- 1Department of Biology and Geosciences, Graduate School of Science, Osaka City University, Osaka, Japan
- 2Japan Science and Technology Agency, Precursory Research for Embryonic Science and Technology, Kawaguchi, Japan
Opsins are light-sensor proteins, each absorbing a specific wavelength of light. This, in turn, drives a specific G protein-mediated phototransduction cascade, leading to a photoreceptor cell response. Recent genome projects have revealed an unexpectedly large number of opsin genes for vision and non-visual photoreception in various animals. However, the significance of this multiplicity of opsins remains largely unknown, except for that of cone visual opsins, which are diversified with respect to spectral sensitivity to achieve vertebrate color vision. Here, an implication of multiplicity is discussed, with focus on an ultraviolet-sensitive non-visual opsin—parapinopsin—that underlies pineal wavelength discrimination in lower vertebrates. Parapinopsins are phylogenetically close to vertebrate visual opsins, which have bleaching properties, but interestingly, parapinopsins are bleach-resistant bistable pigments, which photo-convert to stable photoproducts that revert to their original dark state by subsequent light absorption, similar to invertebrate rhodopsins. The unique characteristics of parapinopsin as an evolutionary intermediate between bistable and bleaching pigments provide insight into the evolutionary transition between signaling molecules that interact with two types of opsin-based pigments. Furthermore, the parapinopsin gene was duplicated in the teleost lineage and the spectral sensitivities of the duplicated parapinopsins were different from each other. On the basis of these results, together with the histochemical findings of parapinopsin, a plausible link between the diversification of a non-visual opsin parapinopsin and diverse pineal functions, wavelength discrimination, and melatonin secretion, implying why multiple opsins exist in animals, is proposed.
Introduction
Many animals utilize light information for vision and non-visual functions such as circadian photoentrainment. In animal photoreception, opsin-based pigments, which are composed of a protein moiety opsin and chromophore retinal, serve as light sensors to capture light and initiate G protein-mediated phototransduction cascades in photoreceptor cells. Thousands of opsin genes have been identified in various animals and are phylogenetically and functionally classified into eight groups that diversified during early eumetazoan evolution (Terakita, 2005; Peirson et al., 2009; Yau and Hardie, 2009; Lamb, 2013; Koyanagi and Terakita, 2014). In addition, recent genome projects have revealed an unexpectedly large number of opsin genes in various animals, indicating that most animals have multiple opsins (Davies et al., 2015). In visual systems, the biological significance of this diversification is clear; multiple types of visual opsins with different spectral sensitivities are responsible for color vision and other visual functions (Nathans et al., 1986; Okano et al., 1992; Nagata et al., 2012; Terakita and Nagata, 2014; see also Figure 3A). However, the significance of the diversification of non-visual opsins remains unclear, despite the importance of understanding the molecular mechanism and evolution of various non-visual photoreceptions. General characteristics and diversities of the opsin family have been previously reviewed (Terakita, 2005; Peirson et al., 2009; Yau and Hardie, 2009; Lamb, 2013; Koyanagi and Terakita, 2014; Davies et al., 2015); therefore, here we addressed this issue based on the molecular properties of opsins by focusing on a pineal photopigment, parapinopsin.
Parapinopsin, a Bistable Ultraviolet-Sensitive Pigment for the Pineal Wavelength Discrimination in Lower Vertebrates
Lower vertebrates can discriminate wavelengths of light with pineals and related organs independently of image-forming color vision in the eyes (Dodt and Meissl, 1982). This so-called pineal “color discrimination” is achieved through antagonism primarily between ultraviolet (UV) and visible light reception, which cause inhibitory and excitatory effects, respectively, on neuronal firing of a specialized type of ganglion cell. Detection of the ratio of UV to visible light in environmental light has been observed in pineal organs of lamprey (Morita and Dodt, 1973) and some teleosts (Morita, 1966; Falcon and Meissl, 1981), as well as in pineal-related organs of frogs (frontal organ; Dodt and Heerd, 1962; Korf et al., 1981) and reptiles (parietal eye; Jenison and Nolte, 1980). We demonstrated that parapinopsin, which was first identified in catfish pineal and parapineal organs (Blackshaw and Snyder, 1997), is the UV-sensitive pigment underlying pineal UV reception in lamprey, through spectroscopic, immunohistochemical, and electrophysiological analyses (Koyanagi et al., 2004). Parapinopsins were also identified from rainbow trout and clawed frog, in which the pineal and related organs were reported to exhibit antagonistic chromatic responses to UV and visible light (Morita, 1966; Korf et al., 1981). In addition, parapinopsin expression was recently reported in the pineal organ of a closely related species of rainbow trout (Nakane et al., 2013). Thus, parapinopsin is considered to be a common molecular basis for UV reception in pineal wavelength discrimination. This idea is supported by the observation that parapinopsin is expressed in photoreceptor cells of the iguana parietal eye, where the ratio of UV to visible light is detected (Wada et al., 2012).
