- 1Department of Earth Sciences, Montana State University, Bozeman, MT, USA
- 2Montana Institute on Ecosystems, Montana State University, Bozeman, MT, USA
- 3Archaeology and Natural History, College of Asia and the Pacific, Australian National University, Canberra, ACT, Australia
On centennial to millennial timescales fire regimes are driven by climate changes, vegetation composition and human activities. We reconstructed the postglacial vegetation and fire history based on pollen and charcoal data from a small lake in Cradle Mountain National Park and investigated the influence that climate, people, and vegetation had on past fire regimes. In the late-glacial period, a slowly warming climate led to a shift in vegetation from coniferous alpine shrubland to Phyllocladus woodland. During this period, fire activity was very low. The initial increase in fires occurred between 12,500 and 11,000 cal yr BP and led to a decline in forest taxa, a resurgence of grasses and a rise in the pyrophytic buttongrass Gymnoschoenus. The highest fire activity in the record occurred between 10,900 and 9400 cal yr BP, the warmest interval of the postglacial period based on independent proxy records. Subalpine trees had depressed levels of pollen during this time. After 9000 cal yr BP, fire activity declined substantially, and fire-sensitive rainforest reached its maximum extent ca. 8500–6500 cal yr BP. A major fire perturbation occurred ca. 3600 cal yr BP, and thereafter rainforest shifted to open Eucalyptus woodland. The comparison of reconstructed fire and vegetation history at Wombat Pool to climate records and archeological data indicated that climate was the primary driver of the observed changes. In the late glacial and early Holocene, climate warming and individual species dispersal traits likely drove changes in vegetation composition that in turn impacted the fire regime. A relatively wet mid-Holocene climate favored rainforest trees whereas the drier and more variable climate of the late Holocene contributed to a decline in rainforest and a shift toward mixed forest as wet sclerophyll elements increased. Archeological evidence suggests humans reoccupied the region ca. 4000 cal yr BP. This may have added an ignition source that was absent in the previous ca. 7000 years and may have contributed to the large fire event ca. 3600 cal yr BP. Although pre-European populations may have been a source of ignition locally, the reconstructed fire history trends from Cradle Mountain National Park match well with large-scale changes in climate patterns.
Introduction
Fire is an important element of the Australian ecosystem that affects vegetation composition and landscape structure (Jackson, 1968, 1999; Bowman, 2000; Wood and Bowman, 2012). Changes in fire frequency have long been recognized as an important driver of ecological change (Jackson, 1968), governing the relative abundance of rainforest, shrubland, and dry Eucalyptus forest. Variations in fire occurrence through time have been attributed to past changes in climate as well as to deliberate burning by humans who have inhabited the island for at least the last 40,000 years (Cosgrove, 1999). Detailed knowledge of fire history in Tasmania, however, is limited, with only four high-resolution charcoal-based studies (Fletcher et al., 2014a,b, 2015; Rees et al., 2015) for the entire island.
The ecological history of northwestern Tasmania, in particular, is poorly known, with the exception of notable studies by Colhoun and van de Geer (1986), Colhoun et al. (1999), and Anker et al. (2001). This mountainous region supports significant tracts of intact subalpine communities, which contain endemic old-growth temperate rainforest trees and coniferous shrubs. In recent decades, subalpine communities have become increasingly fragmented as a result of fire, which is a concern for conservation and forest management (Brown et al., 1983; Kirkpatrick, 1984b; Kirkpatrick and Dickinson, 1984; Kirkpatrick and Balmer, 1991; Johnson and Marsden-Smedley, 2002; Kirkpatrick and Bridle, 2013). Fire is an essential part of these communities (Holz et al., 2015), but little information exists about the long-term relationships between fire, vegetation, and climate. In this study, we describe a high-resolution charcoal and pollen record from a small lake to reconstruct fire activity and vegetation changes over the last 17,000 years in the subalpine region. Wombat Pool (41.65°S, 145.95°E, 998 m elevation) is a small (0.4 ha), high-elevation lake in the northern section of the Cradle Mountain-Lake St Clair National Park, part of the Tasmanian Wilderness World Heritage Area (Figure 1). We compare this lake sediment reconstruction with independent paleoclimatic and archeological data to discern the relative influence of climate and people in shaping the vegetation and fire history.
