 2.32 SD units, respectively.
A CCA ordination of the bottom (pre-disturbance) diatom samples and surficial catchment geology (Figure 6A) shows that the catchments of the eutrophic Iisalmi Route lakes are dominated by fine-grained till, clay, and peat deposits in contrast to the adjacent watercourses where coarse-grained till and bedrock outcrops are more common. Three cyclotelloid taxa (Lindavia radiosa (Grunow) De Toni & Forti, D. pseudostelligera, and L. rossii) were typical for the coarse-grained till areas, while Aulacoseira and small fragilarioid taxa characterized the areas with fine-grained and organic deposits (Figure 6B). Lake Suo-Valkeinen (#42) with its benthic diatom assemblage (e.g., Pinnularia, Eunotia, and Cymbella taxa) plots farthest in the direction of hummocky moraines, perpendicular to the fine- vs. coarse-grained till gradient.
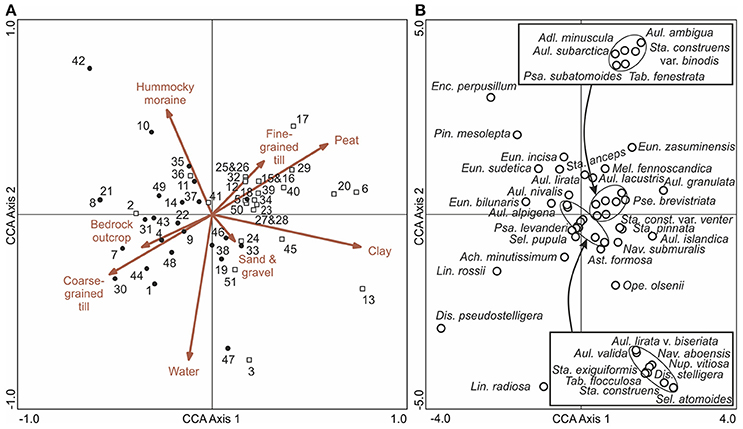
Figure 6. A CCA ordination of bottom (pre-human disturbance) diatom samples with (A) surficial catchment geology (arrows) and (B) diatom taxa (empty circles). In (A), the empty squares represent sampling sites in the Iisalmi Route and the filled circles represent sampling sites in the adjoining watercourses. Diatom taxa with maximum abundances <4% in the bottom samples were excluded from (B) for clarity. The names of the diatom taxa encircled in the center of the ordination are given in the boxes. See Table 1 for the names and locations of the sampling sites.
According to the top sample CCA with passively plotted bottom samples (Figure 7A), the Iisalmi Route lakes are typically shallower and located at a lower altitude from the sea level than the lakes in adjacent watercourses. They also have shorter water residence times, larger catchment areas, and higher DBA/LA and DBA/LV ratios. Most trajectories between the bottom and top samples were toward higher TP, TN, color, EC, and pH and lower Secchi depths (Figure 7B). The nutrient gradients were nearly parallel to the relative abundance of agriculture in the catchments (mean 9.9%, Table 1) and also rather similar to the wetland abundance (mean 2.8%) gradient. The EC and pH gradients, on the other hand, were parallel to infrastructure (mean 2.9%) and opposite to closed forests (mean 63%). However, a few lakes showed trajectories toward reduced EC, pH, and/or nutrients. Lake Suo-Valkeinen (#42), for example, had the longest bottom to top trajectory in the direction of decreasing EC and pH. Nutrient rich lakes had generally longer trajectories than oligotrophic ones and many small, eutrophic headwater lakes showed a more notable transition than the larger central basins of the Iisalmi Route.
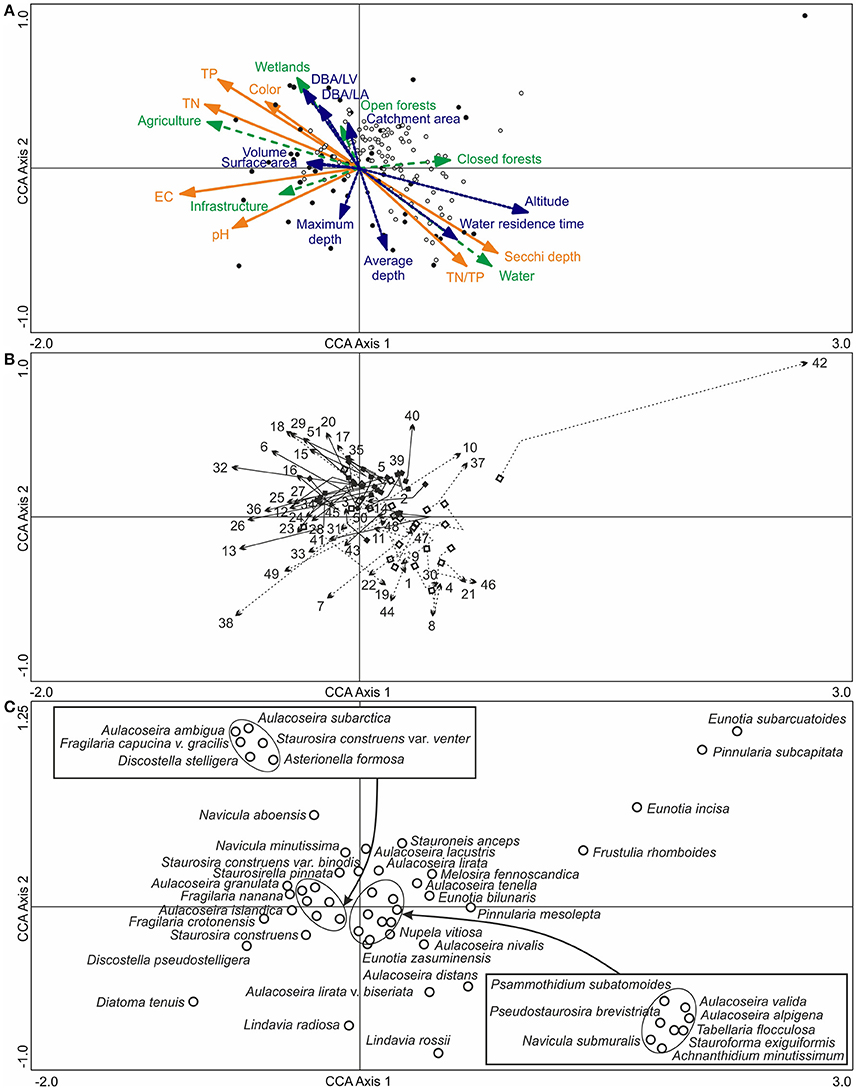
Figure 7. (A) A CCA ordination of the surface diatom samples (filled circles) and modern water quality (orange arrows with solid lines), based on the training set by Tammelin et al. (2017), with the bottom samples (empty circles) plotted passively on top of the ordination. Basin characteristics (blue arrows with dotted lines) and modern catchment land use (green arrows with dashed lines) were added into the ordination as supplementary variables. (B) The pre- to post-human disturbance (bottom to top) trajectories of the sampling sites with squares representing the lower bottom samples and arrowheads the surface sediment samples. The Iisalmi Route sites are marked with solid arrow lines and filled squares and the sites in the adjacent watercourses with dotted arrow lines and empty squares. (C) The top sample diatom taxa (empty circles) with maximum abundances over 5% in the bottom and top samples. The names of the diatom taxa encircled in the center of the ordination are given in the boxes. One small naviculoid species was excluded from the figure as it plotted far from the other taxa in the top right corner of the ordination. See Table 1 for the names and locations of the sampling sites (numbers) and Tammelin et al. (2017) for the description of the modern training set data.
