- 1Department of Environmental Sciences, University of Helsinki, Lahti, Finland
- 2Division of Biology, Kansas State University, Manhattan, KS, USA
Urban green spaces provide ecosystem properties fundamental to the provision of ecosystem services, such as the sequestration of carbon and nutrients and serving as a reservoir for organic matter. Although, urban vegetation influences soil physico-chemical properties, it remains unknown whether ecosystem properties depend on plant species portfolios. We tested the influence of three common functional plant groups (evergreen trees, deciduous trees, grass/lawn) for their ability to modify soils in parks of various ages under cold climatic conditions in Finland. We hypothesized that (i) plant functional groups affect soils differently resulting in divergent ecosystem properties, and (ii) that these ecosystem properties also depend on park age. We included 41 urban parks of varying ages (10, 50, and >100 years) and additional control forests. Park soils were sampled for physico-chemical parameters up to 50 cm depth. Our data indicate that plant functional groups modify soils differently, especially between the evergreen and lawn treatments at 50 and >100 year old parks. Soils under evergreen trees had the lowest pH and generally the highest percentage organic matter, percentage total carbon and percentage total nitrogen. Soil pH remained the same, whereas concentrations of organic matter, total carbon and total nitrogen declined by depth. Soils in the reference forests had lower pH but higher percentages organic matter, total carbon, and total nitrogen than those in parks. We estimate that old parks with evergreen trees can store 35.5 and 2.3 kg N m−2—considerably more than in urban soils in warmer climates. Our data suggest that plant-soil interactions in urban parks, in spite of being constructed environments, are surprisingly similar to those in natural forests.
Introduction
Soils, especially those in natural or semi-natural ecosystems, are increasingly recognized for the ecosystem services they provide (Barrios, 2007; Wall et al., 2015). Besides providing a substrate for plant growth, soils are vital in, e.g., the provision of clean water (Valtanen et al., 2014), recharging groundwater reservoirs (Glynn and Plummer, 2005), detoxification of harmful substances (Lehmann and Stahr, 2007), and storing soil organic matter (carbon and nitrogen pools; Franzluebbers, 2002; Lal, 2004). Many of these ecosystem services are directly or indirectly linked to human health (Wall et al., 2015), emphasizing the importance of soils for humans.
Urban sprawl and densification are increasing urban land use rapidly, drastically affecting soils and their function (Pickett et al., 2001; Byrne, 2007; Pavao-Zuckerman, 2008; Pouyat et al., 2010). As urban land use can be equated to soil use (Pouyat et al., 2007a; Setälä et al., 2014), urbanization not only modifies soil structure but also reduces the soil area/volume. Sites at which productive soils have been lost or replaced by functionally altered soils such as “made lands” (Pouyat et al., 2002; Pavao-Zuckerman, 2008) are often characterized by diminished organic matter (OM), carbon (C), and nutrient stocks (Craul and Klein, 1980; Short et al., 1986). Since soil C content relates to soil function and resultant ecosystem services (Franzluebbers, 2002), C-devoid urban soils may provide fewer benefits than those unaltered by humans (Pouyat et al., 2010). However, when subjected to minor disturbances, e.g., construction of residential gardens or urban green spaces, urban soils can sequester even more carbon than native soils (Pouyat et al., 2002, 2009; Qian and Follett, 2002; Kaye et al., 2005; Frank et al., 2006; Golubiewski, 2006; Raciti et al., 2011; Edmondson et al., 2014a). This high C and N storage is typical of intensively managed turf grass (i.e., lawn), but soils under urban trees also store C and other soil elements (Edmondson et al., 2014a,b; Bae and Ryu, 2015; Livesley et al., 2016). Indeed, theory predicts and evidence shows that the type and biomass of natural and semi-natural vegetation control soil formation, its OM content, plus the composition and activity of the below-ground food web (Wardle, 2002; Ponge, 2003; Bardgett and Wardle, 2010). Consequently, simple and diminished plant communities in urban systems (Pickett et al., 2001; Alberti, 2008; Cilliers and Siebert, 2011) also modify urban brown spaces (i.e., soils, sensu Allison, 2006; Pouyat et al., 2007a) and their function. Supporting this, previous research suggests that altered plant biomass and cover, and the concomitant changes in plant litter can cascade down to urban soils, their decomposers, and organismal life-supporting processes (see Pavao-Zuckerman and Coleman, 2007; Vauramo and Setälä, 2010; Ossola et al., 2015).
The functional attributes of dominant plants (sensu Grime, 1974, 1998) strongly influence soil formation and the function of the plant-soil system (Cornwell et al., 2008; Austin and Zanne, 2015). For example, fast and slow-growing plants differ in litter that boosts the formation of either bacterial or fungal food webs (Moore and Hunt, 1988; Wardle et al., 2004; Bardgett and Wardle, 2010). Depending on the relative dominance of the plant functional groups and the two soil microbial groups, decomposition and thus soil organic matter accumulation differ among systems with such food webs (Meentemeyer, 1978; Coleman et al., 1983; Ponge, 2003; Paterson et al., 2008). Consequently, plant types producing labile litter are prone to faster C and N loss than e.g., slow-growing conifers that produce recalcitrant litter (Wardle et al., 2004; Bardgett and Wardle, 2010). Currently, only little information is available on the effects of plant functional groups on belowground processes in urban soils (but see Vauramo and Setälä, 2010, 2011; Edmondson et al., 2014b; Ossola et al., 2015). To our knowledge, studies that explicitly explore the effects of plant functional groups on urban park soils are non-existent.
The age of individual plants as well as the successional stage of the ecosystem influence plant-soil interactions (Kardol et al., 2006; Gough et al., 2008). The longer plants and soil interact, the stronger the coupling between the two in the rhizosphere (De Deyn et al., 2008) and the more likely the plant driven phenomena to manifest in the soil (Wardle, 2002). In urban systems, old soils, mostly home-yard lawns, may sequester more C and N than recently established home-yards (Qian and Follett, 2002; Frank et al., 2006; Golubiewski, 2006; Pouyat et al., 2009; Raciti et al., 2011). Little information is available on the impacts of vegetation or park age, other than for grasses/lawns, on C and N sequestration in urban greenspace soils. Some observational studies suggest that soil C content is not directly correlated with tree age in public parks (Edmondson et al., 2014b) or park age itself (Livesley et al., 2016), emphasizing the need for rigorous, well-designed studies of both vegetation and the age of urban greenspace on soil properties.
Similarly to virtually all terrestrial ecosystems (Franzluebbers, 2002), urban greenspaces are typified by soils that accumulate organic matter in the topmost layer and a clear decline in %OM and %C with increasing soil depth (Kaye et al., 2005; Raciti et al., 2011; Edmondson et al., 2014a,b). As a result, soil OM and its importance in soil functioning (Franzluebbers, 2002; Lal, 2010) make surface soils primary drivers of soil-derived ecosystem services—also in urban settings (Lorenz and Lal, 2009; Edmondson et al., 2012). Although, deeper soil layers may also store considerable amounts of C and N in home-yards and other urban turf-grass habitats (Pouyat et al., 2010), little is known about how plant species or greenspace age influence the distribution of OM, C and N in urban soil profiles. However, soil macrofauna, e.g., earthworms, potentially mix urban soils (see Lavelle, 1988; Edwards and Bohlen, 1996; Pouyat et al., 2002) leading to less obvious stratification of OM and nutrients with increasing age, simply because soil mixing by earthworms may take decades or even centuries (Edwards and Bohlen, 1996).
Although, urban home-yards and other intensively managed soils in the USA can sequester considerable amounts of C and N (Easton and Petrovic, 2008; Groffman et al., 2009; Pouyat et al., 2010; Raciti et al., 2011), few have studied this topic in European cities (see Edmondson et al., 2012, 2014b). The control of C and N sequestration in urban greenspaces by plants differing in eco-physiology and whether these effects depend on the age of the greenspace remain virtually unknown. We aimed to study the potential of three plant functional groups (evergreen or deciduous trees and lawns) to modify soils and their properties in 41 urban parks of varying age (5 to over 200 years old) under northern climatic conditions in Europe. We highlight that our study is rigorously replicated and provides adequate statistical power to evaluate plant and park age effects without pseudoreplication—a challenge in many studies focusing on urban systems. To compare the urban systems to others with little anthropogenic impacts, we also included natural and semi-natural mixed forests with evergreen (Norway spruce—Picea abies L.) and deciduous (forest linden—Tilia cordata L.) trees, but without lawn, as control sites. Our goal was to test the following three primary hypotheses:
(1) The three vegetation types, being dominant in parks in southern Finland, differ in their ability to modify the soil biogeochemistry (here pH, %OM, %C, and %N and the C/N ratio). These differences translate to variation in soil properties and processes among the vegetation types as a result of fundamental differences in the decomposability of the litter that they produce (Bardgett and Wardle, 2010). Further, rooting depths of the two tree types (deciduous and evergreen) are much greater than those of grasses (Jobbagy and Jackson, 2000), likely manifesting as diverging C and N concentrations in the soil profile.
(2) Urban park soil C and N storage depend on park age (time since park establishment—from here on “park age”) because old trees in old parks span decades or even more than a century to modify soils, whereas young trees in recent parks lack such history.
(3) Soil texture, chemistry and organic matter content are more stratified in young parks than in older parks. This is because in the former systems, roots of the young plants have not reached deeper soil layers and have been unable to deposit root-derived OM throughout the soil profile.
Prevailing urban ecological “theory” suggests that processes in urban green spaces, driven by socio-ecological actions (Grimm et al., 2000; Pickett et al., 2001; Alberti, 2008), should differ from those of more natural systems. Consequently, we predict that the biogeochemistry of the soil, even in old urban parks, differs from that of nearby natural boreal forest habitats of similar age. This is because succession of the managed, constantly disturbed urban park ecosystems differs from that in natural forests, typified by the constant accumulation of soil OM in the uppermost layers due to slow decomposition (Aerts and Chapin, 2000) and the scarcity of larger earthworm taxa (H. Setälä, personal observations) capable of soil mixing (see Carreiro et al., 2009).
