- 1Department of Evolutionary Neuroethology, Max Planck Institute of Chemical Ecology, Jena, Germany
- 2Department of Molecular Ecology, Max Planck Institute of Chemical Ecology, Jena, Germany
Most pollinators visit flowers in the search of nectar rewards. However, as the floral nectar can often not be directly detected by pollinators, many flower visitors use secondary metabolites such as odor- or taste-proxies to anticipate nectar quantity and quality. Plants might exploit these sensory inferences of the pollinator to increase their pollination rates without increasing their caloric investment into their floral rewards. Here we investigated the effects of natural variation in certain primary and secondary floral metabolites in three populations of the wild tobacco, Nicotiana attenuata, on the pollination behavior of the hawkmoth Manduca sexta. Although offering the same caloric value per flower, the plants of these populations differ in the compositions and concentrations of sugars within the nectar. Moreover, the flowers of these plants emitted highly contrasting levels of attractive floral volatiles (benzyl acetone), but did not differ in the amounts of defensive nectar metabolites (nicotine). In wind tunnel assays with M. sexta moths, plants from those populations that released the largest amount of benzyl acetone as well as those that had a higher ratio of nectar sucrose were more frequently visited and re-visited by the hawkmoth. High emissions of benzyl acetone additionally correlated with a higher time investment of the moths into individual flowers on each visit, leading to the largest foraging success of the moths on those flowers that were most strongly scented. We propose that it is the variation of flower metabolites and their detection by the pollinator rather than the actual caloric value of the nectar, which determines pollinator visitations to a certain flower population. Hence, plants could potentially create a specialist pollinator community by altering their floral signals, either by producing volatiles that pollinators prefer or by providing nectar sugars that pollinators are most sensitive to, while at the same time keeping the caloric value of their nectar rewards at a constant level. These findings highlight the importance of variations among floral signals and their detection by specific pollinators for plant diversification as well as for the evolution of specific plant-pollinator interactions.
Introduction
While foraging, pollinators face a “reliability-detectability”-problem (Vet and Dicke, 1992): The calories in the nectar or pollen, which could reliably reflect the value of a successful foraging attempt, only become detectable to the insect after entering the hemolymph, and thus too late for the pollinator's next foraging decision (Yapici et al., 2016). Peripheral chemoreceptors, in contrast, can sense floral volatiles already from a great distance and detect the nectar and pollen composition with great accuracy, already upon contact; however, these volatile compounds are only loosely correlated with the quality of a nectar meal (von Arx et al., 2012) and even gustatory receptors only inform about the nectar or pollen taste but not its nutritional value. To solve this “reliability-detectability” problem many insect pollinators have either evolved close relationships with specific groups of plants, which offer reliable rewards and have thereby acquired innate preferences for floral compounds specific to these plants or show accurate learning abilities for those compounds reliably associated with a flower reward (Waser et al., 1996; Knauer and Schiestl, 2015). Such preferences for certain volatiles potentially enable the pollinators to rapidly detect valuable floral resources without prior sampling, while a special sensitivity for certain taste compounds might enable a reliable evaluation of the nectar or pollen quality before ingestion. However, flower metabolites are often highly variable among plant populations, allowing for the genetic isolation of different plant populations through the specific preferences of certain pollinators to these compounds (Schiestl and Johnson, 2013; Delle-Vedove et al., 2017).
The hawkmoth Manduca sexta, which solely forages for floral nectar, shows a strong innate preference for certain aromatic and terpenoid floral volatiles, which are emitted during the night by those flowers typically pollinated by M. sexta and other long-tonged hawkmoths (Riffell et al., 2013). These flowers not only match the olfactory preferences of M. sexta, but their long and slender corolla shape also allows the moth to forage in a most energy-efficient way (Haverkamp et al., 2016a). Thus, in these hawkmoth-pollination systems flower volatiles appear to function as an honest signal for the energy reward offered by the nectar of a certain plant species to the moth, in the sense that the better the match between the olfactory preference of the moth and volatile emissions of the flower, the higher the energy gain of the moth will be.
Yet, even within this mutualistic relationship, plants may still optimize sugar allocation in their floral nectar to maintain the pollinator interaction at the lowest level of investment or might even trick a pollinator into delivering more pollination service for smaller amounts of nectar rewards. One way for the plants to achieve this would be by exploiting the perceptual bias of a pollinator. Certain flower visitors might for example prefer higher nectar volumes even when this leads to a reduction in nectar concentration, which would then allow the plant to be more attractive while at the same time investing the same or even less resources into the nectar (Nachev et al., 2017). Different pollinators also appear to have different preferences for certain sugar types commonly found in floral nectar; hummingbirds and bees for example generally prefer sucrose over glucose and glucose over fructose, while hawkmoths and other Lepidoptera generally prefer sucrose over fructose over glucose (Wykes, 1952; Romeis and Wäckers, 2000; Kelber, 2003). Hence, a flower mainly visited by moths would achieve greater pollination efficiency by producing sucrose and fructose than by producing glucose. In addition, flowers further lace their nectar with different secondary metabolites, such as caffeine or nicotine, which might stimulate the pollinator to invest more time into a certain flower, to visit more flowers of the same type (Kessler and Baldwin, 2007; Kessler, 2012; Zhou et al., 2017) or enhances its memory formation for the particular flower (Wright et al., 2013; Baracchi et al., 2017). Alternatively, secondary metabolites in the nectar might also be repellent to certain non-adapted pollinators and might thus help to create specific feeding niches for those pollinators, which are better able to tolerate these compounds (Johnson et al., 2006; Egan et al., 2016).
These different selection pressures might vary greatly depending on the local pollinator community. In monkey flowers (Mimulus spp.) for example, the exposure to different pollinator communities has likely driven the differentiation of M. lewisii and M. cardinalis. M. lewisii is adapted to pollination by the bumblebee community at high- to mid- altitudes in the Sierra Mountains, USA, while M. cardinalis is mainly pollinated by hummingbirds at low- to mid-altitudes (Schemske and Bradshaw, 1999). Both plants not only differ in flower color and shape, but also in their production of flower volatiles (Byers et al., 2014). Hence, adaptations to the local pollinator species composition might not only influence flower morphology, but also alter flower chemistry either through changes in the production of floral volatiles or through changes in nectar composition (Egan et al., 2016).