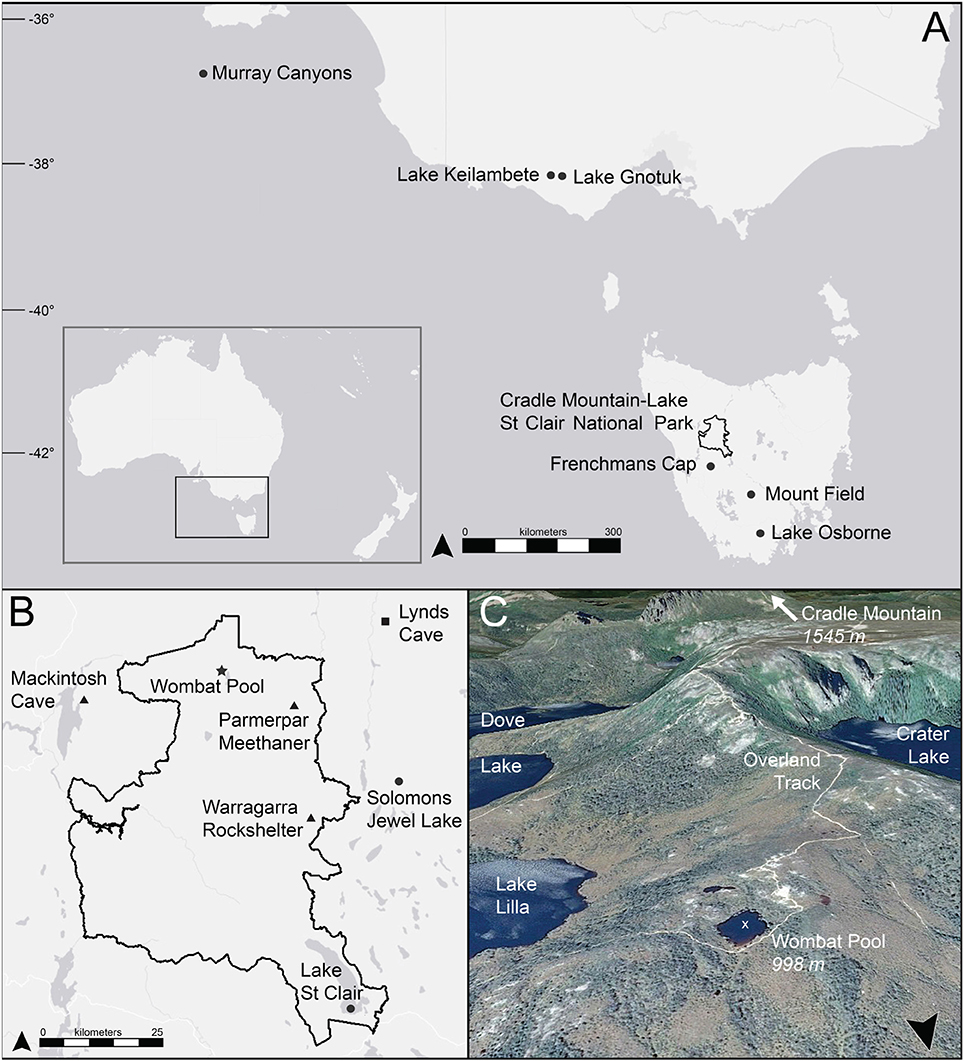
Figure 1. Location of Wombat Pool and other cites mentioned in the text. (A) Regional map; (B) map of Cradle Mountain-Lake St Clair National Park; (C) satellite image of site and surrounding area. Circle = lake or marine sediment record, triangle = archeological site, square = speleothem record, star = Wombat Pool, black line = Cradle Mountain-Lake St Clair National Park boundary, white x = coring location.
Study Area
Climate
Climate information for the northern part of Cradle Mountain-Lake St Clair National Park comes from the Waldheim station at Cradle Valley (903 m; Australian Bureau of Meteorology, http://www.bom.gov.au/climate/averages/tables/cw_096005.shtml). From 1917 to 2009, average winter (JJA) minimum temperature was −0.3°C and average summer (DJF) maximum temperature was 16.3°C. Precipitation is associated with the strength and latitudinal position of the Southern Hemisphere Westerly Winds (SWW), which influence the position of the storm tracks. A strong west-to-east precipitation gradient exists on the island and the presence of western mountains results in an orographic rainfall effect at Cradle Mountain. Average annual precipitation in the national park is 2651 mm, and almost twice as much precipitation is delivered in winter (297 mm in JJA) as in summer (151 mm in DJF). Modern studies have shown a correlative relationship between precipitation anomalies and both El Ninao/Southern Oscillation (ENSO) and Southern Annular Mode (SAM; Hendon et al., 2007; Hill et al., 2009; Risbey et al., 2009). In northern Tasmania, ENSO explains between 10 and 40% of rainfall variability and the relationship is strongest in winter (Risbey et al., 2009). The positive SAM phase is associated with reductions in winter rainfall in Tasmania, while the negative SAM is associated with increases in rainfall in spring and summer (Hendon et al., 2007).
A strong relationship exists in Tasmania between annual area burned and October–May (i.e., spring, summer, and fall) rainfall (Nicholls and Lucas, 2007). Large fire events in recent decades have been associated with abnormally low humidity and high temperatures, and strong gusty winds in the summer months. Typically, this occurs when northwesterly winds come from the heart of the Australian continent and pass over Tasmania (Nicholls and Lucas, 2007).