Pineal UV-sensitive opsins, parapinopsins are phylogenetically classified into the same group as vertebrate visual opsins such as rhodopsins and cone visual opsins. It is well known that vertebrate visual opsins are bleaching pigments; the photoproduct releases its retinal chromophore over time and bleaches. Interestingly, although parapinopsins are closely related to vertebrate visual opsins, parapinopsins are bistable pigments, which is common for invertebrate visual opsins; the photoproduct is stable and reverts to the original dark state by subsequent light absorption (Koyanagi et al., 2004). Spectroscopic analysis of the photoproduct properties of varied opsins revealed that the bistable property is common in animal opsins and the bleaching property was acquired during the course of vertebrate visual opsin evolution (Terakita et al., 2004). Accordingly, parapinopsins could be an evolutionary intermediate between bistable pigment and bleaching pigment, providing an opportunity to study the detailed aspects of the evolution of vertebrate visual signaling (Figure 1A).
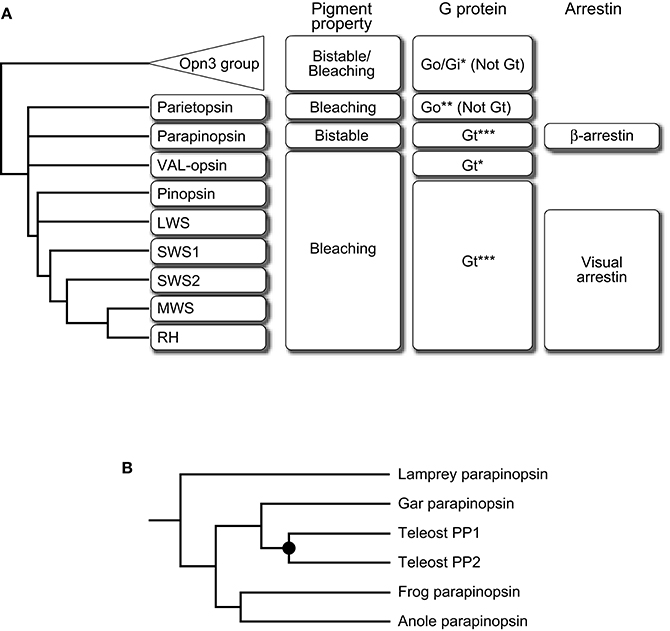
Figure 1. Summary of the transition of pigment property and signal transduction molecules along with evolution of the vertebrate visual system. (A) A schematic drawing of the phylogenetic relationship of subgroups of the Gt-coupled group with Opn3 group as an outgroup is shown on the left side. Pigment property and molecular species of G protein alpha subunit and arrestin are indicated at the right side of each subgroup. Note that vertebrate Opn3 did not exhibit an apparent bistable nature (Sugihara et al., 2016). This summary suggests the direction of their transition, from bistable to bleaching, from Go to Gt, and from β-arrestin to visual arrestin. G protein couplings were determined by G protein activation of opsin-based pigment in vitro (*), opsin-G protein colocalization (**), or both (***). (B) A schematic drawing of the detailed phylogeny in the parapinopsin subgroup. Parapinopsins are diversified in the teleost lineage via teleost-specific whole-genome duplication (filled circle) (Koyanagi et al., 2015). See also Davies et al. (2015) for further information on other non-visual opsins.
Transition of Signaling Molecules that Interact With Opsins
In general, opsin-based pigments activate G proteins upon light absorption to initiate phototransduction cascades and the G protein-mediated signalings are terminated by binding of arrestins. Therefore, it is of interest to compare signaling molecules that couple to visual and non-visual opsins to understand the evolution of visual signaling. In vertebrate visual cells, light-absorbed visual opsin-based pigment interacts with a G protein and an arrestin that are specialized for light sensing, called visual G protein transducin (Gt) and visual arrestin, which starts and shuts off G protein-mediated visual signaling, respectively (Lamb, 2013; Figure 1A). However, a Gt-like visual G protein or visual arrestin has not yet been identified in the genomes of invertebrates, including ascidians (Nakagawa et al., 2002; Yoshida et al., 2002), which are among the invertebrates most closely related to vertebrates. These facts suggest that opsin-Gt and opsin-visual arrestin interactions were established during the course of vertebrate visual opsin evolution but questions remain regarding the detailed timing of the establishment.
