- 1Centre for Integrative Ecology, School of Life and Environmental Sciences, Deakin University, Burwood, VIC, Australia
- 2Institute for Sustainability and Innovation, College of Engineering and Science, Victoria University, Melbourne, VIC, Australia
- 3Department of Ecology, Environment and Evolution, School of Life Sciences, LaTrobe University, Bundoora, VIC, Australia
The examination of links between a high degree of encephalisation (i.e., a large brain mass relative to body size) and the capacity of wildlife to inhabit anthropogenic habitats has formed the basis of several recent studies, although typically they have not uncovered any relationship. It, however, remains unclear whether encephalisation is directly related to a species' capacity to develop tolerance to human proximity (i.e., a reduction in response to approaching humans). It is also unknown whether such a relationship is related to the size of specific areas of the brain. Using published data on flight-initiation distance (FID), the distance at which animals flee from an approaching human, we estimate the degree of tolerance of human proximity for 42 bird species by comparing FIDs in urban and rural areas, with relatively high and low exposure to humans, respectively. We used a phylogenetic, comparative approach to analyse the relationship of degree of tolerance, and of FID in urban and rural populations more directly, to relative sizes of whole brains (42 species) and brain components (25 species) for the species, and examine the effect of the year that the bird species was first recorded in an urban area (year of urbanization). We demonstrate an interaction between bird habitat and year of urbanization on FIDs. Urban populations of species that have a longer history of inhabiting urban areas have lower FIDs (i.e., birds that were urbanized earlier are more tolerant), which may suggest local selection for birds with reduced responsiveness to humans in urban areas. The pattern is not seen in rural populations of the same species, providing additional evidence that it is greater exposure to humans that has resulted in this tolerance. While we found that forebrain mass and optic lobe mass are influential positive predictors of FID there was no indication that degree of tolerance itself was related to any brain size metric and hence no support for the idea that urban populations of species with larger brains are better able to habituate to human presence. This suggests that processes other than encephalisation explain the high degree of tolerance evident in urban-dwelling birds.
Introduction
Some species of birds have colonized urban areas from ancestral, rural populations. The “cognitive buffer” hypothesis suggests that larger-brained animals (such as birds) are better able to adapt to novel environmental conditions, such as those created by urbanization (Sol et al., 2005a). In addition, larger brains may be associated with more proactive (or “bold”) personality types in which animals may be quicker to explore novel environments (Kotrschal et al., 2014). In theory, birds with larger brains may be able to more accurately judge risk when presented with evolutionary novel stimuli, or be more able to learn (habituate or sensitize) to adjust responses appropriately, based on their previous experience (Guay et al., 2013c). Despite these predictions, comparative studies have found no evidence that bird species with relatively larger brains are more likely to colonize urban habitats (Kark et al., 2007; Evans et al., 2011; Møller and Erritzøe, 2015), nor that larger brain size in birds is linked to the time at which a species started living in urban habitats (Møller and Erritzøe, 2015). However, these studies focus on urbanization per se, rather than the individual behaviors that may be associated with adaptation to urban environments. Of these, the capacity to discriminate benign, but common, potential threats (e.g., humans) from more dangerous ones is thought to be especially adaptive in urban environments because escape responses can be costly (Ydenberg and Dill, 1986). One way of measuring such discrimination, risk perception, and responsiveness of animals is through the flight-initiation distance (FID), the distance between a threatening stimulus and an animal when an escape response is initiated (Weston et al., 2012). Many species exhibit reduced FIDs to humans in areas where humans are more common (Kitchen et al., 2010; Weston et al., 2012; McGiffin et al., 2013; Gravolin et al., 2014; van Dongen et al., 2015b; Vines and Lill, 2015). These species can be said to have developed “tolerance” of human proximity—defined as when animals permit closer approaches by humans without overtly responding or fleeing (Blumstein, in press).
This tolerance is often inferred to be caused by habituation (the reduction of responsiveness with increasing exposure to a stimulus) most commonly the result of within-animal learning (Møller, 2010; Weston et al., 2012). But, in fact, other “habituation-like processes” may act to reduce responsiveness in animals in areas where humans are more common (Blumstein, in press). For example, local selection involving bolder individuals settling or remaining in busier areas (van Dongen et al., 2015b) may act across generations to reduce responsiveness over time. This is especially the case where species invade urban areas from adjoining non-urban areas. Under these circumstances species with a longer histories of urbanization are expected to have the greatest reduction in responsiveness because these selection processes will act across generations. Indeed, among birds, the earlier the year in which a species colonizes urban areas the shorter it's FID, suggesting evolution of avian responses to humans (Møller, 2008, 2010; but see Gendall et al., 2015).