Vegetation
Cradle Mountain-Lake St Clair National Park is located in the transition zone between the drier, more fertile east and the wet, infertile region to the west. A mix of dolerite and quartzite substrates with areas of granite comprises the majority of the Park. The vegetation is diverse and composed of a mosaic of pyrophytic and pyrophobic assemblages. One-third of Tasmania's vascular flora are present (Kirkpatrick and Balmer, 1991), including the culturally and ecologically important, endemic conifer trees Athrotaxis selaginoides and A. cupressoides. Cradle Mountain is also one of the few areas in Tasmania where the island's alpine shrub species co-occur (Podocarpus lawrencii, Microcachrys tetragona, Diselma archeri, Microstrobus niphophilus, Nothofagus gunnii, and the two Athrotaxis species; Kirkpatrick and Bridle, 2013). In Tasmania, climatic treeline occurs where the mean temperature of the warmest month is 10°C, which is at approximately 1100 m elevation (Kirkpatrick, 1984a). Downslope of the alpine community are extensive tracks of rainforest, wet sclerophyll forests and patches of waterlogged moorland. Many different rainforest types persist in the area and all are classified as “extremely fire sensitive,” meaning there is a very high potential ecological impact of a single fire in these communities (Pyrke and Marsden-Smedley, 2005). Where growth rates are highest, Nothofagus cunninghammii forms near-monospecific stands; where growth rates slow, Athrotaxis spp. become dominant. Where growth rates are very slow, N. gunnii dominates (Kirkpatrick and Balmer, 1991). Two species of Eucalyptus are important components of the flora in this region. Woodlands of E. subcrenulata interspersed with open scrub, and forest woodlands of E. coccifera form a mosaic with rainforest and moorland dominated by the perennial sedge Gymnoschoenus sphaerocephalus (Kirkpatrick 1984a). Other abundant genera include Leptospermum, Richea, Dicksonia, Melaleuca, Banksia, Pomaderris, Orites, and Astelia. A number of Asteraceae, Poaceae, Restionaceae, and Ericaceae are also present.
Wombat Pool is surrounded by a mosaic of wet sclerophyll forest and shrub communities, areas of buttongrass (Gymnoschoenus species), mixed forest containing rainforest and Eucalyptus species, and alpine shrubland. Athrotaxis cupressoides of different age classes grow directly on the edge of this small lake. Within 100 m of the lake, a woodland community with Eucalyptus coccifera overstory and shrub understory was present. Shrubland with plants from the Proteaceae, Myrtaceae, Ericaceae, and other families, as well as buttongrass tussocks occur within 100 m of the lake. Within 500 m of the site lie patches of mixed forest, containing rainforest species (N. cunninghammii, Phyllocladus, and A. selaginoides), and areas of alpine vegetation with the woody shrubs P. hookeriana and D. archeri.
Materials and Methods
Field
A 331-cm-long sediment core was obtained from Wombat Pool in 2011 using a modified Livingstone piston sampler. The mud-water interface and top 25 cm of sediment was captured with a plastic piston corer. Coring was performed from an anchored platform in the deepest part of the lake (2 m). Cores were extruded in the field and shipped to the National Lacustrine Core Facility (LacCore) at the University of Minnesota.
Lithology
Initial core descriptions were performed at LacCore. Cores were split lengthwise with one half reserved as an archival half and the other used as the working half. The working half was described, imaged and analyzed. The archival halves of the cores are housed at the Paleoecology Laboratory at Montana State University. Density (g cm−3) was measured at 0.5-cm continuous intervals on the split surface of the cores and used as an indicator of changes in porosity and lithology. Magnetic susceptibility (MS) was also measured at 0.5-cm continuous intervals. MS measures changes in inorganic allochthonous sediment input from erosional processes (Gedye et al., 2000).
Chronology
Bulk sediment samples were submitted to Woods Hole NOSAMS AMS facility for radiocarbon dating. Radiocarbon ages were calibrated using the Southern Hemisphere radiocarbon calibration dataset SHCal13 (Hogg et al., 2013). Chronologies were developed by modeling sediment age as a function of sediment depth. Two-sigma calibrated ages and probability distributions were determined for each radiocarbon date using Clam v2.2 (Blaauw, 2010). The age-depth modeling was performed using a smoothing spline (error-weighted with smoothness set at 0.4) and a repeated sampling of point estimates approach (1000 iterations) that allows each date to influence the age model through the probability density function of the calibrated age. Although Bayesian (i.e., Bacon; Blaauw and Christen, 2011) methods were also considered for age-depth modeling, Clam software was used in this study because it employs smoothing functions that result in a smooth curve for the median sample ages.