The diatom assemblages of the deeper and less eutrophic lakes with longer residence times were characterized by taxa, such as L. rossii, A. nivalis, Aulacoseira distans (Ehrenberg) Simonsen, Aulacoseira lirata var. biseriata (Grunow) Haworth, Nupela vitiosa (Schimanski) Siver & Hamilton, and T. flocculosa (Figure 7C). In contrast, A. granulata, A. ambigua, A. subarctica, and Navicula aboensis (Cleve) Hustedt together with elongated and small fragilarioid taxa were typical for the shallower eutrophic lakes with short water residence times. D. tenuis, D. pseudostelligera, and Fragilaria crotonensis Kitton were examples of diatom taxa present in elevated EC and pH conditions and in higher catchment infrastructure percentages like in Lake Siilinjärvi (#38) and Lake Kirmanjärvi (#13).
Discussion
Spatial Variation in Pre-human Disturbance Conditions
Natural drivers of lake ecosystem variation include climate, elevation, and catchment geology (Fritz and Anderson, 2013). Climatic conditions are relatively uniform within the study area leaving elevation and catchment geology as the main factors behind the spatial variation in the pre-disturbance diatom assemblages (Figure 3) and DI-TPs (Figure 4C). The nutrient-rich lakes are located closer to the sea level on peaty, clayey, and fine-grained till catchments and show more homogenous pre-disturbance diatom assemblages than the nutrient-poor lakes on catchments characterized by coarse-grained till and bedrock outcrops (Figure 6, Table 2). Notable differences between proximate lakes within a certain geologic setting and climatic conditions may result from differences in lake morphology, internal processes, and interaction with the landscape (Kratz et al., 1997). Hydrologic landscape is very complex in glaciated areas as different landscape types (fine- vs. coarse-textured) within a region possess distinct hydrologic properties that affect the connectivity to groundwater (Winter, 2001; Plach et al., 2016). Lakes located low in the landscape typically receive more water from groundwater sor surface inlets and less from precipitation than lakes high in the landscape that are often smaller and more dilute (Kratz et al., 1997, 2006). Fine-textured landforms receive higher phosphorus loading than coarse-textured landforms due to short, near-surface flow paths of phosphorus-rich groundwater via peatlands in contrast to larger, longer, and higher yield groundwater flow systems (Plach et al., 2016).
The grain-size variation of tills (deposited by the retreating glacier) seems to control the spatial variation in the natural trophic states of boreal lakes in central and eastern Finland. The pre-disturbance DI-TPs of the eutrophic Iisalmi Route lakes (Figure 4C) are considerably higher than those in the till-dominated catchments to the southeast of our study area (DI-TP median 9 μg l−1; Miettinen et al., 2005). The eutrophic lakes are located on a wedge-shaped belt of fine-grained (clayey/silty) till that stretches from the Bothnian Bay into our study area in the northwest-southeast direction (Tammelin et al., 2017). This fine-grained till deposited under passive ice between actively flowing ice lobes whose meltwaters washed away the finer fractions leaving behind coarse-grained sandy-gravelly till (Lintinen, 1995; Punkari, 1997; Lunkka et al., 2004). Vivianite has been found in the fine-grained tills as water flows slowly through them dissolving nutrients and precipitating phosphorus in the pore spaces of the sediment (Kukkonen and Sahala, 1988). According to Lintinen (1995), several studies imply that the fine-grained till unit overlies a fines-poor basal till with possible interglacial deposits between them. Consequently, the fines fraction of the upper till most likely consists of fines-rich material that was deposited by rivers in the sedimentation basin of the Gulf of Bothnia during ice-free periods (Lintinen, 1995). The geological history of the region thus not only affects the grain size characteristics of the tills but also the provenance of the materials the till is composed of.
In addition to the genetic type of till, clay deposits influence the trophic state variation of central-eastern Finnish lakes according to the positive correlation between pre-disturbance DI-TP and catchment clay percentage (see also Figure 6). The eutrophic lakes of the Iisalmi Route have similar pre-disturbance diatom assemblages (Figure 3) and DI-TPs (Figure 4C) than the naturally eutrophic southern Finnish lakes on clayey coastal catchments (DI-TP median 35–40 μg l−1; Räsänen et al., 2006). The extensive clay deposits in southern Finland accumulated in the Baltic Basin during its past developmental stages and later emerged from under sea-water due to glacio-isostatic land uplift (Tikkanen, 2002b). In contrast, the smaller clay deposits of interior Finland accumulated on the bottom of large lake systems that developed after deglaciation, such as the Ancient Lake Saimaa in our study area. Despite these differences, the effects of these fine-grained soils on lake water quality appear to be similar.
Besides surficial catchment geology, basin morphology appears to have an effect on the lake nutrient status already in the pre-disturbance conditions (Figure 7). Lakes with the highest pre-disturbance TP concentrations are shallower and have shorter water residence times and higher DBA/LV and DBA/LA ratios than the nutrient-poor lakes. Shallow lakes are known to have proportionally higher loadings and lower losses of nutrients than deep lakes (Wetzel, 2001). Naturally eutrophic, shallow lakes have been found, for example, in the Canadian Boreal Plain where similar correlations between lake water TP and water residence time (negative) and DBA/LV (positive) have also been observed (Prepas et al., 2001; Bayley et al., 2007). Correspondingly, positive correlations between the extent of wetlands and modern lake water TP or TP export have been reported in the relatively undisturbed lakes of the Boreal Plain and Boreal Shield ecozones of Canada (Dillon and Molot, 1997; Prepas et al., 2001), although contrary observations also exist (Sass et al., 2008).
Crystalline bedrock could be another possible driver of ecosystem phosphorus status because mafic (iron-rich) rocks have often higher phosphorus concentrations than felsic (silica-rich) rocks (Porder and Ramachandran, 2013). According to Porder and Ramachandran (2013), alkali-rich mafic and intermediate rocks have particularly high phosphorus concentrations, while in sedimentary rocks the concentration decreases as grain size increases. The sheared Savo Belt contains mafic metasedimentary and volcanic rocks and could, therefore, be a potential source of phosphorus to some of the studied lakes. However, bedrock outcrops are scarce in the study area and the distribution of nutrient-rich mafic and intermediate rocks does not seem to correspond to that of the eutrophic lakes based on the bedrock map (Figure 1).