If the degree of tolerance to humans in urban populations is also the result of learning and habituation then the cognitive buffer hypothesis would predict that species with larger brains should display greater degree of tolerance to humans in urban habitats. A key challenge for this theory, however, is how to measure cognitive capacity. Relative whole brain size is often used as a surrogate for a species' cognitive ability and is available for a broad range of birds (Madden, 2001; Sol et al., 2005a; Healy and Rowe, 2007; Guay and Iwaniuk, 2008; Sol, 2009). More recently, and because of recognition of functional specialization of different parts of the brain (Healy and Rowe, 2007), brain components have been used (e.g., Symonds et al., 2014). In birds the detection of, and response to threats is likely to be associated with brain components involved in vision and perception (the optic lobe), cognition and assessment of risk (the forebrain), and physiological and motor responses (the brain stem and the cerebellum) (Paulin, 1993; Burish et al., 2004; Feenders et al., 2008). We therefore predict that the size of these individual brain components influences responses to perceived threats. For example, the forebrain is involved in cognition and learning, processes which underpin behavioral traits associated with habituation. Therefore, species with larger forebrains should show the greatest decrease in FID in urban environments. By contrast, species that are more likely to respond quickly to predators (i.e., greater FIDs overall) are likely to have greater perception abilities and a capacity to respond quickly (hence larger cerebellums, optic lobes, and brain stems). However, analyses of whole brain and brain components, and their link to FID, have yielded conflicting results. Møller and Erritzøe (2014) found that bird species with relatively larger brains overall had reduced FIDs, but that species with relatively larger cerebellums did indeed have longer FIDs. By contrast, Guay et al. (2013c) and Symonds et al. (2014) found no such patterns. The relationships of FID with brain size generally, and with specific brain components in particular, therefore remain unclear.
Despite the apparent lack of a relationship between relative whole brain size and the propensity to inhabit urban environments, there is greater intraspecific variation in brain sizes of species which have also colonized urban areas compared to those that have not, and such species exhibit greater behavioral plasticity (e.g., FIDs, Møller, 2010; Carrete and Tella, 2011; Møller and Erritzøe, 2015). Whilst Carrete and Tella (2011) considered relative whole brain size and FIDs (evoked by cars) in birds in rural vs. urban areas, to date no study has examined the influence of brain size components on the reduction in FID associated with living in urban habitats.
This study uses both whole brain and brain component size to examine whether brain size influences birds' tolerance of humans (as evoked by the commonest stimulus evident in urban areas, people). To investigate this, we examine FIDs of urban and rural populations of avian species, and used these values to generate a “degree of tolerance” exhibited by each species. We tested two predictions: (1) that species with longer histories of urbanization would exhibit a greater degree of tolerance (i.e., a bigger difference between FID in urban vs. rural populations) indicative of a local selection effect, and (2) that brain size and brain component sizes would also influence the extent of the degree of tolerance, due to larger brained species being more likely to habituate to a greater extent.
We also examined the way that the absolute values of FID in urban and rural populations of avian species differ in relation to brain and brain component sizes. If the cognitive buffer theory applies, we predict an interaction between habitat and brain size in predicting FID, such that (1) species with larger overall brain sizes will have smaller FIDs and (2) in species with larger brains there would be a greater difference in FID between rural and urban individuals (see Figure 1). This would provide evidence of larger-brained birds being able to habituate (decrease their FIDs) to humans to a greater extent in urban areas (Guay et al., 2013a).
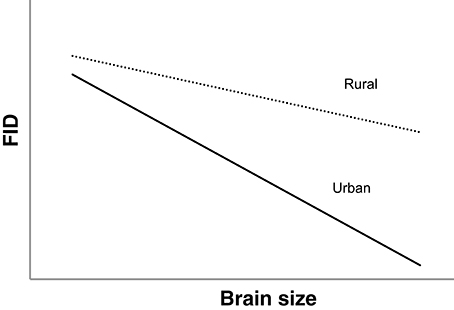
Figure 1. Predicted interspecific relationships between relative brain size and FID in rural (dotted line) and urban birds (solid line) if larger brained birds are better able to adjust to human presence. The pattern demonstrates an interaction between habitat and brain size in predicting FID.
Materials and methods
Data Collection
Data on FID, Starting Distance (SD, the distance at which an investigator approach started), and year of urbanization (first known year of breeding in an urban environment, as derived from observations and historical accounts) were sourced from Garamszegi et al. (2007) and Møller (2008). Data on FID and SD (in France and Denmark) from both the aforementioned sources were combined, as these measurements are repeatable between observers (Guay et al., 2013b; van Dongen et al., 2015a). We derived separate estimates of FID and SD for urban and rural populations of 42 species.
Data on brain and brain component masses were sourced from Mlíkovský (1989a,b,c, 1990) and Portmann (1947). Where brain volumes were reported, brain mass was calculated using the mean density of brain tissue (1.036 g/ml), as reported elsewhere (Iwaniuk and Nelson, 2002). The brain regions were forebrain, cerebellum, optic lobe (comprising the optic tectum and underlying structures, such as the inferior colliculus), and brain stem. Body masses were obtained from Dunning (2008). Although we were able to extract whole brain masses for all 42 species in our analysis, data on individual brain components was restricted to a subset of 25 species. Consequently, analyses examining the role of individual brain components on FID are restricted to this smaller data set.
FID, SD, body mass and brain masses/brain component masses were log transformed prior to analysis to better conform with assumptions of normality. We used the difference in FID values between rural and urban populations to generate a measure of “degree of tolerance” for each species (Glover et al., 2015). However, such a direct comparison of FID is complicated by the differences in SD between the two populations. To control for this, mean FIDs were then plotted against mean SDs for both rural and urban populations (SD is positively related to FID; Weston et al., 2012). The residual FID (ResFID) was then calculated for each species in both habitat types (i.e., ResFIDurban and ResFIDrural). A mean SD for each species (pooling both habitats) was then used to calculate predicted FID in each habitat, based on the coefficients of the correlation between FID and SD (predicted FID = 1.1958*MeanSD + ResFID). These values represented predicted FIDs for each species in each environment at a standardized starting distance. Predicted FIDs were then back transformed and used to calculate degree of tolerance, using the following formula:
Therefore, higher values indicate a greater degree of tolerance in urban habitats.