Charcoal Analysis
In order to reconstruct local fire history (i.e., within a few kilometers of the site), charcoal analysis was performed on 2-cm3 volume samples taken at continuous 0.5-cm intervals following the method described by Whitlock and Larsen (2001). Samples were wet-screened through a 125-μm mesh sieve and charcoal particles were counted under a stereomicroscope at 40x magnification. This size fraction was chosen because modern studies have shown particles of this size class do not travel far from their source and can be employed to reconstruct watershed-scale fires (Clark, 1988; Whitlock and Larsen, 2001). Charcoal concentrations and deposition times were calculated and converted to charcoal accumulation rates (CHAR; particles cm−2 yr−1). To account for temporal variations in sampling intervals resulting from variable sedimentation rates, the CHAR time series was interpolated to 30 years, the median resolution of the record (Higuera et al., 2010). CHAR was then decomposed into background (BCHAR) and peak components (Higuera et al., 2009; http://CharAnalysis.googlepages.com) with a 1250-yr lowess smoother robust to outliers. A mixed Gaussian model was used to decompose the high frequency component of the time series into “noise” and “peaks,” the peak component being the 95th percentile of the noise population (Higuera et al., 2009). Peaks were tested for statistical significance by comparing the original charcoal counts with the values of the previous five samples (150 yr). Where the peak count had at least a 0.5% chance of coming from the same Poisson-distributed population as the smallest number of charcoal particles counted in the preceding 150 years then the peak was considered insignificant (Higuera et al., 2009). Charcoal peaks are assumed to represent one or more fires during the time span of the peak (30 yr). The suitability of the records for fire-episode identification was assessed with the signal-to-noise index (SNI); only periods where SNI >3 were considered appropriate for charcoal peak interpretation (Kelly et al., 2011). A 1000-yr bandwidth was used to calculate the SNI. Fire size and/or intensity were inferred from the magnitude of individual charcoal particle count peaks (Higuera et al., 2009). The fire history was divided into fire regime zones (Fire Intervals, FI) applying a regime shift index (RSI) algorithm described by Rodionov (2004) to the CHAR time series. It incorporated a sequential Student's t-test (Huber's WF = 5, P > 0.01, cut-off = 70 yr) and determined where significant changes in the mean values of two adjacent regimes occur. These transitions were used to delineate fire regime intervals. Plots were made in R version 3.2.2 (R Core Team, 2015).
Pollen Analysis
Sediment samples of 0.25–1.0 cm3 in volume were taken at <300-yr intervals (median sample resolution = 196 yr) for pollen analysis. Samples were prepared according to the standard KOH, HF, HCl and acetolysis method described by Bennett and Willis (2001). A Lycopodium tracer was added to each sample in order to calculate the pollen concentration (grains cm−3) and accumulation rate (PAR; grains cm−2 yr−1). Identification was performed at 400 and 1000x magnification. Analysis was partially conducted at Australian National University using reference collections for Australian taxa housed by the Australasian Pollen and Spore Atlas (apsa.anu.edu.au; APSA-Members., 2007) and the Newcastle Pollen Collection (geo.arizona.edu/palynology/nsw; Shimeld et al., 2000). Pollen counts exceeded 350 terrestrial grains per sample (median = 389) and pollen percentages were based on the terrestrial sum. Interpretation of the pollen data was based on a comparison with modern pollen data from lake-sediment samples in the Cradle Mountain region and with the extensive dataset published by Fletcher and Thomas (2007). Plots were made using the program Tilia v2.0 (Grimm, 1990). The pollen-percentage record was divided into zones using constrained cluster analysis (CONISS; Grimm, 1988).
Results
Lithology
The core lithology consisted of two units. Unit 1 (331−273 cm depth; older than 16,540 cal yr BP; Figure 2) was inorganic glacial silt and clay. This unit had high magnetic susceptibility (−0.9 to 2.0 SI units; mean = 0.624) and density (1.165−1.949 g cm−3; mean = 1.667), indicating substantial mineral clastic input during this period. Unit 2 (272.5 to 0 cm depth; 16,540 to −61 cal yr BP) was composed of fine-detritus gyttja and featured low readings of magnetic susceptibility (−2.2 to 0.3 SI units; mean = −1.478) and density (0.847−1.237 g cm−3; mean = 0.944).
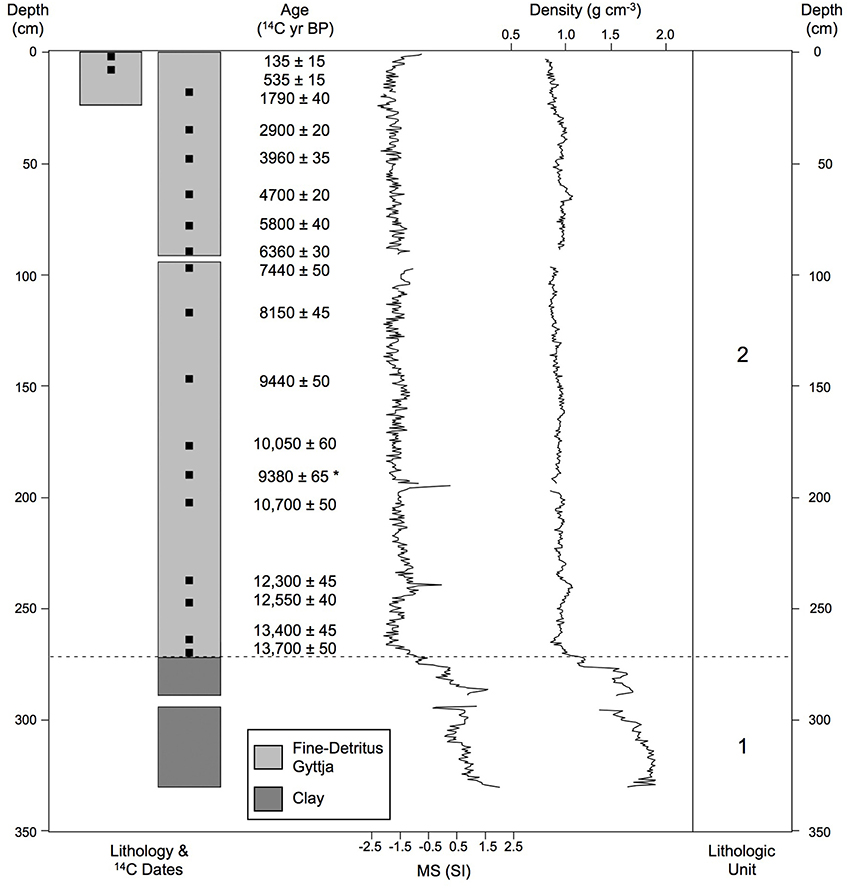
Figure 2. Lithology. Lithology, radiocarbon dates, magnetic susceptibility, and density for the Wombat Pool cores. The asterisk indicates the date that was not used in the chronology.