Pre- to Post-human Disturbance Change
Our results suggest that clear biological, chemical, and physical pre- to post-human disturbance changes have occurred in the studied lakes and their intensity varies spatially. Shallow, naturally eutrophic lakes with short water residence times and high DBA/LA and DBA/LV ratios, located on catchments characterized by fine-grained sediments and peat, have recorded the most prominent transitions (Figures 4, 7, Table 2). In contrast, deeper naturally eutrophic lakes seem to have been better able to retain their pre-disturbance conditions under anthropogenic forcing. The basins located higher in the chain of lakes most likely reduce loading to the lower lakes as nutrients, EC, and color correlate negatively with the catchment water percentage. Nutrient-poor lakes on coarse-grained till catchments show similar stability in their water quality but they have probably experienced milder anthropogenic forcing as agricultural activities are concentrated on the clayey and fine-grained till catchments. The geomorphic setting, hydrological catchment connectivity, and morphology of a lake affect its responses to catchment disturbances resulting in lakes located on fine-textured landforms being more sensitive to disturbances than those on coarse-textured ones (Anderson, 2014; Boeff et al., 2016; Plach et al., 2016). Shallow lakes often show earlier and more pronounced responses than deeper lakes due to their sensitivity to catchment disturbances (Dearing and Jones, 2003; Hargan et al., 2016; Smol, 2016; Dubois et al., 2017).
Our results suggest cultural eutrophication (increased TP and TN), salinization (increased EC), alkalinization (increased pH), and/or brownification (increased color) in many of the study lakes (Figures 4,7). The positive correlations between present-day lake water quality and catchment percentages of agriculture and infrastructure propose agriculture as the driving factor behind cultural eutrophication, while both agriculture and urbanization seem to be behind the salinization of the lake waters. A similar comparison in Minnesota resulted in 50% of urban lakes displaying chloride increase and 30% of urban and agricultural lakes TP increase since the early nineteenth century due to nutrient runoff and road salting (Ramstack et al., 2004). Recently, continental-scale salinization and alkalinization of fresh headwaters have been observed in humid, low salinity, and alkalinity regions across Northern America as a result of salt pollution from road de-icing, agriculture, and wastewater, together with human-accelerated weathering of natural and anthropogenic materials (Dugan et al., 2017; Kaushal et al., 2018). In our study area, the lakes located near the main road running along the esker system and showing an increase in the abundance of D. tenuis, such as Lake Kirmanjärvi (#13), Lake Siilinjärvi (#38), and Lake Pöljänjärvi (#33), are presumably the most vulnerable to road de-icing (Tammelin et al., 2017). Lake brownification (increased dissolved organic carbon, DOC) in the Northern Hemisphere since the 1990s has, in turn, been associated with the recovery from atmospheric acid deposition and climate change (Montleith et al., 2007; Weyhenmeyer and Karlsson, 2009; Brown et al., 2017).
Eutrophication and climate change are particularly difficult to distinguish when dealing with small and shallow lakes, because of their similar influences on lake ecology (Dong et al., 2012; Davidson and Jeppesen, 2013; Jeppesen et al., 2014). The diatom assemblage change in the central-eastern Finnish lakes (Figure 3), apart from A. ambigua, resembles the Aulacoseira and Fragilaria to Cyclotella shift recorded across the Northern Hemisphere and often associated with warming climate (Rühland et al., 2008; 2015). The mean temperature in Finland has risen by more than 2 °C since the mid-nineteenth century and ice cover duration has shortened by ~2 weeks (Korhonen, 2006; Mikkonen et al., 2015). These changes induce increased thermal stratification and, thus, favor small-celled cyclotelloids instead of the heavier Aulacoseira taxa (Rühland et al., 2015). However, almost 40% of the lakes in North Savo are shallow (average depth <3 m) and do not have permanent summer stratification (Vallinkoski et al., 2016), although less ice cover may also elicit similar species changes (Rühland et al., 2015). In shallow lakes, climate change may also increase diversity through new available habitats, while small, benthic fragilarioids often decrease (Rühland et al., 2015). Brown et al. (2017) emphasize the role of DOC and the complex interactions between thermal structure, light, and nutrients behind the Aulacoseira and Fragilaria to Cyclotella shift. It seems that small lakes with high DOC may be less sensitive to climate variability than clearer lakes (Read and Rose, 2013). Therefore, the recent success of A. ambigua could be related, for example, to the shallowness of the lakes, nutrient enrichment, or changes in DOC. The increasing abundances of elongate pennate diatoms have also been linked with multiple stressors, including nutrient enrichment, increased DOC, atmospheric nitrogen deposition, and warming climate (Saros et al., 2005; Kissman et al., 2013; Rühland et al., 2015; Sivarajah et al., 2016; Johnson et al., 2018).
Reliability of the Bottom Sample Reconstructions
Magnetic susceptibility profiles appear to facilitate the useful selection of pre-disturbance sampling depths before intensified anthropogenic catchment erosion in central-eastern Finland (Figure 2). Elevated magnetic susceptibility values have been found to reflect increased accumulation of mineral material into a lake and, thus, intensified catchment erosion (Sandgren and Snowball, 2001). The elevated values occurred mainly within the topmost 60 cm of the studied sediment cores, which is in agreement with previous studies of similar setups. Räsänen et al. (2006), for instance, considered the depth of 60 cm as a minimum for the pre-disturbance conditions of naturally eutrophic southern Finnish lakes estimating that these bottom samples would represent the early nineteenth century at the latest. Miettinen et al. (2005) assumed 30 cm sufficient for reaching the background conditions of oligotrophic eastern Finnish lakes, and used 1 m for eutrophic lakes assuming higher sediment accumulation rates in these basins. Furthermore, the trends in magnetic susceptibility, with higher values toward the core top, were consistent among our study lakes suggesting that sediment mixing is not a considerable problem in the shallower basins.
Selecting the bottom samples based on the magnetic susceptibility profiles allows one to take into account differences in lake and catchment histories but results in varying age for the pre-disturbance samples among the studied lakes. However, previous paleolimnological studies suggest that the most notable limnological changes in the central-eastern Finnish lakes took place during the late nineteenth and twentieth century (including possible recoveries due to lake management) and, thus, the bottom-to-top sample pairs of this study likely catch these changes sufficiently. An increase in the sedimentary mineral content has been observed in eastern Finnish lakes since the early 1800s (Taavitsainen et al., 1998), but modern agriculture with permanent fields replaced slash-and-burn cultivation in our study area as late as in the turn of the nineteenth and twentieth centuries (Soininen, 1974). Major anthropogenic water quality and ecosystem changes, such as those related to cultural eutrophication, acidification, and warming climate, have taken place from the mid-nineteenth century onwards (Rühland et al., 2008; Battarbee et al., 2011), while the most intensive acceleration in sediment accumulation rate has occurred in shallow, low-altitude European lakes only after the mid-twentieth century (Rose et al., 2011).
