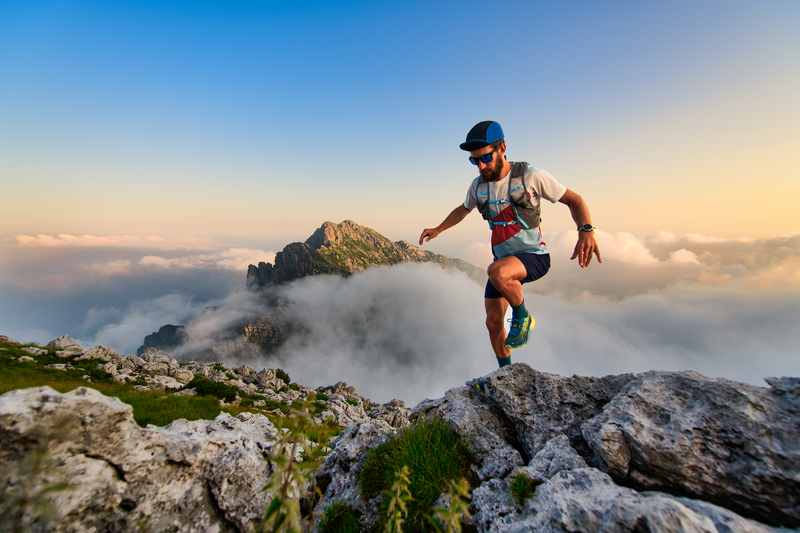
95% of researchers rate our articles as excellent or good
Learn more about the work of our research integrity team to safeguard the quality of each article we publish.
Find out more
REVIEW article
Front. Ecol. Evol. , 06 March 2017
Sec. Paleoecology
Volume 5 - 2017 | https://doi.org/10.3389/fevo.2017.00011
Resource managers around the world are challenged to develop feasible plans for sustainable conservation and/or restoration of the lands, waters, and wildlife they administer—a challenge made greater by anticipated climate change and associated effects over the next century. Increasingly, paleoecologic and geologic archives are being used to extend the period of record of observed data and provide information on centennial to millennial scale responses to long-term drivers of ecosystem change. The development of paleoecology from an emerging field investigating past environments to a highly relevant applied science is reviewed and general examples of the application of paleoecologic research to resource management questions in diverse habitats and regions are provided. Specific examples of the application of paleoecologic research to the restoration of the Greater Everglades Ecosystem of south Florida (U.S.A) are presented. Conducting valuable scientific research that would benefit resource management decisions, however, is not enough. Scientists and resource managers need to be engaged in collaborative discussions from the beginning of the research process to ensure that management questions are being addressed and that the science reaches the people who will benefit from the information. Paleoecology and related disciplines provide an understanding of how ecosystems and individual species function and change over time in response to both natural and anthropogenic drivers. Information on pre-anthropogenic baseline conditions is provided by paleoecologic research, but it is the detection of long-term trends and cycles that allow resource managers to set realistic goals and targets by moving away from the fixed-point baseline concept to one of dynamic landscapes that anticipates and incorporates an expectation of change into decision-making.
Ecosystem restoration is taking place around the world in a variety of habitats and at a variety of spatial scales, ranging from large watershed-scale efforts such as the Chesapeake Bay, the Greater Everglades and the San Francisco Bay-Delta in the United States, the Mata Atlântica tropical forest in Brazil, and the mangrove forests of Andhra Pradesh in India, to small scale local restoration efforts in a number of countries. In Europe, the Water Framework Directive specifically focuses on restoring all surface waters of the EU to “good” ecological and chemical status by 2015 (European Commission, 2015). The urgency of these efforts is highlighted by the United Nations Environmental Program estimate that close to a third of the world's ecosystems have been transformed or destroyed and another third have been significantly disrupted (Nellemann and Corcoran, 2010). In addition, the Millennium Ecosystem Assessment (2005) estimates that ~60% of ecosystem services examined in their report have been degraded or are being used unsustainably. Increasingly, the economic value and societal importance of restoring impaired ecosystems is being realized from international to community levels, and a number of organizations have provided guidance on best practices1 (Parks Canada and the Canadian Parks Council, 2008; Nellemann and Corcoran, 2010; Keenleyside et al., 2012).
Restoration practitioners have acknowledged that understanding the long-term historic condition of an ecosystem is essential to establish the baseline conditions and provide a framework for the goals of restoration (NRC, 1992; Egan and Howell, 2001; SER, 2004; Vigmostad et al., 2005). The temporal scale of historical observation and analysis, however, is often limited to a snapshot of the conditions prior to a specific disturbance. Furthermore, instrumental and observational records are limited in scope, often to only the last five to six decades. The lack of data and the difficulty of obtaining information on the historic condition of an unimpaired system is a common concern of restoration practitioners (Maltby and Dugan, 1994; Cairns and Heckman, 1996; Hobbs and Norton, 1996; SER, 2004). Paleoecologic and geologic archives can be invaluable in identifying the historic condition because ecosystems are dynamic networks of physical and biological components that change over a variety of time scales from diurnal to millennial and beyond. Diurnal, lunar, seasonal, annual, and to some extent decadal scale changes can be directly observed, measured, and/or monitored by scientists; however, to investigate change that occurs over decadal to millennial scales, paleoecologic, and geologic records must be used to extend the period of record past directly recorded data. For these reasons, scientists have advocated the use of paleoecologic and geologic data to provide the long-term perspective on community and ecosystem responses to change (for example, Binford et al., 1983; Davis, 1989; Anderson, 1993; Cohen, 1995; Orson, 1996; Parsons et al., 1999; Swetnam et al., 1999; Aronson and Precht, 2001; Jackson, 2001, 2007; Jackson et al., 2001; Kowalewski, 2001; Alin and Cohen, 2004; Weckström et al., 2004; NRC, 2005; Bottjer, 2006; Froyd and Willis, 2008; Jackson and Hobbs, 2009; Davies and Bunting, 2010; Gell, 2010; Watson et al., 2011; Seddon et al., 2014; Van Riper et al., 2014; Kidwell, 2015; Pellatt et al., 2015).
Extensive information can be derived from sediment cores, ice cores, tree rings, speleothems, corals, fossil assemblages, and other physical and biological recorders of change. These records provide more than just a snapshot of the pre-disturbance unimpaired ecosystem. They provide an understanding of the long-term (multidecadal to millennial) patterns and responses to change that is essential for effective management and restoration (Jackson and Hobbs, 2009; Gell, 2010). Managers and decision-makers need to have an understanding of past ecosystem changes in order to anticipate potential future responses to physical drivers and the interaction of both natural and anthropogenic changes over the next century. Only with this type of long-term perspective can resource managers make decisions that will meet the ultimate goals of restoration—goals that will be realized long after an individual manager's tenure and in a world of shifting political and economic realities.
The anticipated changes in climate and sea-level over the next century increase the importance of resource managers looking beyond short-term time scales. The following critical questions need to be asked at the planning stages of restoration efforts and reassessed as part of adaptive management when restoration is underway. (1) How do current rates of ecosystem change differ from past rates? (2) If there is a trend, is it linear or non-linear (e.g., exponential) and what is the direction of change? (3) If changes are cyclic, what is the magnitude and frequency of the cycles? (4) How have organisms responded to past changes in the physical environment? (5) Which organisms can serve as good indicators of change? Analyses of paleoecologic and geologic archives can address these questions. Paleoecologic data provide a unique perspective on patterns and rates of temporal change in the preserved biological components of an ecosystem, and by inference or direct measurement, the physical and chemical components of the system. Geological archives record the changes in the physical and chemical components of the ecosystem. Combined, these tools provide insight into the natural and anthropogenic drivers of change and the ecological responses to those drivers.
The purpose of this paper is to briefly review the history of paleoecology from early concepts to the present applied science; to provide a general overview of paleoecological applications in a variety of settings; and to provide specific examples of the application of paleoecology to restoration and resource management. For the specific examples, we draw from our own research in the Greater Everglades Ecosystem of south Florida, U.S.A., to illustrate the value and application of centennial to millennial scale perspective for resource managers.
British naturalist Edward Forbes (1815–1854) is credited with being the founder of paleoecology (Hedgpeth, 1957a), although the ancient Greeks were the first to recognize that fossils provided clues to past environments (Ladd, 1957a; Cloud, 1959). In his descriptions of the benthic fauna of the Aegean Sea, Forbes (1843) discussed the constant shifts in the bathymetric zones, and the relationship between changes in sediments, water depth, and assemblages. He related these changing patterns over time to the “interstratification of fossiliferous and non-fossiliferous beds” recognizing the connection between modern processes and what is preserved in the geologic record (Forbes, 1843, p. 173). Laying the groundwork for paleoecology, Forbes explained how zones of different depths in the marine environment can be recognized in uplifted rock layers and he applied this “zoo-geology” method to a brief interpretation of the stratigraphic layers on the island of Neo Kaimeni, Greece.
The earliest usage of the term paleoecology uncovered in this review is by the paleobotanists Berry and Clements in the early twentieth century (Berry, 1911, 1914; Clements, 1916, 1918) Berry identified the essential link to the study of modern organisms in his statement “the living representatives, their habitat, range and variation are of the greatest importance in determining paleoecology” (Berry, 1914, p. 142). Despite the earlier date on Berry's publication, Böger (1970) credits Clements (1916) with being the “creator” of the term paleoecology, referring to Clements definition of paleoecology as the response of fossil organisms and communities to their habitats and the response of the habitats to the organisms and communities (Clements, 1916, p. 279). Clements also made a clear connection between ecology and paleoecology stating that it was “undesirable to attempt a rigid distinction” between the two fields, yet he thought it was important to differentiate paleoecology due to the “inferential” nature of the interpretations (Clements, 1916, p. 280). He recognized the “great perspective” provided by paleoecology—a field that he envisioned as examining the “interrelations of climate, topography, vegetation, animals, and man”—and he saw a comparison of the paleoecologic record to the geologic record as a means of cross-checking the results (Clements, 1916, p. 279). It was also in 1916 that the first fossil assemblage diagrams were introduced by von Post in his lecture at the convention of Scandinavian naturalists. His technique of illustrating changes in the percent abundance of pollen in peat profiles from Sweden (Von Post, 1916) established a method that is used by the majority of paleoecologists today.
In the 1920s there was an increase in interest in marine paleoecology following the structure originally outlined by Forbes. T. W. Vaughan led the effort in the United States to incorporate ecology of modern marine environments into geologic interpretations (Vaughn, 1924). In Europe, O. Abel established the journal Paleobiologica and R. Richter founded the Senckenberg-am-Meer Institute, both furthering the development of the field of paleoecology (Hedgpeth, 1957a). In 1930, Twenhofel (1931) delivered his Presidential Address to the Paleontological Society in the U.S. summarizing the role of the environment in determining the sediments and faunal remains found in the geologic record and highlighting the importance of this information to stratigraphic understanding. The first instructional manual for teaching paleoecology, entitled “Principles and instruction for paleoecological investigations” was published by Gekker (1933) based on his lectures at the Mining Institute in St. Petersburg, Russia and derived from his research on basin analysis of the Russian platform. The manual was followed by numerous articles on the concepts of paleoecology (for example Gekker, 1941, 1948, 1955) and the first textbook on paleoecology (Gekker, 1957), with the English title “Introduction to Paleoecology,” which was subsequently translated into Chinese, Japanese, French, and English and had a worldwide influence on the field.
Between 1935 and 1937 the U.S. National Research Council formed a Committee on Paleoecology, headed by Twenhofel and including paleobotantist E.W. Berry, which produced two reports (Twenhofel, 1936, 1937). These reports added emphasis to Twenhofel's 1931 address with a discussion of the importance of understanding environmental facies and the role of paleoecology in the interpretation of facies. Twenhofel (1936) described the practice of correlating deposits containing the same fossil assemblages because it is assumed that the deposits were synchronous; but he pointed out that deposits with similar assemblages probably represent similar environments that may actually be time transgressive. This role of paleoecology in stratigraphy also was discussed by Vaughn in his 1939 Presidential Address to the Geological Society of America (Vaughn, 1940) and by Gekker in his 1957 textbook. Vaughn concluded his 1940 address by stating that “There should be continuous shuttling from studies of the modern to studies of the ancient and back again from the ancient to the modern. By this process we may confidently expect to increase our understanding of much of the history of the earth” (Vaughn, 1940, p. 466).
Following World War II, interest in paleoecologic research increased significantly. Woodring (1951) presented a paper at the U.S. National Academy of Sciences annual meeting discussing the use of oxygen isotopes to discern water temperatures and resolve conflicting evidence from assemblage analysis. Vaughn continued his efforts during the war and formed a subcommittee within the U.S. National Research Council Committee on Geologic Research in 1941, with the goal of summarizing activities in marine ecology and paleoecology (Ladd, 1957a). After the war, this committee continued under various names and eventually guided the compilation and publication of the two-volume Treatise on Marine Ecology and Paleoecology (Hedgpeth, 1957b, v. 1, Ecology; Ladd, 1957b, v. 2, Paleoecology). These two volumes laid the ground work for much of the marine paleoecologic research that would follow with contributions that focused on ecologic and environmental requirements of key groups of organisms and on the interpretation of physical aspects of past environments.
The publication of Treatise on Marine Ecology and Paleoecology and Gekker's (1957) textbook stimulated the development of paleoecology in the 1960s as a recognized subdiscipline of paleontology. Norman Newell is credited with elevating the field to subdiscipline status (Sepkoski, 2008) in part due to his organization, along with John Imbrie, of a Paleontological Society symposium on paleoecology at the 1961 annual meeting of the Society. Principles of Paleoecology (Ager, 1963) and Approaches to Paleoecology (Imbrie and Newell, 1964) served as foundational texts (Bambach, 2008) within English-speaking countries for the new subdiscipline, identifying the fundamental principles and approaches to paleoecologic studies. The launch of the international journal Palaeogeography, Palaeoclimatology, and Palaeoecology in 1965 with the intent of playing a role in developing “a clearer understanding of the behavior of the earth” (Manten and Nairn, 1965) and of the journal Paleobiology in 1975 were indicative of the increasing interdisciplinary interest in paleontological investigations.
Two pivotal publications were issued in the 1970s. Imbrie and Kipp (1971) introduced the factor analytic transfer function—a statistical method that uses factor analysis and linear regression of data on modern ecological requirements of organisms and applies those equations to the evaluation of fossil assemblages. This represented a significant step in moving paleoecologic analyses from primarily qualitative descriptions of assemblages based on numeric counts of taxa, to statistical data sets with associated errors and measures of confidence (Imbrie et al., 1973; Kipp, 1976). The ability to quantify confidence in interpretations is an important component in applying paleoecologic results to decision-making. The advent and subsequent explosion of personal computers in the 1980s contributed to this move toward rigorous analysis and added a “new perspective” to paleontology in general, and paleoecology specifically (Bambach, 2008, p. 30). The second publication, Valentine's Evolutionary Paleoecology of the Marine Biosphere (Valentine, 1973), was significant because it began to move paleoecology into the realm of understanding the processes by which ecosystems evolve and change over time (Bambach, 2008). The book also is notable because Valentine turned the uniformitarianism phrase around to say “the past is the key to the present” and developed the idea that changes in past environments and ecosystems can be used to understand present configurations. Valentine (1973, p. 466) saw the fossil record as “an interplay between evolutionary trends and environmental fluctuations” and it is this concept that is the foundation of the current paleoecologic investigations that examine late Holocene changes and the combined effects of anthropogenic and natural changes on ecosystems.
