- 1Department of Biology, Pennsylvania State University, University Park, PA, United States
- 2Division of Biology and Biological Engineering, California Institute of Technology, Pasadena, CA, United States
- 3Department of Invertebrate Zoology, National Museum of Natural History, Smithsonian Institution, Washington, DC, United States
- 4School of Biological Sciences, The Swire Institute of Marine Science, The University of Hong Kong, Pokfulam, Hong Kong
- 5Unidad Académica de Sistemas Arrecifales Puerto Morelos, Instituto de Ciencias del Mar y Limnología, Universidad Nacional Autónoma de México, Mexico City, Mexico
- 6National Systematics Laboratory of NOAA's Fisheries Service, National Museum of Natural History, Smithsonian Institution, Washington, DC, United States
- 7Smithsonian Marine Station, Fort Piece, FL, United States
- 8Departamento de Zoologia, Instituto de Biociências, Universidade de São Paulo, São Paulo, Brazil
- 9Department of Biology, University of Mississippi, Oxford, MS, United States
- 10Department of Zoology & Neurobiology, Ruhr-University Bochum, Bochum, Germany
- 11School of Cancer and Pharmaceutical Sciences, King's College London, London, United Kingdom
- 12Centro de Biologia Marinha, Universidade de São Paulo, São Sebastião, Brazil
- 13Biology Department, University of North Carolina at Chapel Hill, Chapel Hill, NC, United States
- 14Department of Biology, University of Texas at Arlington, Arlington, TX, United States
- 15Whitney Laboratory for Marine Bioscience, University of Florida, St. Augustine, FL, United States
- 16Department of Biotechnology, Graduate School of Engineering, Osaka University, Osaka, Japan
- 17Departamento de Ciências Biológicas, Faculdade de Ciências e Letras de Assis, Unesp Universidade Estadual Paulista, Assis, Brazil
- 18TropWATER and College of Marine & Environmental Sciences, James Cook University, Townsville, QLD, Australia
- 19Department of Botany, University of British Columbia Vancouver, Vancouver, BC, Canada
- 20U.S. National Oceanic & Atmospheric Administration, National Ocean Service, National Centers for Coastal Ocean Science, Charleston, SC, United States
- 21Odum School of Ecology, University of Georgia, Athens, GA, United States
- 22Smithsonian Tropical Research Institute, Smithsonian Institution, Washington, DC, United States
The upside-down jellyfish Cassiopea xamachana (Scyphozoa: Rhizostomeae) has been predominantly studied to understand its interaction with the endosymbiotic dinoflagellate algae Symbiodinium. As an easily culturable and tractable cnidarian model, it is an attractive alternative to stony corals to understanding the mechanisms driving establishment and maintenance of symbiosis. Cassiopea is also unique in requiring the symbiont in order to complete its transition to the adult stage, thereby providing an excellent model to understand symbiosis-driven development and evolution. Recently, the Cassiopea research system has gained interest beyond symbiosis in fields related to embryology, climate ecology, behavior, and more. With these developments, resources including genomes, transcriptomes, and laboratory protocols are steadily increasing. This review provides an overview of the broad range of interdisciplinary research that has utilized the Cassiopea model and highlights the advantages of using the model for future research.
Introduction
The upside-down jellyfish Cassiopea is a benthic scyphozoan (Rhizostomeae) commonly found in tropical and sub-tropical shallow coastal ecosystems, such as mangroves and seagrass beds. Cassiopea spp. are unique among scyphomedusae in that their characteristic flat exumbrella rests on the sea bottom, while their convex subumbrella and oral arms face upwards. High light penetrance is important for species within this genus, as the jellyfish hosts one or more photosynthetic dinoflagellate species of the genus Symbiodinium (Hofmann et al., 1996; Lampert, 2016). Similar to their coral relatives, nutrient exchange is a key component supporting this cnidarian-dinoflagellate mutualism (Hofmann and Kremer, 1981; Welsh et al., 2009; Freeman et al., 2016). In addition, Cassiopea spp. rely on the symbionts as a developmental trigger (Hofmann et al., 1978; Colley and Trench, 1985). A fascination with these requisite traits of the upside-down jellyfish has fueled studies on the Cassiopea-Symbiodinium interaction since the 1980s (see recent review by Lampert, 2016). While its efficacy as a system for symbiosis research is well known in the literature, Cassiopea is gaining momentum as a model species in other areas. In mathematics, the symmetric morphology and relatively simple neuromuscular system of the upside-down jellyfish make it an ideal system from which to develop computational fluid dynamic and neuromechanical models (Passano, 2004; Hamlet et al., 2011; Santhanakrishnan et al., 2012). Additionally, Cassiopea has been presented as a possible model organism for the study of behavioral biology as highlighted by recent developments with sleep behavior (Nath et al., 2017; Figure 1). Likewise, this jellyfish genus has been gaining traction as a biomonitor/bioindicator species, with applications for coastal ecosystem management (Templeman and Kingsford, 2012; Epstein et al., 2016; Klein et al., 2016a, 2017). The relative simplicity of culturing Cassiopea polyps and medusae makes this genus a highly amenable laboratory system. With newly established Cassiopea xamachana clonal laboratory lines, and recent advances in affordable genomics tools giving way to draft transcriptome and genome assemblies, the upside-down jellyfish is an appropriate candidate as a model organism. Here, we review Cassiopea research conducted to date and discuss future directions of this highly valuable study system.
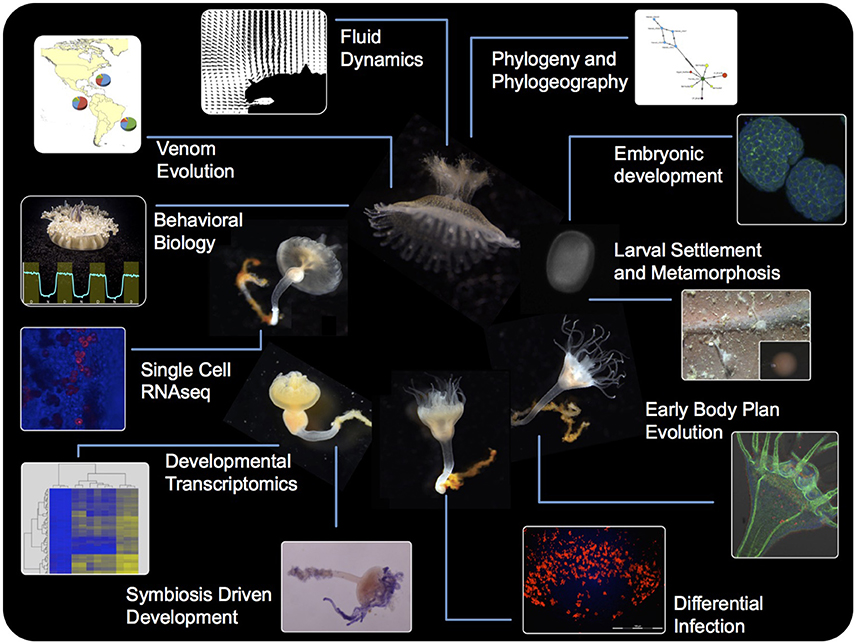
Figure 1. A schematic summarizing the tools and approaches available for the Cassiopea system, highlighting the utility of each life stage toward a multidisciplinary approach to understanding the biology and physiology of this early metazoan.
Evolution and Phylogenetics
The first species of the genus Cassiopea was described from the Caribbean as Medusa frondosa by Pallas (1774), and just one year later a second species from the Red Sea was described as Medusa andromeda (published as a post mortem work; figures published only in Forskål (1776). The generic name Cassiopea was proposed for all jellyfish with foliaceous appendages in 1810 (Péron and Lesueur, 1810). Members of the genus Cassiopea were treated as a family by Tilesius (1831), and the current spelling appeared ~30 years later (Agassiz, 1862). The species-level relationships of the genus Cassiopea are still unresolved since most of the taxonomic studies are based on overlapping, plastic, and polymorphic features (Table 1; Mayer, 1910; Kramp, 1961). This is confounded by vague species descriptions with species diagnoses that are descriptive rather than comparative, although morphology has been useful in distinguishing some congeneric species, i.e., Cassiopea frondosa. In total, 24 nominal species, and varieties thereof, have been proposed to date (Table 1), but only 10 species are presently considered to be valid based on molecular data (Holland et al., 2004; Arai et al., 2017; Morandini et al., 2017). These studies show that populations of C. xamachana are potential introduction of Cassiopea andromeda from the Red Sea, thereby grouping the species into a single lineage. These species designations, which are based on kimura 2-parameter pairwise distance, fall within previous estimations for other scyphozoans, ranging from 0.00 to 0.034 for conspecifics, and 0.102–0.234 for congenerics (Holland et al., 2004; Ortman et al., 2010; Gómez Daglio and Dawson, 2017). Analyses with expanded taxon sampling (Bayha et al., 2010; Kayal et al., 2013, 2017) have placed the monogeneric Cassiopea within the family Cassiopeidae, in an unstable position within the clade Kolpophorae, along with representatives of Mastigiidae, Thysanostomatidae, Versurigidae, and Cepheidae (Figure 2). The Kolpophorae belongs to the order Rhizostomeae, a well-supported clade derived from within the scyphozoan order Semaeostomeae (Bayha et al., 2010; Kayal et al., 2013).
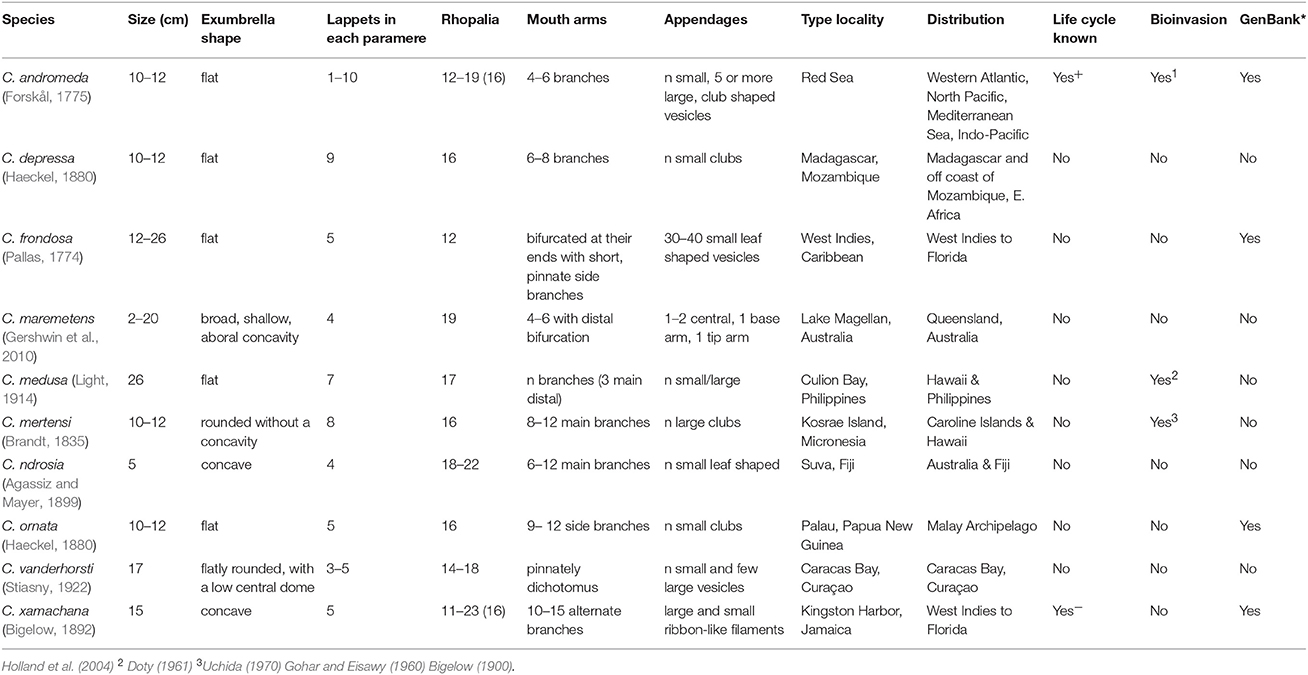
Table 1. Morphological data on valid Cassiopea species, modified from Morandini et al. (2017).