Here we compared the flower traits in three different populations of Nicotiana attenuata (Figure 1) and tested the consequences of these variations for the interaction of these flowers with one of their potential pollinators, the hawkmoth M. sexta. The results indicate that it is rather the sensory bias of the pollinator than the absolute caloric value, which drives pollinator attraction. These results have implications for the evolution of chemical signals in flowers and their perception by insect pollinators as well as for the development of specialist pollination systems.
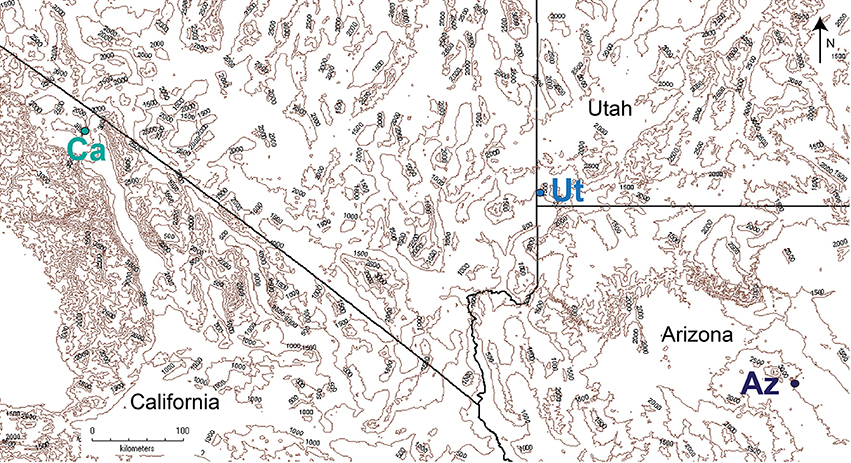
Figure 1. Location of the three wild-type N. attenuata populations used in this study in the western USA. Blue dots indicate locations. Altitude indicated by contour lines at 500 m intervals. Map was generated using QGIS v.2.18.
Materials and Methods
Manduca sexta Moth Rearing
Moths were reared at the Max Planck Institute for Chemical Ecology (MPICE), Jena, Germany as described in Koenig et al. (2015). The moths originated from a colony maintained at the University of Arizona, with no relation to any of the plant populations used in this study and has been maintained at the MPICE for several years. In short: eggs were obtained from female moths ovipositing on N. attenuata plants. After hatching, the caterpillars were transferred to a growth chamber with 27°C, 70% relative humidity (rH), a dark:light cycle of 16:8 h and fed an artificial diet (Koenig et al., 2015). For pupation, caterpillars at the last instar stage were placed individually into wooden blocks and kept under the same climatic conditions until 1 week before enclosure. Pupae were sexed and male and female animals were transferred to separate flight cages with a light regime of 15.5 h daylight, 7.5 h moonlight (0.5 lux) and two 0.5 h transition phases. Climatic conditions were set to 25°C and 70% rH during the daylight phase and to 22°C and 60% rH during the dark phase. For all experiments, we used male moths 72–76 h after enclosure.
Nicotiana attenuata Cultivation
All plants were cultivated in the glasshouse at the MPICE, Jena, Germany. Plants originated from three different wild type N. attenuata native populations: at the D.I. Ranch, Utah (“Ut”) (Santa Clara, UT-USA; altitude 1158 MSL), in Arizona (“Az”) (N 35.215555, W −111.461111, altitude 1967 MSL), and California (“Ca”) (N 37.755277, W −118.594722, altitude 2289 MSL) (Figure 1). Seeds were sterilized and germinated on Petri dishes with Gamborg's B5 media as described in Krügel et al. (2002). Petri dishes with 30 seeds were maintained at 16 h light and 8 h dark conditions in a growth chamber (Percival, Perry, Iowa, USA) for 10 days, and seedlings were transferred to small pots (TEKU JP 3050 104 pots, Pöppelmann, Germany) with Klasmann plug soil (Klasmann-Deilmann, Germany) in a glasshouse table. After 10 days, plants were transferred to 1 L pots and moved to a York Chamber (Johnston Controls, USA) with the same light regime and climatic conditions as the moth flight cages. In all cases one flower per plant was used 1–3 h after opening. Older flowers and surplus flowers were removed the day before.
Wind Tunnel Assays
All behavioral assays were performed in the wind tunnel of the MPICE. The wind tunnel (240 × 90 × 90 cm) was run at a laminar flow of charcoal filtered air under conditions similar to those commonly experienced by foraging hawkmoths (Riffell et al., 2008) (wind speed: 0.37 m/s, temperature: 25°C, relative humidity: 70%). Moths and plants were transferred into separated pre-incubation chambers, set to the same conditions as the wind tunnel, at least 1 h before the experiment. Plants were placed into the tunnel directly before the experiment in such a way that the flower would be at a position 25 cm from the front end, 45 cm from both side walls and 70 cm from the wind tunnel floor. The moths were kept individually in small mesh cages (15 cm × Ø13 cm) and were introduced onto a platform at the rear end of the wind tunnel (10 cm from the rear end, 45 cm from both side walls and 30 cm from the ground of the wind tunnel). The animals were then given 5 min to initiate wing fanning. Moths, which did not start wing fanning during this time, were excluded from the experiment. After take-off, the moths were allowed to fly freely in the tunnel for 4 min during which we observed the foraging behavior via a video camera (Logitech C615, USA, infrared filter removed) recording at 30 Hz and a resolution of 800 × 600 pixels situated at the beginning of the wind tunnel directly behind the flower. We scored a moth, as having approached a flower, as soon as the moth contacted the flower with its proboscis or front legs. Flower handling time was then counted as the time from the first contact until the moth did not have contact anymore with the flower for more than 1 s. In order to exclude learning effects from the examination of the handling time we only considered the first flower approach of each moth for the statistical analyses. Videos whose quality and clarity was inadequate were excluded from the analysis. Finally, we measured the nectar amount of the visited flower and considered a trial as foraging success, whenever the moth had fully removed the nectar from the flower.