Comparative Analysis
As with earlier analyses of brain components and FID (Guay et al., 2013c; Symonds et al., 2014), we employed a phylogenetic comparative approach when analyzing our data, to control for potential non-independence of data due to shared ancestry among species. In this study, we obtained the phylogeny used as the basis for analysis from the “Global Phylogeny of Birds” website—www.birdtree.org (Jetz et al., 2012). Specifically, we downloaded a set of 2000 trees for our subsets of species from the pseudo-posterior distribution of trees using the Hackett et al. (2008) backbone. We then used this tree set to calculate a 50%-majority-rule consensus tree (see Figure 2) using the Mesquite package (Maddison and Maddison, 2010). We conducted our comparative analysis in two different ways. The first approach used degree of tolerance for each species as the response variable. The second approach employed the absolute FID data from two different populations (urban and rural) for each species as the response variable. In the second case we treated the populations as two separate “species” in our analysis. Consequently, we split each species tip in the phylogeny into two, separated by minimal branch length (length = 0.001) from their ancestral node.
We constructed phylogenetic generalized least squares (PGLS) models with brain size (or brain component sizes), body mass, and year of urbanization as predictors of degree of tolerance, and with SD and habitat as additional predictors in the analysis of FID. PGLS analysis was carried out using the caper package in R (Orme et al., 2012).
Because of the high degree of correlation (r>0.8) with body mass, we obtained measures of relative brain size (or relative brain component sizes) by calculating the residuals of the PGLS regression of brain size on body size, i.e., the observed value minus the predicted value from the PGLS regression of the log-transformed trait against log body mass. In the second analysis, to evaluate whether brain size influences the way that rural and urban populations differ in their FID responses, the interaction term between habitat and brain size was also evaluated in models. Likewise, an interaction between year of urbanization and habitat (urban/rural) was also included to assess the prediction that only urban populations should show a response in FID in relation to year of urbanization.
We used an information theoretic approach to analyse the explanatory power of our predictor variables in explaining FID. We conducted the analyses with two different data sets: using either the whole brain mass (42 species) or the 4 individual brain component masses (25 species) in combination with the other predictor variables. All PGLS model combinations of the predictor variables were compared using Akaike's Information Criterion controlled for small sample size (AICc, Burnham and Anderson, 2002; Symonds and Moussalli, 2011). Models with the lowest AICc score are held to be the best approximating models explaining the response variable. We calculated the relative support for models by examining the ΔAICc score (the difference in AICc score between the best approximating model and each other model in the candidate set), and also the Akaike weight (wi)—the probability that the model is the best model in the set). We compiled the list of top models (those models with ΔAIC < 2) with associated parameter estimates for each model. Influential effects were those where the 95% confidence interval around the parameter estimate did not cross zero (Burnham and Anderson, 2002). The evaluation of all models was achieved using the MuMIn package in R (Bartoń, 2014). In the analyses using brain components, because of the high co-linearity between these component masses, we did not include any models that involved combinations of components, just those using individual components, and their interaction terms with habitat.
For visual representation of relationships between FID, habitat, and year of urbanization or brain size, we calculated FID values for each species as the residuals from the PGLS model predicting log FID with log body mass and log SD as predictors.
Results
There was no effect of whole brain mass or individual brain component masses on degree of tolerance to human proximity (Table 1). The only clear predictor of degree of tolerance across species was year of urbanization, which was strongly negatively related to the degree of tolerance (Table 2). Species which have inhabited urban areas longer are more tolerant to human presence (Figure 3). In both analyses of degree of tolerance, the top model returned was the model with year of urbanization as the sole predictor.
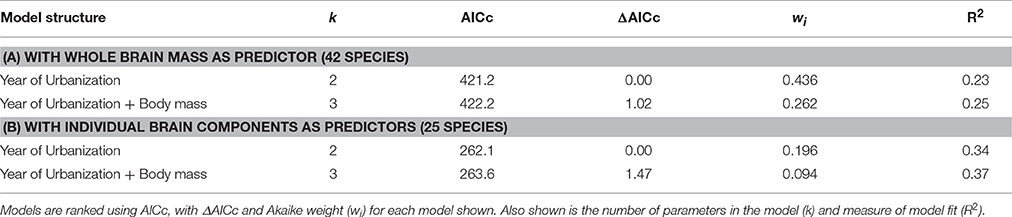
Table 1. The best-ranked PGLS regression models (models with ΔAICc < 2) predicting degree of tolerance across bird species.
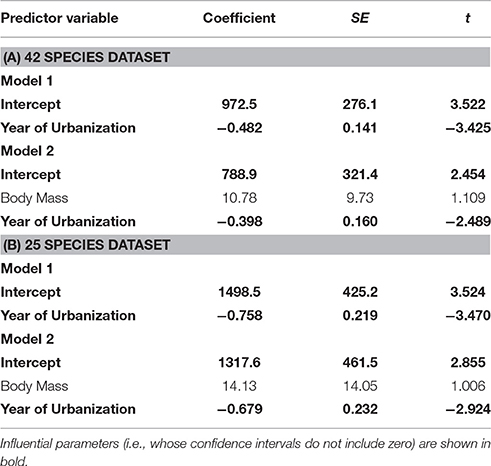
Table 2. Parameter estimates from models with ΔAICc < 2, explaining variation in degree of tolerance among birds.