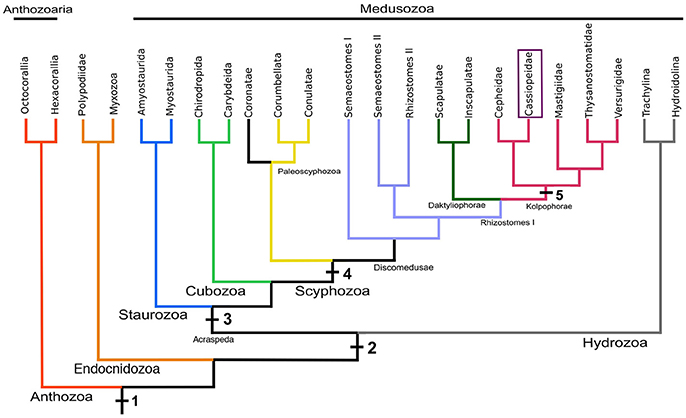
Figure 2. A working hypothesis of Medusozoa phylogeny based on several studies (Bayha et al., 2010; Kayal et al., 2013, 2017), including the monophyletic Scyphozoa, Rhizostomeae, Kolpophorae, and Cassiopeidae. Principal morphological and life cycle characters are plotted on the tree (1, cnidae; 2, medusa stage; 3, rhopalia; 4, strobilation, and ephyra; 5, monodisc strobilation). The topology is not meant to represent a consensus.
While the polyp stage and strobilation of C. xamachana, as well as the polyp stage of C. frondosa, were described by Bigelow (Bigelow, 1892, 1893), a complete description of the life cycle of C. xamachana did not appear in the literature until almost a decade later (Bigelow, 1900). Additional details of the life cycle were later published on a population of C. andromeda from Al-Ghardaqa (Red Sea) (Gohar and Eisawy, 1960). Although the morphology of Cassiopea is unique and known only from extant forms, a fossil dated to the Solnhofen formation lagerstätte (Jurassic, Myogramma speciosum) has a similar arrangement of what appears to be the muscular system (Maas, 1902).
Moving forward, enhanced taxon sampling and character analyses by multi-omics methods on a broad geographical scale will facilitate rigorous testing of phylogenetic hypotheses and assessment of genetic richness related to cryptic species, thereby allowing research on different Cassiopea systems from a comparative evolutionary perspective.
Life History
Cassiopea spp. are gonochoristic brooders, with fixed sex determination, although a case of hermaphroditism has been reported from Hawai'i (Hofmann and Hadfield, 2002). Fertilization has been reported to occur within the gastrovascular cavity of the female (Smith, 1936). The embryos pass from the gastrovascular cavity through the brachial canal out of the secondary mouths, and are subsequently deposited onto the oral disc. Because motile settlement-competent planulae can be found attached to the female's oral disc at any given point, the duration of attachment (i.e., brooding) in the absence of outside disturbance is unknown. Cleavage begins ~1–2 h after the fertilized eggs are visible on the oral disc, and then round, ciliated embryos are present 48 h post-fertilization. By 96 h post-fertilization, elongate ovoid planulae, rotating around the longitudinal axis, swim with clear directionality and are capable of settling and becoming polyps. While a detailed characterization of early embryonic development in Cassiopea is yet to be done, developmental timing appears to be temperature dependent and planula morphology has been well described (Martin and Chia, 1982).
Hox genes are present in C. xamachana (Kuhn et al., 1999; Ferrier and Holland, 2001), and work on other cnidarians suggests these may be involved in patterning the oral-aboral axis. In both the anthozoan Nematostella vectensis and the hydrozoan Clytia hemisphaerica, planula, and polyp stages show differential Hox expression along the oral-aboral axis (Ryan et al., 2007; Chiori et al., 2009). Patterning of the medusa body form remains a more complicated question, as expression of some Hox genes were localized to sensory structures and the bell (Chiori et al., 2009), while others were expressed specifically in oral endoderm. In addition to Hox expression during development, homeobox genes are active during cnidarian regeneration (Schummer et al., 1992) and Cassiopea has gained some attention as a model for regeneration (Cary, 1916; Curtis and Cowden, 1974; Polteva, 1985). Of particular note, all Cassiopea life stages show telomerase activity, with potential elongation of the telomere during the medusa stage (Ojimi et al., 2009; Ojimi and Hidaka, 2010), paving the way for additional research in the genetic mechanisms of regeneration and longevity in non-bilaterian organisms. Future research in Cassiopea development incorporating genomics, transcriptomics, metabolomics, and in situ hybridization will allow further characterization of pre-swimming development, particularly timing and stereotype of gastrulation, and precise identification of morphological characteristics denoting settlement competency. The multi-omics approach will lay the foundations for describing the developmental genes regulating the life history of these scyphozoans and generate testable hypotheses toward understanding the evolution of cnidarian life cycles and bauplans.
Bud Morphogenesis
Polyps of the genus Cassiopea are capable of producing planuloid buds 3–4 mm in length via evagination from the aboral region of the calyx (Van Leishout and Martin, 1992; Figure 3). Bud formation and detachment can occur at a relatively rapid rate, with buds released as frequently as 2–3 per day. Progenitor ectodermal cells are recruited distally and asymmetrically from the site of bud morphogenesis rather than produced by a mitotically active population of cells (Hofmann and Honegger, 1990; Hofmann and Gottlieb, 1991). Planuloid buds form with their future oral pole most proximal to the body. The most distal part of the bud is the future aboral pole, which after detachment will be the leading pole of the swimming bud and the stalk of the future polyp (Curtis and Cowden, 1971). Within 24 h, settled buds will develop a hypostome and up to 16 tentacles, thereby providing the newly developed polyps with the capacity to capture prey. Head (calyx and tentacles) and stalk formation is contingent on settlement to a substrate, with a head-morphogenesis inhibiting signal potentially generated from the aboral pole of the swimming bud (Neumann, 1980). It has been reported that oral halves of transversely bisected buds properly developed the hypostome and tentacles but lacked a stalk, while the aboral halves developed into full scyphistomae (Curtis and Cowden, 1971; Neumann, 1980). Further, treatment of the oral fragment with bud homogenate as well as PKC inhibitors (psychosine, chelerythrine) showed inhibitory effects to head development (Thieme and Hofmann, 2003a,b), suggesting the important involvement of PKC in bud and larval metamorphosis (Fleck and Bischoff, 1992). Conversely, treatments with canathadrin, a serine/threonine protein phosphatase inhibitor, induced development of the head and stalk in scyphistomae, while methionine, homocysteine, trigonelline, nicotinic acid, and cycloleucine treatments all inhibited morphogenesis (Kehls et al., 1999).
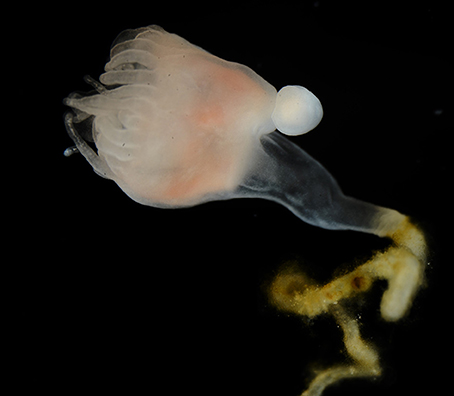
Figure 3. A Cassiopea xamachana poylp with a planuloid bud developing asexually from the aboral region of the calyx.
Settlement and Metamorphosis
Induction of settlement and metamorphosis in cnidarian species, including Cassiopea spp., is contingent on the presence of certain types of bacteria (Hofmann et al., 1978; Neumann, 1979; Leitz, 1997; Vermeij et al., 2009). Both larvae and planuloid buds of Cassiopea spp. settle and metamorphose in response to bacterial biofilm, including Vibrio sp. isolated from an Artemia culture (Hofmann et al., 1978). While the isolated Vibrio sp. showed toxic effects on polyp development, a metabolite (1–10 kDa) that was isolated from the bacteria induced settlement and metamorphosis of Cassiopea planulae (Neumann, 1979). A cholera toxin isolated from Vibrio cholerae was also inductive (Wolk et al., 1985). Collagen digestion by Vibrio alginolyticus also led to the production of inductive fractions, suggesting the involvement of a peptidic cue in polyp development (Hofmann and Brand, 1987). The discovery that certain small molecules and peptides are involved in polyp metamorphosis has led to the design of a synthetic peptide (GPGGPA) with settlement and metamorphosis inductive capacities (Fleck, 1998).
Research efforts attempting to isolate a peptidic cue from Cassiopea's natural substrate, degraded mangrove leaves, resulted in extraction of several bioactive fractions, one of which contained a proline-rich 5.8 kDa peptide capable of inducing settlement and metamorphosis, although the precise amino acid sequence of this peptide or other natural cues have yet to be determined (Fleck and Fitt, 1999; Fleck et al., 1999). While cnidarian species have been shown to settle and metamorphose in response to mono-culture biofilm of multiple bacterial taxa (Tran and Hadfield, 2011), the exact underlying mechanism allowing planulae to react to multiple microbial cues has yet to be fully explored. Variation in induction response to artificial inducers (inorganic ions, phorbolesters, diacylglycerols, tetradecanoyl-phorbol-13 acetate) has been observed between hydrozoan and scyphozoan species (Müller, 1973; Henning et al., 1996; Siefker et al., 2000). The difference in induction response to artificial inducers noted between the moon jellyfish Aurelia aurita and C. xamachana (Fitt et al., 1987; Siefker et al., 2000), for example, are suggestive of potentially differing mechanisms of settlement induction. The evolution of cue specificity and signal transduction mechanisms of cnidarian larvae, and more generally invertebrate larvae, remains a topic requiring further research. The current understanding of Cassiopea species diversity and geographic distribution, coupled with the availability of several lab cultures, offers avenues to conduct comparative studies on the induction response of planulae to both natural and synthetic settlement inducers.
Cassiopea-Symbiodinium Symbiosis
In many scyphozoans, the transition from polyp to medusa, known as strobilation, can be triggered by temperature and other exogenous cues (Spangenberg, 1965; Loeb, 1973; Holst, 2012). Strikingly, strobilation in Cassiopea requires colonization by the endosymbiont Symbiodinium spp. (Gohar and Eisawy, 1960; Hofmann et al., 1996). Without colonization, polyps will not undergo strobilation, although a single strobilation event in aposymbiotic polyps has been reported by Rahat and Adar (1980). Cassiopea spp. are monodisc strobilating scyphozoans, producing a single ephyra at each strobilation event. Strobilation generally occurs over several days, with the first few days characterized by a reduction in tentacle length (due to absorption), followed by the development of a disc-like apical structure bearing rhopalia and a medusoid form; shortly following is constriction by the polyp body resulting in release of the ephyra (Ludwig, 1969; Hofmann et al., 1978). Colonization of the polyp by the symbiont (i.e., dinoflagellate), and subsequent strobilation of an ephyra, happens in several discrete stages. The colonization process begins when symbionts enter the polyp through the mouth and are then phagocytosed by the gastroderm (Colley and Trench, 1983; Fitt and Trench, 1983). Successful colonization of cnidarian cells requires suppression of the host immune response, thereby revealing a link between symbiosis and innate immunity (Miller et al., 2007; Kvennefors et al., 2008). Along with other cnidarians, jellyfish show evidence of producing antimicrobial peptides that indicate their ability to identify between self and non-self (Rinkevich, 2004; Ovchinnikova et al., 2006; Bosch, 2008). This ability would be useful in regulating the host microbial composition as well as symbiont colonization and proliferation, a topic that has garnered significant attention and will benefit from the development of the Cassiopea-Symbiodinium research system (Detournay et al., 2012; Neubauer et al., 2016, 2017).