In the molecular phylogenetic tree of the opsin family, one group (Figure 1A) is formed by vertebrate visual opsins composed of rhodopsins (RH) and four types of cone opsins, namely: middle-wavelength-sensitive (MWS) opsins, short-wavelength-sensitive 1 (SWS1) opsins, short-wavelength-sensitive 2 (SWS2) opsins, and long-wavelength-sensitive (LWS) opsins, along with several non-visual opsins, pinopsins (P), VAL-opsins (VAL), parapinopsins (PP), and parietopsins (PT). The group is generally referred to as the Gt-coupled opsin group because not only visual opsins (Lamb et al., 2016) but also pinopsins (Nakamura et al., 1999; Matsushita et al., 2000; Su et al., 2006) couple with Gt, and frog VAL-opsin has been reported to activate Gt in vitro (Sato et al., 2011). In contrast, parietopsin has been reported to couple with Go type G protein in the lizard parietal eye (Su et al., 2006). In addition, it is known that mosquito Opn3 and pufferfish TMT opsin in the Opn3 group, which is the closest group to the Gt-coupled opsin group, activate Gi and Go type G proteins but not Gt in vitro (Koyanagi et al., 2013; Figure 1A). These observations, together with the phylogeny that the parietopsin subgroup underwent relatively earlier branching in the Gt-coupled opsin group, suggest that the ancestral opsin in this group was not coupled to Gt type G protein (Gt-“uncoupled” opsin; Figure 1A). We recently investigated whether parapinopsins couple with Gt by focusing on pufferfish, zebrafish, and lamprey pineal organs. Biochemical analyses demonstrated that the lamprey and teleost parapinopsins activated Gt in vitro in a light-dependent manner, similar to vertebrate visual opsins (Kawano-Yamashita et al., 2015). In the teleosts, which possess rod and cone types of Gt, Gt1, and Gt2 (Lamb et al., 2016), parapinopsin was colocalized with Gt2. In contrast, in the lamprey, which does not possess Gt2 gene (Muradov et al., 2008), parapinopsin was colocalized with Gt1 type transducin, GtS, suggesting that lamprey parapinopsin couples with GtS instead with Gt2 (Kawano-Yamashita et al., 2015). Although there is a small difference in the Gt species, the results showed that parapinopsins couple with Gt in lamprey and teleost pineal organs. Taken together, opsin-Gt coupling could have been established before the split between parapinopsins and other opsins, including visual opsins (Figure 1A). Thus, the emergence of opsin-Gt coupling predates that of the bleaching pigment, suggesting no apparent correlation between the two events and rather, biological significance of the coupling of bistable pigment to Gt. A possible explanation for the significance is that Gt-mediated signal transduction is required for light-dependent hyperpolarization of photoreceptor cells because parapinopsin-expressing photoreceptor cells in the lamprey pineal organ exhibited hyperpolarization responses (Koyanagi et al., 2004) similar to the bleaching pigment-containing visual and non-visual cells in vertebrates. Interestingly, Gt activation by parapinopsin is provoked and terminated by UV and subsequent orange light irradiation, respectively, due to the bistable nature of parapinopsin (Kawano-Yamashita et al., 2015). A possible contribution of this behavior in pineal wavelength discrimination via Gt-mediated hyperpolarization of photoreceptor cells may provide clues to understanding why a bistable pigment acquired the ability to couple with Gt.
Investigation of arrestin as a negative regulator of parapinopsin-Gt interaction also provided functional and evolutionary clues. In the lamprey pineal organ, the fact that cells expressing parapinopsin and rhodopsin are localized in the dorsal and ventral regions of the pineal organ, respectively (Koyanagi et al., 2004), enabled a comparison of the arrestins that bind to parapinopsin and rhodopsin in the same organ. Surprisingly, ß-arrestin, which is known to bind to activated G protein-coupled receptors (GPCRs) other than opsin-based pigments (Lohse et al., 1990), was localized to parapinopsin-expressing cells (Kawano-Yamashita et al., 2011). The result contrasts the localization of visual arrestin in rhodopsin-expressing cells. In addition, the light-dependent translocation of ß-arrestin to cell membranes in parapinopsin-expressing cultured cells and to the outer segments of pineal parapinopsin-expressing photoreceptor cells was observed, suggesting that ß-arrestin binds to parapinopsin to arrest parapinopsin signaling (Kawano-Yamashita et al., 2011; Figure 1A). Interestingly, under light illumination, the granules containing parapinopsin and ß-arrestin were observed in parapinopsin-expressing cell bodies (Kawano-Yamashita et al., 2011). Because it is well known that ß-arrestin mediates clathrin-mediated GPCR internalization (Lohse et al., 1990; Ferguson et al., 1996; Goodman et al., 1996), the results suggest that the granules were generated in a light-dependent manner by ß-arrestin-mediated internalization of parapinopsins from the outer segments. The ß-arrestin-mediated internalization of parapinopsin enables to eliminate the stable photoproduct and restoring cell conditions to the original dark state. In contrast, vertebrate visual signaling does not require the elimination of photoproducts via internalization because vertebrate visual pigments convert to unstable photoproducts that spontaneously decay by chromophore dissociation. Taken together with the evolutionary scenario that the bleaching pigment evolved from a bistable pigment, vertebrate visual arrestin may have evolved from a “ß-like” arrestin by losing its clathrin-binding domain and function as an internalization mediator after emergence of the bleaching pigment.