The growth in evolutionary paleoecology in the 1980s and 1990s led to an increased understanding of the relationships between the environment, ecology, and evolutionary processes and the importance of temporal and spatial scales on the interaction of these components (Allmon and Bottjer, 2001). In addition, the advances in stable isotope biogeochemistry and chronologic methods allowed for increased precision in comparing the physical and biological components of ecosystems preserved in geological archives and understanding the connections between the components (Allmon and Bottjer, 2001; Kowalewski, 2001; NRC, 2005). Refinement of the use of transfer functions and analog datasets allowed more precise and statistically defensible estimates of past environmental conditions (Birks et al., 1990). These developments provided the foundation for paleoecology to broaden its scope and begin to address research questions directly related to current resource management issues. In 1980, the value of understanding changes on the scale of decades to centuries was acknowledged by the US National Science Foundation when it began the Long Term Ecological Research (LTER) program (Hobbie et al., 2003). Although LTER studies focus on observations of living systems, the importance of extending the record beyond the centennial scale by linking long-term observations to paleoecologic studies was recognized (Davis, 1989; Kowalewski, 2001; Hobbie et al., 2003; NRC, 2005).
The decade of the 1990s saw an increased focus on restoration of degraded ecosystems and the impacts of global change. The role that paleoecology could play in addressing these issues was recognized as scientists and resource managers debated (1) what constitutes an appropriate baseline or reference condition for restoration given the extent of anthropogenic alterations to natural systems; (2) how to account for processes that occur infrequently or very slowly over decadal to centennial timescales and (3) what are the appropriate spatial and temporal scales for understanding ecosystems (Davis, 1989; Swetnam and Betancourt, 1998; Landres et al., 1999; Swetnam et al., 1999; Jackson and Overpeck, 2000; Jackson, 2001; Flessa, 2002). The U.S. National Academy of Sciences Committee on Restoration of Aquatic Ecosystems published a report in 1992 (NRC, 1992) that discussed the need for baseline studies and identified paleoecology as a method for obtaining information on the historic condition of ecosystems. By the end of the 1990s, the value of historical information on ecosystem variability and the use of paleoecology to obtain this information were increasingly recognized as essential components in resource management decisions (Landres et al., 1999; Millar and Woolfenden, 1999; Parsons et al., 1999; Swetnam et al., 1999).
In 2005, the U.S. National Academy of Sciences Committee on The Geologic Record of Ecological Dynamics (NRC, 2005, p. 3) identified three initiatives that apply the geologic and paleontologic record to scientific, management and societal issues: (1) use the record of the past as a “natural laboratory” to understand past responses to change under a range of conditions; (2) use the record “to enhance our ability to predict the response of biological systems to climate change in particular;” and (3) to use the Holocene record to examine anthropogenic vs. non-anthropogenic effects on ecosystems. These three initiatives capture the essence of much of the applied paleoecology and ecosystem history research that has been conducted over the last several decades. Paleoecology is not just viewed as a way to understand the past, but also as a window to the future (Swetnam et al., 1999; Kowalewski, 2001; Bottjer, 2006).
Debates continue about what constitute baseline and reference conditions, and what are the appropriate spatial and temporal scales for studies; and new debates have emerged about novel ecosystems, no-analog communities, and how to define sustainability (Jackson and Overpeck, 2000; Willard and Cronin, 2007; Williams and Jackson, 2007; Jackson and Hobbs, 2009; Watson et al., 2011), but paleoecology has entered the realm of applied science in the twenty-first century. Opportunities for collaboration between paleoecologists and the emerging field of conservation paleobiology offer the potential to further our understanding of interactions between evolutionary patterns, the environment, and biodiversity2. Perhaps paleoecology's greatest contribution to resource management as we move forward into uncertain conditions will be the understanding of ecosystem structure, function and responses to disturbance (Jackson and Hobbs, 2009). As the following sections illustrate, studying the geologic and paleontologic record of the past is playing an important role in shaping resource management decisions—not only looking backward to understand how an ecosystem has evolved and changed over time, but also looking forward to anticipate possible responses to future change. The past can serve as a guide to future decision-making.
Paleoecologic analyses have provided diverse and essential information to resource management agencies and decision-makers over approximately the last 30 years, with an increase in the last decade of studies focused on direct application to specific management questions. Research has been conducted from the tropics to the polar regions and in terrestrial, freshwater, estuarine and marine ecosystems. The examples in this section were selected to provide insight into the scope of applied paleoecological investigations and offer only a small sampling of the vast amount of research in this field. The common themes to all of these examples are that paleoecologic research has provided information on the range of variability in the system that extends beyond the period of direct observation and has examined responses to natural and/or anthropogenic disturbances over appropriate timescales. These examples all illustrate the use of paleoecology in testing hypotheses, establishing baseline conditions, examining ecological functioning and response to disturbances, and providing insight into resilience of species and systems.
Understanding and managing forest ecosystems is particularly difficult given the longevity of individual trees and the decadal to centennial time scales over which forest ecosystems develop. Critical questions are how resilient are forest ecosystems, what are the tipping points, how will forests respond to future climate change and will they shift to alternative states (Reyer et al., 2015). A number of paleoecologic studies have examined the impacts of anthropogenic and natural disturbances on forest ecosystems.
Pellatt et al. (2015) used pollen and charcoal analyses of lake sediment cores to investigate an ~500-year history of threatened Garry Oak ecosystems in British Columbia, Canada. Their results contributed to an understanding of the impact of climate, aboriginal land management practices, and European colonization on the forests and illustrated the strong linkage between forest structure and fire history. In a similar study, Crawford et al. (2015) combined ethnographic, anthropologic and paleoecologic data to investigate whether hunter-gathers influenced forest ecosystems of the Klamath Mountains, California, U.S.A. Bush and Colinvaux (1994) conducted the first paleoecologic investigation of the remote Darien region in Panama, an area that was thought to be one of the last neotropical forest ecosystems unaffected by humans. Their results revealed a 4000-year history of human disturbance and illustrated that the present-day forest formed over the last ~350 years, demonstrating the resiliency of the ecosystem following disturbance. A study of Malaysian Borneo tropical peat swamp forests using pollen and charcoal from sediment cores indicated the forest had been relatively stable over a 2000-year history, despite the occurrence of El Niño Southern Oscillation (ENSO) events, other climate changes and episodic fires (Cole et al., 2015); however, the last 500 years of fire history was evidence of human disturbance that exceeded any previous levels and caused a decline in the forest community. Karpińska-Kołaczek et al. (2014) determined the timing and extent of human impact on the woodlands of northeastern Poland, demonstrating that deforestation was most significant from the Middle Ages to the end of state farming in the 1990s. Other studies have investigated the history of infestations in Balsam Fir forests in Canada (Simard et al., 2002) and tested hypotheses explaining the development of savanna in a region of southeastern Venezuela where the climate is favorable to extensive rainforest development (Ballesteros et al., 2015).
Lacustrine ecosystems have been a focal point of many Holocene paleoecologic and paleoclimatologic studies because lakes serve as catchment basins preserving high-resolution records of natural and anthropogenic changes. The typically high sedimentation rates of these systems and the seasonal shifts in higher latitude lakes can preserve annual to decadal-scale resolution that predates anthropogenic alteration, thus providing exceptional records of temporal changes in the surrounding region (Birks and Birks, 1980; Anderson, 1993; Alin and Cohen, 2004; Quinlan et al., 2008; Smol, 2009). Changes in water quality (eutrophication, acidification, and increased sedimentation) are frequently examined through paleoecologic and sedimentologic records and are indicative of changes in the surrounding watershed (Birks and Birks, 1980; Last and Smol, 2001a,b; Smol et al., 2001a,b; Hawryshyn et al., 2012; Reavie et al., 2014; Cumming et al., 2015).
The Paleoecologic Investigation of Recent Lake Acidification (PIRLA) Project was formed in 1983 to investigate the effects of acid deposition related to burning of fossil fuels on North American lakes, addressing questions critical to scientists and policy-makers (Whitehead et al., 1990). Specifically, the PIRLA Project's goal was to examine if lakes in proximity to factories had undergone significant changes in acidity over the last 100 years. They recognized that long-term data on precipitation and lake-water biogeochemistry were the only way to test hypotheses about the relationship between industry and acid deposition and that the only source of these data was proxy information from sediment cores (Whitehead et al., 1990). In a smaller scale study, Ruggiu et al. (1998) investigated the impacts of industrial pollution on subalpine Lake Orta in Italy. Using diatoms from sediment cores they were able to reconstruct an approximately four century sequence of changes in the lake, detecting twentieth century fluctuations caused by copper contamination, acidification, and nitrification. They also identified periods of improvement in water quality associated with reduced copper loading, advancements in treatment facilities, and additions of lime to the lake to counter the acidification; these results were used to assess the success of restoration efforts underway at the time of the study (Ruggiu et al., 1998). Quinlan et al. (2008) emphasized the importance of long-term data in a similar study on the lakes in Ontario, Canada that assessed the combined effects of eutrophication, acidic deposition, and climate change prior to the beginning of monitoring efforts. Their results demonstrated that particularly when multiple stressors are affecting a system, paleoecologic analyses can integrate the cumulative effects better than modeling or experimental studies (Quinlan et al., 2008). Additionally, the concept that restoration of water quality does not necessarily mean biological recovery is highlighted by their findings (Quinlan et al., 2008). Paleoecologic studies have also provided information on the occurrence of endemic species in ancient lakes and changes in biodiversity due to the combined impacts of climate and anthropogenic stressors. Cohen (1995) examined the effects on endemic diversity from watershed disturbances caused by deforestation and accompanying increased erosion rates in Lake Tanganyika, Africa and demonstrated responses of species over time. Paleoecology was also listed as one of the seven science directions to be taken by the Centre of Socio-Ecological Problems of the Lake Baikal Basin in Russia (Tulokhonov et al., 1995).
Holocene paleoecologic studies of fluvial ecosystems most often focus on the associated wetland, marsh and delta deposits because the dynamic nature of the active channels is not conducive to preservation of detailed records of environmental change. The extent to which the wetland sediments record the ecosystem history of a river is dependent on the degree of connectivity between the river and the associated wetland and the frequency and height of flooding, which affects the sedimentary supply from the river to the wetland (Gell, 2010). The value of wetlands from both an economic and ecological standpoint is now recognized worldwide, although in the past they have often been treated as areas to be altered or modified for societal purposes (Barbier et al., 1997; EPA, 2002). Paleoecologic studies can be invaluable in determining the timing and impact of these alterations and also in predicting how wetlands will respond to future climate and sea level changes.
In an investigation of a wetland on the U.S.A. side of the St. Lawrence River, Rippke et al. (2010) were able to distinguish four distinct periods of succession in the plant communities and to determine that the current dominance of Typha (cattail) is related to peak agricultural disturbance in the late 1800s. Their findings demonstrated that water regulation in the Lake Ontario / St. Lawrence River system was not the cause of the present-day Typha dominance. Similar studies (Orson et al., 1992; Orson, 1999) in tidal marshes of the northeastern United States examined the effects of hydrologic alterations, beginning with early European colonization of the region, on marsh development and succession of plant communities. Orson (1996) also demonstrated the varying responses of freshwater and salt marshes to rising sea level; based on his results, he recommended that management begin to set aside land to accommodate future inland transgressions of the marshes. Litwin et al. (2013) determined that the effects of gravel mining, not river flooding, explained altered erosion rates in a tidal marsh along the Potomac River, Virginia, in eastern U.S.A. Their study used a combination of digital imagery analysis and paleoecologic analysis of sediment cores to assess causes and rates of land loss and provided the U.S. National Park Service with information to assist in restoration of the marsh. An investigation at the Urdaibai Biosphere Reserve on the north coast of Spain established that the marshes regenerated within ten years following abandonment of the agricultural areas and mosquito control structures that were in place by the end of the eighteenth century (Cearreta et al., 2002). Rates of natural response and regeneration are important for land managers to understand because facilitating natural processes is more successful at restoring ecological functioning of marsh ecosystems than engineering marsh restoration projects has been (Cearreta et al., 2002). Understanding pollution sources, eutrophication, turbidity and other water quality issues are important concerns in fluvial and wetland ecosystems (Gell, 2010; Bennion et al., 2014). Gell et al. (2007) analyzed paleoecologic records from wetlands adjacent to the River Murray, Australia, to provide information on the pre-colonization condition (salinity, biota, nutrients, sedimentation rates, and sediment sources) in response to the South Australian government's initiative to establish baseline conditions for the wetlands of the river system. Their study demonstrated the importance of examining long-term data sets to put the present state of the system into ecological context for managers Gell (2010).
Estuaries and coastal regions are highly desirable for development. Thirty-nine percent of the world's population lives within 100 km of the coast (Burke et al., 2001). The demands for potable water and land for development often conflict with resource management needs. These problems will only be compounded with anticipated sea level rise over the next century. Paleoecologic information can provide insight into future changes by providing information about past patterns and rates of change and responses to disturbance.
Large-scale estuarine restoration projects have utilized information on historical variability for several decades. Numerous paleoecologic analyses have been conducted in the Chesapeake Bay region, eastern U.S.A. (summarized in Willard and Cronin, 2007). These studies have indicated (1) the effects of deforestation and the centuries-long impact of land-use on the Chesapeake region (Cooper and Brush, 1993; Brush and Hilgartner, 2000; Willard et al., 2003); (2) the combined effects of climate and land-use (Cooper and Brush, 1993; Cronin and Vann, 2003; Willard et al., 2003; Brush, 2009); and (3) measures of water quality, such as salinity, eutrophication, hypoxia and anoxia (Cooper and Brush, 1993; Karlsen et al., 2000; Cronin and Vann, 2003; Willard et al., 2003; Brush, 2009). The results of these studies provide resource managers and policy-makers with information on pre-European colonization baseline conditions and anthropogenic vs. natural impacts to the Chesapeake Bay region over the last four centuries. In the Colorado River estuary, Mexico, changes in benthic productivity (Kowalewski et al., 2000) and salinity (Rodriquez et al., 2001) were demonstrated through a combination of paleoecologic assemblage analyses and oxygen isotope analyses. Prior to 1930, when the construction of dams altered the natural flow of water in the Colorado River, the delta ecosystem supported population densities of approximately fifty bivalve mollusks per m2, compared with three per m2 observed in the 1999–2000 study—a 90% decrease (Kowalewski et al., 2000). These findings address critical information gaps related to restoration of freshwater flow through the Colorado River because no surveys were conducted prior to alteration of natural flow (Kowalewski et al., 2000; Rodriquez et al., 2001).