Chronology
Eighteen bulk sediment samples from Wombat Pool were submitted for AMS radiocarbon dating analysis (Table 1). One sample was excluded from construction of the age-depth model, because it was judged to be anomalously young. A preliminary model was developed by independently modeling age as a function of depth in each core. The final age-depth model was developed by Monte Carlo fitting smoothing splines through the probability distribution of all calibrated ages (Figure 3). We developed two alternative chronologies based on AMS radiocarbon dates by applying different degrees of smoothing. The preferred chronology allowed for changes in sedimentation rates across different lithologies (Figure 2). The extrapolated age of the core is 20,150 cal yr BP. This age estimate is likely too old, inasmuch as sedimentation rates during deglaciation were probably higher than the extrapolation would imply.
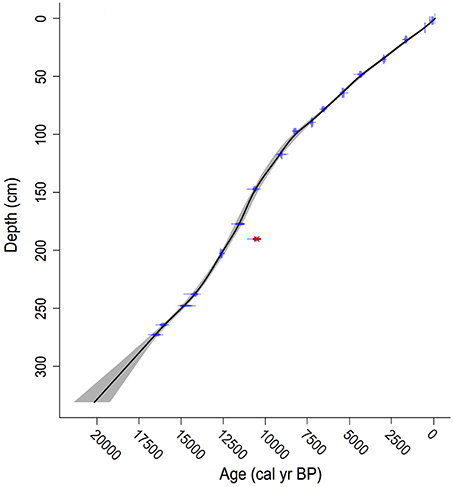
Figure 3. Age-depth model. Age-depth model for Wombat Pool (black line). The 95% confidence intervals are shown as gray shading. The distributions of the calibrated radiocarbon ages that were used to develop the model are shown in blue, and the date that was not included in the model is indicated in red.
Pollen
Pollen Zone 1 (281.0−262.5 cm depth; 17,055−15,865 cal yr BP) featured high percentages of Poaceae (14−30%) and Asteraceae (12−21%; Figure 4). Chenopodiaceae percentages were 3−6% and Allocasuarina values fluctuated between 3 and 8%. Subalpine shrubs were moderately represented, including M. tetragona (2−5%), Ericaceae (3−5% after 272.5 cm; ca. 16,500 cal yr BP), Athrotaxis-Diselma (1−5%), and other subalpine taxa (P. hookeriana, P. lawrencii, and Tasmannia lanceolata; up to 2%). Below 265.5 cm depth (16,000 cal yr BP), Gymnoschoenus percentages range between 4 and 5%, which is significant as buttongrass is a very poor pollen disperser; values as low as 1% have been recorded where the plant is locally abundant (Fletcher and Thomas, 2007). Cyperaceae percentages reached 8% in Zone 1, which were the highest of the record. Comparisons with modern pollen data suggest that the vegetation corresponding to Zone 1 consisted of a coniferous alpine shrubland and buttongrass moorland in the local vicinity of Wombat Pool, and open, grass, and herb vegetation was likely present in the extralocal area (Harris and Kitchener, 2005; Fletcher and Thomas, 2007).
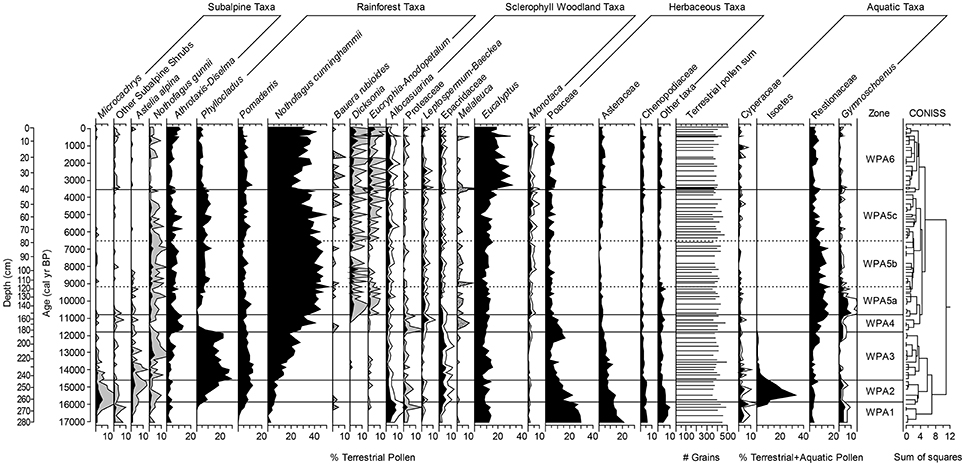
Figure 4. Pollen diagram for percentage of selected taxa from Wombat Pool. Note differing scales on x-axes. Stippled gray shading indicates 10x exaggeration (i.e., Bauera rubioides and Melaleuca), solid gray shading indicates 5x exaggeration, and white shading indication 2x exaggeration. Isoetes values were divided by 50 to aid readability.