Events leading to successful colonization post-phagocytosis can be identified by the relative position of the algae within the phagocytosing cell (Colley and Trench, 1985). Initially, symbionts can be found toward the apical end of phagocytic cells and the basal end by day 3 post-entry. Symbiodinium cells that are unable to migrate basally are destroyed by lysosomal fusion. By day 8, host cells containing successful symbionts detach and migrate into the mesoglea where proliferation of symbiont-containing host-cells subsequently occurs (Colley and Trench, 1985). Strobilation takes place ~3 weeks later, depending on several factors including the symbiont species, temperature and food intake (Fitt and Costley, 1998). While the precise cue(s) responsible for initiation of strobilation is unknown, inhibition of photosynthesis with 3-(3,4-dichlorophenyl)-1,1-dimethylurea (DCMU) results in continuation of the process, albeit at lower rates (Hofmann and Kremer, 1981). In a non-symbiotic scyphozoan, A. aurita, initiation of strobilation was found to involve genes from the retinoic acid pathway (Fuchs et al., 2014; Brekhman et al., 2015). In contrast to A. aurita, treatment of Cassiopea polyps with retinoic acid does not result in strobilation (Silverstone et al., 1977; Pierce, 2005; Cabrales-Arellano et al., 2017). Conversely, indomethacin, a drug with structural resemblance to a peptide involved in strobilation in Aurelia induces metamorphosis of C. xamachana polyps to the mature medusa form (Kuniyoshi et al., 2012; Cabrales-Arellano et al., 2017). This observation suggests that while some aspects of the strobilation mechanism might be shared across Scyphozoa (Helm and Dunn, 2017; Yamamori et al., 2017), the evolution of symbiosis within members of the Rhizostomae may have resulted in co-option of other pathways as a developmental trigger. With the availability of transcriptome and genome level sequence data awaiting extensive query, Cassiopea holds promise as an exciting system for the study of symbiosis-mediated development, as well the mechanisms involved in host colonization by its symbionts.
Nutritional Requirements
For symbiotic cnidarians, the essential nature of the symbiotic relationship strongly suggests that heterotrophic feeding from the surrounding waters may not provide nutrients sufficient to cover the carbohydrate and lipids needed for medusa metamorphosis, growth, and sexual reproduction. A major gap in our knowledge of algal-cnidarian symbiosis has been the understanding of the mechanisms underlying the biosynthesis and regulation of carbon flow from symbiont to host (Yellowlees et al., 2008; Davy et al., 2012), and the uptake of such carbon compounds by the host partner. In this context, identifying the chemical form of photosynthates or so-called “mobile compounds” (Trench, 1971; Venn et al., 2008) has proven challenging, although it most likely involves complex metabolic modifications. In other well-known examples of algal-cnidarian symbiotic relationships in scleractinian corals, glycerol is the dominant photosynthate produced (Gattuso et al., 1993; Hagedorn et al., 2010), while glucose is the dominant photosynthate in the sea anemone genus Exaiptasia (formerly Aiptasia) (Burriesci et al., 2012; Molina et al., 2017). Early 14C (radiocarbon) labeling studies in C. andromeda showed that glucose and glycerol were the only two free metabolites detected after short term labeling (3–60 min) in medusa tissue for samples incubated in the light (Hofmann and Kremer, 1981). These experiments showed that labeled glycerol disappeared in <30 min, likely through utilization in lipid synthesis, as suggested by the authors; these findings were later corroborated with findings of a similar study on the sea anemone Exaiptasia (Davy and Cook, 2001). In vivo carbon fixation by the symbiont is highly efficient in C. xamachana, with rates exceeding daily respiration requirements of the host, supporting a growth rate of 3% body weight per day (Verde and McCloskey, 1998; Welsh et al., 2009; Freeman et al., 2016). Contrary to carbon, nitrogen assimilation levels under both photosynthetic and non-photosynthetic conditions are low, suggesting alternative nitrogen sources are essential for life. Interestingly, nitrogen metabolism appears to be largely driven by non-photosynthetic microbes, highlighting the complexity of the Cassiopea holobiont (Freeman et al., 2016). Lipids are also translocated between host and symbiont (Mortillaro et al., 2009; Imbs, 2013; Imbs et al., 2014), but no cue has been found that explains the underlying mechanism or degree of translocation, although lipid composition and concentrations are known to shift under low light conditions in Cassiopea (Mortillaro et al., 2009). With the development of metabolomic tools and technologies such as NanoSIMS (nano-scale resolution chemical imaging mass spectrometry) (Kopp et al., 2013, 2015), Cassiopea offers an approachable and amenable system for the study of nutritional symbiosis. Given that this host can uptake multiple symbionts, future comparative studies conducted on various colonization regimes and conditions will undoubtedly shed light on cnidarian-Symbiodinium symbiosis.
Similar to the topic of nutrient translocation, little is known about the plasticity in jellyfish nutrient cycling. Jellyfish planulae are all thought to be born symbiont-free, and then following settlement the sessile polyps acquire resources through ingestion of zooplankton. However, <10% of essential carbon is provided by heterotrophic means during the adult medusa stage, and decreased dependence on heterotrophy as a nitrogen source is seen with increasing medusa size (Kremer, 2005). In some coral species, a shift from photosynthesis to heterotrophy may be triggered by thermal stress; this trophic switching is believed to enhance host survival in the event of bleaching events (Rodrigues and Grottoli, 2007; Anthony et al., 2009). Under reduced light conditions, a short-term increase in levels of chlorophyll a was measured in Cassiopea, but no evidence of a shift toward heterotrophy was seen that might compensate for the perceived reduction in photosynthetic activity (Mortillaro et al., 2009). In a separate study, providing nutrient supplements to bleached C. xamachana did not prevent a reduction in medusa body mass (McGill and Pomory, 2008), pointing out the limitation of heterotrophic contributions to the overall energy budget.
Behavior
Fluid Dynamics
Jellyfish use bell contractions while swimming and changing direction in the water and to generate water currents that facilitate feeding and nutrient exchange. Both fluid dynamics and related biological relevance of these activated currents to Cassiopea medusae have been studied using empirical data and computational fluid dynamics (CFD) simulations (Hamlet et al., 2011; Santhanakrishnan et al., 2012). Although the pulsing behavior is cyclic (one cycle comprising a single bell contraction and expansion with pauses in between), it results in continuous flow along the substrate toward the medusa and continuous upward flow above the medusa. During contraction, vortices develop at the bell margins that are forced through the oral arms, where they are broken up. The frilly structure of the oral arms of Cassiopea ensures thorough mixing of the water over their surface and secondary mouths before it is pushed into an upward jet propulsion (Hamlet et al., 2011). Recently, a well-resolved three-dimensional CFD model of bell contraction in the upside-down jellyfish was developed to better ascertain the flows generated by these medusae (Hamlet et al., 2011; Hamlet and Miller, 2012; Santhanakrishnan et al., 2012). Implementation of these models presents the possibility of further investigations of the effect of jellyfish aggregations on the local fluid and nutrient dynamics of a habitat.
At the ecosystem level, the pulsing behavior of upside-down jellyfish affects nutrient release from the substrate. Cassiopea's pulsing was shown to drive pore-water (interstitial water) up from the underlying sediment into the water column, allowing these derived nutrients to be used by the jellyfish and other reef organisms (Jantzen et al., 2010). This type of bioturbation (the disturbance of sediment by organisms) is an important mechanism that couples the nutrient-rich sediments with the oligotrophic waters in which coral reefs occur. Cassiopea itself also releases organic matter, which is taken up by zooplankton in the water column (Niggl et al., 2010). Furthermore, studies have demonstrated that the presence of upside-down jellyfish can have an effect on local nutrient dynamics, greatly affecting local oxygen concentration and ammonium regeneration rates in space (between sediment patches with or without jellyfish) as well as in time (given the diurnal cycle of the photosynthetic symbionts; Welsh et al., 2009). These findings validate the notion that Cassiopea jellyfish play an important role in benthic-pelagic coupling of nutrient cycles, and some researchers even refer to the upside-down jellyfish as an ecosystem engineer (Jantzen et al., 2010). Further research is necessary to better understand the intricate role that Cassiopea medusae play in coastal ecosystems and nutrient cycling.
Quiescence
Animal behavior is the product of numerous pathways, integrating both internal and external information to generate a global behavior. The internal state of an animal greatly affects its behavior—aroused animals act differently than those that are asleep. There is a debate as to how global brain states, like sleep, arise. It has been hypothesized that either specialized regions control the switch between wakefulness and sleep (a top-down mechanism), or neural networks have an emergent bias toward certain global states regulated by local circuits (a bottom-up mechanism). The fundamental question of the cellular mechanism controlling the sleep state is now approachable through detailed analysis of simple behavioral states.
Quiescence in the bilaterian model nematode Caenorhabditis elegans appears to be an emergent property; as the result of an internal network bias, it provides evidence against top-down regulation of a global brain state (Nichols et al., 2017). Unlike C. elegans, neurons in Cassiopea, and other cnidarians, are organized in non-centralized radially symmetrical nerve nets, and yet there exists deep conservation among cnidarian and bilaterian neural structure and neurotransmitters. Recently, a sleep-like state was identified in Cassiopea, making it the earliest diverging lineage (as a representative of Cnidaria) in which sleep has been observed (Nath et al., 2017). Cassiopea medusae display the three behavioral characteristics that define a sleep state: quiescence that is rapidly reversible, homeostatic regulation upon sleep deprivation, and a delayed response to sensory stimuli, called sensory depression. This recent recognition of a defined sleep-like state in Cassiopea, despite its lack of centralized nervous system, presents a unique opportunity to determine if sleep is, in fact, an emergent property of the earliest nerve networks. As a early diverging metazoan, Cassiopea is an important model for understanding scyphozoan behavior and the underlying evolutionary mechanisms that have arisen to control behavior in derived animal lineages.
Cassiopea in the Environment
Bioinvasion and Blooms
Molecular phylogenetic analyses demonstrated that some individuals of Cassiopea from the same geographic area fall into different clades, evidencing cryptic species (Arai, 2001; Holland et al., 2004). At least two non-morphologically differentiated lineages of Cassiopea have been identified as alien species (Table 1). Cassiopea spp. have biological characteristics that make them potentially successful bioinvaders, such as their prolific asexual reproduction (Schiariti et al., 2014) and broad tolerance to variations in temperature and salinity (Morandini et al., 2017). The tenacity of Cassiopea spp. is demonstrated by their often well-established presence in disturbed or human-impacted habitats (Stoner et al., 2011). It is not surprising then, that species of Cassiopea have been documented as invasive in the Mediterranean (Galil et al., 1990; Çevik, 2006; Schembri et al., 2010; Bayha and Graham, 2014), Indo-Pacific (Papua New Guinea), Hawai'i (Holland et al., 2004), Australia (Keable and Ahyong, 2016), and Brazil (Morandini et al., 2017). Populations may occasionally reach enormous numbers (Stoner et al., 2011; Morandini et al., 2017) as a function of their environmental condition. With the frequency of these events potentially increasing according to studies on climate change (Richardson et al., 2009; Brotz et al., 2012), it necessitates development of methods for accurate taxonomic identification of cryptic species. These sudden proliferation events can be useful indicators of shifts in environmental conditions. Recent work has shown, to the contrary, that projected increased temperatures combined with increased exposure to UV-B radiation will lead to a general reduction in Cassiopea population size (Klein et al., 2016b). These findings are perplexing given the perception that jellyfish have an exceptional capacity to adapt to and thrive under conditions associated with ocean warming.
Environmental Monitoring and Ecotoxicology
As marine systems worldwide continue to decline from local and global physical, chemical, and biological threats (De'ath et al., 2012; Epstein et al., 2016), the need to manage marine natural resources and reduce these threats is becoming increasingly more critical. Early detection and quantification tools are needed to assess risks that can have adverse impacts on the biological, physiological, and metabolic conditions at the level of the organism and the ecosystem (Markert et al., 2003; Downs, 2005). The use of jellyfish as biomonitors and/or bioindicators previously was not explored, possibly due to historical perceptions of their tolerance for poor environmental conditions and “boom or bust” population fluctuations. Biomonitors are used to derive both qualitative and quantitative information about the environment. There has been recent interest in establishing Cassiopea as a potential biomonitoring tool (i.e., indicator species) for enhancing water quality management. Previous studies have indicated jellyfish are able to accumulate trace metals in concentrations exceeding those present in ambient seawater, and concern exists that these could have potential for transfer up food webs (Romeo et al., 1987; Romeo and Gnassia-Barelli, 1992). For example, copper and zinc accumulate in Cassiopea (Templeman and Kingsford, 2010, 2012, 2015; Epstein et al., 2016) generally within hours to several days and with varying residence times (Fowler et al., 2004), while phosphate concentrations in C. xamachana can reach an order of magnitude greater than those of ambient conditions, even when such levels are undetectable in seawater (Todd et al., 2006). The rapid response of Cassiopea medusae under exposure to metals and nutrients suggests they could serve as a useful indicator tool to detect and quantify short pulses of contaminants/pollutants entering a system, a role achieved by only a few of the current typical biomonitors.