Materials and Methods
Site Description
A total of 41 parks, belonging to the Finnish LTSER-site network (Forsius et al., 2013), were chosen on the basis of their age [young parks (7–15), intermediate parks (ca. 50 ± 10 years) and old parks (>100 years old, the oldest ones established more than two centuries ago), data on the approximate ages of parks were provided by the Helsinki City Public Works Department and by the Technical and Environmental Services of the city of Lahti] in two cities in southern Finland: Helsinki (60°10′15″N, 024°56′15″E, population size of ca. 1.4 million people) and Lahti (60°59′00″N, 25°39′20″E, population size of 102,000). In both cities, five parks of each age were selected so that each of the parks were—ideally—represented by three vegetation types, i.e., evergreens (Norway spruce [Picea abies]), deciduous trees (Linden [Tilia × vulgaris]), and lawn species (mostly Poa and Festuca species with scattered herbs such as Trifolium pratence and Plantago major). In a few cases it was not possible to find each of the three plant functional groups within the same park forcing us to choose some parks with only one or two vegetation types present. This resulted in a total of 41 study parks across the two cities with at least five replicates per plant treatment per park age. Furthermore, in two parks in Lahti and one in Helsinki, Norway maple (Acer platanoides L) instead of linden, and in three parks in Helsinki, Scots pine (Pinus sylvestris L) instead of spruce were chosen. The size of the parks varied considerably, from ca. 0.1 ha to some ha in size. The parks were frequently mowed without irrigation, fertilizer or pesticide additions. In the city of Lahti, parks belonging to the three age-classes were randomly distributed within the city, while in Helsinki the oldest parks tended to be closer to the urban core than the intermediate and young parks. Five additional forest sites (control) in the proximity of the city of Lahti were included in the experimental design. These unmanaged control forests (several ha in size) are mature (>60 year old) mixed forests dominated by Norway spruce and small-leaved linden (Tilia cordata) without lawn.
During park construction, young and intermediate parks were topped with a 10–20 cm layer of new soil (either a mixture of leaf compost, peat and sand, or mull derived from adjacent arable fields, Markku Saari, personal communication) immediately before planting the trees/lawn. No data are available on the establishment of old parks. Tree age represents the age of the park—trees visibly younger than the age of the park were disregarded. In the case of poor grass survival, some of the old parks in Helsinki and Lahti occasionally received a shallow top layer of fine sand and/or garden soil. In each park, independent of their age, fallen leaves and needles are raked and removed in the fall and again in the spring at the start of the growing season in May. Lawn clippings are not removed after mowing.
The mean annual temperature and average precipitation in Helsinki is 5.3°C and 682 mm, and in Lahti 4.5°C and 636 mm, respectively. In southern Finland, winter lasts 135–145 days, summer 110–120 days, and temperatures can vary between −35 and +35°C within a year (Finnish Meteorological Institute: http://en.ilmatieteenlaitos.fi/; verified 12 March, 2015). Helsinki and Lahti metropolitan regions are classified by NRCS as having Udic moisture and Mesic/Cryic temperature soil regimes, respectively. The bedrock in Helsinki consists of microcline granite and soil of the sites consists primarily of homogeneous clay and silt stratification. In Lahti, the bedrock is characterized by mica schist and mica gneiss as well as microcline granite. Soil at the Lahti sites is mostly gravel and sand till. The Salpausselkä esker, which is a terminal moraine formed in the latest ice age during the Younger Dryas era 12,000 BP, typifies the landscape in the southern part of Lahti where about half of the parks reside. The Helsinki and Lahti metropolitan regions are classified by NRCS as having soils of primarily the Spodosol suborder. However, all urban parks in the two cities are constructed showing no signs of podsolization. It is noteworthy that each park was covered with lawn; even the soils sampled underneath tree canopies (see below) had a lawn understory.
Soil Sampling
Soils were sampled in May 2015 (control forests) and October 2014 (parks) underneath the canopy projection (evergreen and deciduous trees) so that distance to the nearest tree trunk ranged from 1 m (young trees in young parks) to several meters (old trees in old parks). The lawn soils (in parks only) were sampled at least at a distance equal to or greater than the height of the nearest tree to minimize the effect of adjacent trees and their roots. The samples were collected using a metal soil corer (2.54 cm diameter) to a depth of 50 cm. The soil samples were divided vertically into 0–10, 11–20, and 21–50 cm sections and transported to the laboratory for further analyses. The soils were sieved through a 2 mm sieve to remove larger mineral particles, roots and other organic debris and analyzed for soil dry mass (after drying for 48 h at 105°C), %OM (loss on ignition, 5 h at 550°C in a muffle oven), soil pH (1:5 v/v, fresh soil/distilled water), and total carbon (tot-C) and -nitrogen (tot-N) by dry combustion at 1350°C using a LECO CNS-2000 Elemental Analyzer (0.07% C and 0.09% N detection limits). Because of the low carbonate concentration in northern soils, we considered it unnecessary to quantify the proportion of inorganic-C of tot-C. As a result, tot-C is considered analogous to organic-C in the current study. Altogether 270 park soil samples (2 cities × 3 park age classes × 3 vegetation types × 3 soil depths × 5 replicates) and 20 forest soil samples (1 city × 1 age × 2 vegetation types × 2 soil depth × 5 replicates) were analyzed. Only the uppermost 0–10 cm and 11–20 cm soil layers were sampled in the control forests because of rocky soil that made deeper cores impossible.
Statistical Analysis
All statistical analyses were performed in R (version 3.2.2, R Core Team, 2015). Five tests were performed, one each for the response variables pH, %OM, %C, %N, and the C/N ratio. Normality of these variables was determined via the inspection of histograms and the Shapiro-Wilks Normality test. The pH and C/N ratio data were Ln-transformed, whereas %OM, %C, and %N were square-root transformed to approximate normality prior to analyses and a Gaussian error distribution of the response variables was assumed. Using generalized linear mixed models (GLMM), each response variable was tested against (i) vegetation type (a factor with three levels; Lawn, Deciduous, Evergreen), (ii) park age (a factor with three levels; Young, Intermediate, Old) and (iii) sample depth (a factor with three levels; 0–10 cm, 11–20 cm, 21–50 cm), and their two-way and three-way interactions. We included park, nested within the city as a random term in the models. Model selection was performed by removing the three-way interaction first (if none of the p-values were < 0.1). Subsequently, two-way interactions were removed depending on significance (same as above). These models did not include the control forests in the city of Lahti.
To test for differences in these five variables among the natural forests and parks, we performed GLMMs including data from only the natural forests and old parks in Lahti with lawn samples excluded. The models included vegetation type (a factor with four levels; natural evergreen forest, park evergreen trees, natural deciduous forest, park deciduous trees) and sample depth (a factor with two levels; 0–10 cm, 11–20 cm), and their interaction. The interaction term was removed if not significant (if no p-values were < 0.1). All variables except pH were Ln-transformed to approximate normality.
Results
Soil pH as Affected by Vegetation and Park Age
Vegetation and park age clearly influenced soil pH (Figure 1, Table 1). Soils under evergreen trees had a lower pH than soils under lawn and deciduous trees, whereas soil pH did not differ between the latter two. Soils in young parks were less acidic compared to intermediate and old parks, which did not differ. Sampling depth had no direct effect on soil pH. Yet, a significant interaction between depth and vegetation revealed that pH in the uppermost soil layer of lawns was lower than in deeper layers, but this pattern was reversed under deciduous and evergreen trees.
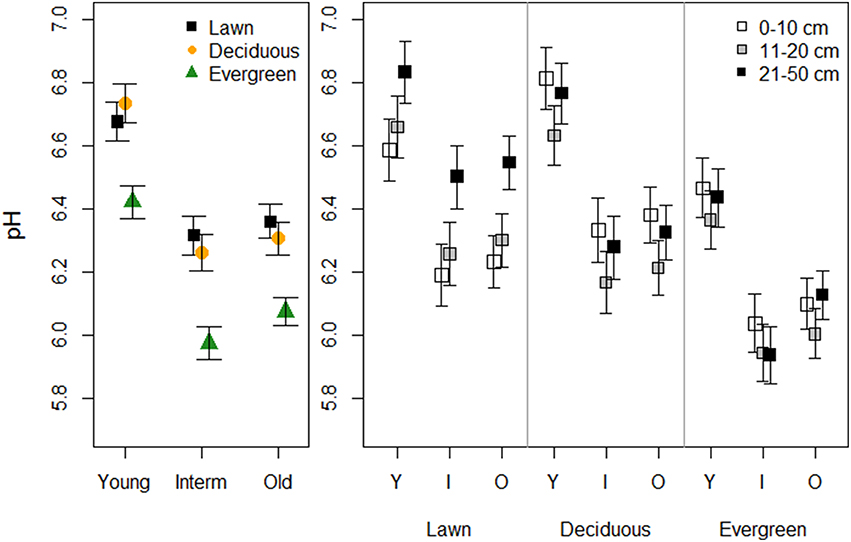
Figure 1. The effects of plant functional groups and park age on soil pH. Predicted means ±SE values are presented. The panel on the left presents data with the three depth intervals pooled, while in the right panel data are shown for each depth interval separately.