Pollen Zone 2 (262.5−244.5 cm depth; 15,865−14,600 cal yr BP) had declining values of Poaceae (12−23%), Asteraceae (7−14%), M. tetragona (0.5−3%), other subalpine shrubs (up to 2%), Allocasuarina (2−4%), and Chenopodiaceae (4−5%), and increasing percentages of Phyllocladus (7−19%) and Pomaderris (7−12%). Astelia alpina, a poor pollen-disperser, reached the highest percentages of the record (up to 3%). Cyperaceae percentages oscillated up to 5% during this period. This pollen assemblage suggests that the vegetation of this period resembled a modern coniferous subalpine shrub community with a significant sedgeland component (Harris and Kitchener, 2005).
Pollen Zone 3 (244.5−183.5 cm depth; 14,600−11,800 cal yr BP) featured Phyllocladus as the dominant pollen taxa (14−29%), and values were highest between 244.5 and 232.5 cm depth (ca. 14,500−14,000 cal yr BP). Pomaderris pollen was initially fairly abundant (9−13%) but percentages declined above 212.5 cm depth (ca. 13,000 cal yr BP). Asteraceae percentages were lower than in the previous two pollen zones, and values of 7−11% were reached below 218.5 cm depth (ca. 13,200 cal yr BP) but declined to 5% above that depth. N. gunnii, a short-distance pollen-disperser, reached values of up to 5% between 218.5 and 195.0 cm depth (ca. 13,000–12,000 cal yr BP). Poaceae percentages were low (4−7%) but increased dramatically at 195.0 cm depth (ca. 12,200 cal yr BP) to 17%. Astelia and Cyperaceae declined in abundance from the beginning of this period until 229.5 cm depth (ca. 13,800 cal yr BP) and were present only in trace amounts after. Pollen Zone 3 suggests a period of mixed rainforest with cold-tolerant taxa near the lake and widespread coverage of the pioneer rainforest species Phyllocladus (Harris and Kitchener, 2005).
Pollen Zone 4 (183.5−153.0 cm depth; 11,800−10,800 cal yr BP) featured a steep decline in the percentages of Phyllocladus pollen to a low of 1%, high levels of Athrotaxis-Diselma (9−14%), increased Poaceae (up to 14%), and the appearance of short-distance pollen-dispersing woody shrubs Leptospermum-Baeckea, Melaleuca and Proteaceae (Banksia marginata, Orites-Bellendena, Persoonia, Telopea; 4−7%). Gymnoschoenus pollen percentages increased at 195.5 cm depth (12,300 cal yr BP) and reached values of 3.4%.
Pollen Zone 5 (153.0−40.5 cm depth; 10,800−3565 cal yr BP) includes three sub-zones. Subzone 5a (153.0−119.5 cm depth; 10,800–9200 cal yr BP) featured the highest percentages of Gymnoschoenus pollen (up to 10%) and a steady presence of pollen of Leptospermum-Baeckea, Pomaderris, and Monotoca (2−3, 5−11, and 1−3%, respectively). Temperate rainforest taxa including Eucryphia-Anodopetalum and the tree fern Dicksonia antarctica appeared in higher than trace amounts during this pollen zone. N. cunninghammii pollen also increased and by 135.0 cm depth (10,000 cal yr BP) reached >40% indicating a local presence (Fletcher and Thomas, 2007). This pollen assemblage suggests that the vegetation resembled modern buttongrass moorland with woody shrub components and rainforest elements (Harris and Kitchener, 2005).
Sustained high levels of N. cunninghammii (39−52% and mostly >40%) dominated Subzone 5b (119.5−78.0 cm depth; 9200−6500 cal yr BP). Pomaderris was lower (3−5%) and Gymnoschoenus fell to values of 0−2%. Percentages of the subalpine tree N. gunnii were primarily between 2 and 4% and Athrotaxis-Diselma fluctuated between 3 and 10%. This pollen assemblage suggests development modern temperate rainforest (Harris and Kitchener, 2005).