In coastal zones and estuaries worldwide, paleoecologic analyses of assemblages have provided valuable information to resource managers on natural variability and responses to anthropogenic and natural disturbances. Jackson et al. (2001) discuss the importance of providing marine resource managers with information on the global impacts of overfishing on coastal ecosystems at the millennial scale using paleoecological, archeological, and historical data. They believe recent attempts at restoring coastal ecosystems, including coral reefs, sea grass beds, and kelp forests, have failed because these efforts “focused only on the most recent symptoms of the problem rather than on their deep historical causes” (Jackson et al., 2001, p. 636). In the Gulf of Finland, diatom distributions in cores have indicated the rate and magnitude of eutrophication and sediment loading over the last two centuries and the impact of these disturbances on species composition and biodiversity (Weckström, 2006; Weckström et al., 2007). These studies revealed differences in urban vs. rural areas and also demonstrated the response of the biota at some sites to decreases in waste water discharge, information that can be used to guide the EU's Water Framework Directive (Weckström, 2006; Weckström et al., 2007). In southeastern Brazil, Martínez et al. (2013) identified distinct changes in benthic assemblages in the Cananéia-Iguape estuarine-lagoonal system associated with construction of the Valo Grande channel and diversion of freshwater. Analysis of cores from Elkhorn Slough estuary, central California, U.S.A., indicated changes in marsh vegetation that corresponded to changes in sedimentation rates (Watson et al., 2011). The study was initiated because concerns about salt marsh transitioning to mud flats in recent years led to restoration planning and the need to characterize the baseline conditions and effects of land use changes. Their results showed that the build-up of salt marsh over the last century was most likely caused by increased sediment input from land-use changes; therefore loss of the salt marsh might not be a cause for concern and sustaining the salt marsh might not be feasible (Watson et al., 2011).
The papers discussed above are a very small subset of the hundreds of papers published in the last few decades applying paleoecologic analyses to resource management and restoration worldwide in virtually all of Earth's habitats. These examples offer a brief overview of the essential information paleoecology provides on pre-anthropogenic baseline conditions (defined differently for each region), the relationship between humans and our environment throughout human history, and the influence of natural changes on ecosystems. They also illustrate the combined effects of natural and anthropogenic drivers of change and introduce the value of applying these concepts to restoration and resource management. The following discussion of research in the Greater Everglades Ecosystem provides more detailed examples of the direct application of paleoecologic information to restoration and resource management.
The Greater Everglades Ecosystem is located in southern Florida and includes Everglades National Park (ENP), which has been designated a World Heritage Site, an International Biosphere Reserve and a Wetland of International Importance (Figure 1). The natural Everglades ecosystem was defined by a combination of the climate, hydrology, geology, and low-lying topography of south Florida, extending from the Kissimmee River in the central portion of the state south to the estuaries of Florida Bay, Biscayne Bay, and the southwest Florida coast, encompassing ~46,000 km2. The seasonal rainfall and low topographic relief make the area highly susceptible to flooding, so beginning in the late 1800s as the population of south Florida increased, there was a demand for protection from flooding, an increase in available land for agriculture and development, and additional water supply. In the early twentieth century, canals were constructed to divert water and roads and railways aided travel through the relatively undeveloped region (Light and Dineen, 1994). The Central and Southern Florida Project for Flood Control was authorized in 1948 (Light and Dineen, 1994) and included the construction of levees, pumps, water storage structures and additional canals. The combined effects of land use and water management significantly altered the natural flow of freshwater through the wetlands to the estuaries and coastal ecosystems during the twentieth century. Approximately 50 percent of the original Everglades remain today (mostly within Everglades National Park), but under an altered hydrologic regime; the rest has been lost to agriculture and development (Davis and Ogden, 1994; McVoy et al., 2011).
Figure 1. Satellite image of South Florida showing features discussed in text. Arthur R. Marshall Loxahatchee National Wildlife Refuge (labeled Loxahatchee NWR) is also Water Conservation Area (WCA) 1. Only the mouth of the Kissimmee River is shown where it empties into Lake Okeechobee; the river begins just south of Orlando and is considered the headwaters of the Everglades. White dotted lines indicate the boundaries of the National Park Service lands. Source of background image is USGS 1993 South Florida Satellite Image Map. United States index map shows location of Florida (gray) and satellite image (black box).
Political, social, and scientific interest in restoring the Everglades led to the development of the Comprehensive Everglades Restoration Plan (CERP) in the 1990s, which was authorized by Congress as part of the Water Resources Development Act of 2000 (U.S. Public Law 106-451) (USACE, 1999). Everglades restoration is envisioned as a 30 to 50-year effort at a cost of $12.5 billion (2008 price levels) (USACE (US Army Corps of Engineers) and SFWMD (South Florida Water Management District)., 2009). As this massive effort proceeds in the face of changing economies, politics and environmental conditions, it is essential that science provides the guidance and framework for restoration. The following examples illustrate the value of paleoecologic analyses in understanding past physical and biological parameters of the ecosystem and in gaining insight into ecosystem responses to projected changes in sea level and climate over the next several hundred years.
The distribution of wetland vegetation in the Everglades is primarily controlled by hydrology (hydroperiods, water depth, and salinity). The main controls on the hydrology over the last ~5000 years have been changes in climate and sea level, as well as human alteration of the natural freshwater flow over the last ~120 years (Willard and Bernhardt, 2011). Extreme changes in hydrology paired with biogeochemical feedback mechanisms are thought to be the main drivers of the formation and evolution of wetland communities such as ridges, sloughs, and tree islands (Wetzel et al., 2005; Ross et al., 2006). Paleoecological records from wetland sediment cores collected throughout the Everglades (Figure 2) illustrate how these drivers influenced the formation of the now iconic mosaic of wetland vegetation communities.
Figure 2. Map of south Florida showing location of cores and monitoring stations. Cores used for the regime shift analyses and the multiple linear regression models (MLRs) discussed in the text are indicated and specific monitoring stations discussed are labeled.
On short time scales, the multidecadal climate phenomena that the Greater Everglades wetlands are sensitive to include the North Atlantic Oscillation (NAO), the Atlantic Multidecadal Oscillation (AMO), and the El Niño Southern Oscillation (ENSO)(Cronin et al., 2002; Donders et al., 2005; Bernhardt and Willard, 2009; Wachnicka and Wingard, 2015). For example, periods of increased El Niño-like conditions are responsible for increased precipitation in the region, which in turn has impacted vegetation communities (Donders et al., 2005; Donders, 2011). On longer times scales, driven by the mean southward position of the Intertropical Convergence Zone (ITCZ), sustained aridity events also shaped the timing of tree island initiation and expansion (Willard et al., 2006; Bernhardt, 2011).
Two climate events in the late Holocene that were influential in the evolution of the ridge and slough landscape and tree island communities were the Medieval Climate Anomaly (MCA: ~650–1050 CE) and the Little Ice Age (LIA: ~1550–1750 CE). The MCA is characterized by sustained La Niña-like conditions and overall drier conditions in the southeastern US. When the pollen records from cores (Figure 3) are compared to modern analog data, they show that the MCA is a period of ridge formation and tree island initiation and expansion (Willard et al., 2006; Bernhardt and Willard, 2009; Bernhardt, 2011). These dry periods are critical in reinforcing biogeochemical feedbacks encouraging the establishment and growth of tree island and ridge vegetation (Ross et al., 2006). The LIA, while a period of cool wet conditions in the eastern US, is characterized by drier conditions in southern Florida (Pederson et al., 2005; Bernhardt and Willard, 2009). These sustained dry conditions stand out as another time period of tree island and sawgrass ridge growth (Willard et al., 2006; Bernhardt and Willard, 2009). Based on the microscopic charcoal proxy record, these intervals of extended aridity also are periods of increased fire (Bernhardt, 2011; Jones et al., 2014). Large changes associated with MCA aridity significantly impacted the rates of carbon accumulation in these systems (Jones et al., 2014).
Figure 3. Summary of changes in pollen percent abundance and peaks in charcoal from a sawgrass ridge core and a tree island core. The Little Ice Age (LIA) and the Medieval Climate Anomaly (MCA), indicated by the light gray bands, brought warmer drier climates to south Florida. (See Willard et al., 2006; Bernhardt and Willard, 2009; Bernhardt, 2011 for the data and an explanation of the environmental interpretation of the pollen record.)
One of the primary goals of studying the paleoecology of the Everglades ecosystem is to help land managers understand the effects of hydrologic modifications on the wetland communities they manage, whether these changes are due to management decisions or to anticipated climate and sea level changes over the next century. The paleoecologic record has demonstrated that past changes in precipitation influenced the distribution of many Everglades wetland communities, as described above. These longer term records serve as a baseline to anticipate how future modifications to the region's hydrology will alter vegetation. Research conducted in Arthur R. Marshall Loxahatchee National Wildlife Refuge (hereafter referred to as the Refuge) illustrates the importance of centennial-scale information for management decision making.
The Refuge, located in the northeastern portion of the Everglades (Figure 1) has undergone a suite of differently managed hydrological regimes since the 1950s (Brandt, 2006). The results of these sustained hydrologic changes are reflected in the pollen assemblages preserved in cores collected across the Refuge (Bernhardt et al., 2013). Using Sagittaria (broad leaf arrowhead) pollen as a proxy for hydrologic variability, Bernhardt et al. (2013) show that since significant alteration of the natural system began in the 1900s, the loss of natural hydrologic variability in the Everglades altered the distribution of wetland vegetation. One of the most significant findings was the loss of distinct hydroperiods in response to south Florida's natural wet and dry seasons. When compared to the last 300 years, the loss in variability in hydroperiods between seasons is greatest during the last 50–100 years. This indicates that the effects of human modification to the natural hydrology have been greater than the last 300 years of climate variability. The pollen assemblages also recorded the formation of a hydrological gradient with the northern part of the Refuge being drier than historical conditions and the southern being much wetter. These data as a whole provide targets for restoration goals if managers are trying to recreate pre-drainage conditions within the Refuge.
Currently, terrestrial and marine ecosystems in south Florida are under pressure from the combined effects of sea level rise, climate change, and twentieth century anthropogenic changes that have altered the natural functioning of the system. In the relatively flat, low-lying region, the impact of sea level rise on the natural and built systems is of particular concern. Rising sea level affects coastal ecosystems in this region long before inundation occurs as a result of saline ground-water intrusion and higher storm surges (Langevin and Zygnerski, 2013). Compounding the effects of rising sea level is the reduction in freshwater delivery to the coastal ecosystems that has occurred throughout the twentieth century following the construction of the complex system of canals and levees across the entire south Florida region. In addition to a reduction in the volume of freshwater, water management has led to changes in direction of water flow, decrease in water quality, and decrease in ground water levels by a few meters (Parker et al., 1955; Kohout and Kolipinski, 1967).
The coastal ecosystems of south Florida contain many biologically distinct components including the largest protected mangrove ecosystem in the western hemisphere, the most extensive living reef in the continental United States, and widespread protected subaquatic grass beds (Kimball, 2007). Each of the coastal biologic zones is characterized by distinct plant, vertebrate, invertebrate, and microbenthic communities with characteristic salinity regimes (McIvor et al., 1994; Fourqurean and Robblee, 1999; Lodge, 2010). These communities have evolved over the last ~5000 years in response to natural variations in sea level and climate. The resistance and resilience of these organisms and ecosystems are highly variable; however, studies have shown that the combined effect of climate and land use changes significantly decreases the ability of individual species or whole communities to absorb and/or respond to change (Scheffer et al., 2001; Folke et al., 2004; Mac Nally et al., 2014). The result often leads to distinct shifts in ecological regimes as the species composition and abundances change in response to ecosystem drivers (Smol et al., 2005; Wachnicka and Wingard, 2015; Randsalu-Wendrup et al., 2016). Paleoecologic analysis of sediment cores collected in the estuaries and coastal regions of south Florida have provided information on the responses of organisms and habitats to changes in sea level, climate, freshwater supply, and water quality (Figure 2).
An examination of changes in biotic communities throughout the time of deposition of the cores clearly indicates the effects of sea level rise over the last four millennia in Biscayne Bay and Florida Bay. The lower portions of many of these cores contain freshwater and mangrove peat deposits with taxa typically found in today's freshwater Everglades wetlands. Over time, these communities were replaced with species typical of the nearshore mangrove zone oligohaline (0.1–5.0 psu) communities, followed by estuarine to marine species, depending on the distance from land (Figures 4, 5). Numerous proxies have been examined (diatoms, ostracodes, mollusks, and foraminifera) and they indicate a similar trend toward increasingly saline environments over the last few centuries (Ishman et al., 1998; Willard et al., 1997; Brewster-Wingard et al., 2001; Wingard et al., 2003, 2004, 2007a; Murray et al., 2010; Wachnicka et al., 2010, 2011, 2013a,b,c; Cheng et al., 2012; Wingard and Hudley, 2012; Wachnicka and Wingard, 2015). Comparing these temporal changes in biotic communities across the region provides insight into the future migration paths of communities and species in response to predicted sea level rise in the twenty-first century.
Figure 4. Variations in percent abundance of three molluscan indicators over time illustrate changes in the nearshore environment of Florida Bay (T24, Figure 2) in response to sea level, climate and anthropogenic alteration. (A) Percent abundance of the three indicators. (B) Polymesoda floridana (southern marsh clam) on a mud flat; these clams can tolerate extremes in salinity but are found on exposed mudflats subjected to tidal and freshwater flooding. (C) A hydrobiid (minute freshwater snail) moving through the water; these snails are carried by the freshwater currents out into the estuaries and can serve as an indication of freshwater flow. Not shown: Cyrenoida floridana (Florida marsh clam) are typically found in very low salinity (oligohaline) water near sources of freshwater influx. Little Ice Age (LIA) and Medieval Climate Anomaly (MCA) are indicated by light gray bands.
Figure 5. Variations in percent abundance of dominant molluscan species in a core from central Biscayne Bay (NN, Figure 2) illustrate increasing salinity over time at the site. Species are arranged from left to right in order of increasing abundance near the core top. In general the shift in abundance toward the core top coincides with increasing preference for more marine (euhaline)/less estuarine (polyhaline) conditions.