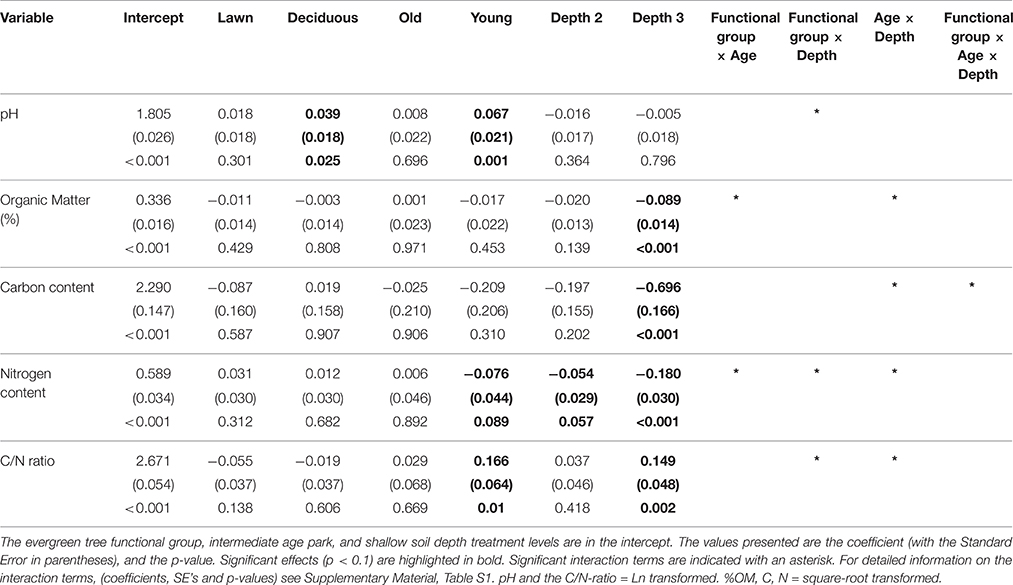
Table 1. GLMM results, testing the effects of plant functional groups (evergreen trees, deciduous trees, lawns), park age (young, intermediate, old), soil depth (0–10, 11–20, and 21–50 cm) and their two-way and three-way interactions on five variables (pH, % organic matter, Carbon content, Nitrogen content, and the C/N ratio).
Comparisons of natural forest and old park soils in Lahti indicated that pH under evergreen (mean 5.11 ± 0.15 SE) and deciduous (5.36 ± 0.13) trees in natural forests was lower than under evergreen (6.29 ± 0.14) and deciduous (6.19 ± 0.13) trees in the old parks (Table 2). Soils were less acidic in the deeper soil layer (5.89 ± 0.15) than in the top layer (5.62 ± 0.15).
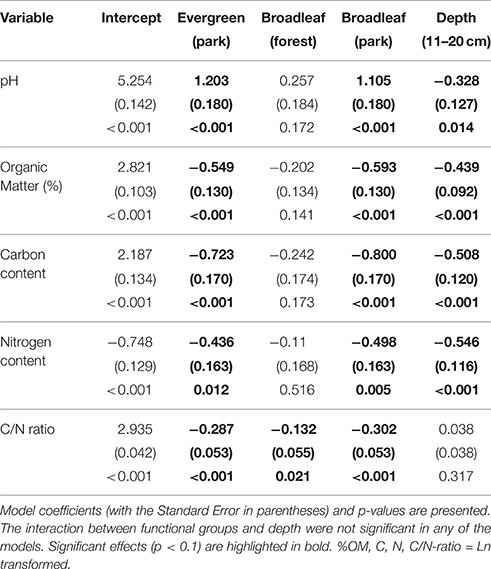
Table 2. Linear model results, testing the effects of plant functional groups in natural forests and parks (a factor with four levels; natural evergreen forests, evergreen trees in old parks, natural deciduous forests, deciduous trees in old parks), sample depth (0–10 cm, 11-20 cm), and their interaction.
Soil Organic Matter Content
Soils under evergreen trees had higher %OM than those under deciduous trees and lawns. Young parks had lower %OM than the older parks (Figure 2, Table 1). Additionally, %OM was more similar between lawns and deciduous trees in the young and intermediate parks than in the old parks (i.e., a significant plant functional group × park age interaction). Deeper soils had consistently lower %OM than shallower soils, with greater differences in young and intermediate parks than in old parks.
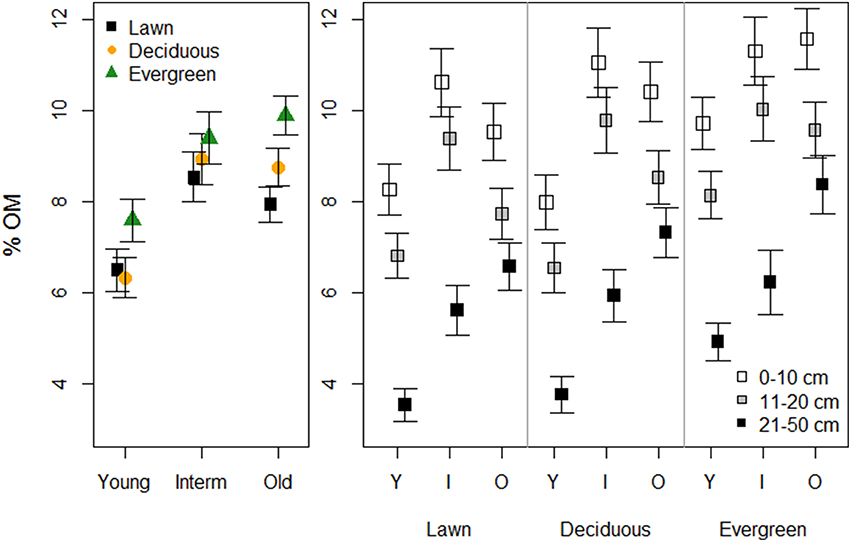
Figure 2. The effects of plant functional groups and park age on soil organic matter concentrations. Predicted means ±SE values are presented. The panel on the left presents data with the three depth intervals pooled, while in the right panel data are shown for each depth interval separately.
In the forest sites, soil organic matter concentrations under evergreen (mean 14.83 ± 2.01 SE) and deciduous (12.03 ± 1.41) trees were higher than those under evergreen (8.22 ± 0.82) and deciduous (7.73 ± 0.63) trees in the old parks (Table 2). Trees did not influence %OM in either parks or forest. Organic matter concentrations were lower in the deeper soil layer (11–20 cm) than in the top layer (0–10 cm) [7.96 ± 0.59 vs. 12.90 ± 1.16].
Soil C and N Concentrations
Soil tot-C and tot-N followed similar patterns in terms of vegetation, park age and soil depth (Figure 3, Table 1). Lawn soils had the lowest and evergreen soils the highest C and N concentrations at each park age category. Young parks had lower soil tot-C and tot-N concentrations than intermediate and old parks. Additionally, differences in tot-C and tot-N between vegetation types in young parks were negligible compared to intermediate, and particularly, old parks. Soil tot-C and tot-N declined with soil depth under each plant functional group independent of park age. However, the magnitude of that decline depended on park age.
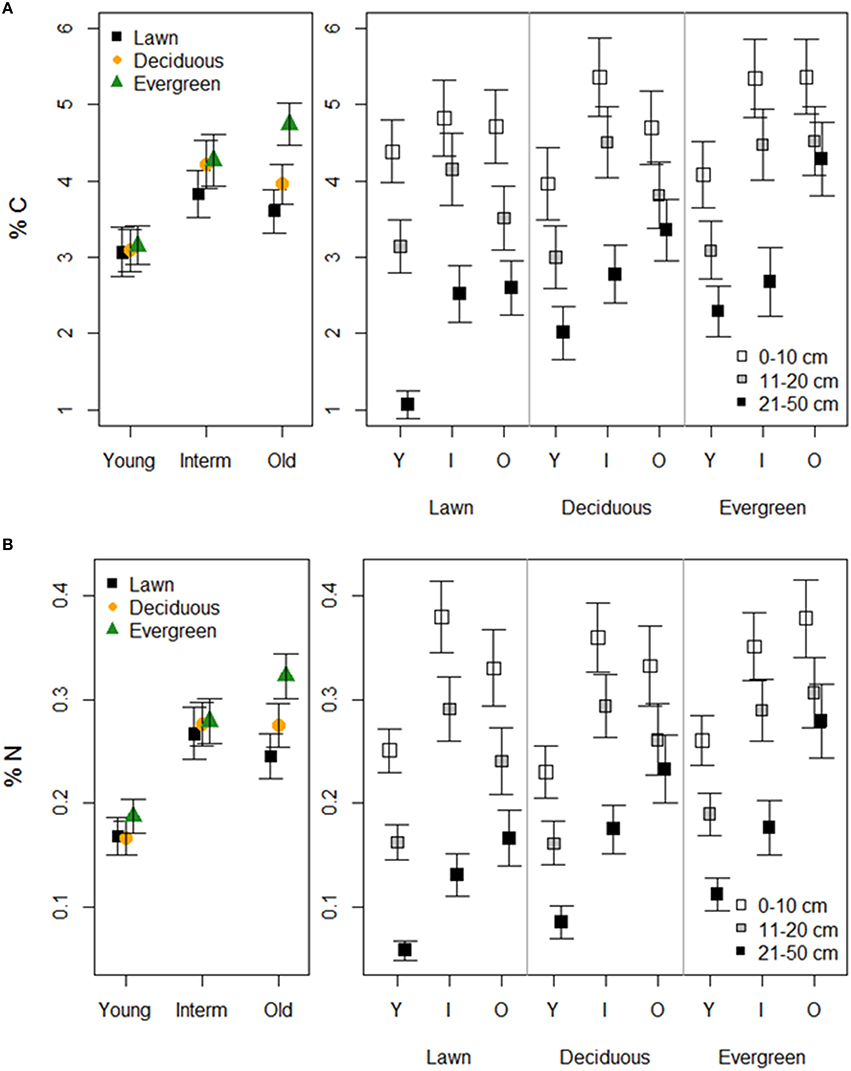
Figure 3. The effects of plant functional groups and park age on soil tot-carbon (A) and tot-nitrogen (B) concentrations. Predicted means ±SE values are presented. Panels on the left present data with the three depth intervals pooled, while panels on the right show results for each depth interval separately.