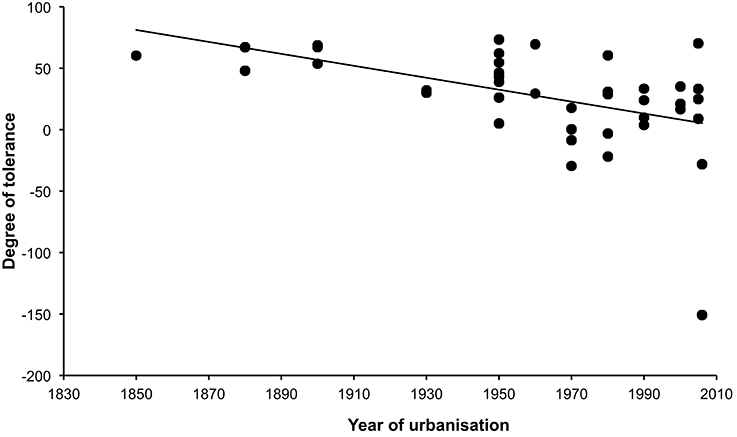
Figure 3. Relationship between degree of tolerance (see text) and year of urbanization in 42 bird species. Species that have a longer history of urbanization tend to show higher degrees of tolerance to humans (more positive values).
In the separate analysis of absolute FID values, all best approximating models featured SD, body mass, and habitat as strong predictors of FID (Table 3), the former two with positive effects, and the latter showing the clear effect that birds in urban populations have shorter FIDs than birds in rural populations (Table 4). In all top models, year of urbanization features as a predictor, although pooled across all species/populations, its individual effect has a parameter estimate whose confidence intervals include zero. However, there is a clear interaction between habitat and year of urbanization. Urban populations of birds have a positive association between FID and year of urbanization, with more recently urbanized bird populations showing longer FIDs. No association between year of urbanization and FID is evident for rural birds (Figure 4).
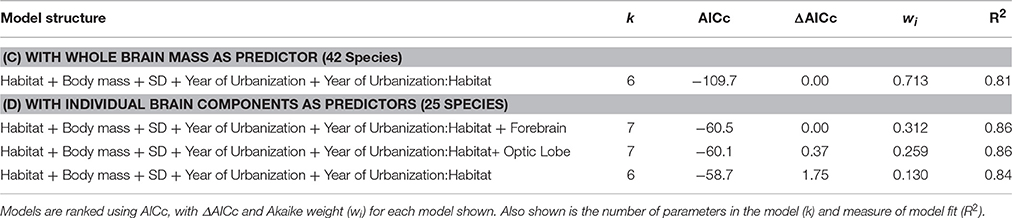
Table 3. The best-ranked PGLS regression models (models with ΔAICc < 2) predicting FID across bird species (including rural and urban populations of those species).
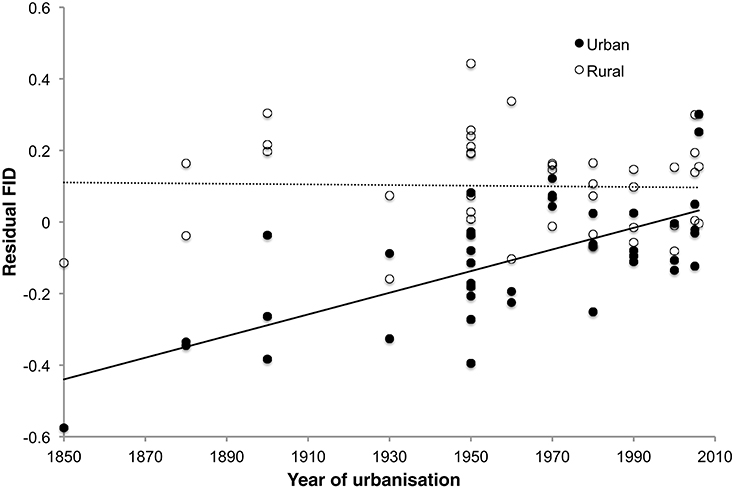
Figure 4. Relationship between FID and year of urbanization in rural and urban populations of 42 bird species. Urban populations of species that have a longer history of urbanization tend to show greater reductions of FID, but there is no such relationship in rural populations of the same species. FID values are the residuals from the regression of absolute log FID against log body mass and log starting distance.
Analysis using whole brain, brain stem or cerebellum masses revealed no direct effect of these variables on FID in the best models (Table 3). However, both forebrain and optic lobe mass appeared as positive predictors of FID in the best models, although their inclusion in models only explains an extra 2% of variance, indicating that their individual effects are weak (Table 4). In no case did an interaction term between brain size and habitat appear in the top models, indicating no evidence that larger-brained bird species show a greater discrepancy in FID between urban and rural populations. Figure 5 demonstrates the relationship between forebrain and optic lobe masses and FID, demonstrating the similar nature of the relationship for both rural and urban populations.