Nectar Measurements
To determine the amount of nectar on each population, nectar was measured by carefully removing the flower base and collecting the nectar with a pre-weighed capillary (Brand, Germany) (n = 15 plants per population, 1 flower per plant). The nectar amount was determined by reweighting the capillary and subtracting the two measurements.
Nicotine concentration in the nectar was measured using a LC-Triple Quadrupole-MS instrument, Bruker EVOQ Elite (Bruker, USA), equipped with an HESI ion source as described in Schäfer et al. (2016) (n = 19, 17, 17 plants for Ca, Ut, and Az, respectively, 1 flower per plant). The quantification of nicotine was calculated with D3-nicotine as internal standard.
Sugar measurements in nectar were performed on the same LC-Triple Quadrupole-MS instrument, Bruker EVOQ Elite (Bruker, USA), equipped with an HESI ion source as described in Schäfer et al. (2016). The quantification of glucose, fructose and sucrose were done relative to sorbitol as internal standard.
Floral Volatiles Measurements
Flower volatiles were trapped using 5 mm long Polydimethylsiloxane (PDMS) tubes (inner Ø1.5 mm, outer Ø2.3 mm, Carl Roth, Germany), during 7 consecutive volatile collections (2 h each) starting at 16 h and ending at 6 h. The samples were processed in an untargeted approach using a TDU-GC-MS (Shimadzu, Japan), equipped with a non-polar Rtx- 5MS column (Restek, United States) as described in Kallenbach et al. (2014). PDMS tubes were placed individually into glass tubes and desorbed under a steady stream of nitrogen at 200°C for 8 min. Volatiles were recaptured on a Tenax® trap at −20°C. The trap was then heated to 230°C and injected into the GC- column using helium as a carrier gas. The oven temperature was first held at 60°C for 1 min., ramped at a rate of 10°C min−1 to 150°C and finally increased to 250°C at a rate of 30°C min−1. Electron impact spectra, were recorded at 70 eV at a range of 33–400 m/z using a scan speed of 2,000 Da s−1.
Data was post-processed using R based package XCMS (Smith et al., 2006) to create a compounds and intensity table. Flower volatiles were identified by comparison to the NIST 2.0 Mass Spectral library and verified by synthetic standards (benzyl acetone, CAS: 2550-26-7; benzyl alcohol, CAS: 100-51-6, both Sigma- Aldrich, Germany).
Finally, a principle component analyses (PCA) was performed to compare the volatile profiles of the three plant populations across all detected volatiles using the R package “vegan” (Oksanen et al., 2016). All statistical analyses were carried out using R version 3.4.0.
Corolla Limb Measurement
The corolla limb diameter of each wild-type population was measured using a ruler after full opening (9–10 p.m.). To estimate the diameter we measured transversally the most outer tips of the corolla. For each population, eight different plants (1 flower each) were measured.
Results
In a wind tunnel setup, we observed and recorded the response of M. sexta hawkmoths to the flowers of three wild-type populations (Figure 2). We found that the hawkmoths initially approached all lines similarly (Figure 2A), but differed significantly in their foraging success, with the lowest success on Ca flowers and the highest on Ut flowers (Figure 2B). Furthermore, moths invested different amounts of time (ANOVA, F = 3.63, P = 0.039) and attempts (GLM-ANOVA, F = 5.214, P = 0.0119) at the different flowers, spending significant less time at Ca flowers (Figure 2C) and re-visited these flowers less often (Figure 2D).
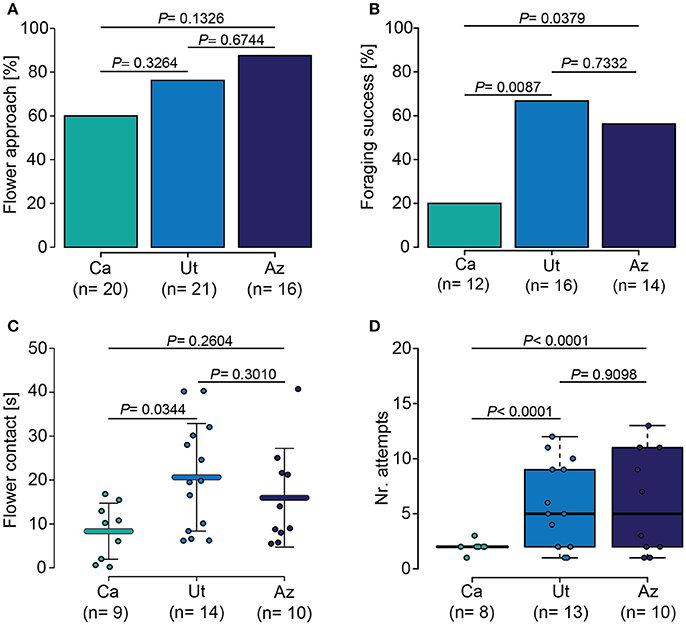
Figure 2. Behavioral response of M. sexta to different N. attenuata populations. (A) Moths initially approached plants of all three populations equally. (B) Moths were significantly more successful when foraging on flowers from the Utah (Ut) and Arizona (Az) populations compared to the California (Ca) population. (C) Moths invested significantly more time into handling flowers from the Ut population than into handling Ca flowers. (D) Moths visited and re-visited flowers of the Ut and Az populations significantly more often than flowers of the Ca population. Error bars in (C) represent the standard deviation. Error bars of the boxplot in (D) indicate 1.5 times the interquartile range; boxes depict 1st and 3rd quartiles. Dots in all graphs show individual measurements. Holm-corrected Fisher's Exact test in case of parametric data in (A,B), ANOVA and GLM followed by a Holm- corrected LSD for parametric data in (C) and non-parametric data in (D), respectively.
We next analyzed the mean nectar amount of the different flower populations and found significant differences among the populations (ANOVA, F = 8.11, P = 0.001), with Ca having a significant larger nectar volume than Ut and Az (Figure 3A). The analysis of the energy content in the nectar based on three sugar components (glucose, fructose, and sucrose) revealed a variation in the energy content per mg of nectar (ANOVA, F = 4.15, P = 0.0366), with a tendency toward a higher content in flowers of the Az population (Figure 3B). When the result on the nectar concentration were combined with the results on the nectar volume, individual flowers from all three populations provided a similar amount of total nectar energy (Kruskal-test, X2 = 4.55, P = 0.1027) (Figure 3C).