Paleoecological inferences should be considered as qualitative guidelines instead of values with known precision and emphasis should be placed on trends instead of single values (Juggins et al., 2013; Whitmore et al., 2015). Therefore, the pre-disturbance nutrient gradient and the spatial distribution of naturally eutrophic lakes are the most relevant results of this study rather than the exact DI-TP values of single lakes. Transfer functions simplify the complex relationship between lake biota and interlinked environmental factors into a single variable of interest (Juggins et al., 2013). Due to this complexity, transfer functions often suffer from secondary environmental gradients that confound the primary gradient of interest, taxa that might not be related to changes in the primary gradient, and poor replicability because of the spatially and temporally varying relationship between the primary and secondary gradients (Juggins, 2013; Juggins et al., 2013; Whitmore et al., 2015). Hence, causal ecological determinants should be reconstructed instead of variables acting as surrogates for complex, underlying factors, and reconstructions should always be carefully interpreted (Juggins, 2013). In order to reduce the uncertainty related to single values, we analyzed two bottom samples from each lake. The similar diatom assemblages and DI-TPs of these two samples (Figures 3, 4C) as well as the three simple percentage measures (Figure 5) suggest relatively reliable DI-TP reconstructions for most of the bottom samples, with the anomalous Lake Suo-Valkeinen (#42) being the most notable exception.
Conclusions
The spatial examination of pre- and post-human disturbance diatom assemblages and diatom-inferred water quality of 48 lakes (51 sampling sites) in central-eastern Finland revealed that naturally eutrophic lakes are more common in glaciated, boreal environments than previously thought. Our results show that naturally eutrophic lakes exist on catchments characterized by fine-grained and organic sediments, including fine-grained till. This further emphasizes the importance of the previously overlooked matter of till grain-size variation as a driver behind the spatial variation in the natural trophic states of boreal lakes. Another significant natural factor seems to be the location of the lake in the hydrologic landscape and its connectivity to groundwater either through shallow flow systems and wetlands or via larger flow systems. Furthermore, the morphology of a lake appears to be important in controlling its sensitivity to anthropogenic forcing as shallow, naturally eutrophic (> 35 μg l−1) lakes with short water residence times have been less able to resist human disturbance than deeper lakes with long water residence times.
Our results indicate that cultural eutrophication is not the only water protection challenge for the relatively remote and dilute boreal lakes, but salinization and alkalinization are also serious threats that should be taken into account. These findings reinforce the recent worrisome evidence of continental-scale salinization and alkalinization of freshwaters in humid, low salinity, and low alkalinity regions across Northern America. However, separating the effects of single drivers, such as land use and climate change, behind the ecosystem and water quality responses is challenging due to complex interactions. In order to achieve efficient water protection, it is crucial to consider the notable variation in the natural conditions of boreal lakes in addition to mitigating the effects of anthropogenic forcing, such as nutrient loading, catchment erosion, salt pollution, and climate change.
Data Availability
The raw data supporting the conclusions of this manuscript will be made available by the authors, without undue reservation, to any qualified researcher. The third party data analyzed in this study (water quality, land use, and catchment geology) were obtained from the open data repositories of the Geological Survey of Finland and the Finnish Environment Institute as stated in the references, the following licenses apply License 2 (open license version 1.1) of the Geological Survey of Finland, and CC BY 4.0, respectively. The access to and use of the VEMALA model is licensed, restrictions apply to parts of the data, and permission is needed for the commercial use of the product. Requests to access the VEMALA model should be directed to the Finnish Environment Institute at j_vesistomallit@ymparisto.fi.
Author Contributions
This study is part of the Ph.D. project of MT and, therefore, the subsampling for bottom diatom samples, diatom identification, data acquisition, and numerical analyses were all made by MT under the supervision of TK. TK is responsible for the original research idea and lake selection. The manuscript was prepared by MT and critically revised by TK. Both authors read and approved the submitted version.
Funding
Geological Survey of Finland funded the sediment sampling, diatom sample preparation, and magnetic susceptibility measurements. MT received personal grants for her Ph.D. from the Finnish Cultural Foundation (grant numbers 00120926, 85131725, 00150935, 00160988, and 00171051), Alfred Kordelin Foundation, and Olvi Foundation.
Conflict of Interest Statement
The authors declare that the research was conducted in the absence of any commercial or financial relationships that could be construed as a potential conflict of interest.
Acknowledgments
We acknowledge the Nutrient load to inland waters (FOKUS II) project of the Natural Resources Institute Finland and the Freshwater Centre of the Finnish Environment Institute for access to their nutrient loading model VEMALA. Further thanks belong to the personnel of the Geological Survey of Finland for sediment sampling, magnetic susceptibility measurements, and diatom slide preparation. We also thank Tuomas Kauti for the bedrock interpretation of the study area and the bedrock map and Anssi Kinnunen for the Python script.
Abbreviations
CCA, Canonical correspondence analysis; CD ratio, Chrysophyte cyst to diatom ratio; DBA/LA, Drainage basin area per lake area; DBA/LV, Drainage basin area per lake volume; DCA, Detrended correspondence analysis; DI-TP, Diatom-inferred total phosphorus; DOC, Dissolved organic carbon; EC, Electrical conductivity; RMSEP, Root mean squared error of prediction; SCD, Squared chord distance; SD unit, Standard deviation unit; TN, Total nitrogen; TP, Total phosphorus; WA-PLS, Weighted averaging partial least squares regression.
References
Ahti, T., Hämet-Ahti, L., and Jalas, J. (1968). Vegetation zones and their sections in northwestern Europe. Ann. Bot. Fenn. 5, 169–211.
Anderson, N. J. (2014). Landscape disturbance and lake response: temporal and spatial perspectives. Freshw. Rev. 7, 77–120. doi: 10.1608/FRJ-7.2.811
Battarbee, R. W., Jones, V. J., Flower, R. J., Cameron, N. G., Bennion, H., Carvalho, L., et al. (2001). “Diatoms,” in Tracking Environmental Change Using Lake Sediments, Volume 3, Terrestrial, Algal, and Siliceous Indicators, eds J. P. Smol, H. J. B. Birks and W. M. Last (Dordrecht: Kluwer Academic Publishers), 155–202.