In the forest sites, soil tot-C was higher under evergreen (mean 8.03 ± 1.54 SE) and deciduous (5.90 ± 0.64) trees than under evergreen (3.75 ± 0.51) and deciduous (3.25 ± 0.30) trees in the old parks (Table 2). The respective values for tot-N were 0.40 ± 0.06 and 0.36 ± 0.05 for evergreen and deciduous trees in the forests, and 0.26 ± 0.04 and 0.23 ± 0.02 for evergreen and deciduous trees in the old parks in Lahti. Tree type had little effect on soil tot-C and tot-N in either of the treed habitats. Both tot-C and tot-N decreased from the surface soil to the layer beneath (tot-C: 0–10 cm = 6.48 ± 0.82, 11–20 cm = 3.65 ± 0.34; tot-N: 0–10 cm = 0.39 ± 0.03, 11–20 cm = 0.22 ± 0.02).
Soil C/N Ratio
Vegetation did not affect the soil C/N ratio in any of the park age classes. However, the C/N ratio was higher in young parks than in the intermediate and old parks (Figure 4, Table 1). The soil C/N ratio increased with soil depth independent of park age, yet younger parks had greater differences between depths than the older parks.
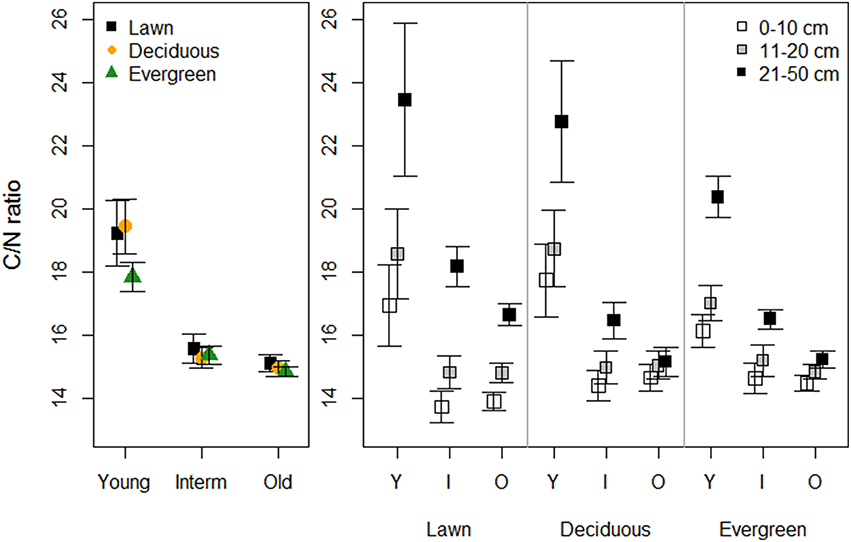
Figure 4. The effects of plant functional groups and park age on the C/N ratio. Predicted means ±SE values are presented. The panel on the left presents data with the three depth intervals pooled, while in the right panel data are shown for each depth interval separately.
The soil C/N ratio under evergreen (mean 19.31 ± 0.99 SE) and deciduous (16.94 ± 0.90) trees in the forest sites was higher than under evergreen (14.47 ± 0.47) and deciduous (14.22 ± 0.34) trees in the old parks (Table 2). Tree type had no influence on the soil C/N ratio in the old parks, whereas in the forest sites, C/N was higher under evergreen (Norway spruce) than deciduous (linden) trees. The C/N ratio did not vary with soil depth (0–10 cm: 15.99 ± 0.75; 11–20 cm: 16.29 ± 0.59).
Discussion
We tested for the first time the hypotheses that (i) eco-physiologically distinct plants (sensu Grime, 1974, 1998) modify the biogeochemistry of urban soils differently (see Wardle, 2002; Bardgett and Wardle, 2010), and that (ii) plant effects diverge among urban parks of different ages. Based on a rigorous and well-replicated experiment, our results indicate that plant functional types (i.e., evergreen and deciduous trees and lawn) differed in their effects on various soil properties, and that these effects were, in general, similar yet magnified as parks aged. More specifically, the most distinct differences in soil properties occurred between evergreen trees and lawns (turf-grass), with intermediate effects of deciduous trees. These results support our first hypothesis and agree with earlier findings on the general importance of plant functional groups in modifying the soil biogeochemistry (Wardle, 2002; Ponge, 2003; Cornwell et al., 2008; Holtkamp et al., 2008; Bardgett and Wardle, 2010). Our second hypothesis, predicting that park age affects the biochemistry of the soil, was also supported. In particular, young park soils contained lower concentrations of OM, tot-C, and tot-N. Our results are also in agreement with previous findings that the accumulation of C in park soils diminishes with soil depth (Golubiewski, 2006; Raciti et al., 2011; Edmondson et al., 2014a,b; Bae and Ryu, 2015; Livesley et al., 2016). However, as the stratification of these soil variables did not relate to park age, our third hypothesis is not supported. Similarly, our results do not corroborate predictions of prevailing urban ecological theory that processes in urban green spaces are strongly controlled by socio-ecological actions (Grimm et al., 2000; Pickett et al., 2001; Alberti, 2008), and as such should differ from those of more natural systems.
For simplicity, we address explanatory variables (plant functional types, park age, and soil depth) separately below, but acknowledge that they are interrelated and the effect of one factor often depends on the magnitude of another (see the significant interactions in Table 1, visualized in Figures 1–4). Finally, we explore the potential of plant types in urban green spaces of different ages in providing ecosystem services under northern European conditions.
General Effects of Plant Functional Groups on Urban Park Soils
Like in most ecosystems, C and N also accumulate in urban soils (Pouyat et al., 2006, 2010) and the accumulation rate reflects the vegetation and its management in the greenspace (see Raciti et al., 2012; Edmondson et al., 2014a,b; Livesley et al., 2016). However, the extent to which plant functional groups can modify urban soils and their C and N stocks is far less known. A few studies conducted in severely disturbed land fill soils (Vauramo and Setälä, 2010), domestic gardens (Edmondson et al., 2014a) and urban public parks (Edmondson et al., 2014b) support our findings that vegetation modifies urban soil properties through, e.g., differences in soil C accumulation in the rhizosphere.
It is difficult to conclude whether the vegetation effects are a result of differences in plant litter, growth strategies, or both. Our results suggest that the type and amount of aboveground litter entering the soils can control the accumulation of OM, SOC, and soil organic nitrogen (SON) in urban soils. For example, the lower soil pH under evergreen trees than under lawns may have retarded the decomposition of both root and needle litter (Ponge, 2003; Rasse et al., 2005), thus boosting OM accumulation in evergreen soils. Further, recurring litter removal can also reduce soil OM accumulation (Kaye et al., 2008). Raking removes deciduous tree leaves more efficiently than evergreen needles that are shed throughout the year (Ukonmaanaho et al., 2008), leaving greater quantities of needle litter behind, perhaps exaggerating the differences in OM accumulation. The fact that soil OM, tot-C, and tot-N concentrations in the old parks (litter removed) were about half of those in the native, unmanaged forests further implies the importance of aboveground litter in the buildup of OM. However, Edmondson et al. (2014b) reported that SOC concentrations in mixed woodland parks with no litter removal did not differ from those in managed parks with litter removal. Given the limited literature on the effects of vegetation on urban soils, and the fact that the few existing studies have been conducted in urban green spaces at different latitudes, plant community compositions, and management intensities, general conclusions on the effects of management and plant functional types are unattainable. More empirical research on the effects of park management (e.g., litter removal) is needed to evaluate the effects of litter on belowground biochemical and food web dynamics in urban systems.
Despite the considerable variation in urban greenspace types and their vegetation (Beer, 2010; Cilliers and Siebert, 2011), a common attribute of urban lands is their high potential for soil C and N accumulation, often greater than adjacent native ecosystems (reviewed in Pouyat et al., 2006). Considering vegetation cover (an estimate of the overall vegetation rather than plant functional groups), urban green spaces with woody vegetation likely possess higher soil C stocks than lawns. For example, Bae and Ryu (2015) observed that mixed forests had the highest SOC stocks, followed by evergreen and broadleaf forests, and lawn in an urban park in Seoul, Republic of Korea. Similarly, in a study of 13 urban green spaces (golf courses) in Melbourne, Australia, Livesley et al. (2016) reported that tree-canopy areas had higher concentrations of C and N than nearby grassy areas. These studies suggest that woody plants can enhance C sequestration in urban soils. This supports our findings that soils associated with trees, evergreens in particular, retained higher soil OM, tot-C, and tot-N concentrations than lawns.
The mechanism by which woody vegetation boosts C accumulation in soils is poorly known. Berthrong et al. (2009) suggested that urban woody vegetation increases the input of organic matter with a high C/N ratio and, since litter with high C/N decomposes more slowly (Stevenson, 1994), the soils will accumulate C stocks over time. Although woody vegetation, compared to lawn, may increase the C/N ratio in urban greenspace soils (Livesley et al., 2016), other urban studies have found no evidence of such an effect (Golubiewski, 2006; Raciti et al., 2011; Edmondson et al., 2014b). In our study, the soil C/N ratio was also unresponsive to vegetation, as indicated by the surprisingly similar soil tot-C and tot-N concentrations relative to vegetation type and soil depth (Figure 3). This suggests that, in relative terms, the inputs (accumulation) and outputs (loss) of the two elements (C and N) were similar in the urban parks studied, which, in turn, indicates that C and N fluxes are tightly coupled and biologically controlled in urban parks. This is somewhat unexpected given the substantial role of anthropogenic factors including the reactive N deposition (MacDonald et al., 2002; Groffman and Pouyat, 2009), the urban heat island effect (Arnfield, 2003) and other physical disturbances (Setälä et al., 2014) that drive soil processes and soil formation in urban habitats (Pouyat et al., 2010).