In contrast to biomonitors, bioindicators are defined as organisms that will exhibit a change in structure or function that is linked to the biological effect of a contaminant at the organism, population, community, or ecosystem level (McCarty, 2002; McCarty and Munkittrick, 2008). Bioindicator species are used in laboratory bioassays to establish criteria for ecological sensitivity (e.g., LC50), but there is still a need to identify model organisms for standardization of these assays (Chapman, 1995; Edwards et al., 1996; Van Gestel and Van Brummelen, 1996). There is clear ecological relevance for exploring Cassiopea as a tool for ecotoxicological assessment—briefly defined as the study of effects of toxic chemicals (especially on the population level). As cnidarians, jellyfish occupy a key evolutionary position as early metazoans, serving important ecological roles as predators and prey (Faimali et al., 2014); additionally, they fuel pelagic food webs with dissolved and particulate organic matter (Niggl et al., 2010). Cassiopea offers an additional benefit as a bioindicator, as both the host jellyfish and dinoflagellate symbiont responses to environmental conditions can be assessed. Studies conducted on different life stages of Cassiopea exposed to copper or zinc demonstrated measurable effects at environmentally relevant concentrations (Templeman and Kingsford, 2015). In other studies, agricultural formulations of diuron and hexazinone at ecologically relevant concentrations caused measurable inhibition with respect to Cassiopea growth, photosynthetic efficiency, and symbiont density within 7 days of exposure (Rowen et al., 2017). A study looking at interactive responses between herbicides and salinity demonstrated that reduced salinity increased herbicide toxicity (Klein et al., 2016a). Pollutants in sewage and agro-chemicals have also been shown to cause reproductive and recruitment failure across most invertebrate phyla (Depledge and Billinghurst, 1999). There is an urgent need for new and improved bioassays to detect and assess new chemicals for their potential effects on marine invertebrates. Cassiopea has the potential to contribute extensively to understanding the effects of contaminants and pollutants on coastal marine ecosystems; the various genomic resources available for Cassiopea provide a tractable means to identify potential in situ molecular biomarkers and cellular diagnostic endpoints.
Other Laboratory Applications
Toxinology and Cnidome
Despite the ubiquity of cnidarian species in a variety of aquatic environments, toxinological research concerning cnidarian venom has been significantly delayed compared to advances seen in research applications related to venomous terrestrial animals (Turk and Kem, 2009). Early clinical research on cnidarian venom validated a diversity of symptoms presented in human sting victims, including but not limited to anaphylaxis (Togias et al., 1985; Radwan and Burnett, 2001). Studies have also emphasized the potential for cnidarian bioactive proteins in pharmacological applications (Jha and Zi-Rong, 2004). Cassiopea medusae have a mild capacity to sting, and only a few cases of human envenomation have been reported to cause symptoms ranging from a rash, swelling, vomiting, and urticaria (Rifkin and Fenner, 1996). Cnidarian venom is delivered to prey and potential predators via the firing of microscopic nematocysts. In C. andromeda, five different types of nematocysts have been identified: a-isorhizas, O-isorhizas, euryteles, large oval birhopaloids, and small lemon-shaped birhopaloid (Heins et al., 2015). Studies including toxinological inferences have focused on several bioactive properties of crude venom extracts, which include cytolysis and hemolysis (Radwan and Burnett, 2001; Radwan et al., 2001, 2005; Torres et al., 2001). Neurotoxic effects were also identified in two of Cassiopea's nematocyst types (birhopaloid and a-isorhiza) (Gülşahin, 2015). The ease at which Cassiopea can be collected globally in mangroves and cultured in the lab, provides a clear advantage to studying this species' venom, which has antitumor and antiprotozoal properties that may serve as future novel pharmacological and therapeutics agents (Orduña-Novoa et al., 2003; Morales-Landa et al., 2007; Mirshamsi et al., 2017). A recent study showed that Cassiopea crude venom induced apoptosis in human breast cancer tissue via reactive oxygen species (ROS) mediated cytotoxicity (Mirshamsi et al., 2017). Cassiopea, therefore, has potential for future bioactive natural product discovery through modern “venomics” approaches (genome, transcriptome, proteome, metabolome) that have applications to elucidating evolutionary mechanisms driving the complexity and diversity of cnidarian venom (Radwan et al., 2001; Casewell et al., 2013; Jouiaei et al., 2015).
Cassiopea Virology
In comparison to bacterial components of the cnidarian holobiont, studies on the virome have received considerably less attention, but are of interest due to the potentially harmful effects that viruses can have on cnidarian health (Thurber and Correa, 2011; Correa et al., 2016). There is increasing recognition for biological and ecological importance of viruses in marine samples (Bosch et al., 2015; Thurber et al., 2017), and their role in evolution (Grasis et al., 2014). Preliminary data from the anthozoan Exaiptasia, has identified several RNA viruses, including those from Partitiviridae and Picornaviridae (Brüwer and Voolstra, 2017). The potential medical relevance, complex life strategies, as well as their host range and evolutionary history, the possible presence of RNA viruses associated with the jellyfish is of great interest to the field (Johnson, 2015; Koonin et al., 2015), and future studies should shed light on the yet-to-be explored evolutionary path of virus-cnidarian co-evolution. Furthermore, some cnidarians express genes that have been implicated as potential anti-retroviral agents (Ramessar et al., 2014), and our preliminary findings of several viruses in Cassiopea validate the necessity of these putative defense mechanisms. Going forward, studies on Cassiopea viromes may provide a unique opportunity to not only expand our understanding of the current host range of Mononega- and retro-like viruses, but also to establish a reliable and robust model system for rigorous investigation of interactions within the holobiont as they relate to possible host immunity, in all stages of the jellyfish's life history.
Cassiopea as a Laboratory Resource
The ease at which the asexual polyp stage can be propagated in the laboratory using artificial seawater makes Cassiopea an attractive system for sustained culturing. Depending on the frequency of feeding, polyps are capable of developing motile planuloid buds within 24 h (Van Leishout and Martin, 1992). Swimming buds can settle onto a suitable substrate within 24 h and undergo metamorphosis into a polyp. Therefore, the continuous release of planuloid buds permits an aposymbiotic polyp to propagate a perpetual colony of asexual polyps. Currently, twelve C. xamachana lines (originally from Florida) have been established by the Cassiopea consortium. A draft C. xamachana genome has been assembled (ENA Accession: PRJEB23739), as well as transcriptomes of the aposymbiotic, symbiotic, and strobila stages (European Nucleotide Archive Accession: PRJEB21012). Future application of metabolomics approaches in Cassiopea research will also help generate a profile of the underlying metabolic pathways and metabolite dynamics that might be affected by environmental factors during key stages of Cassiopea development and symbiosis.
While aposymbiotic polyps can be propagated as described above, symbiotic polyps can be generated by providing the polyps with Symbiodinium spp. Cassiopea polyps are capable of forming associations with virtually all symbiotic Symbiodinium cultures (Thornhill et al., 2006; Lampert, 2016), making this an ideal system for comparative studies. The colonization characteristics of Cassiopea provide two tractable phenotypes to visually confirm variation in the symbiotic association. Symbiodinium density within the amoebocytes can vary depending on the colonizing species (Winstead et al., 2018), and strobilation timing also appears to vary accordingly (Lampert, 2016). Newly strobilated ephyrae are available for experimentation; likewise the adult medusae can also be acquired with relative ease, through animal transfer with public aquariums and through the recreational aquarium trade. In addition, only simple setups are required to rear each stage, making Cassiopea an easily manageable laboratory animal (Widmer, 2008). Fertilized eggs can be obtained from the brooding female adult medusae, confirmed by the initiation of initial cleavage post-collection (Smith, 1936; Gohar and Eisawy, 1960). Cassiopea are gonochoristic, with females identifiable through the presence of specialized central brood tentacles (Hofmann and Hadfield, 2002). Spawning can likely occur year-around under ideal conditions, and fertilized eggs collected directly from the brood tentacles after spawning (Gohar and Eisawy, 1960; Hummelinck, 1968). The planula larvae stage can also be acquired directly from the brooding female and used for additional experimentation. Tools for working with Cassiopea are increasingly being made available to researchers and educators, with many such protocols available online. In particular, a customized whole mount in situ hybridization protocol has been developed for Cassiopea, along with other molecular tools and resources, which are accessible through the online portal (http://sites.psu.edu/cassbase/). Single cell injection for transgenic approaches is currently under development, with a promising outlook for becoming an important resource for mapping jellyfish molecular pathways.
Conclusions
Cassiopea is a system predominantly studied for the many questions that can be addressed on interdependent organisms given its remarkable Cnidaria-Symbiodinium association. This review demonstrates that in addition to the effectiveness of the Cassiopea system for elucidating the complex processes underlying symbiosis, Cassiopea has potential to contribute substantially to our knowledge of a wide range of research topics within the field of invertebrate biology. The recent acquisition of genomics resources and development of several new molecular tools for Cassiopea sets this cnidarian apart as a model for identifying possible genotypic and phenotypic traits to support the hypothesis of co-evolution between Cassiopea and Symbiodinium. Given Cassiopea's position as a well-established lab organism, the time is right for studies that seek to characterize possible changes in molecular composition (at the gene, metabolite or peptide-level) of one or both symbiotic organisms, phenotypic attributes that may have evolved as a result of this interspecific interaction, or the putative effects of ecological intimacy on each organism's level of adaptation to the shared environment. These questions, and countless others, can be properly interrogated by way of rigorous observations, diverse experimentation, and implementation of phylogenetic analyses in an attempt to infer potential reciprocal evolutionary change.
The Cassiopea system also has obvious applications to the fields of bacterial and viral co-evolution, from the dual perspective of understanding microbial symbiosis between either the cnidarian host or the associated dinoflagellate symbiont. Furthermore, Cassiopea shows promise as an effective bioindicator for our rapidly changing oceans, and as a biomonitor for further investigations of the medusa's potential impact on coastal ecology on a broader geographic scale. The relatively straight-forward culturing protocols and approachability of the system make Cassiopea particularly feasible as a teaching tool, not only at the college level but also from Kindergarten through grade 12. This review is a culmination of many years of multidisciplinary, collaborative research that has helped position the upside-down jellyfish Cassiopea as a valuable resource to researchers in the omics era seeking an effective model system to better understand the natural history and biodiversity of some of the earliest multicellular animals through studies on evolution, co-evolution and beyond.
Author Contributions
AO, MA, DB, LS-B, CF, EG-M, AJ-B, PL, ACMo, LM, LDM, ACMa, CN, SP, JS, SS, BS, MT, PT, MV, CW, JW, and MQM: Provided written text for the sections within the manuscript; CA, AC, DH, TG, WF, and MM: provided expertise and edits to the manuscript.
Dedication
We dedicate this manuscript to Robert Trench, who inspired many of us to begin working in Cassiopea.
Conflict of Interest Statement
The authors declare that the research was conducted in the absence of any commercial or financial relationships that could be construed as a potential conflict of interest.
Acknowledgments
We'd like to thank Todd LaJeunesse, Yixian Zheng, and Chen-Ming Fan for providing comments on the manuscript. Some of the images used in Figure 1 were provided by Todd LaJeunesse and Priyanka Kanti Solanki. Many thanks to Jon Norenburg who, as the chair of Invertebrate Zoology at the Smithsonian National Museum of Natural History, supported our endeavors to create the Aquaroom, thereby facilitating many interesting observations of Cassiopea polyps and medusae (some acquired from the National Aquarium) by some coauthors and Aquaroom interns. Financial support was provided from Fundação de Amparo à Pesquisa do Estado de São Paulo (FAPESP) projects 2011/50242-5, 2015/21007-9, 2015/24408-4, 2017/50028-0 and Conselho Nacional de Desenvolvimento Científico e Tecnológico (CNPq) project 304961/2016-7, 404121/2016-0.