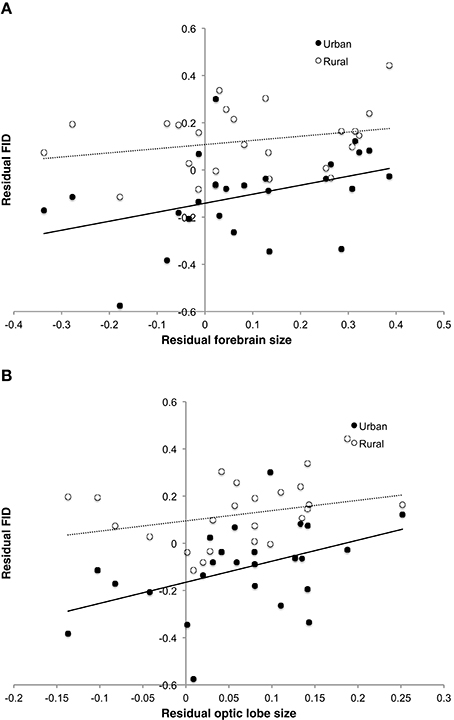
Figure 5. Relationship between FID and (A) forebrain size and (B) optic lobe size in both rural and urban populations of 25 bird species. While rural populations, and species with greater brain component masses, have longer FIDs, there is no difference between populations in the relationship of brain component sizes to FID. FID values are the residuals from the regression of absolute log FID against log body mass and log starting distance. Brain size values are the residuals of the log transformed trait mass against log body mass.
Discussion
Urbanization is associated with reduced responsiveness to, i.e., increased tolerance of, humans (Møller, 2010; this study). As has also been previously reported, the longer a species has been exposed to urbanization the more tolerance to humans is evident (Møller, 2008, 2010; but see Gendall et al., 2015). This pattern of increase in tolerance over time suggests selection is acting whereby more responsive individuals die or do not breed, or less responsive individuals have some other fitness benefits in urban settings, although it may possibly also involve historical founder effects if bolder birds initially instigated colonization of urban areas (Møller, 2010; Weston et al., 2012; van Dongen et al., 2015b). Human behavior, especially the occurrence of hunting in rural areas, may differ between habitats and influence FID (Magige et al., 2009; Sreekar et al., 2015). Alternatively, site selection by birds may involve more responsive individuals moving away from, or not settling in, urban areas (van Dongen et al., 2015b). The relationship between time since urbanization and tolerance, and more specifically the fact that rural birds have apparently not altered their responsiveness through time while urban birds have exhibited a decrease in responsiveness, strengthens the idea that it is specifically urbanization, and not some other variable, that has driven the increased degree of tolerance in species that have longer histories of urbanization. The linear decrease in FIDs through time for urban birds suggests that tolerance appears to occur across generations, and defies the somewhat commonly held view that it is solely learning within individuals (habituation) that is the principle mechanism through which tolerance of humans increases (see Weston et al., 2012).
Encephalisation had no apparent effect on tolerance, either as measured through degree of tolerance or through the interaction between habitat and brain size in predicting FID. These results suggest that habituation, if it also does explain to some extent the increased tolerance in urban birds, is not being mediated by brain size. Three potential influences may explain the lack of an effect. First, our measures of brain size and FID did not adequately characterize intraspecific variation in these traits. Brain sizes vary within populations of birds and whilst mean brain size may be equal, the distribution of brain sizes (standard deviation and skewness) can differ amongst populations and may influence tolerance of humans intraspecifically (Møller and Erritzøe, 2015). Given the high neural density in avian brains, small differences in size may represent substantial differences in cognitive abilities (Olkowicz et al., 2016). Therefore, whilst a relationship between brain size and tolerance may not be detected among species, it may exist within species between individuals (Blumstein, in press). Second, fear is not the sole driver of brain architecture or size. Urban founder effects and selective pressures, including, among other influences, the nutritional requirements associated with growing and maintaining larger brains, are also likely to influence relative bird brain size in urban vs. rural habitats (Møller and Erritzøe, 2015). Finally, studies of whole brain size (and less commonly brain component size) and FID in birds indicate contrasting results linking escape behavior to the size of brain structures (Guay et al., 2013c; Møller and Erritzøe, 2014; Symonds et al., 2014). Hence any effects of encephalisation on the reduction in FID may be difficult to discern, and may reflect the inherent difficulties and generalizations of relating complex behaviors with specific areas of the brain (Healy and Rowe, 2007). Interestingly, decreased fear of humans has co-evolved with decreased brain size in domestic birds (Desforges and Wood-Gush, 1975; Ebinger and Löhmer, 1985, 1987), perhaps mediated by a relatively stronger selection for atrophy of the optical processing areas of the brain as compared to the overall brain mass (Ebinger and Löhmer, 1987) and/or a decreased eye size in domestic birds (Ebinger et al., 1989).
The finest level of neural anatomy available to us across species (brain components) uncovered a generally positive relationship between forebrain mass, optic lobe mass, and FID. Larger forebrains in birds have been linked to behavioral flexibility, such as foraging innovations (Lefebvre et al., 1998; Nicolakakis and Lefebvre, 2000), leading us to expect that larger forebrains could be linked with moderation of FIDs where humans are common and benign. However, contrary to our predictions we found that whilst larger forebrains were associated with longer FIDs among species in general, they were not associated with the degree of decrease in FIDs in urban populations. Perhaps larger forebrains underpin threat perception at greater distances, and this overrides any effects of enhanced risk assessment and moderated response. Forebrain size is subject to ecological constraints and is implicated in major life history differences between birds (e.g., longer-migrating species have smaller forebrains; Winkler et al., 2004; Sol et al., 2005b), and thus is subject to a variety of selective pressures which vary among species, and which we did not include in these analyses. The role of forebrain size, if any, in moderation of escape behaviors in birds warrants further investigation.
