- 1Institute of Integrative Biology, University of Liverpool, Liverpool, United Kingdom
- 2UMR 6553 ECOBIO, University of Rennes 1, Rennes, France
- 3Evolution, Behavior and Environment Group, University of Sussex, Brighton, United Kingdom
The microbiota is increasingly being recognized as having important impacts on many host biological processes. However, evidence of its effects on animal communication and breeding strategy is lacking. In this three-factorial study, we show that females were more willing to mate with related males, with relatedness likely being assessed through the microbiota. By contrast, male mating investment is concurrently determined by both the relatedness and microbiota status of the female. When the microbiota in female Drosophila melanogaster is altered by an antibiotic, male investment in sperm number increased when mating with unrelated females compared to related ones. Contrastingly, the presence of an intact microbiota in females canceled this male outbreeding strategy. As a consequence, the microbiota, when intact, decreased the fitness of the mating couple. Furthermore, we showed that female sexual signaling (cuticular hydrocarbons), with regards to kin recognition, significantly interacts with microbiota. Interestingly, the interaction is significant for hydrocarbons expressed by both sexes, but not for female-specific compounds. Taken together, our results suggest that microbiota can influence kin recognition by disfavoring male outbreeding strategies, likely by inhibiting key olfactory sexual signaling. This represents the first evidence of a host outbreeding strategy counteracted by their microbiota.
Introduction
The host microbiota is increasingly being shown to have important effects on host developmental, physiological, behavioral, and evolutionary processes. In particular, horizontally transmitted endosymbionts are well documented in their ability to manipulate their hosts to increase their own transmission (Hughes et al., 2012). In the 1970s it was suggested that commensal bacteria could alter the scent of the host, thereby potentially affecting animal communication, such as kin recognition and mate choice (Gorman, 1976). However, over the decades since this was proposed, the role of commensal bacteria in influencing host behaviors used to communicate/interact with conspecifics has largely been ignored (Lizé et al., 2013).
The studies that do address the link between microbiota and animal behavior have primarily been conducted on Drosophila melanogaster. For example, it has been shown that virgin D. melanogaster females prefer to mate with unfamiliar flies (Ödeen and Moray, 2008). This behavior may occur to avoid multiple matings with individuals of similar genotypes (see Eakley and Houde, 2004) and potentially microbiomes. In contrast, some studies have shown that both wild and laboratory-reared females prefer to mate with related males over unrelated ones (Loyau et al., 2012; Robinson et al., 2012), with inbred flies preferring to mate with individuals reared on the same diet (Sharon et al., 2010; Najarro et al., 2015). However, as this effect disappears when the diet is supplemented with antibiotics, it has been suggested that these preferences in mate choice are mediated by the commensal gut microbiota (Sharon et al., 2010; but see Leftwich et al., 2017).
The core composition of the D. melanogaster gut microbiota is cultivable, relatively simple—between 1-30 taxa—and exhibits considerably lower bacterial diversity than observed in vertebrates (Broderick and Lemaitre, 2012). Acetobacter, Gluconobacter, Enterococcus, and Lactobacillus are all associated with Drosophila (Brummel et al., 2004; Corby-Harris et al., 2007; Cox and Gilmore, 2007; Ren et al., 2007; Roh et al., 2008; Wong et al., 2011), but specific composition is known to change across diet type and laboratory. For example, flies reared on starch medium were dominated by L. plantarum, whereas flies reared on CMY (cornmeal, molasses, yeast) media exhibited a greater bacterial diversity including Bacillus firmus and Enterococcus faecalis (Sharon et al., 2010). Recent work has shown that the microbiota in D. melanogaster inhibits kin recognition. In fact, males with an intact microbiota, were unable to alter their mating investment (copulation duration) according to their mate relatedness level, while males were able to when their microbiota was altered (Lizé et al., 2014). Further, a higher mating propensity was observed in related pairs, yet increased mating investment (in terms of copulation duration) was observed in unrelated pairs. Thus, in this species, the microbiota may inhibit outbreeding through masking the host sexual signal of relatedness. This may be because the gut bacteria are driving their own preference and exhibiting kin recognition (e.g., Strassman et al., 2011; Wall, 2016), although the potential benefits to the bacteria are unknown. There is the potential for the modulation of female recognition impacting on microbiota fitness, as the microbiota is vertically transmitted by female D. melanogaster to their offspring via egg-smearing (Bakula, 1969). Therefore successful host reproduction will in turn increase microbiota fitness. However, to date the microbiota has never been implicated in inbreeding/outbreeding strategies of an organism.
In the current study, we examined the effect of relatedness, diet, and alteration of the microbiota, on female's mating propensity and male's mating investment, and resultant consequences on the fitness of the mating couple; we measured mating propensity as whether females mated or not, and male mating investment as male sperm transfer. We measured female mating propensity, male sperm transfer, and female resulting egg production in no choice mating tests, when mating occurred with a related (sister, brother) or an unrelated partner, that developed on the same or different food, and whose microbiota was intact or altered via streptomycin, in a fully factorial design (Figure 1). We hypothesized that any difference in behavior due to diet or microbiota manipulations might result from changes in the CHC (cuticular hydrocarbon) profile through olfactory cues that are used during mating in many insects, including D. melanogaster (Ferveur, 2005). Using two subsamples of virgin females reared under each treatment, we performed gas chromatography-mass spectrometry (GC/MS) to examine whether relatedness and diet, in the presence of an intact or altered microbiota, affected CHC profiles.
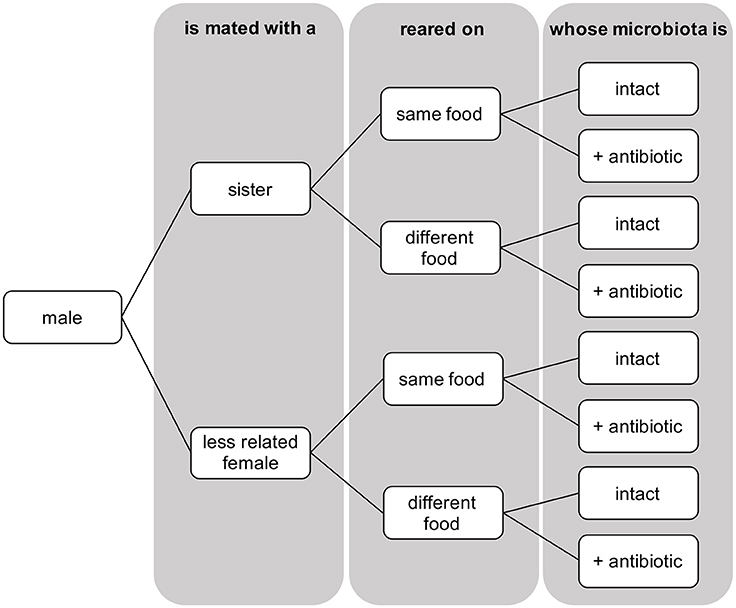
Figure 1. Schematic representation of the three-factorial design showing the eight treatments across which numbers of sperm transferred and eggs laid by females were measured in D. melanogaster.