The responses of south Florida estuarine biota to changes in climate have been determined by comparing paleoecologic records to twentieth century documented climate patterns (Cronin et al., 2001; Wachnicka et al., 2013a,b). Major shifts in the structure of ostracode and diatom assemblages often coincide with shifts in the intensity of ENSO, AMO, and the Pacific Decadal Oscillation (PDO). Wachnicka et al. (2013c) showed that the occurrence of severe and prolonged droughts associated with a combination of extreme cold phases of ENSO, AMO, and PDO, or a combination of warm phases of AMO and cold phases of PDO, corresponded to significant changes in the structure of diatom assemblages in cores from Biscayne Bay (Figure 6). For example, the largest shifts in diatom assemblage structure in Card Sound Bank and No Name Bank cores in the late 1950s coincided with changes in precipitation from below average to above average. During the same time period, the Palmer Drought Severity Index shifted from negative to positive, and PDO and ENSO from cold to warm phases. Additionally, freshwater deliveries from coastal wetlands to Biscayne Bay significantly decreased or ceased due to construction of a dense network of canals and levees along the bay's shoreline in the 1940s and 1950s. Shifts in assemblage structure do not always overlap exactly with shifts in climate indices or hydrological modifications because some locations and some species are more resistant to environmental change than others. Ecosystems with low ecological resilience may easily be tipped into an alternative state by a stochastic event, while those with high ecological resilience may quickly recover from relatively small perturbations. With the current state of knowledge it is impossible to definitively explain the mechanisms responsible for all the shifts in microbenthic community structure in the cores, but research continues on identifying cause and effect relationships and the resilience of different communities in south Florida's estuaries.
Figure 6. Comparison of historical patterns in climate indices (PDSI, AMO, PDO, NAO, ENSO), local weather, sea level, and freshwater flow from major canals along the Biscayne Bay coast to major shifts in diatom assemblage structure. Shifts in diatom assemblages (far right column) are shown from three locations in Biscayne Bay: No Name Bank (black), Featherbed Bank (blue), and Card Sound Bank (green) (NN, FB, and CB, Figure 2); dashed lines mark the most significant shift in each core. Red lines indicate significant shifts in the values measured. Adapted from Wachnicka et al. (2013c).
Compilation of analyses from sediment cores collected in the estuaries and coastal regions of south Florida have demonstrated that an ecological regime shift occurred across Florida Bay and Biscayne Bay in the mid-1950s and early-1960s (Figure 7; Wachnicka et al., 2013b,c) and across the southwest coastal margin in the 1980s, approximately the time period of implementation of the Central and Southern Florida construction projects (Light and Dineen, 1994). Post 1950, diatom communities in most cores show a significant increase in abundance of epiphytic communities and in taxa with wider salinity tolerances (Wachnicka and Wingard, 2015). The frequency and magnitude of shifts in diatom species composition also increased post-1950s (Wachnicka et al., 2013c; Wachnicka and Wingard, 2015), which agrees with research that has indicated the rate of biological change usually increases with continuous changes to environmental conditions (Folke et al., 2004). The diatom analyses are consistent with paleoecologic analyses of other benthic organisms (mollusks, ostracodes, foraminifers) that detected significant shifts between the 1940s and 1960s in cores from south Florida's estuaries (Brewster-Wingard and Ishman, 1999; Alvarez Zarikian et al., 2001; Brewster-Wingard et al., 2001; Cronin et al., 2001; Wingard et al., 2003, 2004).
Figure 7. Regime shifts in percent similarity of diatom assemblage structure over time in seven cores from Biscayne Bay and Florida Bay, since the late 1800s. The regime shift indices represent the cumulative sum of normalized anomalies of percent similarities in consecutive samples relative to a critical level; therefore, a higher value is indicative of increased change relative to the previous sample. Core locations are shown on (Figure 2) (blue diamonds).
Understanding biotic responses to anthropogenic and natural drivers of change over decadal to centennial timescales is essential for management agencies to predict how species and ecosystems will respond to future changes. Information provided by paleoecologic analyses of sediment cores allows scientists to examine the patterns and strength of species and habitat responses to a wide range of environmental stressors over time-scales that extend beyond our oldest recorded observations and measurements. For example, compiled data from cores across the region have demonstrated the inland migration of the mangrove ecotone and the nearshore estuarine species as sea level has risen over the last 4000 to 5000 years. This information demonstrates adaptation and the biotic response of migration, which illustrates the importance of planning for open space to allow for expansion of species ranges and/or migration. Understanding whether individual organisms and habitats will migrate, adapt or go extinct is critical to future planning. Paleoecologic data also assist in distinguishing natural from anthropogenic driven change, such as the regime shifts detected after the 1940s to 1950s. Such knowledge allows managers to make cost-effective decisions about focusing restoration efforts and resources on components of environmental change that they may be able to affect, and coming to terms with components of the ecosystem that will inevitably change.
Water is a defining component of the Everglades and the focus of restoration is to improve the quantity, quality, timing and delivery of water to the freshwater wetlands of the Everglades and the downstream estuaries of south Florida (USACE (U.S. Army Corps of Engineers) and DOI (U.S. Department of the Interior)., 2015). The relationship of the freshwater hydrology (stage, flow, hydroperiods) to estuarine salinity, prior to water management and significant anthropogenic alteration of south Florida (pre 1900 CE), is generally understood. As the volume of freshwater flowing through the Everglades to Florida Bay was reduced during the twentieth century, salinity in the bay increased (McIvor et al., 1994); in addition, unnatural timing of excessive amounts of freshwater delivered through canals during storm events has had deleterious effects on biota. To set targets and performance measures for restoration, however, it is essential to determine specifics of the relationship between freshwater delivery to the bay and salinity in the bay. For example, how much freshwater flow is necessary for oligohaline to mesohaline conditions in the nearshore transitional zones of the Florida Bay estuary? Did freshwater flow only impact the nearshore regions of the estuary under the natural system or were the outer reaches of the bay also affected? Instrumental data or historic scientific investigations that could answer these questions are sparse. The first rain gauges were installed in ENP in 1949, the first hydrologic gauges began operating in 1952 in the Everglades wetlands, and the first salinity stations were installed in Florida Bay in 1988 (Figure 2, triangles), decades after significant alteration of the hydrology and landscape of south Florida had begun. Yet the management teams responsible for overseeing restoration and establishing performance measurements and targets need to understand the hydrologic functioning of the natural system (RECOVER, 2014).
To fill this information gap and understand the salinity patterns within the estuaries prior to instrumental data and detailed field observations, the USGS and several universities began to collect sediment cores from the estuaries of south Florida in the 1990s (Figure 2) to conduct paleoecologic and other analyses (summarized in Wingard et al., 2007a). The focus from the beginning for the USGS core analyses was to understand the spatial and temporal changes in salinity in different regions of Florida Bay to assist resource managers in the establishment of performance measures and targets for restoration (Figures 5, 8). Age models for the cores were established using lead-210, carbon-14 and the presence of exotic pollen (Holmes et al., 2001; Wingard et al., 2007b). The exotic pollen marks the approximate beginning of the twentieth century, an important marker in south Florida because it is prior to the construction of any significant water control structures. Analyses of the cores from 1995–2006 established changing salinity patterns in the estuaries of south Florida as indicated by assemblage analysis of biota (primarily mollusks, ostracodes, benthic foraminifers, and diatoms; Brewster-Wingard et al., 1998, 2001; Ishman et al., 1998; Brewster-Wingard and Ishman, 1999; Huvane and Cooper, 2001; Wingard et al., 2003, 2004; Wachnicka and Wingard, 2015) and isotopic and elemental analyses of faunal remains (Halley and Roulier, 1999; Swart et al., 1999; Dwyer and Cronin, 2001). CERP planning teams and resource managers found this information on past conditions of the natural system interesting, but treated it as anecdotal, uncertain how to apply it directly to the establishment of restoration guidelines. What the core analyses lacked was a method to link the information from specific core sites to bay-wide salinity patterns and, more importantly, to freshwater hydrology in the Everglades wetlands.
Figure 8. Changes in molluscan assemblages from core collected at the mouth of Taylor Creek, northern margin of Florida Bay (T24, Figure 2), which indicate increases in salinity at the site over time. Species-weighted cumulative weighted percent (SW-CWP) value is shown on the far left (see text and Wingard and Hudley, 2012 for explanation of CWP). Species are arranged from left to right, ranging from freshwater to species that tolerate wide fluctuations in salinity (euryhaline).
The solution came through the development and application of a method that links multiple linear regression models that use observed hydrologic data (salinity, stage, flow) to a quantitative estimate of the paleosalinity indicated by molluscan assemblages in the cores (Marshall et al., 2009, 2014). The paleoecology component of this method is a modification of the modern analog technique (referred to as the cumulative weighted percent method—CWP), which applies data on living mollusk species to core assemblages to estimate an average salinity for each sample in a core (Wingard and Hudley, 2012). The modern analog data set contains salinity measurements and species observations from 933 site visits at 193 different sites in south Florida's estuaries3. The CWP paleosalinity estimate is calculated by multiplying the average salinity for each species (derived from field observations) by the percent abundance of each species in each core sample, summing, and dividing by 100. To account for the wide salinity tolerances of euryhaline species, nearshore or basin correction factors can be applied depending on the location of the core and the species. Before applying the CWP technique to the analysis of cores, the method was tested using modern samples collected near water monitoring stations that provided nearly continuous records of salinity. The test results demonstrated that the method could estimate average observed salinity at the water monitoring stations within ± 2 practical salinity units (psu) (Wingard and Hudley, 2012). The verification of the CWP method using known data provided the confidence to proceed with the analyses of five sediment cores from Florida Bay (pentagons Figure 2; CWP on Figure 8; Marshall and Wingard, 2012). The output from the paleoecologic analysis is an estimated salinity for the circa 1900 CE portion of each core—the time period just prior to the beginning of significant anthropogenic alterations of south Florida.
The modeling component of the method uses multiple linear regression models (MLRs) developed from hydrologic monitoring stations located throughout Everglades National Park (Figure 2; Marshall, 2005; Marshall et al., 2011). The MLRs predict (1) salinity in Florida Bay as a function of stage in the wetlands at specific locations; (2) salinity in Florida Bay as a function of salinity at specific locations; (3) flow in the wetlands as a function of stage at specific locations; and (4) stage in the wetlands as a function of stage at specific locations (Marshall et al., 2009, 2014). The key to the method is the relationship between salinity in Florida Bay and stage in the wetlands; this MLR was linked to the South Florida Water Management District's Natural Systems Model (NSM; SFWMD (South Florida Water Management District) and Interagency Modeling Center., 2005), which simulates daily stage and flow in 4-mi2 grids using observed climate data and assumes an unaltered landscape. By linking the NSM to the salinity-to-stage MLRs, estimates of NSM-based salinity were produced for Florida Bay (NSM/MLR; Marshall, 2005; Marshall et al., 2011); however, these NSM/MLR salinity estimates were higher than estimates produced by paleoecologic analysis (RECOVER, 2012a,b; Table 1). The solution was to use the paleo-based salinity estimates (CWP values) from the circa 1900 CE (prior to significant alteration of south Florida) section of each core to produce a simulated time series by adjusting the NSM/MLR (Marshall et al., 2009, 2014). For example, the paleo-based salinity estimate for the Whipray Basin core is −2.3 salinity units (psu) less than the NSM/MLR model (Table 1) so each value in the NSM/MLR time series for Whipray Basin is adjusted by −2.3 psu (Marshall and Wingard, 2012).
Table 1. Comparison of mean salinity values from observed data, model data, and paleosalinity estimates for each sediment core.
The simulated paleo-based time series is substituted for the observed salinity in the statistical models, and the models are run to predict salinity throughout Florida Bay, as well as upstream flow and stage, based on the paleosalinity. This process was repeated for each of the five cores, then the results from each core analysis were weighted using the Mean Square Error Estimate (MSE), then the weighted results were combined to produce a synthesized time series of pre-alteration salinity at each monitoring station in Florida Bay (Marshall et al., 2014). The results indicate that in the absence of water management, Florida Bay salinity would be ~3 to 9 psu lower than current conditions (Figure 9). To achieve these salinities, freshwater flow through the wetlands would need to be 2.1 to 3.7 times greater than existing flows and upstream stage would be approximately 0.25 m higher than current conditions (Figure 10).
Figure 9. Bar chart comparing the observed salinity from monitoring stations in Florida Bay (triangles on Figure 2) to the estimated salinity derived through applying paleosalinity estimates to the NSM/MLR models. Observed salinity is averaged by region of the bay over the period of record. (Period of record varies by region: North and Northeast = 1994–2000; Central and South = 1997–2000; West = 1998–2000). Data from Marshall et al. (2014).
Figure 10. Bar charts comparing the observed monitoring station data (Figure 2) to estimated flow and stage derived through applying paleosalinity estimates to the NSM/MLR models. (A) Comparison of stage at two stations (P33 and CP) to paleo-based estimates. Observed stage is averaged over the period of record. (Period of record is 1/1/1990 to 12/30/2000 for both stations.) (B) Comparison of flow through Shark River at Tamiami Trail (SRS) and Taylor Slough (TSB) at bridge over park road to paleo-based estimates. Observed flow is averaged over the period of record. (Period of record is 10/1978 through 12/2009 for SRS and 10/1960 through 12/2009 for TSB). Data from Marshall et al. (2014).
The results of this linkage between paleoecologic data and statistical models are being used to set targets for the Florida Bay salinity performance measures developed by the CERP's Restoration Coordination and Evaluation (RECOVER) Southern Coastal Systems team (Figure 11; RECOVER, 2012a,b, 2014). In the near future, the paleo-based stage and flow estimates could be used as targets for Everglades freshwater wetlands stage and flow performance measures. Decisions are still being made about alternative methods for restoring more natural flow to Everglades National Park and resource managers are attempting to incorporate sea level rise and changing climate patterns into these decisions. The understanding of the natural hydrologic connectivity of south Florida provided by these models is essential in assessing the future alternatives. In addition, as restoration projects come on line the models can be used for adaptive management to determine performance of various components of restoration. Scientists working on coastal and estuarine restoration can utilize this method to establish pre-anthropogenic hydrologic connections if they have access to available paleoecologic information and hydrologic data. Quantitative understanding of the natural system allows resource managers to establish targets for effective and sustainable restoration.
Figure 11. Graph illustrates the application of the paleo-based NSM/MLR salinity estimates to salinity performance measures and target setting by the RECOVER Southern Coastal Systems team. In this example, 2006 observed salinity data for Whipray Basin (WB, Figure 2), Florida Bay, are compared with the paleo-adjusted target range. For 2006, the salinity target was met only partially (indicated by arrow) when observed salinities dropped to upper range target values in late August and early September. Adapted from RECOVER (2012b).
The most significant challenges faced in applying paleoecologic investigations to the Greater Everglades Ecosystem restoration effort are the same challenges faced by paleoecologists working on resource management issues in any area. First, obtaining accurate chronologies and good age models are essential to determine the timing of significant changes indicated by the biotic remains in the cores and to examine anthropogenic vs. natural effects. In south Florida, hurricanes, human disturbance, and the effect of old carbon being taken up by living organisms complicate the process of developing age models (see Lowe and Walker, 1997 for a discussion of old carbon). Second, building a representative modern analog data set is the key to interpreting the fossil assemblages. The process of gathering modern data, however, takes time, particularly if the goal is to capture a variety of environmental or climatic conditions (for example, wet year vs. dry year). A related concern is whether to continue to gather modern analog data after analyses are completed and use the updated information to reassess previously developed models as conditions change. Ongoing restoration efforts potentially benefit from updated models, but the efforts simultaneously divert scientists from analyses of new locations that might also benefit resource management. Third is the question of preservation and whether the assemblages being analyzed are representative of the life assemblages. A number of authors have dealt with this question (Kidwell and Tomasovych, 2013, references in Table 1), but in south Florida the acidic peats and the alternate wetting and drying of portions of the landscape can lead to poor preservation of certain groups (Sanchez et al., 2013). A fourth challenge is the occurrence of no-analog taxa. Some species and assemblages present in the cores cannot be found living in south Florida today, but their presence in the recent past implies they are indicative of significant changes. Williams and Jackson (2007) discuss no-analog communities and point out that this problem will only increase as climate change occurs over the next century. Finally, efforts to incorporate uncertainty into paleoecologic analyses are a significant challenge (Seddon et al., 2014), but also a solution to dealing with imperfect age models, less than ideal analog datasets, inconsistent preservation, and no analog taxa. Resource managers can be presented with results that indicate the uncertainty inherent in the interpretations. Finding solutions to these challenges is important in applying paleoecologic data to Everglades restoration and to any restoration and resource management question being addressed.