FID is positively correlated with eye size (Møller and Erritzøe, 2010), and the optic lobe in birds increases in size in relation to eye size (Brooke et al., 1999). It has been suggested, based on flight speeds, that larger eyes (and therefore optic lobes) enable detection of stimuli at greater distances (Brooke et al., 1999), which is consistent with the longer FIDs we found here, but again there is no evidence that optic lobe size plays a role in determining the extent to which species reduce their FID in urban environments. Whether the acuity of vision varies between species at the starting distances and stimulus (humans) used in this study remains unclear.
Our results therefore indicate an evolutionary response in FID to urban-living in birds, such that tolerance has increased over historical time, as is shown by the finding that bird species with longer histories of urbanization exhibit a greater degree of tolerance. However, although we find some evidence that brain component (forebrain, optic lobe) sizes are linked with FID response in general among the species studied, there is no evidence that increased encephalisation influences the extent to which tolerance of humans has developed in urban populations of birds. Comparisons of fine-scale brain structures of birds inhabiting environments with different prevailing human regimes may uncover subtler differences.
Author Contributions
Conceived study: MS, PG, MW, WvD, AL. Collected data: PG, WvD, MW. Analysed data: MS, WvD, PG, MW. Contributed funding and materials RR. Wrote the paper: MS, PG, MW, WvD, AL.
Funding
This research was supported by funding from Melbourne Water Corporation and a Victoria University Fellowship and a Faculty of Health Engineering and Science Collaborative Research Grant Scheme to PG.
Conflict of Interest Statement
The authors declare that the research was conducted in the absence of any commercial or financial relationships that could be construed as a potential conflict of interest.
Supplementary Material
The Supplementary Material for this article can be found online at: http://journal.frontiersin.org/article/10.3389/fevo.2016.00117
References
Bartoń, K. A. (2014). MuMIn: Multi-Model Inference. Available online at: http://mumin.r-forge.r-project.org
Blumstein, D. T. (in press). Habituation sensitization: new thoughts about old ideas. Anim. Behav. doi: 10.1016/j.anbehav.2016.05.012.
Brooke, M. D. L., Hanley, S., and Laughlin, S. B. (1999). The scaling of eye size with body mass in birds. Proc. R. Soc. Lond. B Biol. Sci. 266, 405–412.
Burish, M. J., Kueh, H. Y., and Wang, S. S.-H. (2004). Brain architecture and social complexity in modern and ancient birds. Brain Behav. Evol. 63, 107–124. doi: 10.1159/000075674
Burnham, K. P., and Anderson, D. R. (2002). Model Selection and Multimodel Inference, 2nd Edn. New York, NY: Springer.
Carrete, M., and Tella, J. L. (2011). Inter-individual variability in fear of humans and relative brain size of the species are related to contemporary urban invasion in birds. PLoS ONE 6:e18859. doi: 10.1371/journal.pone.0018859
Desforges, M. F., and Wood-Gush, D. G. M. (1975). A behavioural comparison of domestic and Mallard ducks: habituation and flight reactions. Anim. Behav. 23, 692–697. doi: 10.1016/0003-3472(75)90145-1
Ebinger, P., and Löhmer, R. (1985). Relationship of brain weight and body weight in Mallards and domestic ducks. Zool. Anz. 214, 285–290.
Ebinger, P., and Löhmer, R. (1987). A volumetric comparison of brains between greylag geese (Anser anser L.) and domestic geese. J. Hirnforsch. 28, 291–299.