Biological Significance of Non-Visual Opsin Multiplicity
In the case of visual opsins, the diversification with respect to spectral sensitivity is generally involved in the evolution of color vision (e.g., the evolution of trichromatic vision in the primate lineage, Figure 3A) but biological significance of the multiplicity of opsins underlying non-visual photoreception remains unclear. The parapinopsin-involving findings also allow further discussion. We recently found another parapinopsin gene, PP2, in the zebrafish and pufferfish genomes (Koyanagi et al., 2015), in addition to orthologs of the catfish and rainbow trout parapinopsins, PP1s, which have been previously reported (Blackshaw and Snyder, 1997; Koyanagi et al., 2004). A comprehensive survey of parapinopsin genes in vertebrate genome databases revealed that the spotted gar possess only one parapinopsin gene (Figure 1B). Since the spotted gar diverged before the teleost-specific whole-genome duplication (Hoegg et al., 2004; Crow et al., 2006), this suggests that the gene duplication giving rise to PP1 and PP2 occurred via teleost-specific whole-genome duplication (Taylor et al., 2003; Kuraku and Meyer, 2009). Phylogenetic analyses of the Gt-coupled opsin group, including parapinopsins, and synteny analysis of genomic regions around parapinopsin gene(s) in teleosts, including the spotted gar, supported this hypothesis (Koyanagi et al., 2015).
The molecular properties of two teleost parapinopsins also allowed us to speculate the biological significance of diversification of the parapinopsin gene. Zebrafish and pufferfish PP1s and spotted gar parapinopsin had absorption maxima at 360–370 nm in the UV region (Koyanagi et al., 2015), indicating that they serve as UV-sensitive pigments, similar to lamprey parapinopsin (Koyanagi et al., 2004; Figure 2A). In contrast, zebrafish, pufferfish, and rainbow trout PP2s exhibited absorption maxima in the visible light region, with peaks at ~480, 460, and 460 nm, respectively, indicating that teleost PP2 is a blue light-sensitive pigment (Koyanagi et al., 2015; Figure 2B). Regardless of the absorption spectra in the dark state, all of these teleost parapinopsins were bistable pigments, like lamprey parapinopsin and unlike vertebrate visual pigments. These findings demonstrate the diversification of vertebrate bistable pigment with respect to spectral sensitivity (UV and blue sensitivity). In addition, PP1 and PP2 exhibit mutually exclusive expression in the pineal organs of zebrafish, pufferfish, and rainbow trout (Koyanagi et al., 2015). The expression profiles indicate that PP1- and PP2-expressing cells capture light independently, which enables PP2 to be involved in pineal functions apart from wavelength discrimination, which is supported by UV-sensitive parapinopsins. PP1- and PP2-expressing cells were characterized with the transgenic zebrafish in which either PP1- or PP2-expressing cells were labeled (Koyanagi et al., 2015). In the transgenic zebrafish, PP1-expressing cells basically possessed neuronal processes, which is consistent with their involvement in wavelength discrimination (Figure 2C). However, PP2-expressing cells rarely possessed neuronal processes, suggesting that PP2 is involved in non-neural responses rather than in neural responses, including wavelength discrimination (Figure 2D). Interestingly, further histochemical investigations revealed that PP2-expressing cells contain serotonin and aanat2, a key enzyme involved in melatonin synthesis from serotonin, whereas PP1-expressing cells did not contain either molecule (Figures 2E,F). These findings suggest that blue-sensitive PP2 is involved in light-regulation of melatonin secretion (Koyanagi et al., 2015).
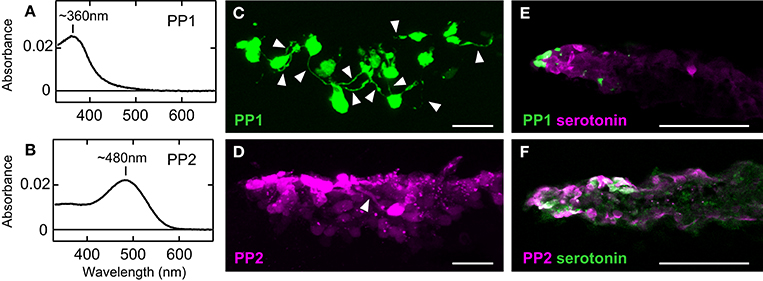
Figure 2. Functional difference between PP1 and PP2. The absorption spectra of zebrafish PP1 (A) and PP2 (B). Neuronal processes (arrowheads) were observed in many GFP-labeled PP1-expressing cells (C), but rarely in RFP-labeled PP2-expressing cells (D) in the zebrafish pineal organ. Distribution of serotonin-containing cells compared to that of PP1-expressing cells (E) and PP2-expressing cells (F) in the pineal organ of adult zebrafish. PP2-expressing cells contain serotonin, a precursor of melatonin, whereas PP1-expressing cells do not. The scale bars represent 20 μm (C,D) and 50 μm (E,F). All data are derived from Koyanagi et al. (2015).