Battarbee, R. W., Morley, D., Bennion, H., Simpson, G. L., Hughes, M., and Bauere, V. (2011). A palaeolimnological meta-database for assessing the ecological status of lakes. J. Paleolimnol. 45, 405–414. doi: 10.1007/s10933-010-9417-5
Bayley, S. E., Creed, I. F., Sass, G. Z., and Wong, A. S. (2007). Frequent regime shifts in trophic states in shallow lakes on the Boreal Plain: Alternative “unstable” states. Limnol. Oceanogr. 52, 2002–2012. doi: 10.4319/lo.2007.52.5.2002
Boeff, K. A., Strock, K. E., and Saros, J. E. (2016). Evaluating planktonic diatom response to climate change across three lakes with differing morphometry. J. Paleolimnol. 56, 33–47. doi: 10.1007/s10933-016-9889-z
Brown, R. E., Nelson, S. J., and Saros, J. E. (2017). Paleolimnological evidence of the consequences of recent increased dissolved organic carbon (DOC) in lake of the northeastern USA. J. Paleolimnol. 57, 19–35. doi: 10.1007/s10933-016-9913-3
Callisto, M., Molozzi, J., and Barbosa, J. L. E. (2014). “Eutrophication of lakes,” in Eutrophication: Causes, Consequences and Control, Volume 2, eds A. A. Ansari and S. G. Sarvajeet (Dordrecht: Springer), 55–71.
Davidson, T. A., and Jeppesen, E. (2013). The role of palaeolimnology in assessing eutrophication and its impacts on lakes. J. Paleolimnol. 49, 391–410. doi: 10.1007/s10933-012-9651-0
Dearing, J. A., and Jones, R. T. (2003). Coupling temporal and spatial dimensions of global sediment flux through lake and marine sediment records. Glob. Planet. Change 39, 147–168. doi: 10.1016/S0921-8181(03)00022-5
Dillon, P. J., and Molot, L. A. (1997). Effect of landscape form on export of dissolved organic carbon, iron, and phosphorus from forested stream catchments. Water Resour. Res. 33, 2591–2600. doi: 10.1029/97WR01921
Dong, X., Bennion, H., Maberly, S. C., Sayer, C. D., Simpson, G. L., and Battarbee, R. W. (2012). Nutrients exert a stronger control than climate on recent diatom communities in Esthwaite water: evidence from monitoring and palaeolimnological records. Freshw. Biol. 57, 2044–2056. doi: 10.1111/j.1365-2427.2011.02670.x
Dubois, N., Saulnier-Talbot, É., Mills, K., Gell, P., Battarbee, R., Bennion, H., et al. (2017). First human impacts and responses of aquatic systems: a review of palaeolimnological records from around the world. Anthropocene Rev. 5, 28–68. doi: 10.1177/2053019617740365
Dugan, H. A., Bartlett, S. L., Burke, S. M., Doubek, J. P., Krivak-Tetley, F. E., Skaff, N. K., et al. (2017). Salting our freshwater lakes. Proc. Nat. Acad. Sci. U.S.A. 114, 4453–4458. doi: 10.1073/pnas.1620211114
Engström, D. R., Fritz, S. C., Almendiger, J. E., and Juggins, S. (2000). Chemical and biological trends during lake evolution in recently deglaciated terrain. Nature 408, 161–166. doi: 10.1038/35041500
Finnish Environment Institute (2014a). VALUE-Valuma-Aluejako (Ehdotus). VALUE – Valuma-Alueen Rajaustyökalu KM10. [Dataset]. Available online at: http://paikkatieto.ymparisto.fi/value/
Finnish Environment Institute (2014b). Corine Land Cover 2012. VALUE – Valuma-Alueen Rajaustyökalu KM10. Inspire ID: 1002025. [Dataset]. Available online at: http://paikkatieto.ymparisto.fi/value/
Finnish Environment Institute (2016). Pintavesien Tilan Tietojärjestelmä, Vedenlaatu PIVET. Ympäristötiedon Hallintajärjestelmä Hertta. [Dataset]. Available online at: http://www.syke.fi/fi-FI/Avoin_tieto/Ymparistotietojarjestelmat
Fritz, S. C., and Anderson, N. J. (2013). The relative influences of climate and catchment processes on Holocene lake development in glaciated regions. J. Paleolimnol. 49, 349–362. doi: 10.1007/s10933-013-9684-z
Geological Survey of Finland (1993). Superficial Deposits of Finland 1:1 000 000. Hakku Service for Finnish Spatial Data, Documents, and Metadata. Version 1.0. [Dataset]. Available online at: https://hakku.gtk.fi/en/locations/search
Geological Survey of Finland (2012). Bedrock of Finland 1:200 000 – DigiKP200. Hakku Service for Finnish Spatial Data, Documents, and Metadata. Version 1.0. Resource Identifier: 1000161. [Dataset]. Available online at: https://hakku.gtk.fi/en/locations/search
Ginn, B. K., Rajaratnam, T., Cumming, B. F., and Smol, J. P. (2015). Establishing realistic management objectives for urban lakes using paleolimnological techniques: an example from Halifax region (Nova Scotia, Canada). Lake Reserv. Manag. 31, 92–108. doi: 10.1080/10402381.2015.1013648
Gottschalk, S. (2011). EU reference conditions in Swedish lakes identified with diatoms as palaeoindicators – a review. Boreal Environ. Res. 16, 473–494.
Guiry, M. D., and Guiry, G. M. (2018). AlgaeBase. World-Wide Electronic Publication; National University of Ireland, Galway. Available online at: http://www.algaebase.org (Accessed March 7, 2018).
Hammer, Ø., Harper, D. A. T., and Ryan, P. D. (2001). PAST: Paleontological statistics software package for education and data analysis. Palaeontol. Electronica 4, 1–9.
Hargan, K. E., Nelligan, C., Jeziorski, A., Rühland, K. M., Paterson, A. M., Keller, W., et al. (2016). Tracking the long-term responses of diatoms and cladocerans to climate warming and human influences across lakes of the ring of fire in the far North of Ontario, Canada. J. Paleolimnol. 56, 153–172. doi: 10.1007/s10933-016-9901-7
Heikkilä, M., Edwards, T. W. D., Seppä, H., and Sonninen, E. (2010). Sediment isotope tracers from Lake Saarikko, Finland, and implications for Holocene hydroclimatology. Quat. Sci. Rev. 29, 2146–2160. doi: 10.1016/j.quascirev.2010.05.010
Heikkilä, M., and Seppä, H. (2003). A 11,000yr palaeotemperature reconstruction from the southern boreal zone in Finland. Quat. Sci. Rev. 22, 541–554. doi: 10.1016/S0277-3791(02)00189-0
Hill, M. O., and Gauch, H. G. Jr. (1980). Detrended correspondence analysis: an improved ordination technique. Vegetatio 42, 47–58. doi: 10.1007/BF00048870
Houk, V. (2003). Atlas of Freshwater Centric Diatoms With a Brief Key and Descriptions, Part I: Melosiraceae, Orthosiraceae, Paraliaceae and Aulacoseiraceae. Czech Phycology Supplement 1. Olomouc: Czech Phycological Society.