Materials and Methods
Wild-type D. melanogaster stocks were isolated from a Wolbachia-free population collected from Dahomey (Benin) in 1970 and maintained in the laboratory since. Flies were reared at 25°C under a 12:12 h light:dark cycle. Recently mated females were placed into laying cages containing grape juice supplemented with a yeast-water paste and allowed to oviposit. Emerging first instar larvae were isolated and placed at a standard density of 10 per vial on one of two diets: 15 ml of either standard corn-based medium (for 1 l of water: 85 g of sugar, 60 g of corn, 20 g of deactivated yeast, 10 g of agar and 25 ml of nipagin), or banana-based medium (for 1 l of water: 137.5 g of banana, 47.5 g of sugar syrup, 30 g of malt, 27.5 g of deactivated yeast, 6.5 g of agar, and 2 g of mold inhibitor). To test whether microbiota altered sperm transfer and/or egg production, half of the vials of both banana and corn-based media were supplemented with the antibiotic streptomycin at a concentration of 0.04% (4 ml of 10 g streptomycin/100 ml ethanol solution per liter melted into the growth medium). Streptomycin is a generalist antibiotic naturally produced by Streptomyces sp bacteria living in the soil (Schatz et al., 1944). Streptomycin destroys mainly Gram-negative bacteria, and some Gram-positive bacteria, but is mostly ineffective against anaerobic bacteria, fungi and viruses (Schatz et al., 1944; Courvalin, 1994). It has already been used in several Drosophila studies in order to disturb their microbiota (Sharon et al., 2010; Lizé et al., 2014; Leftwich et al., 2017), and has been shown to have no deleterious effects on mortality, longevity and growth development when used at concentrations below 0.4% (Graf and Benz, 1970). Streptomycin is also known to have no effect on Wolbachia, an endosymbiont generally found in insects (Fenollar et al., 2003).
Mating Trials
At emergence, virgin flies were collected and isolated under CO2 anesthesia. Emergents were placed at standard densities of 10 females per vial and males kept individually, in vials containing their larval growth medium. At sexual maturity (3 days old), flies were aspirated into a mating vial containing 15ml of neutral food medium (comprising only sugar, yeast and agar—where corn or banana is absent). A single female was added first and allowed a short period of rest before one male was added. The tested individuals always had an intact microbiota, while its mating partner was either antibiotically treated or not (intact). Female propensity at mating was recorded for a total of 257 mating assays, and measured as the proportion of mating occurring within 3 h of observation, providing ample time for copulation to take place as 74.2% of mating occurred within 1 h. When mating occurred, mating pairs (Ntotal = 221) were assigned according to whether the sexual partner was either related (Nwithout streptomycin = 58; Nstreptomycin = 50) or not (Nwithout streptomycin = 58; Nstreptomycin = 55), reared on the same type of food (Nwithout streptomycin = 49; Nstreptomycin = 51) or a different type of food (Nwithout streptomycin = 67; Nstreptomycin = 54), making eight possible treatments in the three-factorial design (Figure 1).
Following copulation, mated females were then transferred to one of two scenarios. Females assigned for egg production were transferred shortly after mating to a vial containing 15 ml of neutral food medium (i.e., where banana or corn is absent) supplemented with two grains of yeast, before being placed at 25°C. Eggs laid by females (Nwithout streptomycin = 39; Nstreptomycin = 48) were counted every 24 h for a total of 72 h, with the female transferred to a new vial of neutral food medium every 24 h. Females assigned for analysis of sperm transfer (Nwithout streptomycin = 58; Nstreptomycin = 59) were immediately dissected according to standard protocol (Price et al., 2008), and a subsample of the transferred ejaculate was counted providing an estimate of the relative number of sperm transferred to each female. Sperm subsamples were measured as four lots of 5 μl of the original 50 μl sperm solution transferred to a siliconized glass slide. The number of sperm per slide was measured as the average of the four droplets of sperm solution multiplied by the dilution factor. The number of sperm transferred to a female during copulation is a standard measure of male investment (e.g., Friberg, 2006). Similarly, the number of eggs produced by a female is commonly used as a measure of female fitness (e.g., Dhole and Pfennig, 2014). Egg or sperm counts could not be assessed for seven females.
GC-MS Analysis of CHC Profiles
On emergence, pairs of sisters from four different parental origins (i.e., families) (NA = 11, NB = 14, NC = 13, ND = 17) reared on the two diets (Nsame diet = 38, Ndifferent = 17), and with (NGC−MS, streptomycin = 35) or without streptomycin (NGC−MS, without streptomycin = 20), as described above, were also frozen for subsequent GC-MS analysis. Two females from the same vial were placed in a 2 ml screw top clear glass vial (Supelco), which was then filled with 50 μl of hexane (Sigma-Aldrich) containing 40 ng/μl hexacosane (Fluka) as internal standard. The vials were shaken gently for 5 min at room temperature to allow any volatiles to enter the hexane. A 2 μl aliquot of each extract was taken for GC-MS analysis. Samples were run in random order and in technical duplicate. The analytical column used was a 30 m BPX5, 0.25 mm i.d., 0.25 μm film (S.G.E), installed in a Thermo Scientific Polaris GC-MS instrument. The carrier gas was helium at a flow rate of 0.8 ml/min. The split-less injection mode was employed and the injection temperature was 230°C. The column oven temperature program was: initial temperature 50°C held for 2 min, then 25°C/min to 150°C, followed by 10°C/min to 290°C with a final time of 14 min. The transfer line temperature was 250°C and the mass spectrometer source temperature was 200°C. The mass range scanned was 40–600 Daltons. GC-MS TIC peak areas were determined using the peak area measuring tool in the Thermo Excalibur software.