The studies demonstrated that teleost PP1 is a UV-sensitive pigment and its pineal expression is conserved in three kinds of teleosts (Koyanagi et al., 2015), including rainbow trout, which has been shown to have pineal wavelength discrimination ability (Morita, 1966). Accordingly, these observations strongly suggest that PP1 underlies UV reception for pineal wavelength discrimination in teleosts. A series of findings including the involvement of parapinopsins in UV reception in the lamprey pineal organ and iguana parietal eye (Koyanagi et al., 2004; Wada et al., 2012), suggests that UV-sensitive parapinopsin is an elemental molecule for wavelength discrimination involving UV-reception in the pineal and related organs of non-mammalian vertebrates. In contrast, PP2 is suggested to underlie a different pineal function: light-regulated melatonin secretion. Because parapinopsin of spotted gar, which diverged before teleost-specific whole-genome duplication (Hoegg et al., 2004; Crow et al., 2006), is a UV-sensitive pigment (Koyanagi et al., 2015) as well as other vertebrate parapinopsins (Koyanagi et al., 2004; Wada et al., 2012), blue-sensitive parapinopsin likely evolved from a UV-sensitive parapinopsin (Koyanagi et al., 2015; Figure 3B). Accordingly, the findings provide a clear example of the functional differentiation among duplicated non-visual opsins; UV-sensitive PP1 could have retained the original function, and blue-sensitive PP2 acquired a novel functional role via a neofunctionalization event (Figure 3B). The diversification of non-visual opsins with respect to spectral sensitivity in the teleost lineage shows good contrast to that of visual opsins, which is involved in the evolution of color vision (Figure 3).
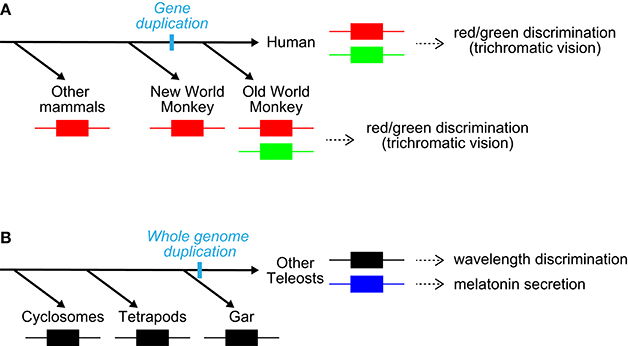
Figure 3. Diversification of visual and non-visual opsins. (A) Diversification of long-wavelength-sensitive opsin with respect to spectral sensitivity (red/green) in the primate lineage (Nathans et al., 1986; Nei et al., 1997). The diversification provided a red/green color discrimination ability. Note that these species also possess an SWS1 pigment. (B) Diversification of non-visual opsin parapinopsin with respect to spectral sensitivity (UV/blue) in the teleost lineage via teleost-specific whole-genome duplication. The diversification generated a new contribution of parapinopsin to light-regulation of melatonin secretion, in addition to wavelength discrimination.
Conclusion
A series of findings on the bistable pigment parapinopsin has improved our understanding of the evolution of both visual and non-visual opsins, and partly revealed biological significance of the multiplicity of opsins in animals. We emphasize here that parapinopsin is the first identified bistable pigment in vertebrates (Koyanagi et al., 2004). After its discovery, many bistable pigments, such as Opn4 or melanopsin, circadian photopigment in mammals, Opn3, Opn5, and peropsin have been identified in various vertebrates and invertebrates (Koyanagi et al., 2005, 2013; Mure et al., 2007; Terakita et al., 2008; Nagata et al., 2010; Yamashita et al., 2010), suggesting that the bistable property is common in animal opsins (Terakita et al., 2004). Mutational analyses of bistable pigments, including parapinopsin, revealed the displacement of the counterion, important amino acid residue for visible light absorption of opsin-based pigment, from Glu181 to Glu113 (bovine rhodopsin numbering system) during the evolution of vertebrate visual pigments (Terakita et al., 2000, 2004). Comparison of characteristics between a bleaching pigment (bovine rhodopsin) with Glu113 counterion and a bistable pigment (parapinopsin) with Glu181 counterion revealed the correlation between the G protein activation ability and the magnitude of light-induced conformational change. The results suggested that counterion displacement resulted in higher G protein activation ability, generated by larger light-induced conformational change of the pigment (Tsukamoto et al., 2009). In addition, counterion displacement could contribute to the evolution of red-sensitive pigment because displacement from Glu181 to Glu113 allows acquisition of His181, an essential amino acid residue for red-sensitivity of the pigment through chloride ion binding (Terakita et al., 2000, 2004). Despite the wide distribution of the bistable nature in animal opsins and a better understanding of the molecular properties of the bistable pigment, its biological significance remains unclear. Studies of the contributions of bistability in vivo, such as that of parapinopsin in pineal wavelength discrimination, may provide insight into the physiological importance of the bistable property.