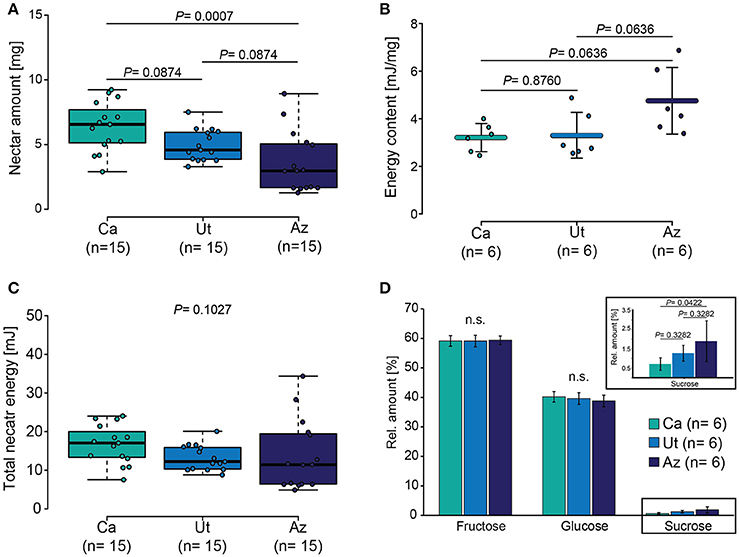
Figure 3. Nectar properties of the three different N. attenuata populations. (A) Boxplot showing the median nectar amount, the 3rd and 4th quartile as well as the interquartile range. (B) Mean ± SD energy content per milligram of nectar. (C) Boxplot of the total nectar energy content. (D) Mean ± SD relative amount of each sugar type (fructose, glucose, and sucrose) in relation to the total sugar amount. Dots in all graphs show individual measurements. Parametric data shown in (B) was tested by ANOVA and non-parametric data shown in (C,D) by Kruskal–Wallis tests; pairwise cases were analyzed with Holm corrected LSD tests.
Despite the similarities regarding the energy content, the populations differed regarding the sugar composition with flowers from the Az populations containing a higher amount of sucrose in the nectar than did flowers from the Ca population (ANOVA, F = 3.86, P = 0.0444) (Figure 3D).
Benzyl acetone and benzyl alcohol were consistently identified in the volatile profiles of all three N. attenuata populations, while other volatiles were only present in trace amounts or could be attributed to background contaminations (Figure 4A). A comparison of the different volatile profiles by PCA revealed a separation of the three plant populations by the first principle component, explaining 66% of the overall variance (Figure 4B). This first component was predominantly influenced by benzyl acetone, while benzyl alcohol contributed little to the discrimination between the three plant populations. When the emissions of benzyl acetone were compared over time, we again found large differences between the plant populations (GLM, F = 9.65, P < 0.005) (Figure 4C), with highest values in flowers of the Ut population 4 h after sunset. In contrast to this, the nicotine content in the nectar of the three plant populations was similar in all cases (Kruskal–Wallis test, X2 = 1.24, P = 0.539) (Figure 4D). Finally the diameter of the corolla limb between the different wild-type lines was found to be smallest in the Ut population and largest in flowers of the Az population (Kruskal–Wallis test, X2 = 16.14, P < 0.001) (Figure 4E).
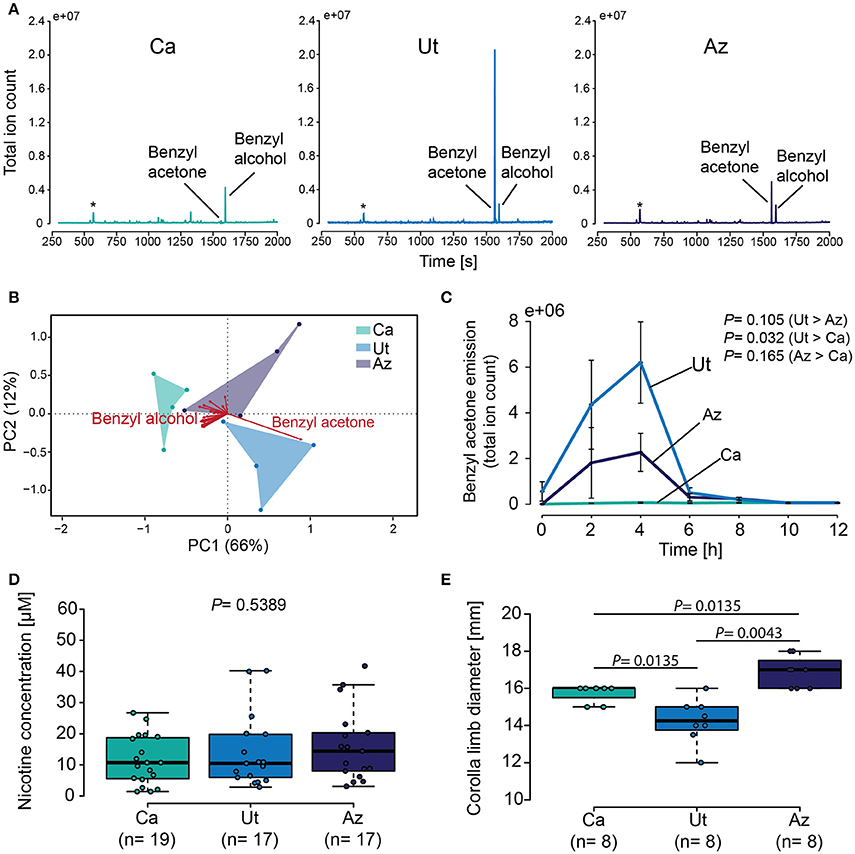
Figure 4. Characterization of the main secondary chemical flower signals and of the flowers visual display. (A) Chromatograms showing flower volatile emissions for plants from the California (Ca), the Utah (Ut) and the Arizona (Az) population from 2 to 4 h after sunset. Asterisk indicates siloxane contamination from collection material. (B) Biplot visualizing the loading of all volatiles present in the floral headspaces of the three different N. attenuata populations. (C) Mean ± SEM benzyl acetone emission during 2 h trapping intervals for 12 h starting at sunset. Samples were trapped using PDMS and measured with a TDU-GC-MS. (D) Nicotine in the floral nectar of the three different plant populations. (E) Boxplot depicting corolla limb diameter. Error bars of the boxplots indicates 1.5 times the interquartile range, boxes depict 1st and 3rd quartiles and dots show individual measurements. GLM with a polynomial distribution on (C), Kruskal–Wallis test, followed by Holm corrected LSD test in (D,E).