In 2001 Kowalewski asked the question is applied paleoecology an oxymoron or reality? He concluded that it is a reality—the past can serve as the key to the future. The examples provided above reinforce Kowalewski's and others' conclusions (for example, Folke et al., 2004; Willis and Birks, 2006; Willard and Cronin, 2007; Jackson and Hobbs, 2009; Gell, 2010; Willis et al., 2010; Dietl and Flessa, 2011; Seddon et al., 2014; Dietl et al., 2015) that studying changes to Earth's ecosystems over centennial to millennial time scales is essential as we move forward with restoration and resource management programs in the twenty-first century. In addition, these examples illustrate implementation of the initiatives outlined in 2005 in the U.S. National Academy of Sciences Committee report on The Geologic Record of Ecological Dynamics (NRC, 2005) by documenting past responses to ecosystem change under a range of conditions; by providing information that can be used to predict biological responses to future changes; and by highlighting potential changes to ecosystems due to anthropogenic impacts.
Having excellent scientific information and valuable results, however, is not enough (Froyd and Willis, 2008; Flessa, 2009; Dietl and Flessa, 2011). Resource managers and scientists need to be engaged in discussions at the beginning of the research process. Science done in isolation from resource management and planning runs the risk of being under-utilized or obsolete by the time the research is published because decisions have already been made and managers have moved on to the next question. The Greater Everglades Ecosystem restoration provides an example of how scientists and resource managers work together. Everglades restoration is organized around teams responsible for developing restoration plans, targets, and performance measures for different components of the system (NRC, 2003; USACE (U.S. Army Corps of Engineers) and DOI (U.S. Department of the Interior)., 2015). Each of these teams is made of groups of resource managers, stakeholders, and scientists with different research specialties; therefore communication begins at the outset. Managers present an information need and scientists can immediately inform the team if the required research is feasible and if yes, give an estimate of the time and cost involved. Alternative research paths and solutions can be discussed that might be more efficient approaches to the same question. As the research proceeds, managers can be kept informed of progress, updated on preliminary findings, and when the research is complete the summary results can be presented directly to the team orally and in reports that summarize the key findings. Publication in peer-reviewed journals is still an essential step in this process, because it provides validation for the decisions the team ultimately makes—decisions that may be questioned in a court of law. However, using the team approach, managers have actionable information in hand before the final publication and scientists know that the right people will use their publications and apply their findings to the decision-making process.
Paleoecologists, conservation paleobiologists, and geologists need to be included in these resource management teams to provide the centennial to millennial scale perspective of ecosystem change and biotic responses to change. The U.S. National Park Service (NPS) has formally recognized the importance of understanding long term change in the landscapes it manages and has begun to adjust the concept of preserving resources “unimpaired4” for the future to allow for the dynamic nature of landscapes (Jarvis, 2016). A goal stated in the 2016 policy memorandum (Jarvis, 2016) is “to steward NPS resources for continuous change that is not yet fully understood.” In addition, the memorandum states that “New science—and new disciplines of science—have expanded our understanding of natural and cultural systems, and have revealed how much we do not yet know about how these systems function” (Jarvis, 2016).
Paleoecology and related disciplines are poised to meet the challenges for NPS and for restoration efforts around the world by providing information on how ecosystems function over time under changing pressures and drivers, and thus extend instrumental and observational records. The concept of a baseline and the application of these ideas have been debated (Millar and Woolfenden, 1999; Froyd and Willis, 2008; Jackson and Hobbs, 2009; Dietl and Flessa, 2011; Watson et al., 2011; Kidwell, 2015), but the majority of scientists and resource managers do not view a baseline as the goal for restoration of an ecosystem to a former condition. They recognize, as NPS has, that ecosystems are dynamic and that a baseline is a snapshot in time that represents a valuable, but arbitrary, starting point for understanding ecosystem changes over time. Often the baseline is considered the condition prior to anthropogenic alteration—a time that varies over centuries depending on where you are geographically—and serves as a reference point (Millar and Woolfenden, 1999; Froyd and Willis, 2008). Paleontologic and geologic archives provide a map of how an ecosystem has changed in response to natural drivers and/or anthropogenic disturbance, and what was the natural range of variability that existed in the past. Measurement of a proxy over a span of centuries to millennia indicates natural trends and disturbances to the system (Figure 12A); projection of this trend into the future provides a realistic restoration target that takes into account the natural trajectory of change in the variable of interest. Examining multiple proxies and combining these data increases the understanding of the interconnected responses between physical and biological components of an ecosystem. The goal of restoration should be to restore the ecosystem to the natural trajectory of change detected from multiple proxies. If the system has been altered too much (due to climate change, sea level rise, land-use or other drivers) alternative scenarios can be proposed based on an understanding of the system's response to change. In addition, paleontologic and geologic archives illustrate natural cycles (Figure 12B), which may indicate that a system is presently at a flexion point and changes can be anticipated if the ecosystem continues to follow these cycles. Combining results from analyses of multiple proxies from multiple sites, across various temporal and spatial scales, will lead to an understanding of the larger processes at work. Paleoecology is inherently multidisciplinary and the most informative results come from collaborations between scientists in related fields working in the near-term living environments and those investigating the longer-term record utilizing all available tools including biotic, isotopic, geochemical, biogeochemical, and sedimentological analyses.
Figure 12. Schematic diagrams illustrating application of long-term records to understanding past history of ecosystem and forecasting future change. (A) Proxy data indicate change in some variable in the past and a trend can be detected. Offset from the natural trend (gray area) occurs after disturbance. Restoration targets (red bull's eye) and goals should be to restore the ecosystem to the natural trajectory of change—not to the pre-disturbance condition. Alternate targets and scenarios (gray dashed lines) can be proposed to adjust natural trends for offsets due to drivers and stressors beyond the control of land management agencies (for example, rising sea level or climate patterns). (B) Illustration of long-term cyclic change. These cycles can be projected into the future providing resource managers an understanding of expected changes. It is important to recognize if an ecosystem currently exists at an inflection point (1) and change is expected (2).
Paleoecology is a thriving scientific discipline that has evolved over the last century to become an essential component of scientific research that is applied to ecosystem restoration and resource management. It provides the long-term perspective necessary to understand processes that occur on decadal to millennial and multimillennial time scales and to gain insight into how ecosystems and species function under changing conditions. Knowledge of past processes and responses provides the best means for establishing realistic and sustainable goals for restoration planning and resource management. Understanding long-term trends and cycles moves management decisions away from the fixed-point baseline concept to the idea of dynamic changing landscapes and allows managers to incorporate an expectation of change into future decision-making. The challenges faced by resource managers will only increase over the next century but new methods of investigating the past will continue to be developed. These new methods and evolving disciplines can be incorporated in to the process, but paleoecologists, geologists, and conservation paleobiologists need to have a seat at the table.
This paper was conceived of and primarily written by GW. Sections and figures detailing research in the Everglades were written by CB (Understanding landscape scale responses to climate and anthropogenic change) and AW (Understanding biotic responses to sea level, climate, and anthropogenic change). All authors reviewed and revised the manuscript.
All funding for this research has been provided by the U.S. Geological Survey, Greater Everglades Priority Ecoystems study area.
The authors declare that the research was conducted in the absence of any commercial or financial relationships that could be construed as a potential conflict of interest.
The Everglades research summarized here was supported by the USGS Greater Everglades Priority Ecosystems Science (GEPES) program. The authors would like to thank Harry Dowsett and Lucy Edwards (USGS, Reston, VA), and Frank Marshall (Cetacean Logic Foundation) for preliminary reviews of the manuscript. Bethany Stackhouse (USGS, Reston, VA) produced Figure 2, and assisted with the compilation of the references.
1. ^The Society for Ecological Restoration International provides links to a number of best practice documents from around the world at their website: http://www.globalrestorationnetwork.org/community-restoration-network/restoration-toolkit/best-practices/
2. ^Conservation Paleobiology is defined as the application of theories and analytical tools of paleontology to biodiversity conservation (Dietl and Flessa, 2011) and the use of geohistorical records to address current problems in the conservation and restoration of biodiversity and ecosystem services (Dietl et al., 2015). See also Dietl and Flessa (eds.) (2009); Dietl (2016); and Flessa (2002) for more information on conservation paleobiology.
3. ^Values as of September, 2015. Data are available at http://sofia.usgs.gov/exchange/flaecohist/.
4. ^The U.S. National Park Service was established in 1916 when the U.S. Congress passed the Organic Act (16 USC l 2 3, and 4). A component of the NPS mission identified in the Organic Act was “to conserve the scenery and the natural and historic objects and the wild life therein and to provide for the enjoyment of the same in such manner and by such means as will leave them unimpaired for the enjoyment of future generations.”
Alin, S. R., and Cohen, A. S. (2004). The live, the dead, and the very dead: taphonomic calibration of the recent record of paleoecological change in Lake Tanganyika, East Africa. Paleobiology 30, 44–81. doi: 10.1666/0094-8373(2004)030<0044:TLTDAT>2.0.CO;2
Allmon, W. D., and Bottjer, D. J. (2001). “Evolutionary paleoecology: the maturation of a discipline,” in The Ecological Context of Macroevolutionary Change, eds W. D. Allmon and D. J. Bottjer (New York, NY: Columbia University Press), 1–8.
Alvarez Zarikian, C. A., Swart, P. K., Hood, T., Blackwelder, P. L., Nelsen, T. A., and Featherstone, C. (2001). A century of environmental variaibility in Oyster Bay using ostracode ecological and isotopic data as paleoenvironmental tools. Bull. Am. Paleontol. 361, 133–143.
Anderson, N. J. (1993). Natural versus anthropogenic change in lakes: the role of the sediment record. Trends Ecol. Evol. 8, 356–361. doi: 10.1016/0169-5347(93)90219-F
Aronson, R. B., and Precht, W. F. (2001). Applied paleoecology and the crisis on Caribbean coral reefs. Palaios 16, 195–196. doi: 10.1669/0883-1351(2001)016<0195:APATCO>2.0.CO;2
Ballesteros, T., Montoya, E., Vegas-Vilarrúbia, T., Giralt, S., Abbott, A. B., and Rull, V. (2015). An 8700-year record of the interplay of environmental and human drivers in the development of the southern Gran Sabana landscape, SE Venezuela. Holocene 24, 1757–1770. doi: 10.1177/0959683614551229
Bambach, R. K. (2008). “Paleontology's greatest hits,” in From Evolution to Geobiology: Research Questions Driving Paleontology at the Start of a New Century, The Paleontological Society Papers 14, eds P. H. Kelley and R. K. Bambach (New Haven, CT: Yale University Printing), 17–40.
Barbier, E. B., Acreman, M., and Knowler, D. (1997). Economic Valuation of Wetlands: A Guide for Policy Makers and Planners. Gland: Ramsar Convention Bureau.
Bennion, H., Kelly, M. G., Juggins, S., Yallop, M. L., Burgess, A., Jamieson, J., et al. (2014). Assessment of ecological status in UK lakes using benthic diatoms. Freshwater Sci. 33, 639–654. doi: 10.1086/675447
Bernhardt, C. E. (2011). Native Americans, regional drought, and tree island evolution in the Florida Everglades. Holocene 27, 731–737. doi: 10.1177/0959683611400204
Bernhardt, C. E., and Willard, D. A. (2009). Response of the Everglades ridge and slough landscape to climate variability and 20th century water-management. Ecol. Appl. 19, 1723–1738. doi: 10.1890/08-0779.1
Bernhardt, C. E., Brandt, L. A., Landacre, B., Marot, M. E., and Willard, D. A. (2013). Reconstructing vegetation response to altered hydrology and its use for restoration, Arthur R. Marshall Loxahatchee National Wildlife Refuge, Florida. Wetlands 33, 1139–1149. doi: 10.1007/s13157-013-0469-y
Berry, E. W. (1911). A study of the Tertiary floras of the Atlantic and Gulf Coastal Plain. Proc. Am. Philos. Soc. 50, 301–315.
Berry, E. W. (1914). The affinities and distribution of the Lower Eocene flora of southeastern North America. Proc. Am. Philos. Soc. 53, 129–250. doi: 10.5962/bhl.title.7610
Binford, M. W., Deevey, E. S., and Crisman, T. L. (1983). Paleolimnology: an historical perspective on lacustrine ecosystems. Annu. Rev. Ecol. Syst. 14, 255–286. doi: 10.1146/annurev.es.14.110183.001351
Birks, H. J. B., and Birks, H. H. (1980). Quaternary Palaeoecology. London: Edward Arnold Publishers.
Birks, H. J. B., Line, J. M., Juggins, S., Stevenson, A. C., and Ter Braak, C. J. F. (1990). Diatoms and pH reconstruction. Philos. Trans. R. Soc. B 237, 263–278. doi: 10.1098/rstb.1990.0062
Böger, H. (1970). Bildung und gebrauch von begriffen in der paläoökologie [Notes on the formation and use of terms in paleoecology]. Lethaia 3, 243–269. doi: 10.1111/j.1502-3931.1970.tb01269.x
Bottjer, D. J. (ed.) (2006). Future Research Directions in Paleontology. The Paleontological Society. Available online at: http://paleosoc.org/wp-content/uploads/2015/10/FRDP-Brochure.pdf
Brandt, L. A. (2006). Benefits Anticipated from the 1995 Water Regulation Schedule for Water Conservation Area 1: Review and Analysis. USFWS Report LOX06-006. Boynton Beach: U.S. Fish and Wildlife Service. Available online at: http://sofia.usgs.gov/publications/reports/wca1_review/WCA1_rev2006_Acc5.pdf
Brewster-Wingard, G. L., and Ishman, S. E. (1999). Historical trends in salinity and substrate in central and northern Florida Bay: a paleoecological reconstruction using modern analogue data. Estuaries 22, 369–383. doi: 10.2307/1353205
Brewster-Wingard, G. L., Ishman, S. E., and Holmes, C. W. (1998). Environmental impacts on the southern Florida coastal waters: a history of change in Florida Bay. J. Coastal Res. 26, 162–172.