Ebinger, P., Röhrs, M., and Pohlenz, J. (1989). Reductions of brain and eye weight in the wild and domestic turkey (Meleagris gallopavo). Z. Zool. Syst. Evolut-forsch. 27, 142–148. doi: 10.1111/j.1439-0469.1989.tb00339.x
Evans, K. L., Chamberlain, D. E., Hatchwell, B. J., Gregory, R. D., and Gaston, K. J. (2011). What makes an urban bird? Glob. Change Biol. 17, 32–44. doi: 10.1111/j.1365-2486.2010.02247.x
Feenders, G., Liedvogel, M., Rivas, M., Zapka, M., Horita, H., Hara, E., et al. (2008). Molecular mapping of movement-associated areas in the avian brain: a motor theory for vocal learning origin. PLoS ONE 3:e1768. doi: 10.1371/journal.pone.0001768
Garamszegi, L. Z., Erritzøe, J., and Møller, A. P. (2007). Feeding innovations and parasitism in birds. Biol. J. Linn. Soc. 90, 441–455. doi: 10.1111/j.1095-8312.2007.00733.x
Gendall, J., Lill, A., and Beckman, J. (2015). Tolerance of disturbance by humans in long-time resident and recent colonist urban doves. Avian Res. 6, 7. doi: 10.1186/s40657-015-0018-x
Glover, H. K., Guay, P.-J., and Weston, M. A (2015). Up the creek with a paddle; avian flight distances from canoes versus walkers. Wetl. Ecol. Manag. 23, 775–778. doi: 10.1007/s11273-015-9411-9
Gravolin, I., Key, M., and Lill, A. (2014). Boldness of urban Australian magpies and local traffic volume. Avian Biol. Res. 7, 244–250. doi: 10.3184/175815514X14151981691872
Guay, P.-J., and Iwaniuk, A. N. (2008). Interspecific variation in relative brain size is not correlated with intensity of sexual selection in waterfowl (Anseriformes). Aust. J. Zool. 56, 311–321. doi: 10.1071/ZO08082
Guay, P.-J., Lorenz, R. D. A., Robinson, R. W., Symonds, M. R. E., and Weston, M. A. (2013a). Distance from water, sex and approach direction influence flight distances among habituated black swans. Ethology 119, 552–558. doi: 10.1111/eth.12094
Guay, P.-J., McLeod, E. M., Cross, R., Formby, A. J., Maldonado, S. P., et al. (2013b). Observer effects occur when estimating alert but not flight-initiation distances. Wildlife Res. 40, 289–293. doi: 10.1071/WR13013
Guay, P.-J., Weston, M. A., Symonds, M. R. E., and Glover, H. K. (2013c). Brains and bravery: little evidence of a relationship between brain size and flightiness in shorebirds. Austral Ecol. 38, 516–522. doi: 10.1111/j.1442-9993.2012.02441.x
Hackett, S. J., Kimball, R. T., Reddy, S., Bowie, R. C. K., Braun, E. L., Braun, M. J., et al. (2008). A phylogenomic study of birds reveals their evolutionary history. Science 320, 1763–1768. doi: 10.1126/science.1157704
Healy, S. D., and Rowe, C. (2007). A critique of comparative studies of brain size. Proc. R. Soc. Lond. B Biol. Sci. 274, 453–464. doi: 10.1098/rspb.2006.3748
Iwaniuk, A. N., and Nelson, J. E. (2002). Can endocranial volume be used as an estimate of brain size in birds? Can. J. Zool. 80, 16–23. doi: 10.1139/z01-204
Jetz, W., Thomas, G. H., Joy, J. B., Hartmann, K., and Mooers, A. O. (2012). The global diversity of birds in space and time. Nature 491, 444–448. doi: 10.1038/nature11631
Kark, S., Iwaniuk, A., Schalimtzek, A., and Banker, E. (2007). Living in the city: can anyone become an ‘urban exploiter’? J. Biogeogr. 34, 638–651. doi: 10.1111/j.1365-2699.2006.01638.x
Kitchen, K., Lill, A., and Price, M. (2010). Tolerance of human disturbance by urban magpie-larks. Aust. Field Ornithol. 27, 1–9.
Kotrschal, A., Lievens, E. J. P., Dahlbom, J., Bundsen, A., Semenova, S., Sundvik, M., et al. (2014). Artificial selection on relative brain size reveals a positive genetic correlation between brain size and proactive personality in the guppy. Evolution 68, 1139–1149. doi: 10.1111/evo.12341
Lefebvre, L., Gaxiola, A., Dawson, S., Timmermans, S., Rosza, L., and Kabai, P. (1998). Feeding innovations and forebrain size in Australasian birds. Behaviour 135, 1077–1097. doi: 10.1163/156853998792913492
Madden, J. (2001). Sex, bowers and brains. Proc. R. Soc. Lond. B Biol. Sci. 268, 833–838. doi: 10.1098/rspb.2000.1425
Maddison, W., and Maddison, D. (2010). Mesquite: A Modular System for Evolutionary Analysis. Version 2.74. Available online at: http://www.mesquiteproject.org/mesquite/download/download.html
Magige, F. J., Holmern, T., Stokke, S., Mlingwa, C., and Røskaft, E. (2009). Does illegal hunting affect density and behaviour of African grassland birds? A case study on ostrich (Struthio camelus). Biodivers. Conserv. 18, 1361–1373. doi: 10.1007/s10531-008-9481-6
McGiffin, A., Lill, A., Beckman, J., and Johnstone, C. P. (2013). Tolerance of human approaches by the common myna along an urban-rural gradient. Emu 113, 154–160. doi: 10.1071/MU12107
Mlíkovský J. (1989a). Brain size in birds: 1. Tinamiformes through Ciconiiformes. Věst. Čs. Společ. Zool. 53, 33–47.
Mlíkovský, J. (1989b). Brain size in birds: 2. Falconiformes through Gaviiformes. Věst. Čs. Společ. Zool. 53, 200–213.
Mlíkovský, J. (1989c). Brain size in birds: 3. Columbiformes through Piciformes. Věst. Čs. Společ. Zool. 53, 252–264.