Discussion
Most pollinating insects visit flowers to satisfy their caloric needs or those of their offspring. However, the foraging efforts these pollinators allocate to a given flower is often not only influenced by the direct caloric value of the floral nectar, but also by the visual and chemosensory cues provided by the flower (Borghi et al., 2017). Testing the importance of these flower signals for a certain pollinator is therefore crucial for our understanding of how variation in these floral traits potentially drives the diversity of plant-pollinator interactions found today. In this study, we analyzed the floral characteristics of three different natural populations of the wild tobacco N. attenuata. We found that although all three populations provided nectar of similar caloric value, marked differences in the amount of floral volatiles as well as minor differences in nectar sugar ratios correlated with significant changes in the visitation rate of the hawkmoth M. sexta.
What makes an insect pollinator visit and re-visit a flower? At first an insect will recognize the flower odor and its visual display and for hawkmoth pollinated flowers both have been shown to influence the feeding decision of the moth (Raguso and Willis, 2005; Klahre et al., 2011). In concurrence with these findings, both the emissions of the main flower odor, benzyl acetone, as well as the visual display varied among the three N. attenuata populations tested in this study. Interestingly though, the Utah population, which emitted the highest amount of benzyl acetone, had the smallest corolla size (Figures 4A,C). Given that the hawkmoths invested significantly more foraging time into flowers from the Utah population and also had the highest foraging success at these flowers, differences in the odor emissions appear to be of greater importance for this particular hawkmoth-flower interaction than the visual display. However, the hawkmoths initially approached all three populations with a similar probability (Figure 2A), indicating that for the first approach to the flower the visual display of the corolla and the remaining volatiles could substitute for the lower benzyl acetone emissions in the California and the Arizona populations. These results are consistent with our previous findings that the main flower odor of N. attenuata, benzyl acetone, greatly enhances the foraging motivation of M. sexta for these flowers, while the visual display and other chemical cues were sufficient to initially attract the moth to a flower (Haverkamp et al., 2016b). Benzyl acetone is detected by the moth antenna, but also by specific olfactory neurons at the tip of the moth proboscis (Haverkamp et al., 2016b). Due to the length of the moth proboscis, the antenna is, for most part of the foraging, at a distance from the flower, at which the floral volatiles already become intermixed with background odors (Riffell et al., 2014). In such complex environments, the neurons on the tip of the proboscis might then allow the moth to still resolve the benzyl acetone signal from different flowers with a sufficient sensitivity to discriminate between high and low emitting plants. Hence, the signal from these receptors might to some extent explain the differences in the foraging success of M. sexta on the different plant populations tested in this study.
Given the apparent importance of benzyl acetone for the interaction of N. attenuata flowers and their hawkmoth pollinators, the low benzyl acetone emissions from the flowers of the California population are surprising. However, attracting hawkmoths for pollination might also result in potential trade-offs for a plant, as female lepidopterans might also use flower signals as oviposit cues (Adler and Bronstein, 2004; Kessler et al., 2010; Lucas-Barbosa et al., 2014; Zhou et al., 2017) and benzyl acetone has indeed been shown to increase oviposition by M. sexta on N. attenuata (Kessler et al., 2015). It is therefore conceivable that in a population with a particularly high herbivore pressure, plants with low benzyl acetone emissions are at an advantage compared to strongly emitting plants (Kessler et al., 2010). In addition, it could be hypothesized that in populations without a pollinator attracted by benzyl acetone, plants which do not bear the metabolic costs of producing this compound would have an advantage over plants emitting benzyl acetone.
After having obtained the nectar from a flower, the decision whether to visit another flower of the same type should be based on the net caloric gain obtained by the insect (Heinrich, 1979; Schmid-Hempel, 1987). This gain depends on the quantity and the quality of the floral nectar, as well as on the ability of the pollinator to extract the nectar from the flower (Heinrich and Raven, 1973; Haverkamp et al., 2016a). In plants however, the amount and the concentration of nectar is often inversely correlated and pollinators might be faced with a choice between flowers offering a higher amount of diluted nectar and others offering less but more concentrated nectar (Kaczorowski et al., 2005). Here, hawkmoths re-visited the flowers from the population with slightly higher nectar concentration more often than flowers from those populations, which had a significantly larger amount of more diluted nectar (Figures 2D, 3A,B). Hence, apart from the benzyl acetone emission, nectar concentration, but not nectar volume, might be an important factor, which contributes to the moths' visitation rates. Potentially, these differences in the behavior of the moth might be due to a stronger perception of a change in sugar concentration than of an increased nectar volume (Nachev et al., 2017). Food volume in insects is assumed to be mainly detected through stretch receptors attached to the gut (Dethier, 1976; Olds and Xu, 2014). In M. sexta, the crop can hold several 100 mg and stretch receptors might therefore not be sufficiently sensitive to detect a loading difference of only 2.8 mg that we found in this study. In contrast, sugar concentration in the nectar is detected by gustatory neurons at the tip of the moth proboscis (Reiter et al., 2015), which at least in lepidopteran caterpillars have been shown to detect differences in sugar concentrations of about 0.1 mM (Schoonhoven and Van Loon, 2002) and might therefore also be able to detect the differences of 0.3 mM in the total sugar concentration found in this study. One could therefore speculate that flowers pollinated by lepidopterans should rather increase their nectar concentration than their total nectar amount up to the point at which the nectar viscosity might become too high for efficient uptake by the proboscis (Kim et al., 2011).