The relative proportion of C and N in the soil can be used as an indicator of soil quality, describing the relative proportion of nitrogen that is either mineralized by soil microbes or immobilized into the microbial biomass (Berthrong et al., 2009). The equal C/N ratio across all studied park soils implies that the availability of N, i.e., soil fertility (Brady and Weil, 2008), is similar beneath the three vegetation types in our study (per age class), despite the 30% higher N concentration under the evergreens than under lawns. It is noteworthy that our parks were neither fertilized nor managed differently during at least the last decades, indicating that soil fertility, judged by C/N ratio, is only minimally controlled by plant types. This is supported by various urban studies under different climates and vegetation types (Kaye et al., 2005; Golubiewski, 2006; Raciti et al., 2011; Dungait et al., 2012). The higher soil C/N ratio under evergreen trees in the natural forest (19.31) compared to that in the old parks (14.47), i.e., the higher availability of N for plant uptake in the old parks, further suggests the effects of park management (e.g., mowing and litter removal) on C and N dynamics. Indeed, litter removal has been suggested to export only little N but substantial amounts of C from urban green spaces (Kaye et al., 2008). Whether the removal of deciduous litter is the main factor causing differences in soil properties between evergreen and deciduous trees in our study is open to debate and merits further research. Our findings strongly suggest that vegetation affects physico-chemical properties of urban soils—a hypothesis that, thus far, has only been tested in natural and semi-natural systems (see Wardle et al., 2004; Bardgett and Wardle, 2010).
The Effects of Park Age
Park age in general had a decisive influence on all the measured soil variables: young parks differed from intermediate and old parks whereas the latter two shared similar soil characteristics. However, as park aged, the effects of vegetation types on soil characteristics were magnified. We hypothesized that the ability of plants to modify soils is park age dependent, i.e., controlled by the time the plants and soils have interacted in the rhizosphere (see De Deyn et al., 2008). Our results support this hypothesis. Interestingly, unlike the other soil parameters, evergreen trees acidified the soil quickly, which was already visible in young parks. This is surprising, given the short time (~10 years) that evergreen trees had grown in the young parks. The polyphenol-rich litter of evergreen trees (Ponge, 2003; Rasse et al., 2005), in concert with their efficient cation exchange capacity (Helmisaari and Mälkönen, 1989), may explain the low soil pH and the high OM and C concentrations in evergreen soils. However, irrespective of vegetation, soil pH declined clearly in the young and intermediate parks, after which the acidification process leveled out.
Besides soil pH, other soil properties also seemed to stabilize after early park establishment: none of the measured soil variables (OM, tot-C, tot-N, the C/N ratio) differed between intermediate and old parks. This corroborates previous findings suggesting that 50 years is long enough for soil stabilization in urban lawns (Raciti et al., 2011) and that the soil C concentrations tend to increase during the first decades after park construction (Golubiewski, 2006; Raciti et al., 2011; Livesley et al., 2016). However, in contrast with Berthrong et al. (2009) and Livesley et al. (2016), who reported an increase in soil C/N with time in urban green spaces, we showed an initial decline in soil C/N after which it stabilized at the intermediate age of parks. The lower C/N ratio is due to the increased proportion of N relative to C, i.e., the accumulation of N and/or a loss, in relative terms, of C in the soil. Because the pattern was the same at each park age, we conclude that the initial decline in the C/N ratio is not related to vegetation itself, but rather to other factors that control C and N inputs and outputs in northern latitudes. In urban milieus, such factor may include N deposition and/or differences in C-rich litter removal.
The higher soil pH and lower OM, tot-C, and tot-N concentrations in young parks compared to the older ones are not only attributable to vegetation effects but may also be influenced by the quality of the top soil used during park construction. Based on expert opinion (Pekka Engblom, Helsinki City Public Works Department, and Markku Saari, Technical and Environmental Services of the city of Lahti), however, common park construction practices, including excavation and topsoil addition, differed little between the young and intermediate parks, suggesting that vegetation drives soil processes in the parks. No information is available on common practices in park construction >100 years ago, but based on the nearly identical soil properties and similar vertical stratification (see below) between intermediate and old parks, it is unlikely that the protocols differed drastically. Furthermore, it is possible that accumulation of heavy metals, known to affect soil processes and plant growth (Selonen and Setälä, 2015), differed between parks of different ages. The soils in young parks may have accumulated less heavy metals compared to the older parks, simply because of the much shorter time the latter parks have been exposed to, e.g., traffic-derived metal deposition. Whether deposition of heavy metals was large enough to influence soil processes in the soil rhizosphere of the small to medium-sized cities of Helsinki and Lahti requires further studies. Furthermore, we cannot rule out the possibility that the lower %OM and %C in the soils of young parks was due to higher temperatures and thus enhanced decomposition rates in these parks. To conclude, our results are consistent with other studies showing that concentrations of C, N, and OM increase with park age, especially during the first decades after park establishment. Although, the soil physico-chemical parameters under lawns and deciduous trees seemingly stabilized by the intermediate age, these parameters still change, albeit slowly, with time in the evergreen rhizosphere. This further emphasizes the importance of specific plant-soil interactions in modifying soils in anthropogenically-disturbed urban milieus.
Vegetation Effects on the Vertical Stratification of Soil Parameters
We hypothesized that soils in young parks are more stratified than soils in intermediate and especially the oldest parks. We predicted that fewer roots deeper in the soil in young parks would explain this variation since the mean residence time of root-derived C is twice as long as shoot-derived C (Rasse et al., 2005). Further, since root turnover is one of the most important mechanisms by which organic matter enters the soil, it can determine the distribution of C in the soil profile (Jobbagy and Jackson, 2000). Similarly to previous studies (Golubiewski, 2006; Raciti et al., 2011; Edmondson et al., 2012, 2014b; Livesley et al., 2016) we showed that soil variables, except soil pH, were sensitive to soil depth, and OM, tot-C and tot-N decreased and the soil C/N ratio increased with soil depth. However, this pattern was rather consistent across the targeted vegetation types and park ages, suggesting that plant roots have a negligible effect on these soil variables. This is unexpected given the clear root stratification detected in other urban studies (Wu, 1985; Bae and Ryu, 2015). We did not quantify plant root distribution. However, based on our cursory visual inspection during sample preparation and other ongoing research effort in the same locations, tree roots were always present throughout the soil layers in the intermediate and old parks, but absent deeper than 20 cm in the young parks at the edge of the canopy projection where our sampling took place. Thus, factors other than tree root distribution likely drive OM, C and N accumulation in the soil profile in urban parks in northern climates. For example, earthworms—capable of mixing soils (Edwards and Bohlen, 1996)—were frequently observed (H. Setälä, personal observations) in all parks and may have unstratified the initially strongly layered young park soils.
However, in the forests where soil pH was ca. 1 pH unit lower than in the old parks, soil pH declined with soil depth underneath both evergreen and deciduous trees. The lower pH in the forests and its stratification are likely results of podsolization, typical to boreal forest soil (Mikola, 1985; Ponge, 2003) and the scarcity of large-sized earthworms in these soils (H. Setälä, personal observations). This also suggests that urban park soils have abundant earthworm populations regardless of age and vegetation and that they do not develop podzols typical to the boreal biome. Furthermore, as shown in several studies, urban soils typically have high pH (e.g., Craul and Klein, 1980; Jim, 1993; Hagan et al., 2012; Kuoppamäki et al., 2014), resulting from materials used in urban infrastructure that are high in calcium, such as concrete (Pouyat et al., 2007b). It is possible that substantial Ca inputs result in oversaturation of Ca and other cations in urban soils, which—in turn—inhibit clear soil stratification indicative of boreal forests.
Vegetation and Ecosystem Services
The ability of soils, including urban soils, to sequester carbon and nitrogen is among the most important soil ecosystem services (see reviews by Pataki et al., 2006; Barrios, 2007; Churkina et al., 2010; Pouyat et al., 2010; Wall et al., 2015). Based on elemental concentrations, we showed—to our knowledge for the first time—that urban park soils in the northern climatic zone are rich in C and N and, importantly, that plant functional groups can control their concentrations for decades and even centuries. To evaluate the potential of these soils to store C on an area basis (e.g., kg C m−2), soil density (bulk density) must be known. We did not determine bulk density because our main objective was to evaluate plant effects at a local, rhizosphere scale rather than to extrapolate the plant effects on C and N stocks at a larger scale, e.g., that of a park. However, using bulk densities typical to similar soils (Golubiewski, 2006; Raciti et al., 2011; Edmondson et al., 2014b; Bae and Ryu, 2015; Livesley et al., 2016), we estimated the potential of our different vegetation types to sequester C and N at a larger scale. We averaged the literature-derived soil bulk density data for each soil layer separately and used 1.22, 1.45, and 1.53 for 0–10, 11–20, and 21–50 cm soil depths, respectively. The depth intervals in these publications were not always equal to ours; we used those most similar to our intervals. Based on these estimates, old parks without trees (i.e., lawn) store, on average, 22.02 kg C m−2 (0–50 cm depth), whereas old parks with deciduous trees store 23.40 kg C m−2 and with evergreen trees as much as 35.50 kg C m−2. The potential of young parks without trees to store C is much lower, 14.90 kg m−2, whereas these parks with deciduous trees and evergreens can store 21.40 and 18.90 kg of C m−2, respectively.
Our soil C density estimates are high and likely attributable to the northern location. The SOC sequestered by urban greenspace soils at lower latitudes range between 2 and 18 kg m−2 (Golubiewski, 2006; Pouyat et al., 2006, 2009; Edmondson et al., 2014b; Livesley et al., 2016). Given the large variation in sampling depths among these studies, comparing our results to others is not straightforward. However, it seems that both carbon concentrations and densities (Golubiewski, 2006; Raciti et al., 2008; Lorenz and Lal, 2009; Livesley et al., 2016) are higher in our park soils than in those reported for greenspace soils in milder climates. This is not surprising given the strong influence of temperature on decomposition (Swift et al., 1979) and the fact that evergreen trees—that have received little attention in previous urban greenspace studies—produce poorly decomposable litter. Using bulk densities we adopted from the literature for the high fertility native forest soils, we estimate that the amount of C stored in the upper 0–20 cm soil is 17.9 and 18.0 kg m−2 for the native forests and old urban parks with evergreens, respectively. This indicates that converting native mixed forests to urban parks does not necessarily lead to noticeable changes in C sequestration in northern climates, at least when the parks are planted with trees.