Statistical Analysis
All statistical analyses were performed using R 3.1.0 (R core development team, 2008). Propensity at mating was analyzed by a generalized linear model assuming a Binomial distribution with a logit link function (McCullagh and Nelder, 1989), followed by post-hoc Tukey HSD tests to determine differences between treatments. Numbers of sperm transferred by males, and eggs laid by females were transformed to normality using a Box-Cox procedure (Box and Cox, 1964). Transformed data were analyzed using two generalized linear models assuming a Gaussian distribution with an identity link function (McCullagh and Nelder, 1989); these were followed by post-hoc Tukey HSD tests to determine differences between treatments. Relatedness, antibiotic treatment, diet and the interactions were used as explanatory variables and the numbers of sperm transferred and eggs laid by females as dependent variables.
Overall the all set of CHC extracted, 14 CHC compounds were quantified by integrating the area under each chromatograph peak for each fly. CHC scores were normalized by calculating the quantity relative to that of an internal standard peak within each sample, which corresponded to a fixed 40 ng/μl concentration of hexacosane. As CHC scores were not normally distributed, they were Log transformed. We used the “MCMCglmm” package in R (Hadfield, 2010) to analyze CHC profiles using multivariate mixed models with Bayesian inference. Due to the high dimensionality of the CHC data (14 traits) and the complexity of the model needed, the models were over-parameterized when ran with all 14 CHCs, so we analyzed three separate groups of CHC: (1) Female-specific dienes (tricosidiene, pentacosadiene, heptacosadiene, and nonacosadiene); (2) Alkanes and monoenes found in both sexes (docosane, tricosene, tricosane, pentacosene, and pentacosane); and (3) Methyl-branched alkanes found in both sexes (methyldocosane, methyl-tetracosane, methyl-hexacosane, methyl-octacosane, and methyltriacontane). For each set of CHCs, we compared two models. The first model included antibiotic treatment and diet (and their interaction) as fixed effects, and family and family × antibiotic treatment interaction as random effects. The second model had the same fixed effect structure, and only family as a random effect. We tested the significance of the genotype-by environment interaction across families and antibiotic treatments by comparing the full model with a model where the family × antibiotic interaction term had been removed. For all models, Markov chains were run for 2,000,000 iterations, with a burn-in of 100,000 and a thinning interval of 50. Each model used a proper prior distribution, and trait-specific variances and co-variances were estimated with the “us” (unstructured) variance structure in MCMCglmm. We compared the models with and without the interaction between family and antibiotic for each subset of CHCs using the model deviance information criterion (DIC) (Spiegelhalter et al., 2002), and estimated the support for each model by calculating the model posterior probability. We then used the posterior distribution of the models to calculate the genetic correlation for expression of each CHC across antibiotic treatments, as a between-environment genetic correlation (Lynch and Walsh, 1998). These models therefore allowed a more detailed analysis of genetic variation that might underlie CHC production and the response to the environmental treatments.
In order to visualize differences in CHC profile between families, we carried out linear discriminant analysis (LDA) with all 14 CHCs modeled against “family” as a four-level factor. This analysis was done twice: first on the subset of data without streptomycin, and second on the subset of data with streptomycin. This allowed us to explore the antibiotic x family interaction by examining the separation between families in overall CHC profile, compared across the two antibiotic treatments.
Results
Probability of Mating With an Unrelated Male (Figure 2)
When modeled as fixed effects, the microbiota status (intact/altered) (GLM, χ2 = 0.232, 254 df, p = 0.629) as well as diet (GLM, χ2 = 3.664, 254 df, p = 0.055) had no significant effect on female mating propensity. By contrast, relatedness significantly affected female's propensity to mate (GLM, χ2 = 5.704, 254 df, p = 0.016), with female mating propensity reduced when the male was unrelated to her (Figure 2). Pairwise comparisons of different combinations of factors showed that female mating propensity was reduced with unrelated males, compared to related (Tukey HSD, p = 0.016), developing on the same diet as the female. This effect disappeared when males developed on a different diet (Tukey HSD, p = 0.972). In the same way, female propensity to mate with unrelated males decreased when unrelated males developed on the same diet as the female compared to unrelated males that developed on a different diet (Tukey HSD, p = 0.026). This is not observed for related males (Tukey HSD, p = 0.999). Moreover, diet and the microbiota interacted significantly, with female propensity to mate reduced with males reared on the same diet as the female, when the microbiota was intact, compared to males reared on a different diet (Tukey HSD, p = 0.013). This effect disappeared when the male microbiota was altered (Tukey HSD, p = 0.834) (Figure 2).
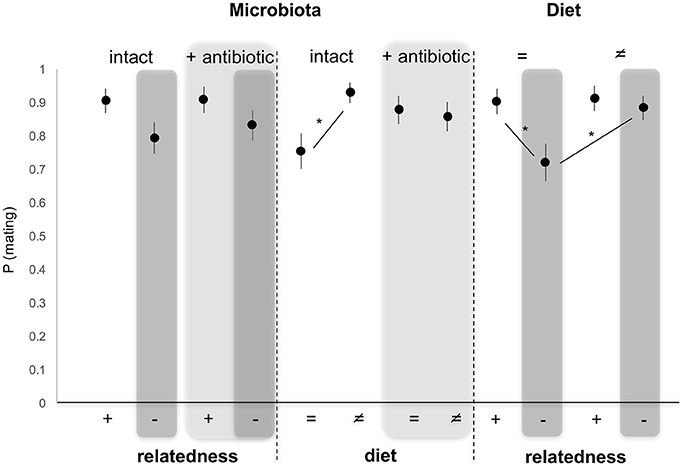
Figure 2. Variation in female propensity to mate when exposed to a single related (brother) (+) or unrelated (–, dark gray) male, reared on the same (=) or different (≠) diet, and whose microbiota was intact or antibiotically-treated (light gray). *Depicts P < 0.05.
Number of Sperm Transferred (Figure 3)
Numbers of sperm transferred to females (our measure of male investment in copulation) were not altered by diet [GLM, F(3, 116) = 0.140, p = 0.708], nor by the microbiota status [GLM, F(3, 116) = 2.214, p = 0.139] as fixed effects. By contrast, numbers of sperm transferred were significantly altered by relatedness of the female partner [GLM, F(3, 116) = 7.850, p = 0.006], with males transferring more sperm to unrelated females compared to sisters (Figure 3). Pairwise comparisons of different combinations of factors showed that males mating with females reared on different diets transferred more sperm to unrelated females than to related one (Tukey HSD, p = 0.014). This effect disappeared when females developed on the same diet as their male partners (Tukey HSD, p = 0.802). In the same way, sperm number was determined by a significant interaction between relatedness and microbiota. Indeed, numbers of sperm transferred increased when males mated with antibiotically-treated unrelated females compared to antibiotically-treated sisters (Tukey HSD, p = 0.035), while this effect disappeared when males mated with untreated unrelated females compared to untreated sisters (Tukey HSD, p = 0.615). This was not a side effect of the altered microbiota (or the use of antibiotic) as there were no significant differences in numbers of sperm transferred when males mated with untreated- or antibiotically-treated unrelated females (Tukey HSD, p = 0.313), as well as when males mated with untreated- or antibiotically-treated sisters (Tukey HSD, p = 0.995) (Figure 3).