Brewster-Wingard, G. L., Stone, J. R., and Holmes, C. W. (2001). Molluscan faunal distribution in Florida Bay, past and present: an integration of down-core and modern data. Bull. Am. Paleontol. 361, 199–231.
Brush, G. S. (2009). Historical land use, nitrogen, and coastal eutrophication: a paleoecological perspective. Estuar. Coasts 32, 18–28. doi: 10.1007/s12237-008-9106-z
Brush, G. S., and Hilgartner, W. B. (2000). Paleoecology of submerged macrophytes in the upper Chesapeake Bay. Ecol. Monogr. 30, 645–667. doi: 10.1890/0012-5(2000)070[0645:POSMIT]2.0.CO;2
Burke, L., Kura, Y., Kassem, K., Revenga, C., Spalding, M., and McAllister, D. (2001). Pilot Analysis of Global Ecosystems: Coastal Ecosystems. Washington DC: World Resources Institute. Available online at: http://www.wri.org/publication/pilot-analysis-global-ecosystems-coastal-ecosystems
Bush, M. B., and Colinvaux, P. A. (1994). Tropical forest disturbance: paleoecological records from Darien, Panama. Ecology 75, 1761–1768. doi: 10.2307/1939635
Cairns, J., and Heckman, J. R. (1996). Restoration ecology: the state of an emerging field. Annu. Rev. Energy Environ. 21, 167–189. doi: 10.1146/annurev.energy.21.1.167
Cearreta, A., Irabien, M. J., Ulibarri, I., Yusta, I., Croudcae, I. W., and Cundy, A. B. (2002). Recent salt marsh development and natural regeneration of reclaimed areas in the Plentzia Estuary, N. Spain. Estuar. Coast. Shelf Sci. 54, 863–886. doi: 10.1006/ecss.2001.0862
Cheng, J., Collins, L. S., and Holmes, C. (2012). Four thousand years of habitat change in Florida Bay, as indicated by benthic foraminifera. J. Foramin. Res. 42, 3–17. doi: 10.2113/gsjfr.42.1.3
Clements, F. E. (1916). Plant Succession: An Analysis of the Development of Vegetation. Carnegie Institute of Washington Publication 242. Washington, DC: Carnegie Institute.
Clements, F. E. (1918). Scope and significance of paleo-ecology. Bull. Geol. Soc. Am. 29, 369–374. doi: 10.1130/GSAB-29-369
Cohen, A. S. (1995). Paleoecological approaches to conservation biology of benthos in ancient lakes: a case study from Lake Taganyika. J. N. Am. Benthol. Soc. 14, 654–668. doi: 10.2307/1467547
Cole, L. E. S., Bhagwat, S. A., and Willis, K. J. (2015). Long-term disturbance dynamics and resilience of tropical peat swamp forests. J. Ecol. 103, 16–30. doi: 10.1111/1365-2745.12329
Cooper, S. E., and Brush, G. S. (1993). A 2,500-year history of anoxia and eutrophication in Chesapeake Bay. Estuaries 5, 617–626. doi: 10.2307/1352799
Crawford, J. N., Mensing, S. A., Lake, F. K., and Zimmerman, S. R. H. (2015). Late Holocene fire and vegetation reconstruction from the western Klamath Mountains, California, USA: a multidisciplinary approach for examining potential human land use impacts. Holocene 25, 1–17. doi: 10.1177/0959683615584205
Cronin, T. M., and Vann, C. D. (2003). The sedimentary record of climatic and anthropogenic influence on the Patuxent estuary and Chesapeake Bay ecosystems. Estuaries 26, 196–209. doi: 10.1007/BF02695962
Cronin, T. M., Dwyer, G. S., Schwede, S. B., Vann, C. D., and Dowsett, H. J. (2002). Climate variability from the Florida Bay sedimentary record: possible teleconnections to ENSO, PNA, and CNP. Climate Res. 19, 233–245. doi: 10.3354/cr019233
Cronin, T. M., Holmes, C. W., Brewster-Wingard, G. L., Ishman, S. E., Dowsett, H., Keyser, D., et al. (2001). Historical trends in epiphytal ostracodes from Florida Bay: implications for seagrass and macro-benthic algal variability. Bull. Am. Paleontol. 361, 159–197.
Cumming, B. F., Laird, K. R., Gregory-Eaves, I., Simpson, K. G., Sokal, M. A., Nordin, R., et al. (2015). Tracking past changes in lake-water phosphorus with a 251-lake calibration dataset in British Columbia: tool development and application in a multiproxy assessment of eutrophication and recovery in Osoyoos Lake, a transboundary lake in Western North America. Front. Ecol. Evol. 3:84. doi: 10.3389/fevo.2015.00084
Davies, A. L., and Bunting, M. J. (2010). Applications of palaeoecology in conservation. Open Ecol. J. 3, 54–67. doi: 10.2174/1874213001003020054
Davis, M. B. (1989). “Retrospective studies,” in Long-Term Studies in Ecology, ed G. E. Likens (New York, NY: Springer-Verlag), 71–89.
Davis, S. M., and Ogden, J. C. (1994). “Introduction,” in Everglades: the Ecosystem and Its Restoration, eds S.M. Davis and J.C. Ogden (Delray Beach, FL: St. Lucie Press), 3–7.
Dietl, G. P. (2016). Brave new world of conservation paleobiology. Front. Ecol. Evol. 4:21. doi: 10.3389/fevo.2016.00021
Dietl, G. P., and Flessa, K. W. (eds.) (2009). “Conservation paleobiology: using the past to manage for the future, Paleontological Society short course, October 17th, 2009,” in The Paleontological Society Papers 15, (New Haven, CT: Yale University Printing).
Dietl, G. P., and Flessa, K. W. (2011). Conservation paleobiology: putting the dead to work. Trends Ecol. Evol. 26, 30–37. doi: 10.1016/j.tree.2010.09.010
Dietl, G. P., Kidwell, S. M., Brenner, M., Burney, D. A., Flessa, K. W., Jackson, S. T., et al. (2015). Conservation Paleobiology: leveraging knowledge of the past to inform conservation and restoration. Annu. Rev. Earth Planet. Sci. 43, 79–103. doi: 10.1146/annurev-earth-040610-133349
Donders, T. H. (2011). Middle Holocene humidity increase in Florida: climate or sea level? Quat. Sci. Rev. 103, 170–174. doi: 10.1016/j.quascirev.2014.09.011
Donders, T. H., Wagner, F., Dilcher, D. L., and Visscher, H. (2005). Mid- to late- Holocene El Niño – Southern Oscillation dynamics reflected in the subtropical terrestrial realm. Proc. Natl. Acad. Sci. U.S.A. 102, 10904–10908. doi: 10.1073/pnas.0505015102
Dwyer, G. S., and Cronin, T. M. (2001). Ostracode shell chemistry as a paleosalinity proxy in Florida Bay. Bull. Am. Paleontol. 361, 249–276.
Egan, D., and Howell, E. A. (eds.) (2001). The Historical Ecology Handbook. Washington, DC: Island Press.
EPA (2002). Functions and Values of Wetlands. U.S. Environmental Protection Agency Fact Sheet EPA843-F-01-002c. Avaiable online at: https://nepis.epa.gov/Exe/ZyPDF.cgi/200053Q1.PDF?Dockey=200053Q1.PDF
European Commission (2015). Good Water Quality in Europe - EU Water Directive. Available online at: http://eur-lex.europa.eu/legal-content/EN/LSU/?uri$=$CELEX:32000L0060
Flessa, K. W. (2009). “Putting the dead to work: translational paleoecology,” in Conservation Paleobiology: Using the Past to Manage for the Future, Paleontological Society Short Course, October 17th, 2009, The Paleontological Society Papers 15, eds G. P. Dietl and K. W. Flessa (New Haven, CT: Yale University Printing), 275–281.
Folke, C., Carpenter, S., Walker, B., Scheffer, M., Elmqvist, T., Gunderson, L., et al. (2004). Regime shifts, resilience, and biodiversity in ecosystem management. Annu. Rev. Ecol. Evol. Syst. 35, 557–581. doi: 10.1146/annurev.ecolsys.35.021103.105711
Forbes, E. (1843). Report on the Mollusca and Radiata of the Aegean Sea, and on their distribution, considered as bearing on Geology. Rep. Br. Assoc. Adv. Sci. 13, 139–207.
Fourqurean, J. W., and Robblee, M. B. (1999). Florida Bay: a history of recent ecological changes. Estuaries 22, 345–357. doi: 10.2307/1353203
Froyd, C. A., and Willis, K. J. (2008). Emerging issues in biodiversity & conservation management: the need for a palaeoecological perspective. Quat. Sci. Rev. 27, 1723–1732. doi: 10.1016/j.quascirev.2008.06.006
Gekker, R. F. (1933). Polozheniya i Instruktsiya dlya Ussledovaniy po Paleoekologii [Principles and Instruction for Paleoecological Investigations]. Moscow: Northwestern Geological Prospecting Trust.
Gekker, R. F. (1941). Zadachi paleoekologii v razrabotke problem evolyutsii organicheskogo [Paleoecological problems in working out problems of organic evolution]. Bull. Acad. Sci. USSR Biol. Ser. 1.
Gekker, R. F. (1948). Ocherednye problemy paleoekologii [Current problems of paleoecology]. Bull. Moscow Soc. Nat. 53, 23.
Gekker, R. F. (1955). Nastavlenie dlya Issledovaniy po Paleoekologii. [Instruction for Paleoecological Investigations, 2nd ed.]. Moscow: Paleontological Institute; Acad. Sci. USSR.
Gekker, R. F. (1957). Vvedenie v Paleoekologiju [Introduction to Paleocology]. Moscow: Gosgeoltekhizdat. [English addition: Hecker, R. F. (1965). Introduction to Paleoecology. New York, NY: Elsevier Publishing.]
Gell, P. (2010). “With the benefit of hindsight: the utility of palaeoecology in wetland condition assessment and identification of restoration targets,” in Ecology of Industrial Pollution, eds L. C. Batty and K. B. Hallberg (New York, NY: Cambridge University Press), 162–188.
Gell, P., Tibby, J., Little, F., Baldwin, D., and Hancock, G. (2007). The impact of regulation and salinisation on floodplain lakes: the lower river Murray, Australia. Hydrobiologia 591, 135–146. doi: 10.1007/s10750-007-0806-3
Halley, R. B., and Roulier, L. M. (1999). Reconstructing the history of eastern and central Florida Bay using mollusk-shell isotope records. Estuaries 22, 358–368. doi: 10.2307/1353204
Hawryshyn, J., Rühland, K. M., Quinlan, R., and Smol, J. P. (2012). Long-term water quality changes in a multiple-stressor system: a diatom-based paleolimnological study of Lake Simcoe (Ontario, Canada). Can. J. Fish. Aquat. Sci. 69, 24–40. doi: 10.1139/f2011-134
Hedgpeth, J. W. (1957a). “Introduction,” in Treatise on Marine Ecology and Paleoecology 1: Ecology, Vol. 67, ed J. W. Hedgpeth (Baltimore, MD: Geological Society of America), 1–16.
Hedgpeth, J. W. (ed.). (1957b). Treatise on Marine Ecology and Paleoecology 1: Ecology. (Baltimore, MD: Geological Society of America).
Hobbie, J. E., Carpenter, S. R., Grimm, N. B., Gosz, J. R., and Seastedt, T. R. (2003). The US long term ecological research program. BioScience 53, 21–32. doi: 10.1641/0006-3568(2003)053[0021:TULTER]2.0.CO
Hobbs, R. J., and Norton, D. A. (1996). Towards a conceptual framework for restoration ecology. J. Restor. Ecol. 4, 93–110. doi: 10.1111/j.1526-100X.1996.tb00112.x
Holmes, C. W., Robbins, J., Halley, R., Bothner, M., Brink, M. T., and Marot, M. (2001). Sediment dynamics of Florida Bay mud banks on a decadal time scale. Bull. Am. Paleontol. 361, 31–40.
Huvane, J. K., and Cooper, S. R. (2001). Diatoms as indicators of environmental change in sediment cores from northeastern Florida Bay. Bull. Am. Paleontol. 361, 145–158.
Imbrie, J., and Kipp, N. G. (1971). “A new micropaleontological method for quantitative paleoclimatology: application to a Late Pleistocene Caribbean core,” in The Late Cenozoic Glacial Ages, ed K. K. Turekian (New Haven, CT: Yale University Press), 71–181.
Imbrie, J., and Newell, N. (eds.) (1964). Approaches to Paleoecology. New York, NY: John Wiley & Sons Inc.
Imbrie, J., Van Donk, J., and Kipp, N. G. (1973). Paleoclimatic investigation of a Late Pleistocene Caribbean deep-sea core: comparison of isotopic and faunal methods. Quat. Res. 3, 10–38. doi: 10.1016/0033-5894(73)90051-3
Ishman, S. E., Cronin, T. M., Brewster-Wingard, G. L., Willard, D. A., and Verardo, D. J. (1998). A record of ecosystem change, Manatee Bay, Barnes Sound, Florida. J. Coastal Res. 26, 125–138.