Urban parks also differed in their soil N stocks. Soils beneath evergreen trees in old parks stored on average 2.29 kg tot-N m−2 whereas those under lawn stored 1.53 kg m−2. Stocks of soil tot-N in young parks were 1.8 and 0.81 kg m−2 under evergreen trees and lawns, respectively. Similarly to tot-C, evergreen trees were associated with the highest and lawns with the lowest stocks of soil tot-N. In old parks the amount of tot-N stored under deciduous trees was 78% of that under evergreen trees, whereas in young parks the two tree types stored an equal amount of N. Knowledge about soil N stocks in urban greenspace soils is scanty (Lorenz and Lal, 2009). Of the few studies available, N stock of over 0.50 kg m−2 (0–100 cm depth) has been reported in urban residential green spaces in Baltimore, USA (Raciti et al., 2011). N density declined slightly with depth—from ca. 0.17 kg m−2 at the surface soil (0–10 cm depth; %N = 0.20) to 0.16 kg m−2 (%N = 0.03) deeper (30–70 cm) in the soil profile (Raciti et al., 2011). These values are considerably lower than ours, suggesting that urban parks, especially old ones with evergreen trees, under northern climates have a particularly high capacity to retain soil N. However, whether soils underneath the divergent plant types differ in terms of preventing NO3 leaching or N2O emissions—processes considered crucial ecosystem services—is unknown. As for soil types, sandy loam, and loamy sand were the dominant soil types in each park of the study, with approximately 75% of the soils falling into these two categories (unpublished data). Judged by visual observation only few sites showed signs of disturbance at depths more than 20 cm; in 5 parks in Helsinki there was evidence of fill material derived from landscaping activities.
Conclusions
We demonstrated that plant functional groups typical in urban parks in northern latitudes differ in their ability to modify soil physico-chemical properties, and that the magnitude of vegetation effects often increased with park age. This is surprising given that the soil, independent of vegetation and park age, was topped by well-growing grass cover and not, except for the lawn, by understory vegetation typical to these trees under natural conditions. We also show that the soil in urban green spaces possess a substantial capacity to store C and N even without irrigation and frequent fertilization, treatments that are suggested to be responsible for the high C sequestration in urban green spaces (see Pouyat et al., 2006, 2010). Evergreen trees were particularly influential for soil characteristics, possibly because of their recalcitrant litter and root exudates that lower pH and increase OM content compared to lawns. This suggests that old parks with evergreen trees are particularly effective in modifying park soils. However, one needs to bear in mind that urban green spaces provide a multitude of services, including esthetic values, and recreational services for park visitors.
Besides plants, diverse microbial communities perform many transformations that control soil properties and provide diverse ecosystem services (Harris and Birch, 1989; Bell et al., 2005; Green and Bohannan, 2006), While these microbial communities govern soil biogeochemical cycles, the structure, activity and diversity of the soil biota are sensitive to a range of biotic and environmental factors (Colloff et al., 2008; Macdonald et al., 2009; Jumpponen et al., 2010). Soil microbes may directly respond to changes in soil geochemistry (Fierer and Jackson, 2006; Rousk et al., 2010), but may also differ among plant species, particularly in the rhizosphere (Grayston et al., 1998; Lothamer et al., 2014). As a result, dissecting the linkages between management decisions that lead to urban greenspace plant species selection, their effects on the soil and rhizosphere microbiomes, and the resultant ecosystem services would be critical next steps to gain deeper appreciation for the interconnectedness between urban land use, soil biogeochemistry, and soil food webs. However, our understanding of the complex interactions between the urban soil biota and environment factors in urban areas remains superficial at best.
Author Contributions
HS, the corresponding author, conceived, designed, and wrote the manuscript and contributed to performing the experiment. GF was responsible for locating and identifying the study sites in the two cities, communicating with city officials and organizing the field work. She also participated in the writing process. JA participated in fieldwork and conducted the soil physical-chemical analyses. He also participated in the writing process. NH participated in fieldwork and in the writing process. AJ participated in planning and writing of the work. DK performed all the statistical analyses, participated in fieldwork and in writing the manuscript.
Conflict of Interest Statement
The authors declare that the research was conducted in the absence of any commercial or financial relationships that could be construed as a potential conflict of interest.
Acknowledgments
We are grateful to Tuukka Ryynänen, Rauni Strömmer, and Martin Romantschuck for help with field sampling. Satu Tegel, Tuuli Ylikotila and Pekka Engblom provided data for the parks in Helsinki, and Markku Saari provided information about parks in Lahti. Three reviewers greatly improved the quality of this paper. The research was funded by an Academy of Finland grant (Grant number 268548).
Supplementary Material
The Supplementary Material for this article can be found online at: http://journal.frontiersin.org/article/10.3389/fevo.2016.00093
References
Aerts, R., and Chapin, F. S. III. (2000). The mineral nutrition of wild plants revisited: a re-evaluation of processes and patterns. Adv. Ecol. Res. 30, 1–67. doi: 10.1016/S0065-2504(08)60016-1
Alberti, M. (2008). Advances in Urban Ecology: Integrating Humans and Ecological Processes in Urban Ecosystems. Seattle, WA: Springer Science+Business Media, LLC.
Allison, S. D. (2006). Brown ground: a soil carbon analogue for the green world hypothesis? Am. Nat. 167, 619–627. doi: 10.1086/503443
Arnfield, A. J. (2003). Two decades of urban climate research: a reviewe of turbulence, exchanges of energy and water, and the urban heat island. Int. J. Climatol. 23, 1–26. doi: 10.1002/joc.859
Austin, A. T., and Zanne, A. E. (2015). Whether in life or in death: fresh perspectives on how plants affect biochemical cycling. J. Ecol. 103, 1367–1371. doi: 10.1111/1365-2745.12486
Bae, J., and Ryu, Y. (2015). Land use and land cover changes explain spatial and temporal variations of the soil organic carbon stocks in a constructed urban park. Land. Urban Plan. 136, 57–67. doi: 10.1016/j.landurbplan.2014.11.015
Bardgett, R. D., and Wardle, D. A. (2010). Above-Ground-Belowground Linkages. Biotic Interactions, Ecosystem Processes and Global Change. New York, NY: Oxford University Press.
Barrios, E. (2007). Soil biota, ecosystem services and land productivity. Ecol. Econ. 64, 269–285. doi: 10.1016/j.ecolecon.2007.03.004
Beer, A. (2010). “Greenspaces, green structure, and green infrastructure planning,” in Urban Ecosystem Ecology, Vol. 55, eds J. Aitkenhead-Peterson and A. Volder (Madison, WI: Agronomy Monograph), 431–448. doi: 10.2134/agronmonogr55.c21
Bell, T., Newman, J. A., Silverman, B. W., Turner, S. L., and Lilley, A. K. (2005). The contribution of species richness and composition to bacterial services. Nature 436, 1157–1160. doi: 10.1038/nature03891
Berthrong, S. T., Jobbagy, E. G., and Jackson, R. B. (2009). A global meta-analysis of soil exchangeable cations, pH, carbon, and nitrogen with afforestation. Ecol. Appl. 19, 2228–2241. doi: 10.1890/08-1730.1
Brady, N. C., and Weil, R. R. (2008). The Nature and Properties of Soils. Upper Saddle River, NJ: Pearson Prentice Hall.
Byrne, L. (2007). Habitat structure: a fundamental concept and framework for urban soil ecology. Urban Ecosyst. 10, 255e274. doi: 10.1007/s11252-007-0027-6
Carreiro, M., Pouyat, R. V., Tripler, C. E., and Zhu, W.-X. (2009). “Carbon and nitrogen cycling in soils of remnant forests along urban-rural gradients: case studies in the New-York metropolitan area and Louisville, Kentucky,” in Ecology of Cities and Towns: A Comparative Approach, eds M. J. McDonnell, A. K. Hahs, and J. H. Breuste (Cambridge: Cambridge University Press), 308–328.
Churkina, G., Brown, D. G., and Keoleian, G. (2010). Carbon stored in human settlements: the conterminous United States. Global Change Biol. 16, 135–143. doi: 10.1111/j.1365-2486.2009.02002.x
Cilliers, S. S., and Siebert, S. J. (2011). “Urban flora and vegetation: patterns and processes” in Urban Ecology, Patterns, Processes, and Applications, ed J. Niemelä (Oxford: Oxford University Press), 148–158.
Coleman, D. C., Reid, C. P. P., and Cole, C. V. (1983). “Biological strategies of nutrient cycling in soil systems,” in Advances in Ecological Research, Vol. 13. eds A. MacFadyen and E. D. Ford, (London: Academic Press), 1–55.
Colloff, M. J., Wakelin, S. A., Gomez, D., and Rogers, S. L. (2008). Detection of nitrogen cycle genes in soils for measuring the effects of changes in land use and management. Soil Biol. Biochem. 40, 1637–1645. doi: 10.1016/j.soilbio.2008.01.019
Cornwell, W. K., Cornelissen, J. H. C., Amatangelo, K., Dorrepaal, E., Eviner, V. T., Godoy, O., et al. (2008). Plant species traits are the predominant control on litter decomposition rates within biomes worldwide. Ecol. Lett. 11, 1065–1071. doi: 10.1111/j.1461-0248.2008.01219.x
Craul, P. J., and Klein, C. J. (1980). Characterization of Street Side soils of Syracuse, Vol. 3. New York, NY: METRIA.