The perception of the nectar quality by the pollinator however, does not only depend on the concentration of the different nectar sugars, but also on their type. Most Lepidoptera, which have been investigated so far, behaviorally responded to sucrose and fructose, with a preference for sucrose, but were almost non-responsive to glucose (Romeis and Wäckers, 2000; Kelber, 2003). Given these preferences, the low amount of sucrose in the nectar of all three N. attenuata populations is surprising, especially as the ratio of nectar sugars does not appear to be under strong phylogenetic constraints (Kaczorowski et al., 2005). However, fructose, which is the second most preferred sugar by hawkmoths, but least acceptable to other pollinators like hummingbirds and bees (Wykes, 1952; Martineez del Rio et al., 1992), was the most common sugar in all three populations (Figure 3D). Hence, the low amount of sucrose might even represent an adaptation against hummingbirds and bees, which commonly visit N. attenuata plants, but have been argued to deliver a lower inter-plant pollination service than hawkmoths as birds and bees transfer pollen over shorter distances or, in case of the bees, even rob the nectar without pollinating the flower (Kessler et al., 2008, 2010). Additionally, the sugar ratios in the nectar of N. attenuata might also be influenced by the high level of plant defenses present in the flower. These defenses are mainly controlled by the phytohormone jasmonate, which has also been found to influence the sugar ratios in the flower tissue (Stitz et al., 2014; Li et al., 2017).
Independent of the mechanism by which the nectar is detected by the hawkmoth, this evaluation should result in the moth learning to visit other flowers of the same type or to avoid these flowers on future foraging trails. It is thus interesting that the moths repeatedly re-visited flowers, which they had emptied before, suggesting that several unsuccessful foraging trails might be necessary for a moth to overwrite its innate foraging preference for certain flowers (Brandenburg et al., 2012; Riffell et al., 2013).
In the case of the secondary metabolite nicotine, we found similar concentrations in the nectar of all three populations tested in this study, with a high variability within each population (Figure 4B). This result is consistent with previous findings, which showed a high variability of nectar nicotine concentrations even within a single plant and it was suggested that this variability increased outcrossing rates presumably by promoting inter-plant movement and by increasing the visitation rate of pollinators to N. attenuata (Kessler et al., 2012). However, the finding that nectar nicotine levels were similar, suggests that nectar nicotine is under different selection pressures than the main flower volatile, benzyl acetone, such as those exerted by florivores, nectar thieves or microbes (Li et al., 2017).
Chemical signals are particularly suited for rapid and local adaptions to certain pollinators as they are often influenced by relatively small genetic changes (Amrad et al., 2016). Unfortunately, the full biosynthetic pathway producing benzyl acetone, the major flower compound in N. attenuata is still unknown. However, for (E)-α-bergamotene, a scent compound which accumulates inside the corolla tube of N. attenuata, the underlying genetic mechanism has recently been identified (Zhou et al., 2017). It was found that the expression of the single gene responsible for the production of (E)-α-bergamotene varied strongly among different populations of N. attenuata. Interestingly, this compound also facilitates pollination by M. sexta suggesting again that minor changes in the expression of single genes, involved in the emission of floral scent, can lead to rapid adaptations of local plant populations to different insect pollinators.
The present study demonstrates how variation in the chemical traits, but not in the actual floral rewards, of three flower populations, which grow less than 500 km apart, correlate with significant differences in the response of an important pollinator to these flowers. Taken together these results highlight the importance of unraveling the chemical signaling between pollinators and flowers, which might lead to possible isolation barriers between plant populations. Unraveling the communication between plants and pollinators appears thus as a crucial step if we are to understand the diversification and co-evolution of both plants and insects.
Author Contributions
All authors contributed to the experimental design of the study. AH and FY performed most experiments, analyzed their results, and wrote the first draft of the manuscript and all authors contributed to the revision.
Funding
Funding was provided by the Max-Planck Society and the European Research Council advanced grant no. 293926 to IB.
Conflict of Interest Statement
The authors declare that the research was conducted in the absence of any commercial or financial relationships that could be construed as a potential conflict of interest.
Acknowledgments
The authors are particularly grateful to Rayko Halitschke, Martin Schäfer, and Ke Pu for their help with the nectar nicotine analysis. Furthermore, we would like to thank Sylke Dietel for her support in maintaining the insect colony and the Glasshouse team of the MPICE for maintaining the different plant lines.
References
Adler, L. S., and Bronstein, J. L. (2004). Attracting antagonists: does floral nectar increase leaf herbivory? Ecology 85, 1519–1526. doi: 10.1890/03-0409
Amrad, A., Moser, M., Mandel, T., de Vries, M., Schuuink, R. C., Freitas, L., et al. (2016). Gain and loss of floral scent production through changes in structural genes during pollinator- mediated speciatio. Curr. Biol. 26, 3303–3312. doi: 10.1016/j.cub.2016.10.023
Baracchi, D., Marples, A., Jenkins, A. J., Leitch, A. R., and Chittka, L. (2017). Nicotine in floral nectar pharmacologically influences bumblebee learning of floral features. Sci. Rep. 7:1951. doi: 10.1038/s41598-017-01980-1