Jackson, J. B. C., Kirby, M. X., Berger, W. H., Bjorndal, K. A., Botsford, L. W., Bourque, B. J., et al. (2001). Historical overfishing and the recent collapse of coastal ecosystems. Science 293, 629–638. doi: 10.1126/science.1059199
Jackson, S. T. (2001). Integrating ecological dynamics across timescales: real-time, Q-time, and deep-time. Palaios 16, 1–2. doi: 10.1669/0883-1351(2001)016<0001:IEDATR>2.0.CO;2
Jackson, S. T. (2007). Looking forward from the past: history, ecology, and conservation. Front. Ecol. Environ. 5:455. doi: 10.1890/1540-9295(2007)5[455:LFFTPH]2.0.CO;2
Jackson, S. T., and Hobbs, R. J. (2009). Ecological restoration in the light of ecological history. Science 325, 567–569. doi: 10.1126/science.1172977
Jackson, S. T., and Overpeck, J. T. (2000). Responses of plant populations and communities to environmental changes of the Late Quaternary. Paleobiology 26, 194–220. doi: 10.1666/0094-8373(2000)26[194:ROPPAC]2.0.CO;2
Jarvis, J. B (2016). Resource Stewardship for the 21st Century – Interim Policy. U.S. National Park Service Policy Memorandum 16-01. Available online at: https://www.nps.gov/policy/PolMemos/PM_16-01.htm
Jones, M. C., Bernhardt, C. E., and Willard, D. A. (2014). Late Holocene vegetation, climate, and land-use impacts on carbon dynamics in the Florida Everglades. Quat. Sci. Rev. 90, 90–105. doi: 10.1016/j.quascirev.2014.02.010
Karlsen, A. W., Cronin, T. M., Ishman, S. E., Willard, D. A., Kerhin, R., Holmes, C. W., et al. (2000). Historical trends in Chesapeake Bay dissolved oxygen based on benthic foraminifera from sediment cores. Estuaries 23, 488–508. doi: 10.2307/1353141
Karpińska-Kołaczek, M., Kołaczek, P., and Stachowicz-Rybka, R. (2014). Pathways of woodland succession under low human impact during the last 13,000 years in northeastern Poland. Quatern. Int. 328–329, 196–212. doi: 10.1016/j.quaint.2013.11.038
Keenleyside, K. A., Dudley, N., Cairns, S., Hall, C. M., and Stolton, S. (2012). Ecological Restoration for Protected Areas: Principles, Guidelines and Best Practices. IUCN Best Practice Protected Area Guidelines Series 18. Available online at: https://portals.iucn.org/library/efiles/documents/PAG-018.pdf
Kidwell, S. M. (2015). Biology in the Anthropocene: challenges and insights from young fossil records. Proc. Natl. Acad. Sci. U.S.A. 112, 4922–4929. doi: 10.1073/pnas.1403660112
Kidwell, S. M., and Tomasovych, A. (2013). Implications of time-averaged death assemblages for ecology and conservation biology. Annul. Rev. Ecol. Evol. Syst. 44, 539–563. doi: 10.1146/annurev-ecolsys-110512-135838
Kimball, D. (2007). Statement of Dan Kimball, Superintendent, Everglades National Park, National Park Service, Department of the Interior, before the Subcommittee on Interior, Environment, and Related Agencies of the House Appropriations Committee concerning climate change and lands administered by the Department of the Interior. Congressional testimony given April 26, 2007. Available online at: http://www.nps.gov/ever/parknews/everclimatechangetestimony.htm
Kipp, N. G. (1976). New transfer function for estimating past sea-surface conditions from sea-bed distribution of planktonic foraminiferal assemblages in the North Atlantic. Geol. Soc. Am. Mem. 145, 3–42. doi: 10.1130/MEM145-p3
Kohout, F. A., and Kolipinski, M. C. (1967). “Biological zonation related to groundwater discharge along the shore of Biscayne Bay, Miami, Florida,” in Estuaries: American Association for the Advancement of Science, Publication 83, ed G. H. Lauff (Washington, DC: AAAS), 488–499.
Kowalewski, M. (2001). Applied marine paleoecology: an oxymoron or reality? Palaios 16, 309–310. doi: 10.1669/0883-1351(2001)016<0309:AMPAOO>2.0.CO;2
Kowalewski, M., Avila Serrano, G. E., Flessa, K. W., and Goodfriend, G. A. (2000). Dead delta's former productivity: two trillion shells at the mouth of the Colorado River. Geology 28, 1059–1062. doi: 10.1130/0091-7613(2000)28<1059:DDFPTT>2.0.CO;2
Ladd, H. S. (1957a). “Introduction,” in Treatise on Marine Ecology and Paleoecology 2: Paleoecology, Vol. 67, ed H. S. Ladd (Baltimore, MD: Geological Society of America), 1–30.
Ladd, H. S. (ed.). (1957b). Treatise on Marine Ecology and Paleoecology 2: Paleoecology. (Baltimore, MD: Geological Society of America).
Landres, P. B., Morgan, P., and Swanson, F. J. (1999). Overview of the use of natural variaibility concepts in managing ecological systems. Ecol. Appl. 9, 1179–1188. doi: 10.1890/1051-0761(1999)009[1179:OOTUON]2.0.CO;2
Langevin, C. D., and Zygnerski, M. (2013). Effect of sea-level rise on salt water intrusion near a coastal well field in southeastern Florida. Groundwater 51, 781–803. doi: 10.1111/j.1745-6584.2012.01008.x
Last, W. M., and Smol, J. P. (eds.). (2001a). “Tracking environmental change using lake sediments: volume 1, basin analysis, coring, and chronological techniques,” in Developments in Paleoenvironmental Research 1, (Dordrecht: Kluwer Academic Publishers).
Last, W. M., and Smol, J. P. (eds.). (2001b). “Tracking environmental change using lake sediments: volume 2, physical and geochemical methods,” in Developments in Paleoenvironmental Research 2, (Dordrecht: Kluwer Academic Publishers).
Light, S. S., and Dineen, J. W. (1994). “Water control in the Everglades: a historical perspective,” in Everglades: The Ecosystem and Its Restoration, eds S. M. Davis and J. C. Ogden (Delray Beach, FL: St. Lucie Press), 47–84.
Litwin, R. J., Smoot, J. P., Pavich, M. J., Oberg, E., Steury, B., Helwig, B., et al. (2013). Rates and probable causes of freshwater tidal marsh failure, Potomac River Estuary, Northern Virginia, USA. Wetlands 33, 1037–1061. doi: 10.1007/s13157-013-0461-6
Lodge, T. E. (2010). The Everglades Handbook: Understanding the Ecosystem, 3rd Edn. Boca Raton, FL: CRC Press.
Lowe, J. J., and Walker, M. J. C. (1997). Reconstructing Quaternary Environments. Essex: Longman Group Ltd.
Mac Nally, R., Albano, C., and Fleishman, E. (2014). A scrutiny of the evidence for pressure-induced state shifts in estuarine and nearshore ecosystems. Austral Ecol. 39, 898–906. doi: 10.1111/aec.12162
Maltby, E., and Dugan, P. J. (1994). “Wetland ecosystem protection, management, and restoration: an international perspective,” in Everglades: The Ecosystem and Its Restoration, eds S.M. Davis and J.C. Ogden (Delray Beach, FL: St. Lucie Press), 29–46.
Manten, A. A., and Nairn, A. E. M. (1965). Palaeogeography, palaeoclimatology, palaeoecology: an introduction. Palaeogeogr. Palaeocol. Palaeoecol. 1, 1–3. doi: 10.1016/0031-0182(65)90002-7
Marshall, F. E. (2005). “Using statistical models to simulate salinity variability in estuaries,” in Estuarine Indicators, ed S.A. Bortone (Boca Raton, FL: CRC Press), 33–52.
Marshall, F. E., and Wingard, G. L. (2012). Florida Bay Salinity and Everglades Wetland Hydrology Circa 1900 CE: A Compilation of Paleoecology-Based Statistical Modeling Analyses. U S Geological Survey Open-File Report 2012-1054. Avaiable online at: http://pubs.usgs.gov/of/2012/1054
Marshall, F. E., Smith, D. T., and Nickerson, D. M. (2011). Empirical tools for simulating salinity in the estuaries of Everglades National Park. Estuar. Coast. Shelf Sci. 95, 377–387. doi: 10.1016/j.ecss.2011.10.001
Marshall, F. E., Wingard, G. L., and Pitts, P. A. (2009). A simulation of historic hydrology and salinity in Everglades National Park: coupling paleoecologic assemblage data with regression models. Estuar. Coasts 32, 37–53. doi: 10.1007/s12237-008-9120-1
Marshall, F. E., Wingard, G. L., and Pitts, P. A. (2014). Estimates of natural salinity and hydrology in a subtropical estuarine ecosystem: implications for Greater Everglades restoration. Estuar. Coasts 37, 1449–1466. doi: 10.1007/s12237-014-9783-8
Martínez, S., Mahiques, M. M., and Burone, L. (2013). Mollusks as indicators of historical changes in estuarine-lagoonal system (Cananéia-Iguape, SE Brazil). Holocene 23, 888–897. doi: 10.1177/0959683612470175
McIvor, C. C., Ley, J. A., and Bjork, R. D. (1994). “Changes in freshwater inflow from the Everglades to Florida Bay including effects on biota and biotic processes: a review,” in Everglades: The Ecosystem and its Restoration, eds S. M. Davis and J. C. Ogden (Delray Beach, FL: St. Lucie Press), 117–146.
McVoy, C. W., Said, W. P., Obeysekera, J., Van Arman, J. A., and Dreschel, T. W. (2011). Landscapes and Hydrology of the Predrainage Everglades. Gainesville, FL: University of Florida Press.
Millar, C. I., and Woolfenden, W. B. (1999). The role of climate change in interpreting historical variability. Ecol. Appl. 9, 1207–1216. doi: 10.1890/1051-0761(1999)009[1207:TROCCI]2.0.CO;2
Millennium Ecosystem Assessment (2005). Ecosystems and Human Well-being: Synthesis. Washington, DC: Island Press.
Murray, J. B., Wingard, G. L., Cronin, T. M., Orem, W. H., Willard, D. A., Holmes, C. W., et al. (2010). Evidence of Environmental Change in Rankin Basin, Central Florida Bay, Everglades National Park. U.S. Geological Survey Open-File Report 2010-1125. Available online at: http://sofia.usgs.gov/publications/ofr/2010-1125/index.html
Nellemann, C. E., and Corcoran, E. (eds.) (2010). Dead Planet, Living Planet – Biodiversity and Ecosystem Restoration for Sustainable Development: A Rapid Response Assessment. United Nations Environment Programme, GRID-Arendal. Available online at: http://www.unep.org/pdf/RRAecosystems_screen.pdf
NRC (National Research Council) (1992). Restoration of Aquatic Ecosystems: Science, Technology, and Public Policy. Washington, DC: National Academy Press.
NRC (National Research Council) (2003). Science and the Greater Everglades Ecosystem Restoration. Washington, DC: National Academy Press.
NRC (National Research Council) (2005). The Geological Record of Ecological Dynamics. Washington, DC.: National Academy Press.
Orson, R. A. (1996). Some applications of paleoecology to the management of tidal marshes. Estuaries 19, 238–246. doi: 10.2307/1352229
Orson, R. A. (1999). A paleoecological assessment of Phragmites australis in New England tidal marshes: changes in plant community structure during the last few millennia. Biol. Invasions 1, 149–158. doi: 10.1023/A:1010047731369
Orson, R. A., Simpson, R. F., and Good, R. E. (1992). The paleoecological development of a Late Holocene, tidal freshwater marsh of the upper Delaware River estuary. Estuaries 15, 130–146. doi: 10.2307/1352687
Parker, G. G., Ferguson, G. E., and Love, S. K. (1955). Water Resources of Southeastern Florida, with Special Reference to the Geology and Ground Water of the Miami Area. U.S. Geological Survey Water-Supply Paper 1255.
Parks Canada the Canadian Parks Council (2008). Principles and Guidelines for Ecological Restoration in Canada's Protected Natural Areas. Available online at: http://www.pc.gc.ca/docs/pc/guide/resteco/index_e.asp
Parsons, D. J., Swetnam, T. W., and Christensen, N. L. (1999). Uses and limitations of historical variability concepts in managing ecosystems. Ecol. Appl. 9, 1177–1178. doi: 10.1890/1051-0761(1999)009[1177:UALOHV]2.0.CO;2
Pederson, D. C., Peteet, D. M., Kurdyla, D., and Guilderson, T. (2005). Medieval warming, Little Ice Age, and European impact on the environment during the last millennium in the lower Hudson Valley, New York, USA. Quat. Res. 63, 238–249. doi: 10.1016/j.yqres.2005.01.001
Pellatt, M. G., McCoy, M. M., and Mathewes, R. W. (2015). Paleoecology and fire history of Garry oak ecosystems in Canada: implications for conservation and environmental management. Biodivers. Conserv. 24, 1621–1639. doi: 10.1007/s10531-015-0880-1
Quinlan, R., Hall, R. I., Paterson, A. M., Cumming, B. J., and Smol, J. P. (2008). Long-term assessment of ecological effects of anthropogenic stressors on aquatic ecosystems from paleoecological analyses: challenges to perspectives of lake management. Can. J. Fish. Aquat. Sci. 65, 933–944. doi: 10.1139/F08-027
Randsalu-Wendrup, L., Conley, D. J., Carstensen, J., and Fritz, S. C. (2016). Paleolimnological records of regime shifts in lakes in response to climate change and anthropogenic activities. J. Paleolimnol. 56, 1–14. doi: 10.1007/s10933-016-9884-4
Reavie, E. D., Heathcote, A. J., and Chraïbi, V. L. S. (2014). Laurentian Great Lakes phytoplankton and their water quality characteristics, including a diatom-based model for paleoreconstruction of phosphorus. PLoS ONE 9:e104705. doi: 10.1371/Journal.pone.0104705
RECOVER (REstoration COordination, VERification) (2012a). 2012 System Status Report Update: Southern Coastal Systems: Salinity Update. Available online at: http://141.232.10.32/pm/ssr_2012/hc_scs_salinity_results_2012.aspx#p7HGMpc_1_2
RECOVER (REstoration COordination, VERification) (2012b). Southern Coastal Systems Performance Measure: Salinity in Florida Bay. Available online at: http://141.232.10.32/pm/recover/recover_docs/perf_measures/062812_rec_pm_scs_salinity_flbay.pdf
RECOVER (REstoration COordination, VERification) (2014). Comprehensive Everglades Restoration Plan, 2014 System Status Report. Available online at: http://141.232.10.32/pm/ssr_2014/ssr_main_2014.aspx
Reyer, C. P. O., Rammig, A., Brouwers, N., and Langerwisch, F. (2015). Forest resilience, tipping points and global change processes. J. Ecol. 103, 1–4. doi: 10.1111/1365-2745.12342
Rippke, M. B., Matthew, T. D., and Farrell, J. M. (2010). Holocene vegetation dynamics of an upper St. Lawrence River wetland: paleoecological evidence for a recent increase in cattail (Typha). Wetlands 30, 805–816. doi: 10.1007/s13157-010-0068-0
Rodriquez, C. A., Flessa, K. W., and Dettman, D. L. (2001). Effects of upstream diversion of Colorado River water on the estuarine bivalve mollusc Mulinia coloradoensis. Conserv. Biol. 15, 249–258. doi: 10.1111/j.1523-1739.2001.99463.x
Ross, M. S., Mitchell-Bruker, S., Sah, J. P., Stothoff, S., Ruiz, P. L., Reed, D. L., et al. (2006). Interaction of hydrology and nutrient limitation in the ridge and slough landscape of the southern Everglades. Hydrobiologia 569, 37–59. doi: 10.1007/s10750-006-0121-4
Ruggiu, D., Luglié, A., Cattaneo, A., and Panzani, P. (1998). Paleoecological evidence for diatom response to metal pollution in Lake Orta (N. Italy). J. Paleolimnol. 20, 333–345. doi: 10.1023/A:100792992652
Sanchez, C., Gaiser, E. E., Saunders, C. J., Wachnicka, A. H., Oehm, N., and Craft, C. (2013). Challenges in using siliceous subfossils as a tool for inferring past water level and hydroperiod in Everglades marshes. J. Paleolimnol. 49, 45–66. doi: 10.1007/s10933-012-9624-3
Scheffer, M., Carpenter, S., Foley, J. A., Folke, C., and Walker, B. (2001). Catastrophic shifts in ecosystems. Nature 413, 591–596. doi: 10.1038/35098000
Seddon, A. W. R., Mackay, A. W., Baker, A. G., Birks, H. J. B., Breman, E., Buck, C. E., et al. (2014). Looking forward through the past: identification of 50 priority research questions in palaeoecology. J. Ecol. 102, 256–267. doi: 10.1111/1365-2745.12195
Sepkoski, D. (2008). “Evolutionary paleontology and the fossil record: a historical introduction,” in From Evolution to Geobiology: Research Questions Driving Paleontology at the Start of a New Century, The Paleontological Society Papers 14, eds P. H. Kelley and R. K. Bambach (New Haven, CT: Yale University Printing), 41–53.