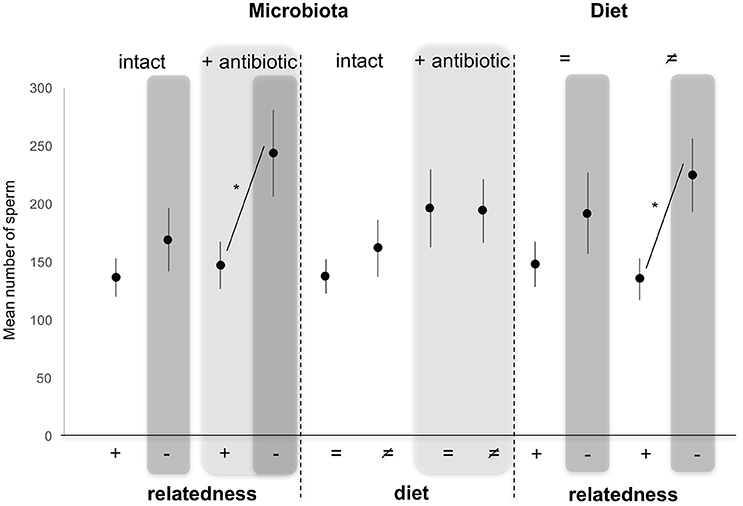
Figure 3. Variation in mean sperm (±s.e.) numbers counted in females after mating with a related (brother) (+) or an unrelated (–, dark gray) male, reared on the same (=) or different (≠) diet, and whose microbiota was intact or antibiotically-treated (light gray). *Depicts P < 0.05.
Numbers of Eggs Laid by Females (Figure 4)
Numbers of eggs laid by females (our measure of fitness consequences) were not altered by diet [GLM, F(3, 86) = 1.946, p = 0.166] as a fixed effect. By contrast, numbers of eggs laid were significantly decreased when the microbiota was altered [GLM, F(3, 86) = 7.315, p = 0.008], and increased when males mated with unrelated females compared to sisters [GLM, F(3, 86) = 4.895, p = 0.029] as fixed effects (Figure 4). Pairwise comparisons of different combinations of factors showed that numbers of eggs laid by females reared on a different food than their male partner decreased significantly when females were antibiotically treated compared to untreated females (Tukey HSD, p = 0.038), while this effect disappeared when females were reared on the same diet as their male partner (Tukey HSD, p = 0.750) (Figure 4).
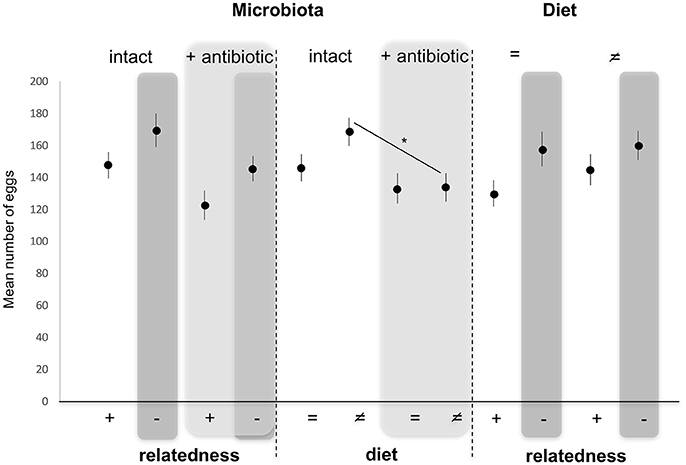
Figure 4. Variation in mean egg (±s.e.) numbers counted in females after mating with a related (brother) (+) or an unrelated (–, dark gray) male, reared on the same (=) or different (≠) diet, and whose microbiota was intact or antibiotically-treated (light gray). *Depicts P < 0.05.
Variation in CHC Profile in Relation to Relatedness and Diet in the Presence or Absence of Gut Bacteria (Figure 5)
CHCs were extracted from pairs of female sisters reared on different diets, and whose microbiota was intact or antibiotically-treated, and analyzed by GC-MS. Of these CHCs, we analyzed 14 of them using multivariate mixed models with Bayesian inference (Markov Chain Monte Carlo method) (Supplementary Data Sheet 1). Due to the high dimensionality of the CHC data (14 traits) and the complexity of the model needed, three separate groups of CHC were analyzed: (1) Female-specific dienes (tricosidiene, pentacosadiene, heptacosadiene, and nonacosadiene); (2) Alkanes and monoenes found in both sexes (docosane, tricosene, tricosane, pentacosene, and pentacosane); and (3) Methyl-branched alkanes found in both sexes (methyldocosane, methyl-tetracosane, methyl-hexacosane, methyl-octacosane and methyltriacontane). For each set of CHCs, we compared two models. The first model included antibiotic treatment and diet (and their interaction) as fixed effects, and family and family × antibiotic treatment interaction as random effects. The second model had the same fixed effect structure, and only family as a random effect. By excluding the family × antibiotic interaction when compared to the first model, comparisons of these two models tested the significance of the genotype-by environment interaction across families and antibiotic treatment.
Overall, the fixed effects of diet, antibiotic treatment and diet x antibiotic were not significant for any CHC (results not shown). The best model for the expression of female-specific CHCs did not include the interaction between relatedness and antibiotic treatment, but the best models for the two groups of CHCs expressed by both sexes did include the relatedness × antibiotic interaction (Table 1). This interaction term suggests that there is genetic variation between these families in terms of how CHC production responds to the intact/altered microbiota.
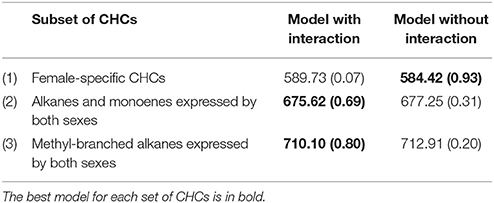
Table 1. Summary of the model results showing the model DIC (deviance information criterion) and the model posterior probability (in brackets) for the models with and without the family × antibiotic interaction for each subset of CHCs.