Hurlbert, S. H. (1971). The nonconcept of species diversity: a critique and alternative parameters. Ecology 52, 577–586. doi: 10.2307/1934145
Huttunen, I., Huttunen, M., Piirainen, V., Korppoo, M., Lepistö, A., Räike, A., et al. (2016). A national-scale nutrient loading model for Finnish watersheds – VEMALA. Environ. Model Assess. 21, 83–109. doi: 10.1007/s10666-015-9470-6
Jenny, J.-P., Francus, P., Normandeau, A., Lapointe, F., Perga, M.-E., Ojala, A., et al. (2016). Global spread of hypoxia in freshwater ecosystems during the last three centuries is caused by rising local human pressure. Global Change Biol. 22, 1481–1489. doi: 10.1111/gcb.13193
Jeppesen, E., Meerhoff, M., Davidson, T. A., Trolle, D., Søndergaard, M., Lauridsen, T. L., et al. (2014). Climate change impacts on lakes: an integrated ecological perspective based on a multi-faceted approach, with special focus on shallow lakes. J. Limnol. 73, 84–107. doi: 10.4081/jlimnol.2014.844
Johnson, B. E., Noble, P. J., Heyvaert, A. C., Chandra, S., and Karlin, R. (2018). Anthropogenic and climatic influences on the diatom flora within the fallen leaf lake watershed, Lake Tahoe Basin, California over the last millennium. J. Paleolimnol. 59, 159–173. doi: 10.1007/s10933-017-9961-3
Juggins, S. (2007). C2 Version 1.5 User Guide, Software for Ecological and Palaeoecological Data Analysis and Visualization. Newcastle upon Tyne: University of Newcastle.
Juggins, S. (2013). Quantitative reconstructions in palaeolimnology: new paradigm or sick science? Quat. Sci. Rev. 64, 20–32. doi: 10.1016/j.quascirev.2012.12.014
Juggins, S., Anderson, N. J., Ramstack Hobbs, J. M., and Heathcote, A. J. (2013). Reconstructing epilimnetic total phosphorus using diatoms: statistical and ecological constraints. J. Paleolimnol. 49, 373–390. doi: 10.1007/s10933-013-9678-x
Juggins, S., and Birks, H. J. B. (2012). “Quantitative environmental reconstructions from biological data,” in Tracking Environmental Change Using Lake Sediments. Volume 5: Data Handling and Numerical Techniques, eds H. J. B. Birks, A. F. Lotter, S. Juggins, and J. P. Smol (Dordrecht: Springer Science+Business media B.V.), 431–494.
Kansanen, P. H., Jaakkola, T., Kulmala, S., and Suutarinen, R. (1991). Sedimentation and distribution of gamma-emitting radionuclides in bottom sediments of southern Lake Päijänne, Finland, after the Chernobyl accident. Hydrobiologia 222, 121–140. doi: 10.1007/BF00006100
Kauppila, T., Kanninen, A., Viitasalo, M., Räsänen, J., Meissner, K., and Mattila, J. (2012). Comparing long term sediment records to current biological quality element data – implications for bioassessment and management of a eutrophic lake. Limnologica 42, 19–30. doi: 10.1016/j.limno.2011.07.001
Kaushal, S. S., Likens, G. E., Pace, M. L., Utz, R. M., Haq, S., Gorman, J., et al. (2018). Freshwater salinization syndrome on a continental scale. Proc. Nat. Acad. Sci. U.S.A. 115, E574–E583. doi: 10.1073/pnas.1711234115
Khan, M. N., and Mohammad, F. (2014). “Eutrophication: challenges and solutions,” in Eutrophication: Causes, Consequences and Control, Vol. 2, eds A. A. Ansari and S. G. Sarvajeet (Dordrecht: Springer), 1–15.
Kissman, C. E. H., Williamson, C. E., Rose, K. C., and Saros, J. E. (2013). Response of phytoplankton in an alpine lake to inputs of dissolved organic matter through nutrient enrichment and trophic forcing. Limnol. Oceanogr. 58, 867–880. doi: 10.4319/lo.2013.58.3.0867
Korhonen, J. (2006). Long-term changes in lake ice cover in Finland. Hydrol. Res. 37, 347–363. doi: 10.2166/nh.2006.019
Krammer, K. (1992). Pinnularia eine Monographie der Europäischen Taxa. Bibliotheca Diatomologica, Band 26. Berlin-Stuttgart: Gebrüder Borntraeger.
Krammer, K., and Lange-Bertalot, H. (1986). “Bacillariophyceae 1: Naviculaceae,” in Süsswasserflora von Mitteleuropa, Band 2, eds H. Ettl, J. Gerloff, H. Heyning and D. Mollenhauer (Stuttgart: Gustav Fischer Verlag), 867.
Krammer, K., and Lange-Bertalot, H. (1988). “Bacillariophyceae 2: Bacillariaceae, Epithemiaceae, Surirellaceae,” in Süsswasserflora von Mitteleuropa, Band 2, eds H. Ettl, J. Gerloff, H. Heyning, and D. Mollenhauer (Stuttgart: Gustav Fischer Verlag), 596.
Krammer, K., and Lange-Bertalot, H. (1991a). “Bacillariophyceae 3: Centrales, Fragilariaceae, Eunotiaceae,” in Süsswasserflora von Mitteleuropa, Band 2, eds H. Ettl, G. Gärtner, J. Gerloff, H. Heyning, and D. Mollenhauer (Stuttgart: Gustav Fischer Verlag), 576.
Krammer, K., and Lange-Bertalot, H. (1991b). “Bacillariophyceae 4: achnanthaceae, Kritishe Ergänzungen zu Navicula (Lineolatae) und Gomphonema,”in Süsswasserflora von Mitteleuropa, Band 2, eds H. Ettl, J. Gerloff, H. Heyning, and D. Mollenhauer (Stuttgart: Gustav Fischer Verlag), 536.
Kratz, T. K., Webster, K. E., Bowser, C. J., Magnuson, J. J., and Benson, B. J. (1997). The influence of landscape position on lakes in northern Wisconsin. Freshw. Biol. 37, 209–217. doi: 10.1046/j.1365-2427.1997.00149.x
Kratz, T. K., Webster, K. E., Riera, J. L., Lewis, D. B., and Pollard, A. I. (2006). “Making sense of the landscape: geomorphic legacies and the landscape position of lakes”, in Long-Term Dynamics of Lakes in the Landscape – Long-Term Ecological Research on North Temperate Lakes, eds J. J. Magnuson, T. K. Kratz, and B. J. Benson (New York, NY: Oxford University Press), 49–66.
Kukkonen, E., and Sahala, L. (1988). “Moreenikerrostumat”, in Quaternary Deposits in the Iisalmi Map-Sheet Area, Geological Map of Finland 1:100 000 Sheet 3341, Explanation to the Maps of Quaternary Deposits, eds E. Kukkonen and L. Sahala (Espoo: Geological Survey of Finland), 11–15.