Author Contributions
All authors made substantial and intellectual contributions to this work. MK and AT wrote the paper. All authors read and approved the final manuscript.
Conflict of Interest Statement
The authors declare that the research was conducted in the absence of any commercial or financial relationships that could be construed as a potential conflict of interest.
Acknowledgments
This work was supported by JSPS KAKENHI Grant Number 15H05777 to AT, Grant Numbers 26291070, 15K14568 to MK and Grant Number 14J40094 to EK, Human Frontier Science Program to AT, JST PRESTO Grant Number JPMJPR13A2 to MK, and JSPS Research Fellowships for Young Scientists to EK.
References
Blackshaw, S., and Snyder, S. H. (1997). Parapinopsin, a novel catfish opsin localized to the parapineal organ, defines a new gene family. J. Neurosci. 17, 8083–8092.
Crow, K. D., Stadler, P. F., Lynch, V. J., Amemiya, C., and Wagner, G. P. (2006). The “fish-specific” Hox cluster duplication is coincident with the origin of teleosts. Mol. Biol. Evol. 23, 121–136. doi: 10.1093/molbev/msj020
Davies, W. I., Tamai, T. K., Zheng, L., Fu, J. K., Rihel, J., Foster, R. G., et al. (2015). An extended family of novel vertebrate photopigments is widely expressed and displays a diversity of function. Genome Res. 25, 1666–1679. doi: 10.1101/gr.189886.115
Dodt, E., and Heerd, E. (1962). Mode of action of pineal nerve fibers in frogs. J. Neurophysiol. 25, 405–429.
Dodt, E., and Meissl, H. (1982). The pineal and parietal organs of lower vertebrates. Experientia 38, 996–1000. doi: 10.1007/BF01955342
Falcon, J., and Meissl, H. (1981). The photosensory function of the pineal organ of the pike (Esox lucius L.). Correlation between structure and function. J. Comp. Physiol. A 144, 127–137. doi: 10.1007/BF00612806
Ferguson, S. S., Downey, W. E. III., Colapietro, A. M., Barak, L. S., Menard, L., and Caron, M. G. (1996). Role of β-arrestin in mediating agonist-promoted G protein-coupled receptor internalization. Science 271, 363–366.
Goodman, O. B. Jr., Krupnick, J. G., Santini, F., Gurevich, V. V., Penn, R. B., Gagnon, A. W., et al. (1996). β-arrestin acts as a clathrin adaptor in endocytosis of the beta2-adrenergic receptor. Nature 383, 447–450.
Hoegg, S., Brinkmann, H., Taylor, J. S., and Meyer, A. (2004). Phylogenetic timing of the fish-specific genome duplication correlates with the diversification of teleost fish. J. Mol. Evol. 59, 190–203. doi: 10.1007/s00239-004-2613-z
Jenison, G., and Nolte, J. (1980). An ultraviolet-sensitive mechanism in the reptilian parietal eye. Brain Res. 194, 506–510. doi: 10.1016/0006-8993(80)91232-9
Kawano-Yamashita, E., Koyanagi, M., Shichida, Y., Oishi, T., Tamotsu, S., and Terakita, A. (2011). β-arrestin functionally regulates the non-bleaching pigment parapinopsin in lamprey pineal. PLoS ONE 6:e16402. doi: 10.1371/journal.pone.0016402
Kawano-Yamashita, E., Koyanagi, M., Wada, S., Tsukamoto, H., Nagata, T., and Terakita, A. (2015). Activation of transducin by bistable pigment parapinopsin in the pineal organ of lower vertebrates. PLoS ONE 10:e0141280. doi: 10.1371/journal.pone.0141280
Korf, H. W., Liesner, R., Meissl, H., and Kirk, A. (1981). Pineal complex of the clawed toad, Xenopus laevis Daud.: structure and function. Cell Tissue Res. 216, 113–130.