For each CHC individually, the genetic correlation across antibiotic treatments was generally low, although 95% credible intervals were wide and overlapping in each case due to the relatively small number of families used in the calculations (Table 2). Consistent with the overall results of the models, where the family × antibiotic interaction was not significant for female-specific CHCs, correlations for these CHCs tended to be higher than for the CHCs expressed by both sexes.
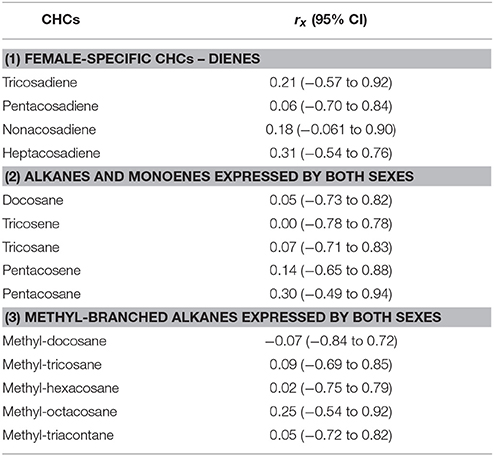
Table 2. Genetic correlation of each CHC across antibiotic treatments (rx), calculated as a cross-environment genetic correlation following (Lynch and Walsh, 1998), with 95% credible interval (CI) around each estimate.
Further analysis of CHCs used linear discriminant analysis (LDA) to visualize overall differences in CHC profiles among family groups (Supplementary Data Sheet 1). This analysis used all 14 CHC traits as the explanatory variables, and analyzed separation between the four families. This was done separately for the data with and without streptomycin treatment, in order to explore the antibiotic × family interaction identified for some CHCs in the linear modeling above. The results show that although families group together fairly consistently when the microbiota is intact or antibiotically treated (Figure 5), this grouping differs according to the microbiota status. Unexpectedly, separation appears to be clearer when microbiota is intact (without antibiotic), although the separation on the first discriminant vector (LD1) depends heavily on two families with low sample size (colored red and blue in Figure 5). Separation between families with antibiotic is clear as an interaction between the variation described by the first two discriminant vectors (Figure 5).
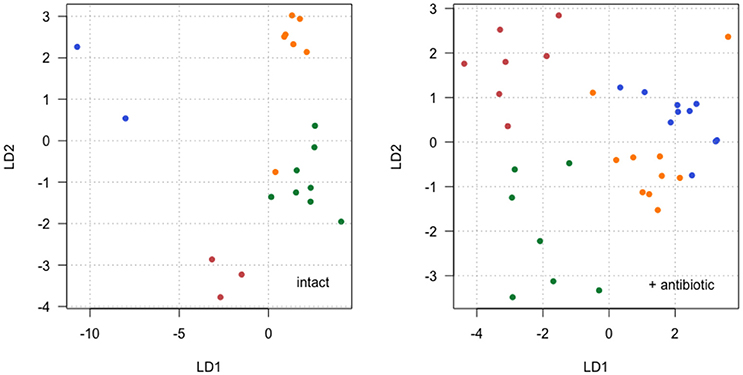
Figure 5. Individual female CHC scores along the first linear discriminant vector (LD1) plotted against scores along the second LD vector (LD2) for two separate LDA: intact microbiota (Left) and with antibiotic added (Right). Families are identified by point color with each color representing a different family. In the LDA without antibiotic (Left), 71 and 22% of variation between families is described by LD1 and LD2 respectively, and in the LDA with antibiotic (Right), 6 and 25% of variation between families is described by LD1 and LD2 respectively.
Discussion
In females, we found the major criterion determining female propensity to mate is the relatedness of the male they mate with, with mating being less probable with unrelated males compared to related ones (brothers). Interestingly, relatedness interacted significantly with diet, and diet interacted significantly with the microbiota the male harbored (intact/altered). Indeed, females were able to detect male relatedness only when these males developed on the same diet as the female. Moreover, the female ability to detect that a male developed on the same diet as she did, is dependent on the microbial status of the male. Therefore, although female mating propensity is determined by relatedness of their male partners, assessment of male relatedness is based on the diet the male developed on, but only when the male microbiota was intact. Female D. melanogaster relied on microbiota-depend olfactory signals of males to detect whether they developed on same diet as the female, and males developing on a different diet to the female are potentially viewed as being more likely to be unrelated to her than males developing on the same diet as she did. Some of our results are contrary to those of Sharon et al. (2010), who found that flies preferentially mate with individuals reared on the same media, but mate randomly when the microbiota is suppressed (but see Leftwich et al., 2017). This difference is likely due to the strain of flies used (inbred versus outbred), Wolbachia infection status (Wolbachia positive vs. negative) and the additional measures used in our current study (relatedness of mating pairs).
Whilst we have not quantified the microbiome in this study, previous studies have demonstrated the effect of antibiotics and of diet on the resident microbiota in D. melanogaster (e.g., Sharon et al., 2010; Chandler et al., 2011; Ridley et al., 2013; Wong et al., 2013; Broderick et al., 2014; Leftwich et al., 2017). For example, Ridley et al. (2013) determined the microbiota for D. melanogaster reared on a conventional glucose-agar-yeast diet and the same diet supplemented with chlortetracycline (CT) (50 μg ml−1). They found that the addition of CT to the dietary media caused an overall reduction in the bacteria present but the occurrence of small numbers of some species, e.g., Lactobacillus brevis and L. plantarum were only present in the CT reared flies. Similarly, Broderick et al. (2014) found that shifting flies onto an autoclaved diet limited gut bacterial diversity to only L. plantarum, A. pasteurianus, and L. brevis. Further, sampling of wild populations of D. melanogaster living on varying diet of decaying vegetation and apples, demonstrated drastically different bacteria, with none of the same species detected in each population (Wong et al., 2013).