Lange-Bertalot, H., and Moser, G. (1994). Brachysira, Monographie der Gattung. Bibliotheca Diatomologica, Band 29. Belin-Stuttgart: Gebrüder Borntraeger.
Lintinen, P. (1995). Origin and physical characteristics of till fines in Finland. Geol. Surv. Finland Bull. 379, 1–83.
Lunkka, J. P., Johansson, P., Saarnisto, M., and Sallasmaa, O. (2004). “The glaciation of Finland,” in Quaternary Glaciations–Extent and Chronology, Part I: Europe, eds J. Ehlers and P. L. Gibbard (Amsterdam: Elsevier, B.V.), 93–100.
Miettinen, J. O., Kukkonen, M., and Simola, H. (2005). Hindcasting baseline values for water colour and total phosphorus concentration in lakes using sedimentary diatoms – implications for lake typology in Finland. Boreal Environ. Res. 10, 31–43.
Mikkonen, S., Laine, M., Mäkelä, H. M., Gregow, H., Tuomenvirta, H., Lahtinen, M., et al. (2015). Trends in the average temperature in Finland, 1847-2013. Stoch. Environ. Res. Risk Assess. 29, 1521–1529. doi: 10.1007/s00477-014-0992-2
Montleith, D. T., Stoddard, J. L., Evans, C. D., de Wit, H. A., Forsius, M., Høgåsen, T., et al. (2007). Dissolved organic carbon trends resulting from changes in atmospheric deposition chemistry. Nature 450, 537–540. doi: 10.1038/nature06316
Pirinen, P., Simola, H., Aalto, J., Kaukoranta, J.-P., Karlsson, P., and Ruuhela, R. (2012). Climatological Statistics of Finland 1981-2010. Finnish Meteorological Institute Reports 2012:1. Finnish Meteorological Institute, Helsinki.
Plach, J. M., Ferone, J.-M., Gibbons, Z., Smerdon, B. D., Mertens, A., Mendoza, C. A., et al. (2016). Influence of glacial landform hydrology on phosphorus budgets of shallow lakes on the Boreal Plain, Canada. J. Hydrol. 535, 191–203. doi: 10.1016/j.jhydrol.2016.01.041
Porder, S., and Ramachandran, S. (2013). The phosphorus concentration of common rocks – a potential driver of ecosystem P status. Plant Soil 367, 41–55. doi: 10.1007/s11104-012-1490-2
Porter, S. D. (2008). Algal attributes: an autoecological classification of algal taxa collected by the National Water-Quality Assessment Program. US Geological Survey Data Series 329. Reston, VA: U.S. Geological Survey.
Prepas, E. E., Planas, D., Gibson, J. J., Vitt, D. H., Prowse, T. D., Dinsmore, W. P., et al. (2001). Landscape variables influencing nutrients and phytoplankton communities in Boreal Plain lakes of northern Alberta: a comparison of wetland- and upland-dominated catchments. Can. J. Fish. Aquat. Sci. 58, 1286–1299. doi: 10.1139/f01-081
Punkari, M. (1997). Glacial and glaciofluvial deposits in the interlobate areas of the Scandinavian ice sheet. Quat. Sci. Rev. 16, 741–753. doi: 10.1016/S0277-3791(97)00020-6
Putkinen, N., Eyles, N., Putkinen, S., Ojala, A. E. K., Palmu, J.-P., Sarala, P., et al. (2017). High-resolution LiDAR mapping of glacial landforms and ice stream lobes in Finland. Bull. Geol. Soc. Finl. 89, 64–81. doi: 10.17741/bgsf/89.2.001
Ramstack, J. M., Fritz, S. C., and Engstrom, D. R. (2004). Twentieth century water quality trends in Minnesota lakes compared with presettlement variability. Can. J. Fish. Aquat. Sci. 61, 561–576. doi: 10.1139/f04-015
Räsänen, J., Kauppila, T., and Salonen, V.-P. (2006). Sediment-based investigation of naturally or historically eutrophic lakes – implications for lake management. J. Environ. Manage. 79, 253–265. doi: 10.1016/j.jenvman.2005.08.001
Read, J. S., and Rose, K. C. (2013). Physical responses of small temperate lakes to variation in dissolved organic carbon concentrations. Limnol. Oceanogr. 58, 921–931. doi: 10.4319/lo.2013.58.3.0921
Rose, N. L., Morley, D., Appleby, P. G., Battarbee, R. W., Alliksaar, T., Guilizzoni, P., et al. (2011). Sediment accumulation rates in European lakes since AD 1850: trends, reference conditions and exceedance. J. Paleolimnol. 45, 447–468. doi: 10.1007/s10933-010-9424-6
Rühland, K. M., Paterson, A. M., and Smol, J. P. (2015). Lake diatom responses to warming: reviewing the evidence. J. Paleolimnol. 54, 1–35. doi: 10.1007/s10933-015-9837-3
Rühland, K., Paterson, A., and Smol, J. P. (2008). Hemispheric-scale patterns of climate-related shifts in planktonic diatoms from North American and European Lakes. Global Change Biol. 14, 2740–2754. doi: 10.1111/j.1365-2486.2008.01670.x
Saarnisto, M. (2000). “Shoreline displacement and emergence of lake basins,” in Carbon in Finnish lake Sediments, Geological Survey of Finland Special Paper 29, ed H. Pajunen (Espoo: Geological Survey of Finland), 25–34.
Sandgren, P., and Snowball, I. (2001). “Application of mineral magnetic techniques to paleolimnology,” in Tracking Environmental Change Using Lake Sediments. Volume 2: Physical and Chemical Techniques, eds W. M. Last and J. P. Smol (Dordrecht: Kluwer Academic Publishers), 1–21.
Saros, J. E., Michel, T. J., Interlandi, S. J., and Wolfe, A. P. (2005). Resource requirements of Asterionella formosa and Fragilaria crotonensis in oligotrophic alpine lakes: implications for recent phytoplankton community reorganizations. Can. J. Fish. Aquat. Sci. 62, 1681–1689. doi: 10.1139/f05-077
Sass, G. Z., Creed, I. F., and Devito, K. J. (2008). Spatial heterogeneity in trophic status of shallow lakes on the Boreal Plain: influence of hydrologic setting. Water Resour. Res. 44:W08444. doi: 10.1029/2007WR006311
Saulnier-Talbot, É. (2016). Paleolimnology as a tool to achieve environmental sustainability in the Anthropocene: an overview. Geosciences 6:26. doi: 10.3390/geosciences6020026
Simola, H., and Arvola, L. (2005). “Lakes of Northern Europe,” in The Lakes Handbook, Volume 2: Lake Restoration and Rehabilitation, eds P. E. O'Sullivan and C. S. Reynolds (Oxford: Blackwell Publishing Ltd), 117–158.