References
Agassiz, L. (1862). “Acalephs in general, Ctenophorae, Discophorae, Hydroidae, homologies of the Radiata,” in Contributions to the Natural History of the United States of America, Vol. 4 (Boston, MA; London: Little, Brown & Co; Trübner & Co.).
Agassiz, A., and Mayer, A. G. (1899). Acalephs from the Fiji Islands. Bull. Museum Comp. Zoöl. 32, 157–189.
Anthony, K. R. N., Hoogenboom, M. O., Maynard, J. A., Grottoli, A. G., and Middlebrook, R. (2009). Energetics approach to predicting mortality risk from environmental stress: a case study of coral bleaching. Funct. Ecol. 23, 539–550. doi: 10.1111/j.1365-2435.2008.01531.x
Arai, M. N. (2001). Pelagic coelenterates and eutrophication: a review. Hydrobiologia 451, 69–87. doi: 10.1023/A:1011840123140
Arai, Y., Gotoh, R. O., Yokoyama, J., Sato, C., Okuizumi, K., and Hanzawa, N. (2017). Phylogenetic relationships and morphological variations of upside-down jellyfishes, Cassiopea spp. inhabiting Palau Island. Biogeography 19, 133–141
Bayha, K. M., and Graham, W. M. (2014). “Nonindigenous marine jellyfish: invasiveness, invisibility, and impacts,” in Jellyfish Blooms, eds K. A. Pitt and C. H. Lucas (Dordrecht: Springer), 45–78.
Bayha, K. M., Dawson, M. N., Collins, A. G., Barbeitos, M. S., and Haddock, S. H. (2010). Evolutionary relationships among scyphozoan jellyfish families based on complete taxon sampling and phylogenetic analyses of 18S and 28S ribosomal DNA. Integr. Comp. Biol. 50, 436–455. doi: 10.1093/icb/icq074
Bigelow, R. P. (1893). Some Observations on Polyclonia frondosa. Baltimore, MD: Johns Hopkins University Circulars. 106.
Bigelow, R. P. (1900). “The anatomy and development of Cassiopea xamachana,” in Memoirs of the Boston Society of Natural History, Vol. 5 (Baltimore, MD: Johns Hopkins Press), 751–785.
Bosch, T. C. G. (2008). “The path less explored: innate immune reactions in Cnidarians,” in Innate Immunity of Plants, Animals, and Humans, ed H. Heine (Berlin; Heidelberg: Springer Berlin Heidelberg), 27–42.
Bosch, T. C., Grasis, J. A., and Lachnit, T. (2015). Microbial ecology in Hydra: why viruses matter. J. Microbiol. 53, 193–200. doi: 10.1007/s12275-015-4695-2
Brandt, J. F. (1835). Prodomus descriptionis animalium ab H. Mertensio observatorum. Fasc. I. Polypos, Acalephas Discophoras et Siphonophoras, nec non Echinodermata continens. Recueil des Actes de la Séance Publique de l'Académie Impériale des Sciences de St. Pétersbourg 1834, 201–275.
Brekhman, V., Malik, A., Haas, B., Sher, N., and Lotan, T. (2015). Transcriptome profiling of the dynamic life cycle of the scypohozoan jellyfish Aurelia aurita. BMC Genomics 16:74. doi: 10.1186/s12864-015-1320-z
Brotz, L., Cheung, W. W. L., Kleisner, K., Pakhomov, E., and Pauly, D. (2012). Increasing jellyfish populations: trends in large marine ecosystems. Hydrobiologia 690, 3–20. doi: 10.1007/s10750-012-1039-7
Brüwer, J. D., and Voolstra, C. R. (2017). Insights into viral community composition of the cnidarian model metaorganism Aiptasia using RNA-Seq data. PeerJ Preprints 5:e3343v1. doi: 10.7287/peerj.preprints.3343v1
Burriesci, M. S., Raab, T. K., and Pringle, J. R. (2012). Evidence that glucose is the major transferred metabolite in dinoflagellate-cnidarian symbiosis. J. Exp. Biol. 215, 3467–3477. doi: 10.1242/jeb.070946
Cabrales-Arellano, P., Islas-Flores, T., Thomé, P. E., and Villanueva, M. A. (2017). Indomethacin reproducibly induces metamorphosis in Cassiopea xamachana scyphistomae. PeerJ 5:e2979. doi: 10.7717/peerj.2979
Cary, L. R. (1916). The influence of the marginal sense organs on the rate of regeneration in Cassiopea xamachana. J. Exp. Zool. 21, 1–32. doi: 10.1002/jez.1400210102
Casewell, N. R., Wuster, W., Vonk, F. J., Harrison, R. A., and Fry, B. G. (2013). Complex cocktails: the evolutionary novelty of venoms. Trends Ecol. Evol. 28, 219–229. doi: 10.1016/j.tree.2012.10.020
Çevik, C. (2006). A new record of an alien jellyfish from the Levantine coast of Turkey - Cassiopea andromeda (Forsskål, 1775) (Cnidaria: Scyphozoa:Rhizostomea). Aquat. Invas. 1, 196–197. doi: 10.3391/ai.2006.1.3.18
Chapman, P. M. (1995). Ecotoxicology and pollution - Key issues. Mar. Pollut. Bull. 31, 167–177. doi: 10.1016/0025-326X(95)00101-R
Chiori, R., Jager, M., Denker, E., Wincker, P., Da Silva, C., Le Guyader, H., et al. (2009). Are Hox genes ancestrally involved in axial patterning? Evidence from the hydrozoan Clytia hemisphaerica (Cnidaria). PLoS ONE 4:e4231. doi: 10.1371/journal.pone.0004231
Colley, N. J., and Trench, R. K. (1983). Selectivity in phagocytosis and persistence of symbiotic algae by the scyphistoma stage of the jellyfish Cassiopeia xamachana. Proc. R. Soc. B 219, 61–82. doi: 10.1098/rspb.1983.0059
Colley, N. J., and Trench, R. K. (1985). Cellular events in the reestablishment of a symbiosis between a marine dinoflagellate and a coelenterate. Cell Tissue Res. 239, 93–103. doi: 10.1007/BF00214908
Correa, A. M., Ainsworth, T. D., Rosales, S. M., Thurber, A. R., Butler, C. R., and Vega Thurber, R. L. (2016). Viral outbreak in corals associated with an in situ bleaching event: atypical herpes-like viruses and a new megavirus infecting symbiodinium. Front. Microbiol. 7:127. doi: 10.3389/fmicb.2016.00127
Curtis, S., and Cowden, R. R. (1971). Normal and experimentally modified development of buds in Cassiopea (Phylum Coelenterata; Class Schyphozoa). Acta Embryol. Exp. 3, 239–259.
Curtis, S., and Cowden, R. R. (1974). Some aspects of regeneration in the scyphistoma of Cassiopea (Class Scyphozoa) as revealed by the use of antimetabolites and microspectrophotometry. Am. Zool. 14, 851–866. doi: 10.1093/icb/14.2.851
Davy, S. K., Allemand, D., and Weis, V. M. (2012). Cell biology of cnidarian-dinoflagellate symbiosis. Microbiol. Mol. Biol. Rev. 76, 229–261. doi: 10.1128/MMBR.05014-11
Davy, S., and Cook, C. (2001). The relationship between nutritional status and carbon flux in the zooxanthellate sea anemone Aiptasia pallida. Mar. Biol. 139, 999–1005. doi: 10.1007/s002270100640
De'ath, G., Fabricius, K. E., Sweatman, H., and Puotinen, M. (2012). The 27-year decline of coral cover on the Great Barrier Reef and its causes. Proc. Natl. Acad. Sci. U.S.A. 109, 17995–17999. doi: 10.1073/pnas.1208909109
Depledge, M. H., and Billinghurst, Z. (1999). Ecological significance of endocrine disruption in marine invertebrates. Mar. Pollut. Bull. 39, 32–38. doi: 10.1016/S0025-326X(99)00115-0
Detournay, O., Schnitzler, C. E., Poole, A., and Weis, V. M. (2012). Regulation of cnidarian-dinoflagellate mutualisms: evidence that activation of a host TGFβ innate immune pathway promotes tolerance of the symbiont. Dev. Comp. Immunol. 38, 525–537. doi: 10.1016/j.dci.2012.08.008
Doty, M. S. (1961). Acanthophora, a possible invader of the marine flora of Hawaii. Pac. Sci. 15, 547–552.
Downs, C. A. (2005). Cellular Diagnostics and Its Application to Aquatic and Marine Toxicology. Boca Raton, FL: CRC Press.
Edwards, C. A., Subler, S., Chen, S. K., and Bogomolov, D. M. (1996). “Essential criteria for selecting bioindicator species, processes or systems to assess the environmental impacts of chemicals on soil ecosystems,” in Bioindicator Systems for Soil Pollution, 1st Edn, eds N. M. Van Straalen and D. A. Krivolutsky (Springer)
Epstein, H. E., Templeman, M. A., and Kingsford, M. J. (2016). Fine-scale detection of pollutants by a benthic marine jellyfish. Mar. Pollut. Bull. 107, 340–346. doi: 10.1016/j.marpolbul.2016.03.027
Faimali, M., Garaventa, F., Piazza, V., Costa, E., Greco, G., Mazzola, V., et al. (2014). Ephyra jellyfish as a new model for ecotoxicological bioassays. Mar. Environ. Res. 93, 93–101. doi: 10.1016/j.marenvres.2013.07.004
Ferrier, D. E. K., and Holland, P. W. H. (2001). Ancient origin of the Hox gene cluster. Nat. Rev. Genet. 2, 33–38. doi: 10.1038/35047605
Fitt, W. K., and Costley, K. (1998). The role of temperature in survival of the polyp stage of the tropical rhizostome jellyfish Cassiopea xamachana. J. Exp. Mar. Biol. Ecol. 222, 79–91. doi: 10.1016/S0022-0981(97)00139-1
Fitt, W. K., and Trench, R. K. (1983). Endocytosis of the symbiotic dinoflagellate Symbiodinium microadriaticum Freudenthal by endodermal cells of the scyphistomae of Cassiopea xamachana and resistance of the algal cells to host digestion J. Cell Sci. 64, 195–212.
Fitt, W. K., Hofmann, D. K., Wolk, M., and Rahat, M. (1987). Requirement of exogenous inducers for metamorphosis of axenic larvae and buds of Cassiopea andromeda (Cnidaria- Scyphozoa). Mar. Biol. 94, 415–422. doi: 10.1007/BF00428248
Fleck, J. (1998). Chemical fate of a metamorphic inducer in larvae-like buds of the Cnidarian Cassiopea andromeda. Biol. Bull. 194, 83–91. doi: 10.2307/1542516
Fleck, J., and Bischoff, A. (1992). Protein kinase C is possibly involved in chemical induction of metamrophosis in Cassiopea spp. (Cnidaria: Scyphozoa). Proc. Seventh Int. Coral Reef Symp. 1, 456–462.
Fleck, J., and Fitt, W. K. (1999). Degrading mangrove leaves of Rhizophora mangle Linne provide a natural cue for settlement and metamorphosis of the upside down jellyfish Cassiopea xamachana Bigelow. J. Exp. Mar. Biol. Ecol. 234, 83–94. doi: 10.1016/S0022-0981(98)00140-3
Fleck, J., Fitt, W. K., and Hahn, M. G. (1999). A proline-rich peptide originating from decomposing mangrove leaves is one natural metamorphic cue of the tropical jellyfish Cassiopea xamachana. Mar. Ecol. Prog. Ser. 183, 115–124. doi: 10.3354/meps183115
Forskål, P. (1775). Descriptiones Animalium Avium, Amphibiorum, Piscium, Insectorum, Vermium; Quae in Intinere Orientali Observavit Petrus Forskål. Copenhagen: Mölleri.
Forskål, P. (1776). Icones Rerum Naturalium, Quas in Intinere Orientali Depingi Curavit. Copenhagen: Hauniae.
Fowler, S. W., Teyssie, J. L., Cotret, O., Danis, B., Rouleau, C., and Warnau, A. (2004). Applied radiotracer techniques for studying pollutant bioaccumulation in selected marine organisms (jellyfish, crabs and sea stars). Nukleonika 49, 97–100.