At the beginning of Subzone 5c (78.0−40.5 cm depth; 6500−3565 cal yr BP), N. cunninghammii dropped below 40% and percentages became more variable suggesting the population may have moved farther from the lake at this time and/or become more sparse. Throughout this zone, N. cunninghammii fluctuated between 28 and 49% (mean = 37%). Pomaderris reached up to 10% of the terrestrial sum in Subzone 5c (78.0−40.5 cm depth; 6500–3565 cal yr BP), while levels of Athrotaxis-Diselma oscillated dramatically—as low as 2.5% and as high as 12.3%. N. gunnii percent fell below 2%.
Pollen Zone 6 (40.5 to 0 cm depth; 3565 to –61 cal yr BP) marks the dominance of Eucalyptus pollen, especially between 40.5 and 22.5 cm depth (2000–3500 cal yr BP) when it reached levels of 19−31%. N. cunninghammii dropped to 19−29%, but this taxon is strongly over-represented in the regional pollen rain, and these percentages do not indicate a local presence (Fletcher and Thomas, 2007). The pollen assemblage between 40.5 and 22.5 cm (2000–3500 cal yr BP) suggests the establishment of a modern wet sclerophyll forest dominated by Eucalyptus spp. (Harris and Kitchener, 2005). After 22.5 cm depth (2000 cal yr BP), Eucalyptus and N. cunninghammii percentages were more variable; however, N. cunninghammii persisted at low values and only reached >40% once at 5.5 cm depth (ca. 200 cal yr BP). In the last ca. 800 years (10.5−0 cm depth), Athrotaxis-Diselma pollen was higher than it was in the previous 4000 years, reaching percentages of up to 11%. Dicksonia also reached the highest values of the record (4–6%). In uppermost 2.5 cm of the core (last 100 years) Eucryphia-Anodopetalum pollen reached values of 5–6%, which was its best representation of the record. The data suggest the presence of a mixed forest with wet sclerophyll elements as well as subalpine and temperate rainforest (Harris and Kitchener, 2005) during the last 2000 years.
Charcoal
Throughout the record, there is a close correspondence between the timing of shifts between fire intervals (FI) and pollen zones, however, the shifts do not occur at exactly the same time (Figure 5). This section describes the results of charcoal analysis. Fire interval (FI) 1 (281.0−246.0 cm depth, 17,055–14,720) had a mean CHAR value of 0.06 particles cm−2 yr−1. Fires were very infrequent and four small fire events were identified in this period (mean = 9.11 particles cm−2 peak−1). FI 2 (246.0–188.0 cm depth; 14,720–11,990 cal yr BP; mean CHAR = 0.43 particles cm−2 yr−1) was characterized by low levels of biomass burning with more (3.5–5/1000 yr) local fires than before. Twelve fire episodes were identified (11 with SNI > 3.0; mean = 7.50 particles cm−2 peak−1). FI 3 (188.0–155.5 cm depth; 11,990–10,880 cal yr BP) had much higher rates of charcoal accumulation than the previous two intervals (CHAR mean = 2.465 particles cm−2 yr−1). The shift from FI 2 to 3 was marked by a large steplike increase in charcoal levels. Three fire episodes were recorded in FI 3, but the low SNI suggests that it is difficult to determine whether these were local fire events (Kelly et al., 2011).
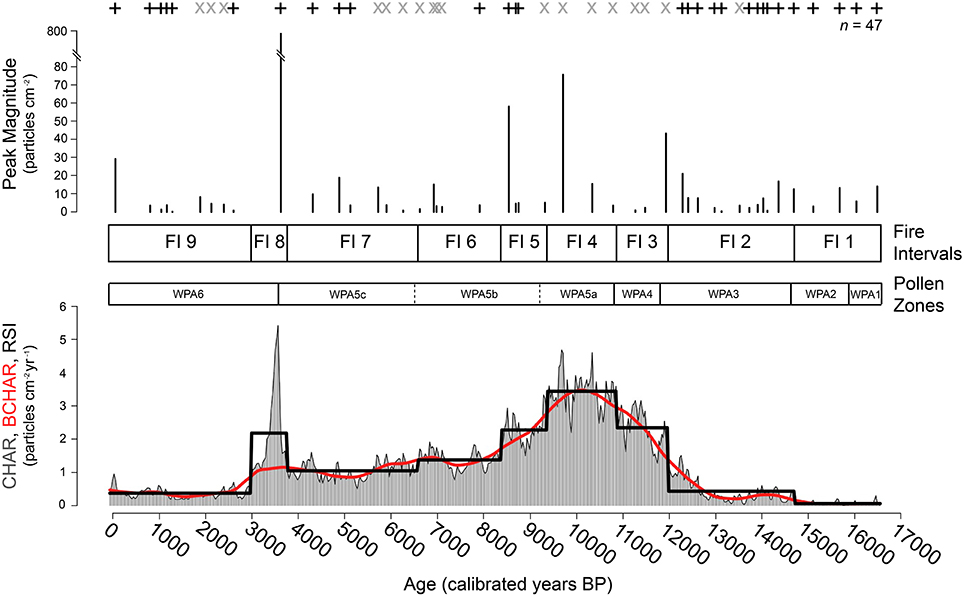
Figure 5. Charcoal summary. Charcoal data for Wombat Pool. Charcoal accumulation rate (CHAR; gray histogram), background CHAR (red line), charcoal peaks (signal-to-noise ratio >3.0 = +, signal-to-noise ratio <3.0 = ×), peak magnitude, and signal-to-noise ratio were determined using CharAnalysis (Higuera et al., 2009). Solid black line on bottom figure indicates Fire Intervals derived from regime shift index algorithm (RSI; Rodionov, 2004). Fire Intervals (FI 1-9) and Pollen Zones (WPA1-6) are displayed.