In males, we found that the major factor determining male sperm investment is relatedness, which is only detected by the male when the female developed on a different diet to the male, providing that the female microbiota was altered. In fact, males transferred more sperm to unrelated females than to sisters when females developed on a different diet than the male, or when female microbiota had been altered via antibiotics. This translated into significantly higher numbers of eggs laid by inseminated females. This indicates that males increase their investment when mating with unrelated females only when the female developed on a different diet that the male, or when the microbiota is altered. Our results suggest that males are not able to fine tune their sperm investment when females developed on the same diet as them, because, either directly or via metabolite production, the microbiota mask female olfactory cues allowing kin recognition to occur in D. melanogaster. This has fitness consequences for mating partners for at least 3 days after mating, as females mated to an unrelated male will lay more eggs over this period. The previous suggestion that ecology determines kin recognition is supported here (Lizé et al., 2014). D. melanogaster is a generalist, polyandrous species that incurs little cost when mating with a related partner. If kin recognition is of secondary importance within this species, it is probable that other aspects of its ecology, such as familiarity or environment, provide more important mate choice cues. Therefore, this study suggests that an intact microbiota in females could disfavor male outbreeding strategies in laboratory D. melanogaster.
Our analysis of CHC profiles suggested that variation occur in females according to family, and that the microbiota only impacts on the CHCs that can be expressed by both sexes (a significant family × microbiota interaction for alkanes, monoenes, and methyl-branched alkanes). Therefore, any modification of the expression of these compounds in one sex are susceptible to also occur in the other sex in a common environment, which would maintain male-female recognition as partners. Maintaining male-female recognition may have fitness consequences for the microbiota, as this is vertically transmitted by female D. melanogaster to their offspring via egg-smearing (Bakula, 1969), so successful host reproduction will increase microbiota fitness. This family × microbiota interaction was non-significant for the dienes we analyzed, which are only expressed in females in this species (Dallerac et al., 2000; Chertemps et al., 2006). It is therefore likely that quantitative variation in expression of CHCs that are shared between males and females (alkanes, monoenes, and methyl-branched alkanes) could be used as kin recognition cues by males to determine whether a female is related, but that this sexual signal of relatedness is masked by the microbiota at least in females. D. melanogaster also acquire microbiota via horizontal transfer between individuals. For example, when females deposit eggs onto a food source, attractant pheromones are also released encouraging additional females to aggregate (Bartelt et al., 1985; Wertheim et al., 2005). It may be that the resulting transfer of microbiota among females and emerging larvae is responsible for masking this sexual signal of relatedness.
Although females D. melanogaster mate preferentially with their brothers, males invest more sperm in unrelated females with impaired microbiota, which results in more eggs being laid by these unrelated females. Three hypotheses (detailed below) may explain these contradictory strategies developed by males and females of this species: (1) there is a male trade-off between searching costs and kin recognition benefits in nature (Kokko and Ots, 2006), (2) females take advantage of the microbiota in the context of sexual conflict, (3) the microbiota manipulates the host outbreeding strategy.
(1) Male trade-off between searching costs and kin recognition benefits in nature
In males, a trade-off might exist between distinguishing related females in nature, whose microbiota is much more diverse (Chandler et al., 2011), and the energy allocated by males to find females. If the energy allocated to find a female in nature is higher than the fitness reward gained by distinguishing a related female, then males should not differentiate females based on relatedness. Context-dependent kin recognition is widely used by amphibian (Blaustein and Waldman, 1992; Hokit et al., 1996; Nichols, 2017), and hymenopteran species (Hepper, 1991; Starks et al., 1998; Buczkowski and Silverman, 2005) and this could explain why kin recognition is observed only when the microbiota is altered in the laboratory in D. melanogaster.
(2) Females take advantage of the microbiota in the context of sexual conflict
Our data showed that female mating propensity was influenced by male relatedness. Other studies have shown that D. melanogaster females prefer to mate with related males, and that they are therefore more prone to inbreeding (Loyau et al., 2012; Robinson et al., 2012). Our data with regards to male investment demonstrated the opposite, with males promoting outbreeding but only when female microbiota was altered. This sheds light on the sexual conflict that has been described in this species (Chapman et al., 2003), but also highlights that female may use their microbiota to take advantage of this conflict, thereby rendering a male outbreeding strategy impossible.
(3) The microbiota manipulates the host breeding strategy
Finally, another alternative would be that outbreeding in D. melanogaster is costly for the microbiota. Currently, there is no data describing this phenomenon and further studies are needed to demonstrate it. However, host outbreeding strategies increase the genetic diversity in their offspring, which is known to increase immune defense (van Houte et al., 2016). Increasing immune defense might be costly for the microbiota (Bolnick et al., 2014).
In this study, female propensity for mating was greater for related males, with relatedness being assessed through the diet the male developed in, and most probably through the microbiota induced by diets. By contrast, males invested more sperm in unrelated females, whose microbiota was antibiotically treated, and this influences subsequent egg production. D. melanogaster is a generalist species, thus the alteration of the composition of the microbiota community as a result of feeding on different food sources is likely to have important ecological consequences for mating behavior, and in turn divergence between populations. To our knowledge, this is the first evidence that an intact microbiota, via its effects on the host chemical communication, can alter the host breeding strategy by disfavoring outbreeding.
Ethics Statement
No ethical approval or specific permit was needed for rearing and experimental use of Drosophila melanogaster.
Author Contributions
AL, TP, and ZL designed the experiments. CH, HC, FI, MP, TP, and AL performed the experiments. ZL, FI, CH, and AL wrote the manuscript and all authors amended it.
Funding
This work was supported by the Natural Environment Research Council (grant number NE/L002450/1).
Conflict of Interest Statement
The authors declare that the research was conducted in the absence of any commercial or financial relationships that could be construed as a potential conflict of interest.
Acknowledgments
The authors thank Professor Rob Beynon for GC-MS advice, and Professor Steve Paterson for comments on the manuscript. We also thank Chris Maguire, Rudi Verspoor, Zara-Louise Cowan, Paulina Giraldo-Perez, Helena Crosland, and Rowan Connell, for assisting with sperm counting and Dr. Raphael Aggio for statistical advice. Finally, we thank the reviewers whose comments improved the manuscript.
Supplementary Material
The Supplementary Material for this article can be found online at: https://www.frontiersin.org/articles/10.3389/fevo.2018.00029/full#supplementary-material
References
Bakula, M. (1969). The persistence of a microbial flora during post-embryogenesis of Drosophila melanogaster. J. Invert. Pathol. 14, 365–374. doi: 10.1016/0022-2011(69)90163-3
Bartelt, R., Schaner, A., and Jackson, L. (1985). Cis-vaccenyl acetate as an aggregation pheromone in Drosophila melanogaster. J. Chem. Ecol. 11, 1747–1756. doi: 10.1007/BF01012124
Blaustein, A. R., and Waldman, B. (1992). Kin recognition in anuran amphibians. Anim. Behav. 44, 207–221. doi: 10.1016/0003-3472(92)90027-7
Bolnick, D. I., Snowberg, L. K., Caporaso, J. G., Lauber, C., Knight, R., and Stutz, W. E. (2014). Major histocompatibility complex class IIb polymorphism influences gut microbiota composition and diversity. Mol. Ecol. 23, 4831–4845. doi: 10.1111/mec.12846
Box, G. E. P., and Cox, D. R. (1964). An analysis of transformations. J. Roy. Stat. Soc. B 26, 211–252.