Freeman, C. J., Stoner, E. W., Easson, C. G., Matterson, K. O., and Baker, D. M. (2016). Symbiont carbon and nitrogen assimilation in the Cassiopea–Symbiodinium mutualism. Mar. Ecol. Prog. Ser. 544, 281–286. doi: 10.3354/meps11605
Fuchs, B., Wang, W., Graspeuntner, S., Li, Y., Insua, S., Herbst, E. M., et al. (2014). Regulation of polyp-to-jellyfish transition in Aurelia aurita. Curr. Biol. 24, 263–273. doi: 10.1016/j.cub.2013.12.003
Galil, B. S., Spanier, E., and Ferguson, W. W. (1990). The scyphomedusae of the Mediterranean coast of Israel, including two lessepsian migrants new to the Mediterranean. Zool. Med. 64, 95–105.
Gattuso, J.-P., Yellowlees, D., and Lesser, M. (1993). Depth- and light-dependent variation of carbon partitioning and utilization in the zooxanthellate scleractinian coral Stylophora pistillata. Mar. Ecol. Prog. Ser. 92, 267–276. doi: 10.3354/meps092267
Gershwin, L.-A., Zeidler, W., and Davie, P. J. F. (2010). Medusae (Cnidaria) of Moreton Bay, Queensland, Australia. Mem. Queensl. Mus. 54, 47–108.
Gohar, H. A. F., and Eisawy, A. M. (1960). The development of Cassiopea andromeda. Publ. Mar. Biol. Stat. 11, 148–190.
Gómez Daglio, L., and Dawson, M. N. (2017). Species richness of jellyfishes (Scyphozoa : Discomedusae) in the Tropical Eastern Pacific: missed taxa, molecules, and morphology match in a biodiversity hotspot. Invertebr. Syst. 31:635. doi: 10.1071/IS16055
Grasis, J. A., Lachnit, T., Anton-Erxleben, F., Lim, Y. W., Schmieder, R., Fraune, S., et al. (2014). Species-specific viromes in the ancestral holobiont Hydra. PLoS ONE 9:e109952. doi: 10.1371/journal.pone.0109952
Gülşahin, N. (2015). Preliminary study on nematocyst types and venom isolation of Cassiopea andromeda Forskål, 1775 (Scyphozoa, Cnidaria) from Turkey. Cent. Nerv. Syst. Agents Med. Chem. 16, 208–212. doi: 10.2174/1871524915666150826092321
Hagedorn, M., Carter, V. L., Ly, S., Andrell, R. M., Yancey, P. H., Leong, J. A., et al. (2010). Analysis of internal osmolality in developing coral larvae, Fungia scutaria. Physiol. Biochem. Zool. 83, 157–166. doi: 10.1086/648484
Hamlet, C. L., and Miller, L. A. (2012). Feeding currents of the upside down jellyfish in the presence of background flow. Bull. Math. Biol. 74, 2547–2569. doi: 10.1007/s11538-012-9765-6
Hamlet, C., Santhanakrishnan, A., and Miller, L. A. (2011). A numerical study of the effects of bell pulsation dynamics and oral arms on the exchange currents generated by the upside-down jellyfish Cassiopea xamachana. J. Exp. Biol. 214, 1911–1921. doi: 10.1242/jeb.052506
Heins, A., Glatzel, T., and Holst, S. (2015). Revised descriptions of the nematocysts and the asexual reproduction modes of the scyphozoan jellyfish Cassiopea andromeda (Forskål, 1775). Zoomorphology 134, 351–366. doi: 10.1007/s00435-015-0263-x
Helm, R. R., and Dunn, C. W. (2017). Indoles induce metamorphosis in a broad diversity of jellyfish, but not in a crown jelly (Coronatae). PLoS ONE 12:e0188601. doi: 10.1371/journal.pone.0188601
Henning, G., Hofmann, D. K., and Benayahu, Y. (1996). The phorbol ester TPA induces metamorphosis in Red Sea coral planulae (Cnidaria- Anthozoa). Experientia 52, 744–749. doi: 10.1007/BF01925586
Hofmann, D. K., and Brand, U. (1987). Induction of metamorphosis in the symbiotic scyphozoan Cassiopea andromeda- role of marine bacteria and of biochemicals. Symbiosis 4, 99–116.
Hofmann, D. K., and Gottlieb, M. (1991). Bud formation in the scyphozoan Cassiopea andromeda-epithelial dynamics and fate map. Hydrobiologia 216, 53–59. doi: 10.1007/BF00026443
Hofmann, D. K., and Hadfield, M. G. (2002). Hermaphroditism, gonochorism, and asexual reproduction in Cassiopea sp.—an immigrant in the islands of Hawai'i. Invertebr. Reprod. Dev. 41, 215–221.
Hofmann, D. K., and Honegger, T. G. (1990). Bud formation and metamorphosis in Cassiopea andromeda (Cnidaria-Scyphozoa)- a developmental and ultrastructural study. Mar. Biol. 105, 509–518. doi: 10.1007/BF01316322
Hofmann, D. K., and Kremer, B. P. (1981). Carbon metabolism and strobilation in Cassiopea andromeda (Cnidaria: Scyphozoa): significanec of endosymbiotic dinoflagellates. Mar. Biol. 65, 25–33. doi: 10.1007/BF00397064
Hofmann, D. K., Fitt, W. K., and Fleck, J. (1996). Checkpoints in the life-cycle of Cassiopea spp.: control of metagenesis and metamorphosis in a tropical jellyfish. Int. J. Dev. Biol. 40, 331–338.
Hofmann, D. K., Neumann, R., and Henne, K. (1978). Strobilation, budding and initiation of scyphistoma morphogenesis in the rhizostome Cassiopea andromeda (Cnidaria- Scyphozoa). Mar. Biol. 47, 161–176. doi: 10.1007/BF00395637
Holland, B. S., Dawson, M. N., Crow, G. L., and Hofmann, D. K. (2004). Global phylogeography of Cassiopea (Scyphozoa: Rhizostomeae): molecular evidence for cryptic species and multiple invasions of the Hawaiian Islands. Mar. Biol. 145, 1119–1128. doi: 10.1007/s00227-004-1409-4
Holst, S. (2012). Effects of climate warming on strobilation and ephyra production of North Sea scyphozoan jellyfish. Hydrobiologia 690, 127–140. doi: 10.1007/s10750-012-1043-y
Hummelinck, W. P. (1968). Caribbean Scyphomedusae of the Genus Cassiopea, Vol. 25. Hague: Studies on teh Fauna of Curaçao and other Caribbean Islands.
Imbs, A. B. (2013). Fatty acids and other lipids of corals: composition, distribution, and biosynthesis. Russ. J. Mar. Biol. 39, 153–168. doi: 10.1134/S1063074013030061
Imbs, A. B., Yakovleva, I. M., Dautova, T. N., Bui, L. H., and Jones, P. (2014). Diversity of fatty acid composition of symbiotic dinoflagellates in corals: evidence for the transfer of host PUFAs to the symbionts. Phytochemistry 101, 76–82. doi: 10.1016/j.phytochem.2014.02.012
Jantzen, C., Wild, C., Rasheed, M., El-Zibdah, M., and Richter, C. (2010). Enhanced pore-water nutrient fluxes by the upside-down jellyfish Cassiopea sp. in a Red Sea coral reef. Mar. Ecol. Prog. Ser. 411, 117–125. doi: 10.3354/meps08623
Jha, R. K., and Zi-Rong, X. (2004). Biomedical compounds from marine organisms. Mar. Drugs 2, 123–146. doi: 10.3390/md203123
Johnson, W. E. (2015). Endogenous retroviruses in the genomics era. Annu. Rev. Virol. 2, 135–159. doi: 10.1146/annurev-virology-100114-054945
Jouiaei, M., Yanagihara, A. A., Madio, B., Nevalainen, T. J., Alewood, P. F., and Fry, B. G. (2015). Ancient venom systems: a review on cnidaria toxins. Toxins 7, 2251–2271. doi: 10.3390/toxins7062251
Kayal, E., Bentlage, B., Pankey, M. S., Ohdera, A. H., Medina, M., Plachetzki, D. C., et al. (2017). Comprehensive phylogenomic analyses resolve cnidarian relationships and the origins of key organismal traits. PeerJ. 5:e3172v1. doi: 10.7287/peerj.preprints.3172v1
Kayal, E., Roure, B., Philippe, H., Collins, A. G., and Lavrov, D. V. (2013). Cnidarian phylogenetic relationships as revealed by mitogenomics. BMC Evol. Biol. 13:1. doi: 10.1186/1471-2148-13-5
Keable, S. J., and Ahyong, S. T. (2016). First records of the invasive “upside-down jellyfish”, Cassiopea (Cnidaria: Scyphozoa: Rhizostomeae: Cassiopeidae), from coastal lakes of New South Wales, Australia. Rec. Aust. Mus. 68, 23–30. doi: 10.3853/j.2201-4349.68.2016.1656
Kehls, N. E., Herrmann, K., and Berking, S. (1999). The protein phosphatase inhibitor cantharidin induces head and foot formation in buds of Cassiopea andromeda. Int. J. Dev. Biol. 43, 51–58.
Klein, S. G., Pitt, K. A., and Carroll, A. R. (2016a). Reduced salinity increases susceptibility of zooxanthellate jellyfish to herbicide toxicity during a simulated rainfall event. Environ. Poll. 209, 79–86. doi: 10.1016/j.envpol.2015.11.012
Klein, S. G., Pitt, K. A., and Carroll, A. R. (2016b). Surviving but not thriving: inconsistent responses of zooxanthellate jellyfish polyps to ocean warming and future UV-B scenarios. Sci. Rep. 6:28859. doi: 10.1038/srep28859
Klein, S. G., Pitt, K. A., Nitschke, M. R., Goyen, S., Welsh, D. T., Suggett, D. J., et al. (2017). Symbiodinium mitigate the combined effects of hypoxia and acidification on a noncalcifying cnidarian. Glob. Change Biol. 23, 3690–3703. doi: 10.1111/gcb.13718
Koonin, E. V., Dolja, V. V., and Krupovic, M. (2015). Origins and evolution of viruses of eukaryotes: the ultimate modularity. Virology 479–480, 2–25. doi: 10.1016/j.virol.2015.02.039
Kopp, C., Pernice, M., Domart-Coulon, I., Djediat, C., Spangenberg, J. E., Alexander, D. T., et al. (2013). Highly dynamic cellular-level response of symbiotic coral to a sudden increase in environmental nitrogen. MBio 4, e00052–e00013. doi: 10.1128/mBio.00052-13
Kopp, C., Wisztorski, M., Revel, J., Mehiri, M., Dani, V., Capron, L., et al. (2015). MALDI-MS and NanoSIMS imaging techniques to study cnidarian-dinoflagellate symbioses. Zoology 118, 125–131. doi: 10.1016/j.zool.2014.06.006
Kramp, P. L. (1961). Synopsis of the medusae of the world. J. Mar. Biol. Assoc. UK 40, 7–382. doi: 10.1017/S0025315400007347
Kremer, P. (2005). Ingestion and elemental budgets for Linuche unguiculata, a scyphomedusa with zooxanthellae. J. Mar. Biol. Assoc. U.K. 85, 613–625. doi: 10.1017/S0025315405011549
Kuhn, K., Streit, B., and Schierwater, B. (1999). Isolation of hox genes from the Scyphozoan Cassiopeia xamachana- implications for the early evolution of Hox Genes. J. Exp. Zool. 285, 63–75. doi: 10.1002/(SICI)1097-010X(19990415)285:1<63::AID-JEZ8>3.0.CO;2-X
Kuniyoshi, H., Okumura, I., Kuroda, R., Tsujita, N., Arakawa, K., Shoji, J., et al. (2012). Indomethacin induction of metamorphosis from the asexual stage to sexual stage in the moon jellyfish, Aurelia aurita. Biosci. Biotechnol. Biochem. 76, 1397–1400. doi: 10.1271/bbb.120076
Kvennefors, E. C., Leggat, W., Hoegh-Guldberg, O., Degnan, B. M., and Barnes, A. C. (2008). An ancient and variable mannose-binding lectin from the coral Acropora millepora binds both pathogens and symbionts. Dev. Comp. Immunol. 32, 1582–1592. doi: 10.1016/j.dci.2008.05.010
Lampert, K. P. (2016). “Cassiopea and its zooxanthellae,” in The Cnidaria, Past, Present and Future: The World of Medusa and Her Sisters, eds S. Goffredo and Z. Dubinsky (Springer International Publishing), 415–423.