Koyanagi, M., Kawano, E., Kinugawa, Y., Oishi, T., Shichida, Y., Tamotsu, S., et al. (2004). Bistable UV pigment in the lamprey pineal. Proc. Natl. Acad. Sci. U.S.A. 101, 6687–6691. doi: 10.1073/pnas.0400819101
Koyanagi, M., Kubokawa, K., Tsukamoto, H., Shichida, Y., and Terakita, A. (2005). Cephalochordate melanopsin: evolutionary linkage between invertebrate visual cells and vertebrate photosensitive retinal ganglion cells. Curr. Biol. 15, 1065–1069. doi: 10.1016/j.cub.2005.04.063
Koyanagi, M., Takada, E., Nagata, T., Tsukamoto, H., and Terakita, A. (2013). Homologs of vertebrate Opn3 potentially serve as a light sensor in nonphotoreceptive tissue. Proc. Natl. Acad. Sci. U.S.A. 110, 4998–5003. doi: 10.1073/pnas.1219416110
Koyanagi, M., and Terakita, A. (2014). Diversity of animal opsin-based pigments and their optogenetic potential. Biochim. Biophys. Acta 1837, 710–716. doi: 10.1016/j.bbabio.2013.09.003
Koyanagi, M., Wada, S., Kawano-Yamashita, E., Hara, Y., Kuraku, S., Kosaka, S., et al. (2015). Diversification of non-visual photopigment parapinopsin in spectral sensitivity for diverse pineal functions. BMC Biol. 13:73. doi: 10.1186/s12915-015-0174-9
Kuraku, S., and Meyer, A. (2009). The evolution and maintenance of Hox gene clusters in vertebrates and the teleost-specific genome duplication. Int. J. Dev. Biol. 53, 765–773. doi: 10.1387/ijdb.072533km
Lamb, T. D. (2013). Evolution of phototransduction, vertebrate photoreceptors and retina. Prog. Retin. Eye Res. 36, 52–119. doi: 10.1016/j.preteyeres.2013.06.001
Lamb, T. D., Patel, H., Chuah, A., Natoli, R. C., Davies, W. I., Hart, N. S., et al. (2016). Evolution of vertebrate phototransduction: cascade activation. Mol. Biol. Evol. 33, 2064–2087. doi: 10.1093/molbev/msw095
Lohse, M. J., Benovic, J. L., Codina, J., Caron, M. G., and Lefkowitz, R. J. (1990). β-Arrestin: a protein that regulates beta-adrenergic receptor function. Science 248, 1547–1550.
Matsushita, A., Yoshikawa, T., Okano, T., Kasahara, T., and Fukada, Y. (2000). Colocalization of pinopsin with two types of G-protein α-subunits in the chicken pineal gland. Cell Tissue Res. 299, 245–251. doi: 10.1007/s004419900145
Morita, Y. (1966). Lead pattern of the pineal neuron of the rainbow trout (Salmo irideus) by illumination of the diencephalon. Pflugers Arch. 289, 155–167. doi: 10.1007/BF00412906
Morita, Y., and Dodt, E. (1973). Slow photic responses of the isolated pineal organ of lamprey. Nova Acta Leopoldina 38, 331–339.
Muradov, H., Kerov, V., Boyd, K. K., and Artemyev, N. O. (2008). Unique transducins expressed in long and short photoreceptors of lamprey Petromyzon marinus. Vision Res. 48, 2302–2308. doi: 10.1016/j.visres.2008.07.006
Mure, L. S., Rieux, C., Hattar, S., and Cooper, H. M. (2007). Melanopsin-dependent nonvisual responses: evidence for photopigment bistability in vivo. J. Biol. Rhythms 22, 411–424. doi: 10.1177/0748730407306043
Nagata, T., Koyanagi, M., Tsukamoto, H., Saeki, S., Isono, K., Shichida, Y., et al. (2012). Depth perception from image defocus in a jumping spider. Science 335, 469–471. doi: 10.1126/science.1211667
Nagata, T., Koyanagi, M., Tsukamoto, H., and Terakita, A. (2010). Identification and characterization of a protostome homologue of peropsin from a jumping spider. J. Comp. Physiol. A Neuroethol. Sens. Neural Behav. Physiol. 196, 51–59. doi: 10.1007/s00359-009-0493-9
Nakagawa, M., Orii, H., Yoshida, N., Jojima, E., Horie, T., Yoshida, R., et al. (2002). Ascidian arrestin (Ci-arr), the origin of the visual and nonvisual arrestins of vertebrate. Eur. J. Biochem. 269, 5112–5118. doi: 10.1046/j.1432-1033.2002.03240.x
Nakamura, A., Kojima, D., Imai, H., Terakita, A., Okano, T., Shichida, Y., et al. (1999). Chimeric nature of pinopsin between rod and cone visual pigments. Biochemistry 38, 14738–14745. doi: 10.1021/bi9913496
Nakane, Y., Ikegami, K., Iigo, M., Ono, H., Takeda, K., Takahashi, D., et al. (2013). The saccus vasculosus of fish is a sensor of seasonal changes in day length. Nat. Commun. 4:2108. doi: 10.1038/ncomms3108
Nathans, J., Thomas, D., and Hogness, D. S. (1986). Molecular genetics of human color vision: the genes encoding blue, green, and red pigments. Science 232, 193–202.