De Deyn, G. B., Cornelissen, J. H. C., and Bardgett, R. D. (2008). Plant functional traits and soil carbon sequestration in contrasting biomes. Ecol. Lett. 11, 516–531. doi: 10.1111/j.1461-0248.2008.01164.x
Dungait, J. A. J., Cardenas, L. M., Blackwell, M. S. A., Wu, L., Withers, P. J. A., Chadwick, D. R., et al. (2012). Advances in the understanding of nutrient dynamics and management in UK agriculture. Sci. Total Environ. 434, 39–50. doi: 10.1016/j.scitotenv.2012.04.029
Easton, Z. M., and Petrovic, A. M. (2008). “Determining nitrogen loading rates based on land use in an urban watershed,” in The Fate of Nutrients and Pesticides in the Urban Environment, Vol. 997, ACS Symposium Series, American Chemical Society, eds M. T. Nett, M. J. Carroll, B. P. Horgan, and A. M. Petrovic (Washington, DC), 19–42.
Edmondson, J. L., Davies, Z. G., McCormack, S. A., Gaston, K. J., and Leake, J. R. (2014a). Land-cover effects on soil organic carbon stocks in a European city. Sci. Total Environ. 472, 444–453. doi: 10.1016/j.scitotenv.2013.11.025
Edmondson, J. L., Davies, Z. G., McHugh, N., Gaston, K. J., and Leake, J. R. (2012). Organic carbon hidden in urban ecosystems. Sci. Rep. 2:963. doi: 10.1038/srep00963
Edmondson, J. L., O'Sullivan, O. S., Inger, R., Potter, J., McHugh, N., Gaston, K. J., et al. (2014b). Urban tree effects on soil organic carbon. PLoS ONE 9:e101872. doi: 10.1371/journal.pone.0101872
Edwards, C. A., and Bohlen, P. J. (1996). Biology and Ecology of Earthworms. London, UK: Springer Sciences & Business Media.
Fierer, N., and Jackson, R. B. (2006). The diversity and biogeography of soil bacterial communities. Proc. Natl. Acad. Sci. U.S.A. 103, 626–631. doi: 10.1073/pnas.0507535103
Forsius, M., Anttila, S., Arvola, L., Bergström, I., Hakola, H., Heikkinen, H. I., et al. (2013). Impacts and adaptation options of climate change on ecosystem services in Finland: a model based study. Curr. Opin. Environ. Sustainab. 5, 26–40. doi: 10.1016/j.cosust.2013.01.001
Frank, K. W., O'Reilly, K. M., Crum, J. R., and Calhoun, R. N. (2006). The fate of nitrogen applied to a mature Kentucky bluegrass turf. Crop Sci. 46, 209–2015. doi: 10.2135/cropsci2005.04-0039
Franzluebbers, A. J. (2002). Soil organic matter stratification ratio as an indicator of soil quality. Soil Tillage Res. 66, 95–106. doi: 10.1016/S0167-1987(02)00018-1
Glynn, P. D., and Plummer, N. P. (2005). Geochemistry and the understanding of ground-water systems. Hydrogeol. J. 13, 263–287. doi: 10.1007/s10040-004-0429-y
Golubiewski, N. E. (2006). Urbanization increases grassland carbon pools: effects of landscaping in Colorado's front range. Ecol. Appl. 16, 555–571. doi: 10.1890/1051-0761(2006)016[0555:UIGCPE]2.0.CO;2
Gough, C. M., Vogel, C. S., Schmid, H. P., and Curtis, P. S. (2008). Controls on Annual Forest Carbon Storage: lessons from the past and predictions for the future. Bioscience 58, 609–622. doi: 10.1641/B580708
Grayston, S. J., Wang, S. Q., Campbell, C. D., and Edwards, A. C. (1998). Selective influence of plant species on microbial diversity in the rhizosphere. Soil Biol. Biochem. 30, 369–378. doi: 10.1016/S0038-0717(97)00124-7
Green, J., and Bohannan, B. J. (2006). Spatial scaling of microbial biodiversity. Trends Ecol. Evol. 21, 501–507. doi: 10.1016/j.tree.2006.06.012
Grime, J. P. (1974). Vegetation classification by reference to strategies. Nature 250, 26–31. doi: 10.1038/250026a0
Grime, J. P. (1998). Benefits of plant diversity to ecosystems: immediate, filter and founder effects. J. Ecol. 86, 902–910. doi: 10.1046/j.1365-2745.1998.00306.x
Grimm, N. B., Grove, M. J., Pickett, S. T. A., and Redman, C. L. (2000). Integrated approaches to long-term studies of urban ecological systems. Bioscience 50, 571–584. doi: 10.1641/0006-3568(2000)050[0571:IATLTO]2.0.CO;2
Groffman, P. M., and Pouyat, R. V. (2009). Methane uptake in urban forests and lawns. Environ. Sci. Technol. 43, 5229–5335. doi: 10.1021/es803720h
Groffman, P. M., Williams, C. O., Pouyat, R. V., Band, L. E., and Yesilonis, I. C. (2009). Nitrate leaching and nitrous oxide flux in urban forests and grasslands. J. Environ. Qual. 38, 1846–1860. doi: 10.2134/jeq2008.0521
Hagan, D., Dobbs, C., Timilsina, N., Escobedo, F., Toor, G. S., and Andreu, M. (2012). Anthropogenic effects on the physical and chemical properties of subtropical coastal urban soils. Soil Use Manag. 28, 78–88. doi: 10.1111/j.1475-2743.2011.00379.x
Harris, J. A., and Birch, P. (1989). Soil microbial activity in opencast coal-mine restorations. Soil Use Manag. 5, 155–160. doi: 10.1111/j.1475-2743.1989.tb00777.x
Helmisaari, H.-S., and Mälkönen, E. (1989). Acidity and nutrient content of throughfall and soil leachate in three Pinus sylvestris stands. Scand. J. Forest Res. 4, 13–28. doi: 10.1080/02827588909382542
Holtkamp, R., Kardol, P., van der Val, A., Dekker, S. C., van der Putten, W. H., and de Ruiter, P. C. (2008). Soil food web structure during ecosystem development after land abandonment. Appl. Soil Ecol. 39, 23–34. doi: 10.1016/j.apsoil.2007.11.002
Jim, C. Y. (1993). Soil compaction as a constraint to tree growth in tropical and subtropical urban habitats. Environ. Conserv. 20, 35–49. doi: 10.1017/S0376892900037206
Jobbagy, E. G., and Jackson, R. B. (2000). The vertical distribution of soil organic carbon and its relation to climate and vegetation. Ecol. Appl. 10, 423–436. doi: 10.1890/1051-0761(2000)010[0423:TVDOSO]2.0.CO;2
Jumpponen, A., Jones, K. L., Mattox, J. D., and Yeage, C. (2010). Massively parallel 454-sequencing of fungal communities in Quercus spp. ectomycorrhizas indicates seasonal dynamics in urban and rural sites. Mol. Ecol. 19, 41–53. doi: 10.1111/j.1365-294X.2009.04483.x
Kardol, P., Bezemer, M. T., and van der Putten, W. H. (2006). Temporal variation in plant-soil feedback controls succession. Ecol. Lett. 9, 1080–1088. doi: 10.1111/j.1461-0248.2006.00953.x
Kaye, J. P., Majumdar, A., Gries, C., Buyantuyev, A., Grimm, N. B., Hope, D., et al. (2008). Hierarchical Bayesian scaling of soil properties across urban, agricultural, and desert ecosystems. Ecol. Appl. 18, 132–145. doi: 10.1890/06-1952.1
Kaye, J. P., McCulley, R. L., and Burke, I. C. (2005). Carbon fluxes, nitrogen cycling, and soilmicrobial communities in adjacent urban, native and agricultural ecosystems. Glob. Change Biol. 11, 575–587. doi: 10.1111/j.1365-2486.2005.00921.x
Kuoppamäki, K., Setälä, H., Rantalainen, A. L., and Kotze, D. J. (2014). Urban snow indicates pollution originating from road traffic. Environ. Pollut. 195, 56–63. doi: 10.1016/j.envpol.2014.08.019
Lal, R. (2004). Soil carbon sequestration to mitigate climate change. Geoderma 123, 1–22. doi: 10.1016/j.geoderma.2004.01.032
Lal, R. (2010). Sequestering carbon in soils of agro-ecosystems. Food Policy 36, 33–39. doi: 10.1016/j.foodpol.2010.12.001
Lavelle, P. (1988). Earthworm activities and the soil system. Biol. Fertil. Soils 6, 237–251. doi: 10.1007/BF00260820
Lehmann, A., and Stahr, K. (2007). Nature and significance of anthropogenic urban soil. J. Soils Sediments 7, 247–260. doi: 10.1065/jss2007.06.235
Livesley, S. J., Ossola, A., Threlfall, C. G., Hahs, A. K., and Williams, N. S. G. (2016). Soil carbon and carbon/nitrogen ratio change under tree canopy, tall grass, and turf grass areas of urban green space. J. Environ. Qual. 45, 215–223. doi: 10.2134/jeq2015.03.0121
Lorenz, K., and Lal, R. (2009). Biogeochemical C and N cycles in urban soils. Environ. Int. 35, 1–8. doi: 10.1016/j.envint.2008.05.006
Lothamer, K., Brown, S. P., Mattox, J. D., and Jumpponen, A. (2014). Comparison of root-associated communities of native and non-native ectomycorrhizal hosts in an urban landscape. Mycorrhiza 24, 267–280. doi: 10.1007/s00572-013-0539-2
Macdonald, C. A., Thomas, N., Robinson, L., Tate, K. R., Ross, D. J., Dando, J., et al. (2009). Physiological, biochemical and molecular responses of the soil microbial community after afforestation of pastures with Pinus radiata. Soil Biol. Biochem. 41, 1642–1651. doi: 10.1016/j.soilbio.2009.05.003
MacDonald, J., Dise, N., Matzner, E., Armbruster, M., Gundersen, P., and Forsius, M. (2002). Nitrogen input together with ecosystem nitrogen enrichment predict nitrate leaching from European forests. Global Change Biol. 8, 1028–1033. doi: 10.1046/j.1365-2486.2002.00532.x
Meentemeyer, V. (1978). Macroclimate and lignin control of litter decomposition rates. Ecology 59, 465–472. doi: 10.2307/1936576
Mikola, P. (1985). The Effect of Tree Species on the Biological Properties of Forest Soil. National Swedish Environmental Protection Board, Rapport 3017.