Simpson, G. L. (2012). “Analogue methods in palaeolimnology,” in Tracking Environmental Change Using Lake Sediments, Vol. 5: Data Handling and Numerical Techniques, eds H. J. B. Birks, A. F. Lotter, S. Juggins and J. P. Smol (Dordrecht: Springer), 495–522.
Sivarajah, B., Rühland, K. M., Labaj, A. L., Paterson, A. M., and Smol, J. P. (2016). Why is the relative abundance of Asterionella formosa increasing in a Boreal Shield lake as nutrient levels decline? J. Paleolimnol. 55, 357–367. doi: 10.1007/s10933-016-9886-2
Smith, V. H., and Schindler, D. W. (2009). Eutrophication science: where do we go from here? Trends. Ecol. Evolut. 24, 201–207. doi: 10.1016/j.tree.2008.11.009
Smol, J. P. (2008). Pollution of Lakes and Rivers: a Paleoenvironmental Perspective, 2nd Edn. Oxford: Blackwell Publishing Ltd.
Smol, J. P. (2016). Arctic and sub-arctic shallow lakes in a multiple-stressor world: a paleoecological perspective. Hydrobiologia 778, 253–272. doi: 10.1007/s10750-015-2543-3
Soininen, A. (1961). The Colonization of Northern Savo in the 15th and 16th Centuries. Historiallisia Tutkimuksia LVIII. Helsinki: Suomen historiallinen seura. [in Finnish, summary in English].
Soininen, A. (1974). Old Traditional Agriculture in Finland in the 18th and 19th Centuries. Historiallisia Tutkimuksia 96. Helsinki: Suomen historiallinen seura. [in Finnish, summary in English].
Spaulding, S. A., Lubinski, D. J., and Potapova, M. (2010). Diatoms of the United States. Availablie online at: http://westerndiatoms.colorado.edu. (Accessed 7 March, 2018).
Spearman, C. (1904). The proof and measurement of association between two things. Am. J. Psychol. 15, 72–101. doi: 10.2307/1412159
Taavitsainen, J.-P., Simola, H., and Grönlund, E. (1998). Cultivation history beyond the periphery: early agriculture in the North European boreal forest. J. World Prehist. 12, 199–253. doi: 10.1023/A:1022398001684
Tammelin, M., Kauppila, T., and Viitasalo, M. (2017). Factors controlling recent diatom assemblages across a steep local nutrient gradient in central-eastern Finland. Hydrobiologia 799, 309–325. doi: 10.1007/s10750-017-3229-9
ter Braak, C. J. F. (1986). Canonical correspondence analysis: a new eigenvector technique for multivariate direct gradient analysis. Ecology 67, 1167–1179. doi: 10.2307/1938672
ter Braak, C. J. F., and Šmilauer, P. (2002). CANOCO Reference Manual and CanoDraw for Windows User's Guide: Software for Canonical Community Ordination (version 4.5). Ithaca: Microcomputer Power.
ter Braak, C. J. F., and Juggins, S. (1993). Weighted averaging partial least squares regression (WA-PLS): an improved method for reconstructing environmental variables from species assemblages. Hydrobiologia 269, 485–502. doi: 10.1007/BF00028046
ter Braak, C. J. F., and Prentice, I. C. (1988). A theory of gradient analysis. Adv. Ecol. Res. 18, 272–317. doi: 10.1016/S0065-2504(08)60183-X
Thompson, R., Battarbee, R. W., O'Sullivan, P. E., and Oldfield, F. (1975). Magnetic susceptibility of lake sediments. Limnol. Oceanogr. 20, 687–698.
Vaasjoki, M., Korsman, K., and Koistinen, T. (2005). “Overview”, in The Precambrian geology of Finland – Key to the Evolution of the Fennoscandian Shield, eds M. Lehtinen, P. A. Nurmi and O. T. Rämö (Amsterdam: Elsevier, B.V.), 1–18.
Väliranta, M., Weckström, J., Siitonen, S., Seppä, H., Alkio, J., Juutinen, S., et al. (2011). Holocene aquatic change in boreal vegetation zone of northern Finland. J. Paleolimnol. 45, 339–352. doi: 10.1007/s10933-011-9501-5
Vallinkoski, V.-M., Miettinen, T., and Aalto, J. (2016). Vesien Tila Hyväksi Yhdessä, Pohjois-Savon Vesienhoidon Toimenpideohjelma Vuosille 2016–2021. Pohjois-Savon elinkeino-, liikenne- ja ympäristökeskus, Raportteja 1/2016, Juvenes Print Oy.
Werner, P., and Smol, J. P. (2005). Diatom-environmental relationships and nutrient transfer functions from contrasting shallow and deep limestone lakes in Ontario, Canada. Hydrobiologia 533, 145–173. doi: 10.1007/s10750-004-2409-6
Wetzel, R. G. (2001). Limnology – Lake and River Ecosystems, 3rd Edn. San Diego, CA: Academic Press.
Weyhenmeyer, G. A., and Karlsson, J. (2009). Nonlinear response of dissolved organic carbon concentrations in boreal lakes to increasing temperatures. Limnol. Oceanogr. 54, 2513–2519. doi: 10.4319/lo.2009.54.6_part_2.2513
Whitmore, T. J., Lauterman, F. M., Smith, K. E., and Riedinger-Whitmore, M. A. (2015). Limnetic total phosphorus transfer functions for lake management: considerations about their design, use, and effectiveness. Front. Ecol. Evol. 3:107. doi: 10.3389/fevo.2015.00107
Keywords: diatoms, naturally eutrophic lakes, fine-grained till, basin morphology, hydrologic landscape, pre- to post-human disturbance change, eutrophication, salinization
Citation: Tammelin M and Kauppila T (2018) Quaternary Landforms and Basin Morphology Control the Natural Eutrophy of Boreal Lakes and Their Sensitivity to Anthropogenic Forcing. Front. Ecol. Evol. 6:65. doi: 10.3389/fevo.2018.00065
Received: 28 March 2018; Accepted: 30 April 2018;
Published: 18 May 2018.
Edited by:
Christopher Carcaillet, Ecole Pratique des Hautes Etudes, Université de Sciences Lettres de Paris, FranceReviewed by:
Patrick Rioual, Institute of Geology & Geophysics (CAS), ChinaJohn Smol, Queen's University, Canada
Copyright © 2018 Tammelin and Kauppila. This is an open-access article distributed under the terms of the Creative Commons Attribution License (CC BY). The use, distribution or reproduction in other forums is permitted, provided the original author(s) and the copyright owner are credited and that the original publication in this journal is cited, in accordance with accepted academic practice. No use, distribution or reproduction is permitted which does not comply with these terms.
*Correspondence: Mira Tammelin, mira.tammelin@utu.fi