Møller, A. P. (2008). Flight distance of urban birds, predation, and selection for urban life. Behav. Ecol. Sociobiol. 63, 63–75. doi: 10.1007/s00265-008-0636-y
Møller, A. P. (2010). Interspecific variation in fear responses predicts urbanization in birds. Behav. Ecol. 21, 365–371. doi: 10.1093/beheco/arp199
Møller, A. P., and Erritzøe, J. (2010). Flight distance and eye size in birds. Ethology 116, 458–465. doi: 10.1111/j.1439-0310.2010.01754.x
Møller, A. P., and Erritzøe, J. (2014). Predator-prey interactions, flight initiation distance and brain size. J. Evol. Biol. 27, 34–42. doi: 10.1111/jeb.12272
Møller, A. P., and Erritzøe, J. (2015). Brain size and urbanization in birds. Avian Res. 6, 8. doi: 10.1186/s40657-015-0017-y
Nicolakakis, N., and Lefebvre, L. (2000). Forebrain size and innovation rate in European birds: feeding, nesting and confounding variables. Behaviour 137, 1415–1429. doi: 10.1163/156853900502646
Olkowicz, S., Kocourek, M., Lučan, R. K., Porteš, M., Fitch, W. T., Herculano-Houzel, S., et al. (2016). Birds have primate-like numbers of neurons in the forebrain. Proc. Natl. Acad. Sci. U.S.A. 113, 7255–7260. doi: 10.1073/pnas.1517131113
Orme, D., Freckleton, R., Thomas, G., Petzoldt, T., Fritz, S., Isaac, N., et al. (2012). Caper: Comparative Analyses of Phylogenetics and Evolution in R. R package version 0.5. Available online at: http://CRAN.R-project.org/package=caper
Paulin, M. G. (1993). The role of the cerebellum in motor control and perception. Brain Behav. Evol. 41, 39–50.
Portmann, A. (1947). Études sur la cérébralisation chez les oiseaux - II. - Les indices intra-cérébraux. Alauda 15, 1–15.
Sol, D. (2009). Revisiting the cognitive buffer hypothesis for the evolution of large brains. Biol. Lett. 5, 130–133. doi: 10.1098/rsbl.2008.0621
Sol, D., Duncan, R. P., Blackburn, T. M., Cassey, P., and Lefebvre, L. (2005a). Big brains, enhanced cognition, and response of birds to novel environments. Proc. Natl. Acad. Sci. U.S.A. 102, 5460–5465. doi: 10.1073/pnas.0408145102
Sol, D., Lefebvre, L., and Rodríguez-Teijeiro, J. D. (2005b). Brain size, innovative propensity and migratory behaviour in temperate Palaearctic birds. Proc. R. Soc. Lond. B Biol. Sci. 272, 1433–1441. doi: 10.1098/rspb.2005.3099
Sreekar, R., Goodale, E., and Harrison, R. (2015). Flight initiation distance as a behavioral indicator of hunting pressure: a case study of the sooty-headed bulbul (Pycnonotus aurigaster) in Xishuangbanna, SW China. Trop. Conserv. Sci. 8, 505–512.
Symonds, M. R. E., and Moussalli, A. (2011). A brief guide to model selection, multimodel inference and model averaging in behavioural ecology using Akaike's information criterion. Behav. Ecol. Sociobiol. 65, 13–21. doi: 10.1007/s00265-010-1037-6
Symonds, M. R. E., Weston, M. A., Robinson, R. W., and Guay, P.-J. (2014). Comparative analysis of classic brain component sizes in relation to flightiness in birds. PLoS ONE 9:e91960. doi: 10.1371/journal.pone.0091960
van Dongen, W. F. D., McLeod, E. M., Mulder, R. A., Weston, M. A., and Guay, P.-J. (2015a). The height of approaching humans does not affect flight-initiation distance. Bird Study 62, 285–288. doi: 10.1080/00063657.2015.1026309
van Dongen, W. F. D., Robinson, R. W., Weston, M. A., Mulder, R. A., and Guay, P.-J. (2015b). Variation at the DRD4 locus is associated with wariness and local site selection in urban black swans. BMC Evol. Biol. 15:253. doi: 10.1186/s12862-015-0533-8
Vines, A., and Lill, A. (2015). Boldness and urban dwelling in little ravens. Wildlife Res. 42, 590–597. doi: 10.1071/WR14104
Weston, M. A., McLeod, E. M., Blumstein, D. T., and Guay, P.-J. (2012). A review of flight-initiation distances and their application to managing disturbance to Australian birds. Emu 112, 269–286. doi: 10.1071/MU12026
Winkler, H., Leisler, B., and Bernroider, G. (2004). Ecological constraints on the evolution of avian brains. J. Ornithol. 145, 238–244. doi: 10.1007/s10336-004-0040-y
Keywords: brain size, flight initiation distance, habituation, optic lobe, phylogenetic generalized least squares regression, urbanization
Citation: Symonds MRE, Weston MA, van Dongen WFD, Lill A, Robinson RW and Guay P-J (2016) Time Since Urbanization but Not Encephalisation Is Associated with Increased Tolerance of Human Proximity in Birds. Front. Ecol. Evol. 4:117. doi: 10.3389/fevo.2016.00117
Received: 20 July 2016; Accepted: 20 September 2016;
Published: 04 October 2016.
Edited by:
Amanda D. Rodewald, Cornell University, USAReviewed by:
Shawn M. Wilder, Oklahoma State University–Stillwater, USAKarl L. Evans, University of Sheffield, UK
Copyright © 2016 Symonds, Weston, van Dongen, Lill, Robinson and Guay. This is an open-access article distributed under the terms of the Creative Commons Attribution License (CC BY). The use, distribution or reproduction in other forums is permitted, provided the original author(s) or licensor are credited and that the original publication in this journal is cited, in accordance with accepted academic practice. No use, distribution or reproduction is permitted which does not comply with these terms.
*Correspondence: Matthew R. E. Symonds, bWF0dGhldy5zeW1vbmRzQGRlYWtpbi5lZHUuYXU=