Moore, J. C., and Hunt, H. W. (1988). Resource compartmentalization and the stability of real ecosystems. Nature 333, 261–263. doi: 10.1038/333261a0
Ossola, A., Hahs, A. K., and Livesley, S. J. (2015). Habitat complexity influences fine scale hydrological processes and the incidence of stormwater runoff in managed urban ecosystems. J. Environ. Manage 159, 1–10. doi: 10.1016/j.jenvman.2015.05.002
Pataki, D. E., Alig, R. J., Fung, A. S., Golubiewski, N. E., Kennedy, C., and McPherson, E. G. (2006). Urban ecosystems and the North American carbon cycle. Glob. Change Biol. 12, 2092–2101. doi: 10.1111/j.1365-2486.2006.01242.x
Paterson, E., Osler, G., Dawson, L. A., Gebbing, T., Sim, A., and Ord, B. (2008). Labile and recalcitrant plant fractions are utilized by distinct microbial communities in soil: independent of the presence of roots and mycorrhizal fungi. Soil Biol. Biochem. 40, 1103–1113. doi: 10.1016/j.soilbio.2007.12.003
Pavao-Zuckerman, M. A. (2008). The nature of urban soils and their role in ecological restoration in cities. Restor. Ecol. 16, 642–649. doi: 10.1111/j.1526-100X.2008.00486.x
Pavao-Zuckerman, M. A., and Coleman, D. C. (2007). Urbanization alters the functional composition, but not taxonomic diversity, of the soil nematode community. Appl. Soil. Ecol. 35, 329–339. doi: 10.1016/j.apsoil.2006.07.008
Pickett, S. T. A., Cadenasso, M. L., Grove, J. M., Nilon, C. H., Pouyat, R. V., Zipperer, W. C., et al. (2001). Urban ecological systems: linking terrestrial ecological, physical, and socioeconomic components of metropolitan areas. Annu. Rev. Ecol. Syst. 32, 127–157. doi: 10.1146/annurev.ecolsys.32.081501.114012
Ponge, J.-F. (2003). Humus forms in terrestrial ecosystems: a framework to biodiversity. Soil Biol. Biochem. 35, 935–945. doi: 10.1016/S0038-0717(03)00149-4
Pouyat, R. V., Belt, K., Pataki, D., Groffman, P. M., Hom, J., and Band, L. (2007b). “Urban land-use change effects on biogeochemical cycles” in Terrestrial Ecosystems in a Changing World, eds J. G. Canadell, D. Pataki, and L. F. Pitelka (Berlin; Heidelberg; New York, NY: Global Change, The IGBP Series, Springer), 45–58.
Pouyat, R., Groffman, P., Yesilonis, I., and Hernandez, L. (2002). Soil carbon pools and fluxes in urban ecosystems. Environ. Poll. 116, 107–118. doi: 10.1016/S0269-7491(01)00263-9
Pouyat, R. V., Szlavecz, K., Yesilonis, I. D., Groffman, P. M., and Schwarz, K. (2010). “Chemical, physical, and biological characteristics of urban soils,” in Urban Ecosystem Ecology, Vol. 55., eds J. Aitkenhead-Peterson and A. Volder (Madison, WI: Agronomy Monograph), 119–152.
Pouyat, R. V., Yesilonis, I. D., and Nowak, D. J. (2006). Carbon storage by urban soils in the USA. J. Environ. Qual. 35, 1566–1575. doi: 10.2134/jeq2005.0215
Pouyat, R. V., Yesilonis, I. D., Russell-Anelli, J., and Neerchal, N. K. (2007a). Soil chemical and physical properties that differentiate urban land-use and cover types. Soil Sci. Soc. Am. J. 71:1010–1019. doi: 10.2136/sssaj2006.0164
Pouyat, R. V., Yesilonis, I., and Golubiewski, N. (2009). A comparison of soil organic carbon stocks between residential turf grass and native soil. Urban Ecosyst. 12, 45–62. doi: 10.1007/s11252-008-0059-6
Qian, Y. L., and Follett, R. F. (2002). Assessing soil carbon sequestration in turfgrass systems using long-term soil testing data. Agron. J. 94, 930–935. doi: 10.2134/agronj2002.9300
Raciti, S. M., Groffman, P. M., and Fahey, T. J. (2008). Nitrogen retention in urban lawns and forests. Ecol. Appl. 18, 1615–1626. doi: 10.1890/07-1062.1
Raciti, S. M., Groffman, P. M., Jenkins, J. C., Pouyat, R. V., Fahey, T. J., Pickett, S. T. A., et al. (2011). Accumulation of carbon and nitrogen in residential soils with different land use histories. Ecosystems 14, 287–297. doi: 10.1007/s10021-010-9409-3
Raciti, S. M., Hutyra, L. R., Rao, P., and Finzi, A. C. (2012). Inconsistent definitions of “urban” results in different conclusions about the size of urban carbon and nitrogen stocks. Ecol. Applic. 22, 1015–1035. doi: 10.1890/11-1250.1
Rasse, D. P., Rumpell, C., and Dignac, M.-F. (2005). Is soil carbon mostly root carbon? Mechanisms for specific stabilization. Plant Soil 269, 341–356. doi: 10.1007/s11104-004-0907-y
R Core Team (2015). R: A Language and Environment for Statistical Computing. Vienna: R Foundation for Statistical Computing.
Rousk, J., Bååth, E., Brookes, P. C., Lauber, C. L., Lozupone, C., Capporaso, J. G., et al. (2010). Soil bacterial and fungal communities across a pH gradient in an arable soil. ISME J. 4, 1340–1351. doi: 10.1038/ismej.2010.58
Selonen, S., and Setälä, H. (2015). Soil processes and tree growth at shooting ranges in a boreal forest reflect contamination history and lead-induced changes in soil food webs. Sci. Total Environ. 518–519, 320–327. doi: 10.1016/j.scitotenv.2015.03.018
Setälä, H., Birkhofer, K., Brady, M., Byrne, L., Holt, G., de Vries, F., et al. (2014). Urban and agricultural soils: conflicts and trade-offs in the optimization of ecosystem services. Urban Ecosyst. 17, 239–253. doi: 10.1007/s11252-013-0311-6
Short, J. R., Fanning, D. D., and Patterson, J. C. (1986). Soils of the Mall in Washington, DC: I Statistical summary of properties. Soil Sci. Soc. Am. J. 50, 699–705. doi: 10.2136/sssaj1986.03615995005000030030x
Swift, M. J., Heal, O. W., and Anderson, J. M. (1979). Decomposition in Terrestrial Ecosystems, 1st Edn. Oxford: Blackwell Scientific Publications.
Ukonmaanaho, L., Merilä, P., Nojd, P., and Nieminen, T. M. (2008). Litterfall production and nutrient return to the forest floor in Scots pine and Norway spruce stands in Finland. Boreal Environ. Res. 13, 67–91.
Valtanen, M., Sillanpää, N., and Setälä, H. (2014). The effects of urbanization on runoff pollutant concentrations, loadings and their seasonal patterns under cold climate. Water, Air, Soil Pollut. 225, 1977. doi: 10.1007/s11270-014-1977-y
Vauramo, S., and Setälä, H. (2010). Urban belowground food-web responses to plant community manipulation – impacts on nutrient dynamics. Landsc. Urban Plann. 97, 1–10. doi: 10.1016/j.landurbplan.2010.04.004
Vauramo, S., and Setälä, H. (2011). Decomposition of labile and recalcitrant litter types under different plant communities in urban soils. Urban Ecosyst. 14, 59–70. doi: 10.1007/s11252-010-0140-9
Wall, D. H., Nielsen, U. N., and Six, J. (2015). Soil biodiversity and human health. Nature 528, 69–76. doi: 10.1038/nature15744
Wardle, D. A. (2002). Communities and Ecosystems: Linking the Aboveground and Belowground Components. Monographs in Population Biology 34. Princeton, NJ: Princeton University Press.
Wardle, D. A., Bardgett, R. D., Klironomos, J. N., Setälä, H., van der Putten, W. H., and Wall, D. H. (2004). Ecological linkages between aboveground and belowground biota, Science 304, 1629–1633. doi: 10.1126/science.1094875
Keywords: plant functional groups, soil carbon, nitrogen and organic matter concentration, soil ecosystem services, urban park age, soil depth
Citation: Setälä HM, Francini G, Allen JA, Hui N, Jumpponen A and Kotze DJ (2016) Vegetation Type and Age Drive Changes in Soil Properties, Nitrogen, and Carbon Sequestration in Urban Parks under Cold Climate. Front. Ecol. Evol. 4:93. doi: 10.3389/fevo.2016.00093
Received: 14 April 2016; Accepted: 20 July 2016;
Published: 03 August 2016.
Edited by:
Galina Churkina, Institute for Advanced Sustainability Studies, GermanyReviewed by:
Wayne C. Zipperer, United States Department of Agriculture Forest Service, USAOlyssa Starry, Portland State University, USA
Copyright © 2016 Setälä, Francini, Allen, Hui, Jumpponen and Kotze. This is an open-access article distributed under the terms of the Creative Commons Attribution License (CC BY). The use, distribution or reproduction in other forums is permitted, provided the original author(s) or licensor are credited and that the original publication in this journal is cited, in accordance with accepted academic practice. No use, distribution or reproduction is permitted which does not comply with these terms.
*Correspondence: Heikki M. Setälä, aGVpa2tpLnNldGFsYUBoZWxzaW5raS5maQ==
Nan Hui, bmFuLmh1aUBoZWxzaW5raS5maQ==