Broderick, N. A., and Lemaitre, B. (2012). Gut associated microbes of Drosophila melanogaster. Gut Microb. 3, 307–321. doi: 10.4161/gmic.19896
Broderick, N. A., Buchon, N., and Lemaitre, B. (2014). Microbiota-induced changes in Drosophila melanogaster host gene expression and gut morphology. mBio 5, e01117–e01114. doi: 10.1128/mBio.01117-14
Brummel, T., Ching, A., Seroude, L., Simon, A. F., and Benzer, S. (2004). Drosophila lifespan enhancement by exogenous bacteria. Proc. Natl. Acad. Sci. U.S.A. 101, 12974–12979. doi: 10.1073/pnas.0405207101
Buczkowski, G., and Silverman, J. (2005). Context-dependent nestmate discrimination and the effect of action thresholds on exogenous cue recognition in the Argentine ant. Anim. Behav. 69, 741–749. doi: 10.1016/j.anbehav.2004.06.027
Chandler, J. A., Lang, J. M., Bhatnagar, S., Eisen, J. A., and Kopp, A. (2011). Bacterial communities of diverse Drosophila species: ecological context of a host–microbe model system. Plos Genet. 7:e1002272. doi: 10.1371/journal.pgen.1002272
Chapman, T., Arnqvist, G., Bangham, J., and Rowe, L. (2003). Sexual conflict. Trends Ecol. Evol. 18, 41–47. doi: 10.1016/S0169-5347(02)00004-6
Chertemps, T., Duportets, L., Labeur, C., Ueyama, M., and Wicker-Thomas, C. (2006). A female-specific desaturase gene responsible for diene hydrocarbon biosynthesis and courtship behaviour in Drosophila melanogaster. Insect. Mol. Biol. 15, 465–473. doi: 10.1111/j.1365-2583.2006.00658.x
Corby-Harris, V., Pontaroli, A. C., Shimkets, L. J., Bennetzen, J. L., Habel, K. E., and Promislow, D. E. L. (2007). Geographical distribution and diversity of bacteria associated with natural populations of Drosophila melanogaster. Appl. Environ. Microbiol. 73, 3470–3479. doi: 10.1128/AEM.02120-06
Courvalin, P. (1994). Transfer of antibiotic resistance genes between gram-positive and gram-negative bacteria. Antimicrob. Agents Chemother. 38, 1447–1451. doi: 10.1128/AAC.38.7.1447
Cox, C. R., and Gilmore, M. S. (2007). Native microbial colonization of Drosophila melanogaster and its use as a model of Enterococcus faecalis pathogenesis. Infect. Immun. 75, 1565–1576. doi: 10.1128/IAI.01496-06
Dallerac, R., Labeur, C., Jallon, J.-M., Knipple, D. C., Roelofs, W. L., and Wicker-Thomas, C. (2000). A delta 9 desaturase gene with a different substrate specificity is responsible for the cuticular diene hydrocarbon polymorphism in Drosophila melanogaster. Proc. Natl. Acad. Sci. U.S.A. 97, 9449–9454. doi: 10.1073/pnas.150243997
Dhole, S., and Pfennig, K. S. (2014). Age-dependent male mating investment in Drosophila pseudoobscura. PLoS ONE 9:e88700. doi: 10.1371/journal.pone.0088700
Eakley, A. L., and Houde, A. E. (2004). Possible role of female discrimination against 'redundant' males in the evolution of colour pattern polymorphism in guppies. Proc. R. Soc. B. 271, S299–S301. doi: 10.1098/rsbl.2004.0165
Fenollar, F., Maurin, M., and Raoult, D. (2003). Wolbachia pipientis growth kinetics and susceptibilities to antibiotics determined by immunofluorescence staining and real-time PCR. Antimicrob. Agents Chemother. 47, 1665–1671. doi: 10.1128/AAC.47.5.1665-1671.2003
Ferveur, J. F. (2005). Cuticular hydrocarbons: their evolution and roles in Drosophila pheromonal communication. Behav. Genet. 35, 279–295. doi: 10.1007/s10519-005-3220-5
Friberg, U. (2006). Male perception of female mating status: its effect on copulation duration, sperm defence and female fitness. Anim. Behav. 72, 1259–1268. doi: 10.1016/j.anbehav.2006.03.021
Gorman, M. L. (1976). A mechanism for individual recognition by odour in Herpestes auropunctatus (Carnivora: Viverridae). Anim. Behav. 24, 141–145. doi: 10.1016/S0003-3472(76)80107-8
Graf, E., and Benz, G. (1970). Toxicity of streptomycin and terramycin, and influence on growth and developmental time of Drosophila melanogaster. Experientia 26, 1339–1341. doi: 10.1007/BF02113017
Hadfield, J. D. (2010). MCMC methods for multi-response generalized linear mixed models: the MCMCglmm R package. J. Stat. Softw. 33, 1–22. doi: 10.18637/jss.v033.i02
Hokit, G. D., Walls, S. C., and Blaustein, A. R. (1996). Context-dependent kin discrimination in larvae of the marbled salamander, Ambystoma opacum. Anim. Behav. 52, 17–31. doi: 10.1006/anbe.1996.0149
Hughes, D. P., Brodeur, J., and Thomas, F. (2012). Host Manipulation by Parasites. Oxford, UK: Oxford University Press.
Kokko, H., and Ots, I. (2006). When not to avoid inbreeding. Evolution 60, 467–475. doi: 10.1111/j.0014-3820.2006.tb01128.x
Leftwich, P. T., Hutchings, M. I., and Chapman, T. (2017). Gut microbiomes and reproductive isolation in Drosophila. Proc. Natl. Acad. Sci. U.S.A. 114, 12767–12772. doi: 10.1073/pnas.1708345114
Lizé, A., McKay, R., and Lewis, Z. (2013). Gut microbiota and kin recognition. Trends Ecol. Evol. 28, 325–326. doi: 10.1016/j.tree.2012.10.013
Lizé, A., McKay, R., and Lewis, Z. (2014). Kin recognition in Drosophila: the importance of ecology and gut microbiota. ISME J. 8, 469–477. doi: 10.1038/ismej.2013.157
Loyau, A., Cornuau, J. H., Clobert, J., and Danchin, E. (2012). Incestuous sisters: mate preference for brothers over unrelated males in Drosophila melanogaster. PLoS ONE 7:e51293. doi: 10.1371/journal.pone.0051293
Lynch, M., and Walsh, B. (1998). Genetics and Analysis of Quantitative Traits. Sunderland: Sinauer Associates.