SER (Society for Ecological Restoration International Science Policy Working Group). (2004). The SER International Primer on Ecological Restoration, ver. 2. Available online at: http://www.ser.org/resources/resources-detail-view/ser-international-primer-on-ecological-restoration.
SFWMD (South Florida Water Management District) Interagency Modeling Center. (2005). Documentation of the South Florida Water Management Model. West Palm Beach, FL: South Florida Water Management District. Available online at: http://www.sfwmd.gov/portal/page/portal/xweb%20-%20release%202/south%20florida%20water%20management%20model (Accessed 9/8/2016).
Simard, I., Morin, H., and Potelle, B. (2002). A new paleoecological approach to reconstruct long-term history of spruce budworm outbreaks. Can. J. For. Res. 32, 428–438. doi: 10.1139/X01-215
Smol, J. P. (2009). “Conservation biology and environmental change: a paleolimnological perspective,” in Conservation Paleobiology: Using the Past to Manage for the Future, Paleontological Society Short Course, The Paleontological Society Papers 15 eds G. P. Dietl and K. W. Flessa (New Haven, CT: Yale University Printing), 25–37.
Smol, J. P., Birks, H. J., and Last, W. M. (eds.). (2001a). “Tracking environmental change using lake sediments: volume 3, terrestrial, algal, and siliceous indicators,” in Developments in Paleoenvironmental Research 3. (Dordrecht: Kluwer Academic Publishers).
Smol, J. P., Birks, H. J., and Last, W. M. (eds.). (2001b). “Tracking environmental change using lake sediments: volume 4, zoological indicators,” in Developments in Paleoenvironmental Research 3. (Dordrecht: Kluwer Academic Publishers).
Smol, J. P., Wolfe, A. P., Birks, H. J. B., Douglas, M. S., Jones, V. J., Korhola, A., et al. (2005). Climate-driven regime shifts in the biological communities of arctic lakes. Proc. Natl. Acad. Sci. U.S.A. 102, 4397–4402. doi: 10.1073/pnas.0500245102
Swart, P. K., Healy, G., Greer, L., Lutz, M., Saied, A., Anderegg, D., et al. (1999). The use of proxy chemical records in coral skeletons to ascertain past environmental conditions in Florida Bay. Estuaries 22, 384–397. doi: 10.2307/1353206
Swetnam, T. W., Allen, C. D., and Betancourt, J. L. (1999). Applied historical ecology: using the past to manage for the future. Ecol. Appl. 9, 1189–1206. doi: 10.1890/1051-0761(1999)009[1189:AHEUTP]2.0.CO;2
Swetnam, T. W., and Betancourt, J. L. (1998). Mesoscale disturbance and ecological response to decadal climatic variability in the American southwest. J. Climate 11, 3128–3147. doi: 10.1175/1520-0442(1998)011%3C3128:MDAERT%3E2.0.CO;2
Tulokhonov, A. K., Gomboev, B. O., and Bardakhanova, T. B. (1995). “The Baikal region social ecology issues,” in Science Policy: New Mechanisms for Scientific Collaboration between East and West, Science and Technology Policy 1, NATO ASI Series 4, eds V. A. Koptyug and J. Klerkx (Kluwer Academic Publishers), 209–214.
Twenhofel, W. H. (1931). Environment in sedimentation and stratigraphy. Bull. Geol. Soc. Am. 42, 407–424. doi: 10.1130/gsab-42-407
Twenhofel, W. H. (1936). “Introduction,” in National Research Council, Report of the Committee on Paleoecology, 1935-1936 (Washington, DC: National Research Council), 1–9.
Twenhofel, W. H. (1937). “Introduction,” in National Research Council, Report of the Committee on Paleoecology, 1936-1937 (Washington, DC: National Research Council), 1–6.
USACE (U.S. Army Corps of Engineers) (1999). “Central and South Florida comprehensive review study,” in Final Integrated Feasibility Report and Programmatic Environmental Impact Statement. (Jacksonville, FL: U.S. Army Corps of Engineers). Available online at: http://141.232.10.32/pub/restudy_eis.aspx#p7HGMpc_1_2.
USACE (US Army Corps of Engineers) SFWMD (South Florida Water Management District). (2009). 2007-2008 Update Comprehensive Everglades Restoration Plan Report to the Public: An Annual Update. Available online at: http://141.232.10.32/pm/program_docs/cerp_report_public_2008.aspx.
USACE (U.S. Army Corps of Engineers) DOI (U.S. Department of the Interior). (2015). Central & Southern Florida Project: Report to Congress: Comprehensive Everglades Restoration Plan. Available online at: http://www.evergladesrestoration.gov/content/cerpreports/cerp_2015_rpt_to_congress.pdf.
Valentine, J. W. (1973). Evolutionary Paleoecology of the Marine Biosphere. Englewood Cliffs, NJ: Prentice-Hall Inc.
Van Riper, C., Nichols, J. D., Wingard, G. L., Kershner, J. L., Cloern, J., Jacobson, R. B., et al. (2014). USGS ecosystem research for the next decade: advancing discovery and application in parks and protected areas through collaboration. George Wright Forum 31, 129–136.
Vaughn, T. W. (1924). Oceanography in its relations to other Earth sciences. J. Washington Acad. Sci. 14, 307–333.
Vaughn, T. W. (1940). Ecology of modern marine organisms with reference to paleogeography. Bull. Geol. Soc. Am. 51, 433–468. doi: 10.1130/gsab-51-433
Vigmostad, K. E., Mays, N., Hance, A., and Cangelosi, A. (2005). Large-Scale Ecosystem Restoration and the Federal Policy Process. Northeast-Midwest Institute Center for Policy Initiatives Report, 3–8. Available online at: http://www.nemw.org/reports/ecosystem-restoration.
Von Post, L. (1916). Forest tree pollen in south Swedish peat bog deposits. Pollen Spores 9, 375–401.
Wachnicka, A., and Wingard, G. L. (2015). “Biological indicators of changes in water quality and habitats of the coastal and estuarine areas of the Greater Everglades ecosystem,” in Microbiology of the Everglades Ecosystem, eds J. A. Entry, A. D. Gottlieb, K. Jayachandrahan, and A. Ogram (Boca Raton, FL: CRC Press), 218–240.
Wachnicka, A., Collins, L., and Gaiser, E. (2013a). Response of diatom assemblages to 130 years of environmental change in Florida Bay (U.S.A.). J. Paleolimnol. 49, 83–101. doi: 10.1007/s10933-011-9556-3
Wachnicka, A., Gaiser, E., and Boyer, J. (2011). Autecology and distribution of diatoms in Biscayne Bay, Florida: implications for bioassessment and paleoenvironmental studies. Ecol. Indic. 11, 622–632. doi: 10.1016/j.ecolind.2010.08.008
Wachnicka, A., Gaiser, E., and Collins, L. (2013b). Correspondence of historic salinity fluctuations in Florida Bay, U.S.A., to atmospheric variability and anthropogenic changes. J. Paleolimnol. 49, 103–115. doi: 10.1007/s10933-011-9534-9
Wachnicka, A., Gaiser, E., Collins, L., Frankovich, T., and Boyer, J. (2010). Distribution of diatoms and development of diatom-based models for inferring salinity and nutrient concentrations in Florida Bay and adjacent coastal wetlands (U.S.A.). Estuar. Coasts 33, 1080–1098. doi: 10.1007/s12237-010-9283-4
Wachnicka, A., Gaiser, E., Wingard, L., Briceño, H., and Harlem, P. (2013c). Impact of Late Holocene climate variability and anthropogenic activities on Biscayne Bay (Florida, USA): evidence from diatoms. Palaeogeogr. Palaeocol. Palaeoecol. 371, 80–92. doi: 10.1016/j.palaeo.2012.12.020
Watson, E. B., Wasson, K., Pasternack, G. B., Woolfolk, A., Van Dyke, E., Gray, A. B., et al. (2011). Applications from paleoecology to environmental management and restoration in a dynamic coastal environment. Restor. Ecol. 19, 765–775. doi: 10.1111/j.1526-100X.2010.00722.x
Weckström, K. (2006). Assessing recent eutrophication in coastal waters of the Gulf of Finland (Baltic Sea) using subfossil diatoms. J. Paleolimnol. 35, 571–592. doi: 10.1007/s10933-005-5264-1
Weckström, K., Juggins, S., and Korhola, A. (2004). Quantifying background nutrient concentrations in coastal waters: a case study from an urban embayment of the Baltic Sea. Ambio 33, 324–327. doi: 10.1579/0044-7447-33.6.324
Weckström, K., Korhola, A., and Weckström, J. (2007). Impacts of eutrophication on diatom life forms and species richness in coastal waters of the Baltic Sea. Ambio 36, 155–160. doi: 10.1579/0044-7447(2007)36[155:IOEODL]2.0.CO;2
Wetzel, P. R., van der Valk, A. G., Newman, S., Gawlick, D. E., Troxler, T., Coronado-Molina, C. A., et al. (2005). Maintaining tree islands in the Florida Everlades: nutrient redistribution is the key. Front. Ecol. Environ. 3, 370–376. doi: 10.1890/1540-9295(2005)003[0370:MTIITF]2.0.CO;2
Whitehead, D. R., Charles, D. F., and Goldstein, R. A. (1990). The PIRLA project (Paleoecological Investigation of Recent Lake Acidification): an introduction to the synthesis of the project. J. Paleolimnol. 3, 187–194. doi: 10.1007/BF00219458
Willard, D. A., and Bernhardt, C. E. (2011). Impacts of past climate and sea level change on Everglades wetlands: placing a century of anthropogenic change into a late-Holocene context. Climatic Change 107, 59–80. doi: 10.1007/s10584-011-0078-9
Willard, D. A., and Cronin, T. M. (2007). Paleoecology and ecosystem restoration: case studies from Chesapeake Bay and the Florida Everglades. Front. Ecol. Environ. 5, 491–498. doi: 10.1890/070015
Willard, D. A., Bernhardt, C. E., Holmes, C. W., Landacre, B., and Marot, M. (2006). Response of Everglades tree islands to environmental change. Ecol. Monogr. 76, 565–583. doi: 10.1890/0012-9615(2006)076[0565:ROETIT]2.0.CO;2
Willard, D. A., Brewster-Wingard, G. L., Fellman, C., and Ishman, S. E (1997). Paleontological Data from Mud Creek Core 1, Southern Florida. US Geological Survey Open-file Report 97–736. Available online at: http://pubs.usgs.gov/pdf/of/ofr97736.html.
Willard, D. A., Cronin, T. M., and Verardo, S. (2003). Late-Holocene climate and ecosystem history from Chesapeake Bay sediment cores, USA. Holocene 13, 201–214. doi: 10.1191/0959683603hl607rp
Williams, J. W., and Jackson, S. T. (2007). Novel climates, no-analog communities, and ecological surprises. Front. Ecol. Environ. 5, 475–482. doi: 10.1890/070037
Willis, K. J., and Birks, J. B. (2006). What is natural? The need for a long-term perspective in biodiversity conservation. Science 314, 1261–1265. doi: 10.1126/science.1122667
Willis, K. J., Bailey, R. M., Bhagwat, S. A., and Birks, H. J. B. (2010). Biodiversity baselines, thresholds and resilience: testing predictions and assumptions using paleoecological data. Trends Ecol. Evol. 25, 583–591. doi: 10.1016/j.tree.2010.07.006
Wingard, G. L., and Hudley, J. W. (2012). Application of a weighted-averaging method for determining paleosalinity: a tool for restoration of south Florida's estuaries. Estuar. Coasts 35, 262–280. doi: 10.1007/s12237-011-9441-3
Wingard, G. L., Cronin, T. M., and Orem, W. (2007a). “Ecosystem history,” in Florida Bay Science Program: a synthesis of research on Florida Bay, Florida Fish and Wildlife Research Institute Report TR-11, eds J. Hunt and W. Nuttle. 9–29. Available online at: http://f50006a.eos-intl.net/ELIBSQL12_F50006A_Documents/Technical%20Report%20TR-11_R.pdf.
Wingard, G. L., Cronin, T. M., Dwyer, G. S., Ishman, S. E., Willard, D. A., Holmes, C. W., et al. (2003). Ecosystem History of Southern and Central Biscayne Bay: Summary Report on Sediment Core Analyses. U.S. Geological Survey Open-file Report 03-375. Available online at: http://sofia.usgs.gov/publications/ofr/03-375/.
Wingard, G. L., Cronin, T. M., Holmes, C. W., Willard, D. A., Dwyer, G. S., Ishman, S. E., et al. (2004). Ecosystem History of Southern and Central Biscayne Bay: Summary Report on Sediment Core Analyses – Year Two. U.S. Geological Survey Open-file Report 2004-1312. Available online at: http://sofia.usgs.gov/publications/ofr/2004-1312.
Wingard, G. L., Hudley, J. W., Holmes, C. W., Willard, D. A., and Marot, M. (2007b). Synthesis of Age Data and Chronology for Florida Bay and Biscayne Bay Cores Collected for the Ecosystem History of South Florida's Estuaries Projects. U.S. Geological Survey Open-file Report 2007-1203. Available online at: http://f50006a.eos-intl.net/ELIBSQL12_F50006A_Documents/Technical%20Report%20TR-11_R.pdf.
Keywords: applied paleoecology, ecosystem restoration, baseline, resource management, future change, Everglades
Citation: Wingard GL, Bernhardt CE and Wachnicka AH (2017) The Role of Paleoecology in Restoration and Resource Management—The Past As a Guide to Future Decision-Making: Review and Example from the Greater Everglades Ecosystem, U.S.A. Front. Ecol. Evol. 5:11. doi: 10.3389/fevo.2017.00011
Received: 20 September 2016; Accepted: 17 February 2017;
Published: 06 March 2017.
Edited by:
Gavin L. Simpson, University of Regina, CanadaReviewed by:
Mark Bush, Florida Institute of Technology, USACopyright © 2017 Wingard, Bernhardt and Wachnicka. This is an open-access article distributed under the terms of the Creative Commons Attribution License (CC BY). The use, distribution or reproduction in other forums is permitted, provided the original author(s) or licensor are credited and that the original publication in this journal is cited, in accordance with accepted academic practice. No use, distribution or reproduction is permitted which does not comply with these terms.
*Correspondence: G. Lynn Wingard, bHdpbmdhcmRAdXNncy5nb3Y=
Disclaimer: All claims expressed in this article are solely those of the authors and do not necessarily represent those of their affiliated organizations, or those of the publisher, the editors and the reviewers. Any product that may be evaluated in this article or claim that may be made by its manufacturer is not guaranteed or endorsed by the publisher.
Research integrity at Frontiers
Learn more about the work of our research integrity team to safeguard the quality of each article we publish.