Nei, M., Zhang, J., and Yokoyama, S. (1997). Color vision of ancestral organisms of higher primates. Mol. Biol. Evol. 14, 611–618. doi: 10.1093/oxfordjournals.molbev.a025800
Okano, T., Kojima, D., Fukada, Y., Shichida, Y., and Yoshizawa, T. (1992). Primary structures of chicken cone visual pigments: vertebrate rhodopsins have evolved out of cone visual pigments. Proc. Natl. Acad. Sci. U.S.A. 89, 5932–5936. doi: 10.1073/pnas.89.13.5932
Peirson, S. N., Halford, S., and Foster, R. G. (2009). The evolution of irradiance detection: melanopsin and the non-visual opsins. Philos. Trans. R. Soc. Lond. B Biol. Sci. 364, 2849–2865. doi: 10.1098/rstb.2009.0050
Sato, K., Yamashita, T., Ohuchi, H., and Shichida, Y. (2011). Vertebrate ancient-long opsin has molecular properties intermediate between those of vertebrate and invertebrate visual pigments. Biochemistry 50, 10484–10490. doi: 10.1021/bi201212z
Su, C. Y., Luo, D. G., Terakita, A., Shichida, Y., Liao, H. W., Kazmi, M. A., et al. (2006). Parietal-eye phototransduction components and their potential evolutionary implications. Science 311, 1617–1621. doi: 10.1126/science.1123802
Sugihara, T., Nagata, T., Mason, B., Koyanagi, M., and Terakita, A. (2016). Absorption characteristics of vertebrate non-visual Opsin, Opn3. PLoS ONE 11:e0161215. doi: 10.1371/journal.pone.0161215
Taylor, J. S., Braasch, I., Frickey, T., Meyer, A., and Van de Peer, Y. (2003). Genome duplication, a trait shared by 22000 species of ray-finned fish. Genome Res. 13, 382–390. doi: 10.1101/gr.640303
Terakita, A., Koyanagi, M., Tsukamoto, H., Yamashita, T., Miyata, T., and Shichida, Y. (2004). Counterion displacement in the molecular evolution of the rhodopsin family. Nat. Struct. Mol. Biol. 11, 284–289. doi: 10.1038/nsmb731
Terakita, A., and Nagata, T. (2014). Functional properties of opsins and their contribution to light-sensing physiology. Zool. Sci. 31, 653–659. doi: 10.2108/zs140094
Terakita, A., Tsukamoto, H., Koyanagi, M., Sugahara, M., Yamashita, T., and Shichida, Y. (2008). Expression and comparative characterization of Gq-coupled invertebrate visual pigments and melanopsin. J. Neurochem. 105, 883–890. doi: 10.1111/j.1471-4159.2007.05184.x
Terakita, A., Yamashita, T., and Shichida, Y. (2000). Highly conserved glutamic acid in the extracellular IV-V loop in rhodopsins acts as the counterion in retinochrome, a member of the rhodopsin family. Proc. Natl. Acad. Sci. U.S.A. 97, 14263–14267. doi: 10.1073/pnas.260349597
Tsukamoto, H., Farrens, D. L., Koyanagi, M., and Terakita, A. (2009). The magnitude of the light-induced conformational change in different rhodopsins correlates with their ability to activate G proteins. J. Biol. Chem. 284, 20676–20683. doi: 10.1074/jbc.M109.016212
Wada, S., Kawano-Yamashita, E., Koyanagi, M., and Terakita, A. (2012). Expression of UV-sensitive parapinopsin in the iguana parietal eyes and its implication in UV-sensitivity in vertebrate pineal-related organs. PLoS ONE 7:e39003. doi: 10.1371/journal.pone.0039003
Yamashita, T., Ohuchi, H., Tomonari, S., Ikeda, K., Sakai, K., and Shichida, Y. (2010). Opn5 is a UV-sensitive bistable pigment that couples with Gi subtype of G protein. Proc. Natl. Acad. Sci. U.S.A. 107, 22084–22089. doi: 10.1073/pnas.1012498107
Yau, K. W., and Hardie, R. C. (2009). Phototransduction motifs and variations. Cell 139, 246–264. doi: 10.1016/j.cell.2009.09.029
Keywords: bistable pigment, pineal photoreception, signal transduction, G protein, arrestin, wavelength discrimination, melatonin secretion, gene duplication
Citation: Koyanagi M, Kawano-Yamashita E, Wada S and Terakita A (2017) Vertebrate Bistable Pigment Parapinopsin: Implications for Emergence of Visual Signaling and Neofunctionalization of Non-visual Pigment. Front. Ecol. Evol. 5:23. doi: 10.3389/fevo.2017.00023
Received: 14 October 2016; Accepted: 21 March 2017;
Published: 11 April 2017.
Edited by:
Wayne Iwan Lee Davies, University of Western Australia, AustraliaReviewed by:
Jon Vidar Helvik, University of Bergen, NorwayCristiano Bertolucci, University of Ferrara, Italy
Copyright © 2017 Koyanagi, Kawano-Yamashita, Wada and Terakita. This is an open-access article distributed under the terms of the Creative Commons Attribution License (CC BY). The use, distribution or reproduction in other forums is permitted, provided the original author(s) or licensor are credited and that the original publication in this journal is cited, in accordance with accepted academic practice. No use, distribution or reproduction is permitted which does not comply with these terms.
*Correspondence: Akihisa Terakita, dGVyYWtpdGFAc2NpLm9zYWthLWN1LmFjLmpw