Najarro, M. A., Sumethasorn, M., Lamoureux, A., and Turner, T. L. (2015). Choosing mates based on the diet of your ancestors: replication of non-genetic assortative mating in Drosophila melanogaster. Peer J. 3:e1173. doi: 10.7717/peerj.1173
Nichols, H. J. (2017). The causes and consequences of inbreeding avoidance and tolerance in cooperatively breeding vertebrates. J. Zool. 303, 1–14. doi: 10.1111/jzo.12466
Ödeen, A., and Moray, C. M. (2008). Drosophila melanogaster virgins are more likely to mate with strangers than familiar flies. Naturwissenschaften 3, 253–256. doi: 10.1007/s00114-007-0314-3
Price, T. A. R., Bretman, A. J., Snook, R. R., Hurst, G. D. D., and Wedell, N. (2008). Sex ratio distorter reduces sperm competitive ability in an insect. Evolution 62, 1644–1652. doi: 10.1111/j.1558-5646.2008.00386.x
R core development team (2008) R: A Language and Environment for Statistical Computing. Vienna: R Foundation for Statistical Computing.
Ren, C., Webster, P., Finkel, S. E., and Tower, J. (2007). Increased internal and external bacterial load during Drosophila aging without life-span trade-off. Cell Metab. 6, 144–152. doi: 10.1016/j.cmet.2007.06.006
Ridley, E. V., Wong, A. C. N., and Douglas, A. E. (2013). Microbe-dependent and nonspecific effects of procedures to eliminate the resident microbiota from Drosophila melanogaster. Appl. Environ. Microbiol. 79, 3209–3214. doi: 10.1128/AEM.00206-13
Robinson, S. P., Kennington, W. J., and Simmons, L. W. (2012). Preference for related mates in the fruit fly, Drosophila melanogaster. Anim. Behav. 84, 1169–1176. doi: 10.1016/j.anbehav.2012.08.020
Roh, S. W., Nam, Y. D., Chang, H. W., Kim, K. H., Kim, M. S., Ryu, J. H., et al. (2008). Phylogenetic characterization of two novel commensal bacteria involved with innate immune homeostasis in Drosophila melanogaster. Appl. Environ. Microbiol. 74, 6171–6177. doi: 10.1128/AEM.00301-08
Schatz, A., Bugle, E., and Waksman, S. A. (1944). Streptomycin, a substance exhibiting antibiotic activity against gram-positive and gram-negative bacteria. Exp. Biol. Med. 55, 66–69. doi: 10.3181/00379727-55-14461
Sharon, G., Segal, D., Ringo, J. M., Hefetz, A., Zilber-Rosenberg, I., and Rosenberg, E. (2010). Commensal bacteria play a role in mating preference of Drosophila melanogaster. Proc. Natl. Acad. Sci. U.S.A. 107, 20051–20056. doi: 10.1073/pnas.1009906107
Spiegelhalter, D. J., Best, N. G., Carlin, B. P., and van der Linde, A. (2002). Bayesian measures of model complexity and fit. J. Roy. Stat. Soc. B 64, 583–639. doi: 10.1111/1467-9868.00353
Starks, P. T., Fisher, D. J., Watson, R. E., Melikian, G. L., and Nath, S. D. (1998). Context dependent nestmate discrimination in the paper wasp, Polistes dominulus: a critical test of the optimal acceptance threshold model. Anim. Behav. 56, 449–458. doi: 10.1006/anbe.1998.0778
Strassman, J. E., Gilbert, O. M., and Queller, D. C. (2011). Kin discrimination and cooperation in microbes. Annu. Rev. Microbiol. 65, 349–367. doi: 10.1146/annurev.micro.112408.134109
van Houte, S., Ekroth, A. K. E., Broniewski, J. M., Chabas, H., Ashby, B., Bondy-Denomy, J., et al. (2016). The diversity generating benefits of a prokaryotic adaptive immune system. Nature 532, 585–588. doi: 10.1038/nature17436
Wall, D. (2016). Kin recognition in bacteria. Annu. Rev. Microbiol. 70, 143–160. doi: 10.1146/annurev-micro-102215-095325
Wertheim, B., van Baalen, E.-J. A., Dicke, M., and Vet, L. E. M. (2005). Pheromone-mediated aggregation in non-social arthropods: an evolutionary ecological perspective. Annu. Rev. Entomol. 50, 321–346. doi: 10.1146/annurev.ento.49.061802.123329
Wong, A. C. N., Chaston, J. M., and Douglas, A. E. (2013). The inconstant gut microbiota of Drosophila species revealed by 16S rRNA gene analysis. ISME J. 7, 1922–1932. doi: 10.1038/ismej.2013.86
Keywords: microbiota, sexual signaling, chemical communication, kin recognition, mating behavior, outbreeding strategy
Citation: Heys C, Lizé A, Colinet H, Price TAR, Prescott M, Ingleby F and Lewis Z (2018) Evidence That the Microbiota Counteracts Male Outbreeding Strategy by Inhibiting Sexual Signaling in Females. Front. Ecol. Evol. 6:29. doi: 10.3389/fevo.2018.00029
Received: 04 December 2017; Accepted: 09 March 2018;
Published: 22 March 2018.
Edited by:
Peter Schausberger, Universität Wien, AustriaReviewed by:
Michael J. Pauers, Milwaukee Public Museum, United StatesYukie Sato, University of Tsukuba, Japan
Copyright © 2018 Heys, Lizé, Colinet, Price, Prescott, Ingleby and Lewis. This is an open-access article distributed under the terms of the Creative Commons Attribution License (CC BY). The use, distribution or reproduction in other forums is permitted, provided the original author(s) and the copyright owner are credited and that the original publication in this journal is cited, in accordance with accepted academic practice. No use, distribution or reproduction is permitted which does not comply with these terms.
*Correspondence: Anne Lizé, YW5uZS5saXplQGxpdmVycG9vbC5hYy51aw==
†These authors have contributed equally to this work.