Leitz, T. (1997). Induction of settlement and metamorphosis of Cnidarian larvae: signals and signal transduction. Invertebr. Reprod. Dev. 31, 109–122. doi: 10.1080/07924259.1997.9672569
Light, S. F. (1914). Some philippine scyphomedusae, including two new genera, five new species, and one new variety. Philipp. J. Sci. 9, 195–231.
Loeb, M. J. (1973). The effect of light on strobilation in the Chesapeake Bay Sea Nettle Chrysaora quinquecirrha. Mar. Biol. 20, 144–147. doi: 10.1007/BF00351452
Ludwig, F. D. (1969). Die zooxanthellen bei Cassiopea andromeda Eschscholtz 1829 und ihre Bedeutung für die Strobilation. Zoologische Jahrbücher. Abteilung Anat. Ontogenie Tiere 86:33.
Maas, O. (1902). Ueber Medusen aus dem Solenhofer Schiefer und der unteren Kreide der Karpaten. Palaeontographica 48, 297–322.
Markert, B. A., Breure, A. M., and Zechmeister, H. G. (eds.). (2003). “Definitions, strategies and principles for bioindication/biomonitoring of the environment,” in Trace Metals and other Contaminants in the Environment (Amsterdam: Elsevier), 3–39.
Martin, V. J., and Chia, F.-S. (1982). Fine structure of a scyphozoan planula, Cassiopeia xamachana. Biol. Bull. 163, 320–328. doi: 10.2307/1541269
McCarty, L. S. (2002). Issues at the interface between ecology and toxicology. Toxicology 181–182, 497–503. doi: 10.1016/S0300-483X(02)00465-1
McCarty, L. S., and Munkittrick, K. R. (2008). Environmental biomarkers in aquatic toxicology: Fiction, fantasy, or functional? Hum. Ecol. Risk Assess. Int. J. 2, 268–274. doi: 10.1080/10807039609383607
McGill, C. J., and Pomory, C. M. (2008). Effects of bleaching and nutrient supplementation on wet weight in the jellyfish Cassiopea xamachana(Bigelow) (Cnidaria: Scyphozoa). Mar. Freshw. Behav. Physiol. 41, 179–189. doi: 10.1080/10236240802369899
Miller, D. J., Hemmrich, G., Ball, E. E., Hayward, D. C., Khalturin, K., Funayama, N., et al. (2007). The innate immune repertoire in cnidaria - ancestral complexity and stochastic gene loss. Genome Biol. 8:R59. doi: 10.1186/gb-2007-8-4-r59
Mirshamsi, M. R., Omranipour, R., Vazirizadeh, A., Fakhri, A., Zangeneh, F., Mohebbi, G. H., et al. (2017). Persian Gulf Jellyfish (Cassiopea andromeda) venom fractions induce selective injury and cytochrome c release in mitochondria obtained from breast adenocarcinoma patients. Asian Pac. J. Cancer Prev. 18, 277–286. doi: 10.22034/APJCP.2017.18.1.277
Molina, V. H., Castillo-Medina, R. E., and Thomé, P. E. (2017). Experimentally induced bleaching in the Sea Anemone Exaiptasia supports glucose as a main metabolite associated with its symbiosis. J. Mar. Biol. 2017, 1–7. doi: 10.1155/2017/3130723
Morales-Landa, J. L., Zapata-Pérez, O., Cedillo-Rivera, R., Segura-Puertas, L., Simá-Alvarez, R., and Sánchez-Rodríguez, J. (2007). Antimicrobial, antiprotozoal, and toxic activities of Cnidarian extracts from the Mexican Caribbean Sea. Pharm. Biol. 45, 37–43. doi: 10.1080/13880200601026325
Morandini, A. C., Stampar, S. N., Maronna, M. M., and Da Silveira, F. L. (2017). All non-indigenous species were introduced recently? The case study of Cassiopea (Cnidaria: Scyphozoa) in Brazilian waters. J. Mar. Biol. Assoc. U.K. 97, 321–328. doi: 10.1017/S0025315416000400
Mortillaro, J. M., Pitt, K. A., Lee, S. Y., and Meziane, T. (2009). Light intensity influences the production and translocation of fatty acids by zooxanthellae in the jellyfish Cassiopea sp. J. Exp. Mar. Biol. Ecol. 378, 22–30. doi: 10.1016/j.jembe.2009.07.003
Müller, W. A. (1973). Induction of metamorphosis by bacteria and ions in the planulae of Hydractinia echinata- an approach to the mode of action. Publ. Seto Mar. Biol. Lab. 20, 195–208. doi: 10.5134/175777
Nath, R. D., Bedbrook, C. N., Abrams, M. J., Basinger, T., Bois, J. S., Prober, D. A., et al. (2017). The Jellyfish Cassiopea exhibits a sleep-like state. Curr. Biol. 27, 2984.e2983–2990.e2983. doi: 10.1016/j.cub.2017.08.014
Neubauer, E. F., Poole, A. Z., Neubauer, P., Detournay, O., Tan, K., Davy, S. K., et al. (2017). A diverse host thrombospondin-type-1 repeat protein repertoire promotes symbiont colonization during establishment of cnidarian-dinoflagellate symbiosis. Elife 6:e24494. doi: 10.7554/eLife.24494
Neubauer, E. F., Poole, A. Z., Weis, V. M., and Davy, S. K. (2016). The scavenger receptor repertoire in six cnidarian species and its putative role in cnidarian-dinoflagellate symbiosis. PeerJ 4:e2692. doi: 10.7717/peerj.2692
Neumann, R. (1979). Bacterial induction of settlement and metamorphosis in the planula larvae of Cassiopea andromeda (Cnidaria- Scyphozoa, Rhizostomeae). Mar. Ecol. Prog. Ser. 1, 21–28. doi: 10.3354/meps001021
Neumann, R. (1980). Bakterielle Metamorphoseauslösung und Kontrolle der Morphogenese bei Schwimmknospen und Planulalarven von Cassiopea andromeda. Ph.D., Cologne University.
Nichols, A. L. A., Eichler, T., Latham, R., and Zimmer, M. (2017). A global brain state underlies C. elegans sleep behavior. Science 356, 1–9. doi: 10.1126/science.aam6851
Niggl, W., Naumann, M. S., Struck, U., Manasrah, R., and Wild, C. (2010). Organic matter release by the benthic upside-down jellyfish Cassiopea sp. fuels pelagic food webs in coral reefs. J. Exp. Mar. Biol. Ecol. 384, 99–106. doi: 10.1016/j.jembe.2010.01.011
Ojimi, M. C., and Hidaka, M. (2010). Comparison of telomere length among different life cycle stages of the jellyfish Cassiopea andromeda. Mar. Biol. 157, 2279–2287. doi: 10.1007/s00227-010-1495-4
Ojimi, M. C., Isomura, N., and Hidaka, M. (2009). Telomerase activity is not related to life history stage in the jellyfish Cassiopea sp. Comp. Biochem. Physiol. A Mol. Integr. Physiol. 152, 240–244. doi: 10.1016/j.cbpa.2008.10.008
Orduña-Novoa, K., Segura-Puertas, L., Sánchez-Rodriguez, J., Meléndez, A., Nava-Ruíz, C., Rembao, D., et al. (2003). Possible antitumoral effect of the crude venom of Cassiopea xamachana (Cnidaria- Scyphozoa) on tumors of the central nervous system induced by N-Ethyl-N-Nitrosourea (ENU) in rats. Proc. West. Pharmacol. Soc. 46, 85–87.
Ortman, B. D., Bucklin, A., Pagès, F., and Youngbluth, M. (2010). DNA barcoding the Medusozoa using mtCOI. Deep Sea Res. II Top. Stud. Oceanogr. 57, 2148–2156. doi: 10.1016/j.dsr2.2010.09.017
Ovchinnikova, T. V., Balandin, S. V., Aleshina, G. M., Tagaev, A. A., Leonova, Y. F., Krasnodembsky, E. D., et al. (2006). Aurelin, a novel antimicrobial peptide from jellyfish Aurelia aurita with structural features of defensins and channel-blocking toxins. Biochem. Biophys. Res. Commun. 348, 514–523. doi: 10.1016/j.bbrc.2006.07.078
Pallas, P. S. (1774). Spicilegia Zoologica. Quibus Novae Imprimis et Obscurae Animalium Species Iconibus, Descriptionibus Atque Commentariis Illustrantur. Berolini: Prostant apud Gottl.
Passano, L. M. (2004). Spasm behavior and the diffuse nerve-net in Cassiopea xamachana (Scyphozoa-Coelenterata). Hydrobiologia 530, 91–96. doi: 10.1007/s10750-004-3113-2
Péron, F., and Lesueur, C. A. (1810). Tableau des caractères génériques et spécifiques de toutes les espèces de Méduses connues jusqu'à ce jour. Ann. Mus. Natl. d'Histoire Nat. 14, 325–366.
Pierce, J. (2005). A system for mass culture of Upside-down jellyfish Cassiopea spp. as a potential food item for medusivores in captivity. Int. Zoo Yearbook 39, 62–69. doi: 10.1111/j.1748-1090.2005.tb00005.x
Polteva, D. G. (1985). Observations of asexual reproduction and regeneration in Cassiopea (Scyphozoa, Coelenterata). Zoologicheski 64, 172–180.
Radwan, F. F. Y., and Burnett, J. W. (2001). Toxinological studies of the venom from Cassiopea xamachana nematocysts isolated by flow cytometry. Comp. Biochem. Physiol. C 128, 65–73. doi: 10.1016/S1532-0456(00)00179-4
Radwan, F. F., Burnett, J. W., Bloom, D. A., Coliano, T., Eldefrawi, M. E., Erderly, H., et al. (2001). A comparison of the toxinological characteristics of two Cassiopea and Aurelia species. Toxicon 245–257. doi: 10.1016/S0041-0101(00)00121-5
Radwan, F. F., Roman, L. G., Baksi, K., and Burnett, J. W. (2005). Toxicity and mAChRs binding activity of Cassiopea xamachana venom from Puerto Rican coasts. Toxicon 45, 107–112. doi: 10.1016/j.toxicon.2004.10.002
Rahat, M., and Adar, O. (1980). Effect of symbiotic zooxanthellae and temperature on budding and strobilation in Cassiopeia andromeda (Eschscholz). Biol. Bull. 159, 394–401. doi: 10.2307/1541102
Ramessar, K., Xiong, C., Krumpe, L., Buckheit, R., Wilson, J., Mcmahon, J., et al. (2014). Isolation and characterization of a novel class of potent anti-HIV proteins from an Australian soft coral. FASEB J. 28:975. doi: 10.1096/fasebj.28.1_supplement.975.6
Richardson, A. J., Bakun, A., Hays, G. C., and Gibbons, M. J. (2009). The jellyfish joyride: causes, consequences and management responses to a more gelatinous future. Trends Ecol. Evol. 24, 312–322. doi: 10.1016/j.tree.2009.01.010
Rifkin, J., and Fenner, P. (1996). “Genus Cassiopea (upside-down jellyfish),” in Venomous and Poisonous Marine Animals: a Medical and Biological Handbook, eds J. W. Burnett, P. J. Fenner, and J. F. Rifkin (Sydney, NSW: UNSW Press), 180–235.