Borghi, M., Fernie, A. R., Schiestl, F. P., and Bouwmeester, H. J. (2017). The sexual advantage of looking, smelling, and tasting Good : The metabolic network that produces signals for pollinators. Trends Plant Sci. 22, 338–350. doi: 10.1016/j.tplants.2016.12.009
Brandenburg, A., Kuhlemeier, C., and Bshary, R. (2012). Innate adjustment of visitation behavior to rewarding and reward-minimized Petunia axillaris (Solanacea) plants by hawkmoth Manduca sexta (Sphingidae). Ethology 118, 654–661. doi: 10.1111/j.1439-0310.2012.02055.x
Byers, K. J. R. P., Vela, J. P., Peng, F., Riffell, J. A., and Bradshaw, H. D. (2014). Floral volatile alleles can contribute to pollinator-mediated reproductive isolation in monkeyflowers (Mimulus). Plant J. 80, 1031–1042. doi: 10.1111/tpj.12702
Delle-Vedove, R., Schatz, B., and Dufay, M. (2017). Understanding intraspecific variation of floral scent in light of evolutionary ecology. Ann. Bot. 120, 1–20. doi: 10.1093/aob/mcx055
Egan, P. A., Stevenson, P. C., Tiedeken, E. J., Wright, G. A., Boylan, F., and Stout, J. C. (2016). Plant toxin levels in nectar vary spatially across native and introduced populations. J. Ecol. 104, 1106–1115. doi: 10.1111/1365-2745.12573
Haverkamp, A., Bing, J., Badeke, E., Hansson, B. S., and Knaden, M. (2016a). Innate olfactory preferences for flowers matching proboscis length ensure optimal energy gain in a hawkmoth. Nat. Commun. 7:11644. doi: 10.1038/ncomms11644
Haverkamp, A., Yon, F., Keesey, I. W., Mißbach, C., Koenig, C., Hansson, B. S., et al. (2016b). Hawkmoths evaluate scenting flowers with the tip of their proboscis. Elife 5:e15039. doi: 10.7554/eLife.15039
Heinrich, B., and Raven, P. H. (1973). Energetics and pollination ecology. Science 176, 597–602. doi: 10.1126/science.176.4035.597
Johnson, S. D., Hargreaves, A. L., and Brown, M. (2006). Dark, bitter-tasting nectar functions as a filter of flower visitors in a bird-pollinated plant. Ecology 87, 2709–2716. doi: 10.1890/0012-9658(2006)87[2709:DBNFAA]2.0.CO;2
Kaczorowski, R. L., Gardener, M. C., and Holtsford, T. P. (2005). Nectar traits in Nicotiana section Alatae (Solanaceae) in relation to floral traits, pollinators, and mating system. Am. J. Bot. 92, 1270–1283. doi: 10.3732/ajb.92.8.1270
Kallenbach, M., Oh, Y., Eilers, E. J., Veit, D., Baldwin, I. T., and Schuman, M. C. (2014). A robust, simple, high-throughput technique for time-resolved plant volatile analysis in field experiments. Plant J. 78, 1060–1072. doi: 10.1111/tpj.12523
Kelber, A. (2003). Sugar preferences and feeding strategies in the hawkmoth Macroglossum stellatarum. J. Comp. Physiol. A Neuroethol. Sensory Neural Behav. Physiol. 189, 661–666. doi: 10.1007/s00359-003-0440-0
Kessler, D. (2012). Context dependency of nectar reward-guided oviposition. Entomol. Exp. Appl. 144, 112–122. doi: 10.1111/j.1570-7458.2012.01270.x
Kessler, D., and Baldwin, I. T. (2007). Making sense of nectar scents: the effects of nectar secondary metabolites on floral visitors of Nicotiana attenuata. Plant J. 49, 840–54. doi: 10.1111/j.1365-313X.2006.02995.x
Kessler, D., Bhattacharya, S., Diezel, C., Rothe, E., Gase, K., Schöttner, M., et al. (2012). Unpredictability of nectar nicotine promotes outcrossing by hummingbirds in Nicotiana attenuata. Plant J. 71, 529–538. doi: 10.1111/j.1365-313X.2012.05008.x
Kessler, D., Diezel, C., and Baldwin, I. T. (2010). Changing pollinators as a means of escaping herbivores. Curr. Biol. 20, 237–242. doi: 10.1016/j.cub.2009.11.071
Kessler, D., Gase, K., and Baldwin, I. T. (2008). Field experiments with transformed plants reveal the sense of floral scents. Science 321, 1200–1202. doi: 10.1126/science.1160072
Kessler, D., Kallenbach, M., Diezel, C., Rothe, E., Murdock, M., and Baldwin, I. T. (2015). How scent and nectar influence floral antagonists and mutualists. Elife 4:e07641. doi: 10.7554/eLife.07641
Kim, W., Gilet, T., and Bush, J. W. (2011). Optimal concentrations in nectar feeding. Proc. Natl. Acad. Sci. U.S.A. 108, 16618–16621. doi: 10.1073/pnas.1108642108
Klahre, U., Gurba, A., Hermann, K., Saxenhofer, M., Bossolini, E., Guerin, P. M., et al. (2011). Pollinator choice in Petunia depends on two major genetic loci for floral scent production. Curr. Biol. 21, 730–739. doi: 10.1016/j.cub.2011.03.059
Knauer, A. C., and Schiestl, F. P. (2015). Bees use honest floral signals as indicators of reward when visiting flowers. Ecol. Lett. 18, 135–143. doi: 10.1111/ele.12386
Koenig, C., Hirsh, A., Bucks, S., Klinner, C., Vogel, H., Shukla, A., et al. (2015). A reference gene set for chemosensory receptor genes of Manduca sexta. Insect Biochem. Mol. Biol. 66, 51–63. doi: 10.1016/j.ibmb.2015.09.007
Krügel, T., Lim, M., Gase, K., Halitschke, R., and Baldwin, I. T. (2002). Agrobacterium-mediated transformation of Nicotiana attenuata, a model ecological expression system. Chemoecology 12, 177–183. doi: 10.1007/PL00012666
Li, R., Wang, M., Wang, Y., Schuman, M. C., Weinhold, A., Schäfer, M., et al. (2017). Flower-specific jasmonate signaling regulates constitutive floral defenses in wild tobacco. Proc. Natl. Acad. Sci. U.S.A. 114, E7205–E7214. doi: 10.1073/pnas.1703463114
Lucas-Barbosa, D., Poelman, E. H., Aartsma, Y., Snoeren, T. A., van Loon, J. J., and Dicke, M. (2014). Caught between parasitoids and predators - survival of a specialist herbivore on leaves and flowers of mustard plants. J. Chem. Ecol. 40, 621–631. doi: 10.1007/s10886-014-0454-9
Martineez del Rio, C., Baker, H. G., and Baker, I. (1992). Ecological and evolutionary implications of digestive processes: bird preferences and the sugar constituents of floral nectar and fruit pulp. Experientia 48, 544–551. doi: 10.1007/BF01920237
Nachev, V., Stich, K. P., Winter, C., Bond, A., Kamil, A., and Winter, Y. (2017). Cognition-mediated evolution of low-quality floral nectars. Science 355, 1–5. doi: 10.1126/science.aah4219
Oksanen, J., Blanchet, F., Kindt, R., Legendre, P., and O'Hara, R. (2016). Vegan: Community Ecology Package. R Package. 2.3-3. doi: 10.4135/9781412971874.n145. Available online at: https://CRAN.R-project.org/package=vegan.