Rinkevich, B. (2004). Allorecognition and xenorecognition in reef corals- a decade of interactions. Hydrobiologia 530, 443–450. doi: 10.1007/s10750-004-2686-0
Rodrigues, L. J., and Grottoli, A. G. (2007). Energy reserves and metabolism as indicators of coral recovery from bleaching. Limnol. Oceanogr. 52, 1874–1882. doi: 10.4319/lo.2007.52.5.1874
Romeo, M., and Gnassia-Barelli, M. (1992). Importance of gelatinous plankton organisms in storage and transfer of trace metals in the northwestern Mediterranean. Mar. Ecol. Prog. Ser. 82, 267–274. doi: 10.3354/meps082267
Romeo, M., Gnassia-Barelli, M., and Carre, C. (1987). Trace metals: Cd, Cu, Pb AND Zn in Gelatinous Macroplanknton from the Northwestern Mediterranean. Water Res. 21, 1287–1292. doi: 10.1016/0043-1354(87)90182-5
Rowen, D. J., Templeman, M. A., and Kingsford, M. J. (2017). Herbicide effects on the growth and photosynthetic efficiency of Cassiopea maremetens. Chemosphere 182, 143–148. doi: 10.1016/j.chemosphere.2017.05.001
Ryan, J. F., Mazza, M. E., Pang, K., Matus, D. Q., Baxevanis, A. D., Martindale, M. Q., et al. (2007). Pre-bilaterian origins of the Hox cluster and the Hox code: evidence from the sea anemone, Nematostella vectensis. PLoS ONE 2:e153. doi: 10.1371/journal.pone.0000153
Santhanakrishnan, A., Dollinger, M., Hamlet, C. L., Colin, S. P., and Miller, L. A. (2012). Flow structure and transport characteristics of feeding and exchange currents generated by upside-down Cassiopea jellyfish. J. Exp. Biol. 215, 2369–2381. doi: 10.1242/jeb.053744
Schembri, P. J., Deidun, A., and Vella, P. J. (2010). First record of Cassiopea andromeda (Scyphozoa: Rhizostomeae: Cassiopeidae) from the central Mediterranean Sea. Mar. Biodivers. Rec. 3:e6. doi: 10.1017/S1755267209990625
Schiariti, A., Morandini, A. C., Jarms, G., Von Glehn Paes, R., Franke, S., and Mianzan, H. (2014). Asexual reproduction strategies and blooming potential in Scyphozoa. Mar. Ecol. Prog. Ser. 510, 241–253. doi: 10.3354/meps10798
Schummer, M., Scheurlen, I., Schaller, C., and Galliot, B. (1992). HOM/HOX homeobox genes are present in hydra (Chlorohydra viridissima) and are differentially expressed during regeneration. EMBO J. 11, 1815–1823.
Siefker, B., Kroiher, M., and Berking, S. (2000). Induction of metamorphosis from the larval to the polyp stage is similar in Hydrozoa and a subgroup of Scyphozoa (Cnidaria, Semaeostomeae). Helgol. Mar. Res. 54, 230–236. doi: 10.1007/s101520000053
Silverstone, M., Tosteson, T. R., and Cutress, C. E. (1977). The effect of lodide and various lodocompounds on initiation of strobilation in Aurelia. Gen. Comp. Endocrinol. 32, 108–113. doi: 10.1016/0016-6480(77)90087-9
Smith, H. G. (1936). Contribution to the anatomy and physiology of Cassiopea frondosa. Pap Tortugas Lab. Carnegie Inst. Washington 31, 17–52.
Spangenberg, D. B. (1965). A study of strobilation in Aurelia aurita under controlled conditions. J. Exp. Zool. 160, 1–9. doi: 10.1002/jez.1401600102
Stiasny, G. (1922). Ueber einige von Dr. C. J. van der Horst bei Curaçao gesammelte Medusen. Bijdragen tot de Kennis der Fauna van Curaçao 23, 83–92.
Stoner, E. W., Layman, C. A., Yeager, L. A., and Hassett, H. M. (2011). Effects of anthropogenic disturbance on the abundance and size of epibenthic jellyfish Cassiopea spp. Mar. Pollut. Bull. 62, 1109–1114. doi: 10.1016/j.marpolbul.2011.03.023
Templeman, M. A., and Kingsford, M. J. (2010). Trace element accumulation in Cassiopea sp. (Scyphozoa) from urban marine environments in Australia. Mar. Environ. Res. 69, 63–72. doi: 10.1016/j.marenvres.2009.08.001
Templeman, M. A., and Kingsford, M. J. (2012). Variation in soft tissue chemistry among scyphozoan and cubozoan jellyfishes from the Great Barrier Reef, Australia. Hydrobiologia 690, 279–290. doi: 10.1007/s10750-012-1051-y
Templeman, M. A., and Kingsford, M. J. (2015). Predicting aqueous copper and zinc accumulation in the upside-down jellyfish Cassiopea maremetens through the use of biokinetic models. Envir. Monit. Assess. 187:416. doi: 10.1007/s10661-015-4657-5
Thieme, C., and Hofmann, D. K. (2003a). Control of head morphogenesis in an invertebrate asexually produced larva-like bud (Cassiopea andromeda; Cnidaria: Scyphozoa). Dev. Genes Evol. 213, 127–133. doi: 10.1007/s00427-003-0300-5
Thieme, C., and Hofmann, D. K. (2003b). An endogenous peptide is involved in internal control of metamorphosis in the marine invertebrate Cassiopea xamachana (Cnidaria: Scyphozoa). Dev. Genes Evol. 213, 97–101. doi: 10.1007/s00427-003-0298-8
Thornhill, D. J., Daniel, M. W., Lajeunesse, T. C., Schmidt, G. W., and Fitt, W. K. (2006). Natural infections of aposymbiotic Cassiopea xamachana scyphistomae from environmental pools of Symbiodinium. J. Exp. Mar. Biol. Ecol. 338, 50–56. doi: 10.1016/j.jembe.2006.06.032
Thurber, R. L. V., and Correa, A. M. S. (2011). Viruses of reef-building scleractinian corals. J. Exp. Mar. Biol. Ecol. 408, 102–113. doi: 10.1016/j.jembe.2011.07.030
Thurber, R. V., Payet, J. P., Thurber, A. R., and Correa, A. M. (2017). Virus-host interactions and their roles in coral reef health and disease. Nat. Rev. Microbiol. 15, 205–216. doi: 10.1038/nrmicro.2016.176
Tilesius, W. G. (1831). Beiträge zur Naturgeschichte der Medusen. I. Cassiopeae. Nova Acta Phys. Med. Acad. Caesareae Leopoldino Carol. Nat. Curiosorum 15, 247–288.
Todd, B. D., Thornhill, D. J., and Fitt, W. K. (2006). Patterns of inorganic phosphate uptake in Cassiopea xamachana: a bioindicator species. Mar. Pollut. Bull. 52, 515–521. doi: 10.1016/j.marpolbul.2005.09.044
Togias, A. G., Burnett, J. W., Kagey-Sobotka, A., and Lichtenstein, L. M. (1985). Anaphylaxis after contact with a jellyfish. J. Allergy Clin. Immunol. 75, 672–675. doi: 10.1016/0091-6749(85)90092-2
Torres, M., Aguilar, M. B., Falcón, A., Sánchez, L., Radwan, F. F., Burnett, J. W., et al. (2001). Electrophysiological and hemolytic activity elicited by the venom of the jellyish Cassiopea xamachana. Toxicon 39, 1297–1307. doi: 10.1016/S0041-0101(01)00081-2
Tran, C., and Hadfield, M. G. (2011). Larvae of Pocillopora damicornis (Anthozoa) settle and metamorphose in response to surface-biofilm bacteria. Mar. Ecol. Prog. Ser. 433, 85–96. doi: 10.3354/meps09192
Trench, R. K. (1971). The physiology and biochemistry of zooxanthellae symbiotic with marine coelenterates. I. The assimilation of photosynthetic products of zooxanthellae by two marine coelenterates. Proc. R. Soc. B 177, 225–235. doi: 10.1098/rspb.1971.0024
Turk, T., and Kem, W. R. (2009). The phylum Cnidaria and investigations of its toxins and venoms until 1990. Toxicon 54, 1031–1037. doi: 10.1016/j.toxicon.2009.06.031
Uchida, T. (1970). Occurrence of a rhizostome medusa, Cassiopea mertensii Brandt from the Hawaiian Islands. Ann. Zool. Japon. 43, 102–104.
Van Gestel, C. A. M., and Van Brummelen, T. C. (1996). Incorporation of the biomarker concept in ecotoxicology calls for a redefinition of terms. Ecotoxicology 5, 217–225. doi: 10.1007/BF00118992
Van Leishout, J., and Martin, V. (1992). Development of Planuloid Buds of Cassiopea xamachana (Cnidaria- Scyphozoa). Trans. Am. Microsc. Soc. 111, 89–110. doi: 10.2307/3226666
Venn, A. A., Loram, J. E., and Douglas, A. E. (2008). Photosynthetic symbioses in animals. J. Exp. Bot. 59, 1069–1080. doi: 10.1093/jxb/erm328
Verde, E. A., and McCloskey, L. R. (1998). Production, respiration, and photophysiology of the mangrove jellyfish Cassiopea xamachana symbiotic with zooxanthellae- effect of jellyfish size and season Mar. Ecol. Prog. Ser. 168, 147–162.
Vermeij, M. J., Smith, J. E., Smith, C. M., Vega Thurber, R., and Sandin, S. A. (2009). Survival and settlement success of coral planulae: independent and synergistic effects of macroalgae and microbes. Oecologia 159, 325–336. doi: 10.1007/s00442-008-1223-7
Welsh, D. T., Dunn, R. J. K., and Meziane, T. (2009). Oxygen and nutrient dynamics of the upside down jellyfish (Cassiopea sp.) and its influence on benthic nutrient exchanges and primary production. Hydrobiologia 635, 351–362. doi: 10.1007/s10750-009-9928-0
Widmer, C. L. (2008). How to Keep Jellyfish in Aquariums: An Introductory Guide for Maintaining Healthy Jellies. Tuscon, AZ: Wheatmark.
Winstead, D., Ohdera, A. H., Medina, M., and Lajeunesse, T. C. (2018). Symbiodinium proliferation inside a cnidarian host vessel are competitive and dynamic. Symp. Oral Abstracts Integr. Comp. Biol. 58, e1–e264. doi: 10.1093/icb/icy001
Wolk, M., Rahat, M., Fitt, W. K., and Hofmann, D. K. (1985). Cholera toxin and thyrotropine can replace natural inducers required for the metamorphosis of larvae and buds of the scyphozoan Cassiopea andromeda. Roux's Arch. Dev. Biol. 194, 487–490. doi: 10.1007/BF00868150
Yamamori, L., Okuizumi, K., Sato, C., Ikeda, S., and Toyohara, H. (2017). Comparison of the inducing effect of indole compounds on medusa formation in different classes of medusozoa. Zool. Sci. 34, 173–178. doi: 10.2108/zs160161
Keywords: jellyfish, symbiosis, toxinology, sleep, venom, scyphozoan systematics, bioindicator
Citation: Ohdera AH, Abrams MJ, Ames CL, Baker DM, Suescún-Bolívar LP, Collins AG, Freeman CJ, Gamero-Mora E, Goulet TL, Hofmann DK, Jaimes-Becerra A, Long PF, Marques AC, Miller LA, Mydlarz LD, Morandini AC, Newkirk CR, Putri SP, Samson JE, Stampar SN, Steinworth B, Templeman M, Thomé PE, Vlok M, Woodley CM, Wong JC, Martindale MQ, Fitt WK and Medina M (2018) Upside-Down but Headed in the Right Direction: Review of the Highly Versatile Cassiopea xamachana System. Front. Ecol. Evol. 6:35. doi: 10.3389/fevo.2018.00035
Received: 29 January 2018; Accepted: 20 March 2018;
Published: 09 April 2018.
Edited by:
Sandie M. Degnan, The University of Queensland, AustraliaReviewed by:
Adam Michael Reitzel, University of North Carolina at Charlotte, United StatesLucas Leclere, UMR7009 Laboratoire de Biologie du Développement de Villefranche sur Mer, France
Copyright © 2018 Ohdera, Abrams, Ames, Baker, Suescún-Bolívar, Collins, Freeman, Gamero-Mora, Goulet, Hofmann, Jaimes-Becerra, Long, Marques, Miller, Mydlarz, Morandini, Newkirk, Putri, Samson, Stampar, Steinworth, Templeman, Thomé, Vlok, Woodley, Wong, Martindale, Fitt and Medina. This is an open-access article distributed under the terms of the Creative Commons Attribution License (CC BY). The use, distribution or reproduction in other forums is permitted, provided the original author(s) and the copyright owner are credited and that the original publication in this journal is cited, in accordance with accepted academic practice. No use, distribution or reproduction is permitted which does not comply with these terms.
*Correspondence: Aki H. Ohdera, YXVvMTQwQHBzdS5lZHU=
Mónica Medina, bXVtNTVAcHN1LmVkdQ==