Olds, W. H., and Xu, T. (2014). Regulation of food intake by mechanosensory ion channels in enteric neurons. Elife 3:e04402. doi: 10.7554/eLife.04402
Raguso, R. A., and Willis, M. A. (2005). Synergy between visual and olfactory cues in nectar feeding by wild hawkmoths, Manduca sexta. Anim. Behav. 69, 407–418. doi: 10.1016/j.anbehav.2004.04.015
Reiter, S., Campillo Rodriguez, C., Sun, K., and Stopfer, M. (2015). Spatiotemporal coding of individual chemicals by the gustatory system. J. Neurosci. 35, 12309–12321. doi: 10.1523/JNEUROSCI.3802-14.2015
Riffell, J. A., Abrell, L., and Hildebrand, J. G. (2008). Physical processes and real-time chemical measurement of the insect olfactory environment. J. Chem. Ecol. 34, 837–853. doi: 10.1007/s10886-008-9490-7
Riffell, J. A., Lei, H., Abrell, L., and Hildebrand, J. G. (2013). Neural basis of a pollinator's buffet: olfactory specialization and learning in Manduca sexta. Science 339, 200–204.
Riffell, J. A., Shlizerman, E., Sanders, E., Abrell, L., Medina, B., Hinterwirth, A. J., et al. (2014). Flower discrimination by pollinators in a dynamic chemical environment. Science 344, 1515–1518. doi: 10.1126/science.1251041
Romeis, J., and Wäckers, F. L. (2000). Feeding responses by female Pieris brassicae butterflies to carbohydrates and amino acids. Physiol. Entomol. 25, 247–253. doi: 10.1046/j.1365-3032.2000.00188.x
Schäfer, M., Brütting, C., Baldwin, I. T., and Kallenbach, M. (2016). High-throughput quantification of more than 100 primary- and secondary-metabolites, and phytohormones by a single solid-phase extraction based sample preparation with analysis by UHPLC–HESI–MS/MS. Plant Methods 12:30. doi: 10.1186/s13007-016-0130-x
Schemske, D. W., and Bradshaw, H. D. (1999). Pollinator preference and the evolution of floral traits in monkeyflowers (Mimulus). Proc. Natl. Acad. Sci. U.S.A. 96, 11910–11915. doi: 10.1073/pnas.96.21.11910
Schiestl, F. P., and Johnson, S. D. (2013). Pollinator-mediated evolution of floral signals. Trends Ecol. Evol. 28, 307–315. doi: 10.1016/j.tree.2013.01.019
Schmid-Hempel, P. (1987). Efficient nectar-collecting by honeybees I. Economic models. J. Anim. Ecol. 56, 209–218. doi: 10.2307/4810
Schoonhoven, L. M., and Van Loon, J. J. A. (2002). An inventory of taste in caterpillars: each species its own key. Acta Zool. Acad. Sci. Hungaricae 48, 215–263.
Smith, C. A., Want, E. J., O'Maille, G., Abagyan, R., and Siuzdak, G. (2006). XCMS: processing mass spectrometry data for metabolite profiling using nonlinear peak alignment, matching, and identification. Anal. Chem. 78, 779–787. doi: 10.1021/ac051437y
Stitz, M., Hartl, M., Baldwin, I. T., and Gaquerel, E. (2014). Jasmonoyl-l-Isoleucine coordinates metabolic networks required for anthesis and floral attractant emission in wild tobacco (Nicotiana attenuata). Plant Cell 26, 3964–3983. doi: 10.1105/tpc.114.128165
Vet, L. E. M., and Dicke, M. (1992). Ecology of infochemical use by natural enemies in a tritrophic context. Annu. Rev. Entomol. 37, 141–172. doi: 10.1146/annurev.en.37.010192.001041
von Arx, M., Goyret, J., Davidowitz, G., and Raguso, R. A. (2012). Floral humidity as a reliable sensory cue for profitability assessment by nectar-foraging hawkmoths. Proc. Natl. Acad. Sci. U.S.A. 109, 9471–9476. doi: 10.1073/pnas.1121624109
Waser, N. M., Chittka, L., Price, M. V., Williams, N. M., and Ollerton, J. (1996). Generalization in pollination systems, and why it matters? Ecol. Entomol. 77, 1043–1060. doi: 10.2307/2265575
Wright, G. A., Baker, D. D., Palmer, M. J., Stabler, D., Mustard, J. A., Power, E. F., et al. (2013). Caffeine in floral nectar enhances a pollinator's memory of reward. Science 339, 1202–1204. doi: 10.1126/science.1228806
Wykes, G. R. (1952). The preferences of honeybees for solutions of various sugars which occur in nectar. J. Exp. Biol. 29, 511–519.
Yapici, N., Cohn, R., Schusterreiter, C., Ruta, V., and Vosshall, L. B. (2016). A taste circuit that regulates ingestion by integrating food and hunger signals. Cell 165, 715–729. doi: 10.1016/j.cell.2016.02.061
Keywords: pollination, chemosensation, nectar, flower volatiles, plant populations, Manduca sexta, Nicotiana attenuata
Citation: Haverkamp A, Hansson BS, Baldwin IT, Knaden M and Yon F (2018) Floral Trait Variations Among Wild Tobacco Populations Influence the Foraging Behavior of Hawkmoth Pollinators. Front. Ecol. Evol. 6:19. doi: 10.3389/fevo.2018.00019
Received: 26 September 2017; Accepted: 14 February 2018;
Published: 28 February 2018.
Edited by:
Stefano Colazza, Università degli Studi di Palermo, ItalyReviewed by:
Stefan Dötterl, University of Salzburg, AustriaSalvatore Cozzolino, University of Naples Federico II, Italy
Copyright © 2018 Haverkamp, Hansson, Baldwin, Knaden and Yon. This is an open-access article distributed under the terms of the Creative Commons Attribution License (CC BY). The use, distribution or reproduction in other forums is permitted, provided the original author(s) and the copyright owner are credited and that the original publication in this journal is cited, in accordance with accepted academic practice. No use, distribution or reproduction is permitted which does not comply with these terms.
*Correspondence: Markus Knaden, bWtuYWRlbkBpY2UubXBnLmRl
Felipe Yon, ZnlvbkBpY2UubXBnLmRl
†Senior authors.