FI 4 (155.5–122.0 cm depth; 10,880–9380 cal yr BP; mean CHAR = 3.44 particles cm−2 yr−1), had the highest levels of biomass burning of the record. None of the three fire episodes identified in this period had >3.0 SNI. FI 5 (122.0–102.5 cm depth; 9380–8390 cal yr BP; mean CHAR = 2.275 particles cm−2 yr−1) had high biomass burning and two of the largest magnitude peaks of the record (8690 ± 15 cal yr BP with 40.695 particles cm−2 peak−1 and 8540 ± 15 cal yr BP with 58.236 particles cm−2 peak−1). Biomass burning was substantially reduced in FI 6 (102.5–79.5 cm depth; 8390–6590 cal yr BP; mean CHAR = 1.375). FI 7 (79.5–53.0 cm depth; 6590–3770 cal yr BP; mean CHAR = 1.048 particles cm−2 yr−1) featured low biomass burning. Three of the fire episodes identified in this period had SNI values that exceed 3.0 (mean = 10.80 particles cm−2 peak−1).
A fire regime shift to higher biomass burning occurred at 53.0 cm depth (3770 cal yr BP). FI 8 (53.0–34.5 cm depth; 3770–2990 cal yr BP; mean CHAR = 2.18 particles cm−2 yr−1) indicates a period of fires, punctuated by the largest charcoal peak of the entire record at 41.0 cm (3620 ± 15 cal yr BP; 764.671 particles cm−2 peak−1). In the 800 years after this large fire event, pollen of Gymnoschoenus, Poaceae, Asteraceae, and Pomaderris increased in abundance and Melaleuca and Monotoca pollen had the highest values of the record. An interval of low biomass burning and low fire frequency characterized the fire regime of the last ca. 3000 years (FI 9—34.5: 0 cm depth; 2990: –61 cal yr BP; mean CHAR = 0.374 particles cm−2 peak−1).
Discussion
Late-Glacial Period (ca. 17,000–14,500 cal yr BP)
The Last Glacial Maximum (LGM) in Tasmania was relatively short-lived and ice cover was geographically confined. Ice caps were present in the Central Plateau and West Coast Ranges, and systems of valley and cirque glaciers formed on surrounding mountains, including at Cradle Mountain (Barrows et al., 2002; O'Donnell, 2010). Rapid contraction of glaciers occurred between ca. 18,000 and 16,000 cal yr BP with most locales becoming ice-free by 16,000 cal yr BP (Mackintosh et al., 2006). At Wombat Pool, the catchment was unstable and had poorly developed soils prior to ca. 16,000 cal yr BP as evidenced by the inorganic composition of the sediments (silt and clay) and elevated values for density and magnetic susceptibility. The low level of biomass burning was partly a result of sparse discontinuous fuels. After ca. 16,000 cal yr BP, the catchment likely became stabilized as evidenced by the change in lithology to organic gyttja and the decrease in MS and density values.
The pollen data suggest that shrubs and herbs dominated the local vegetation. Moderate values for A. alpina, M. tetragona, Gymnoschoenus, and Ericaceae suggest both alpine and moorland communities surrounded the lake, whereas high values for grasses and Asteraceae taxa and moderate amounts of Chenopodiaceae indicate the extralocal vegetation contained considerable areas of herbaceous cover (Macphail, 1975). Pollen from N. cunninghammii and Eucalyptus was present in small amounts, and based on glacial refugia estimates (Kirkpatrick and Fowler, 1998) and modern pollen-vegetation relationships (Fletcher and Thomas, 2007), were likely transported moderate to long distances before being deposited at Wombat Pool. About 15,000 cal yr BP, Phyllocladus aspleniifolius, the early-colonizing rainforest species started to increase and by 14,500 cal yr BP was an important part of the local vegetation.
Evidence for cool conditions at this time comes from marine, ice, and pollen data from the region (Figure 6). Sea-surface temperatures (SSTs) inferred from alkenones and faunal assemblages in marine cores obtained south of Tasmania (44–45° S) and in the Murray Basin region northwest of Tasmania (36° S) were higher than the glacial period but lower than later Holocene levels (Calvo et al., 2007; Sikes et al., 2009). Likewise, Antarctic air temperature, based on δ18O data, remained low (Grootes et al., 2001; EPICA Members., 2004). High levels of Poaceae and Asteraceae and low levels of tree taxa characterized many of the pollen records from Tasmania and the southeastern Australian mainland during this period (Colhoun and van de Geer, 1986; D'Costa et al., 1989; Colhoun et al., 1999; Hopf et al., 2000; Colhoun and Shimeld, 2012).
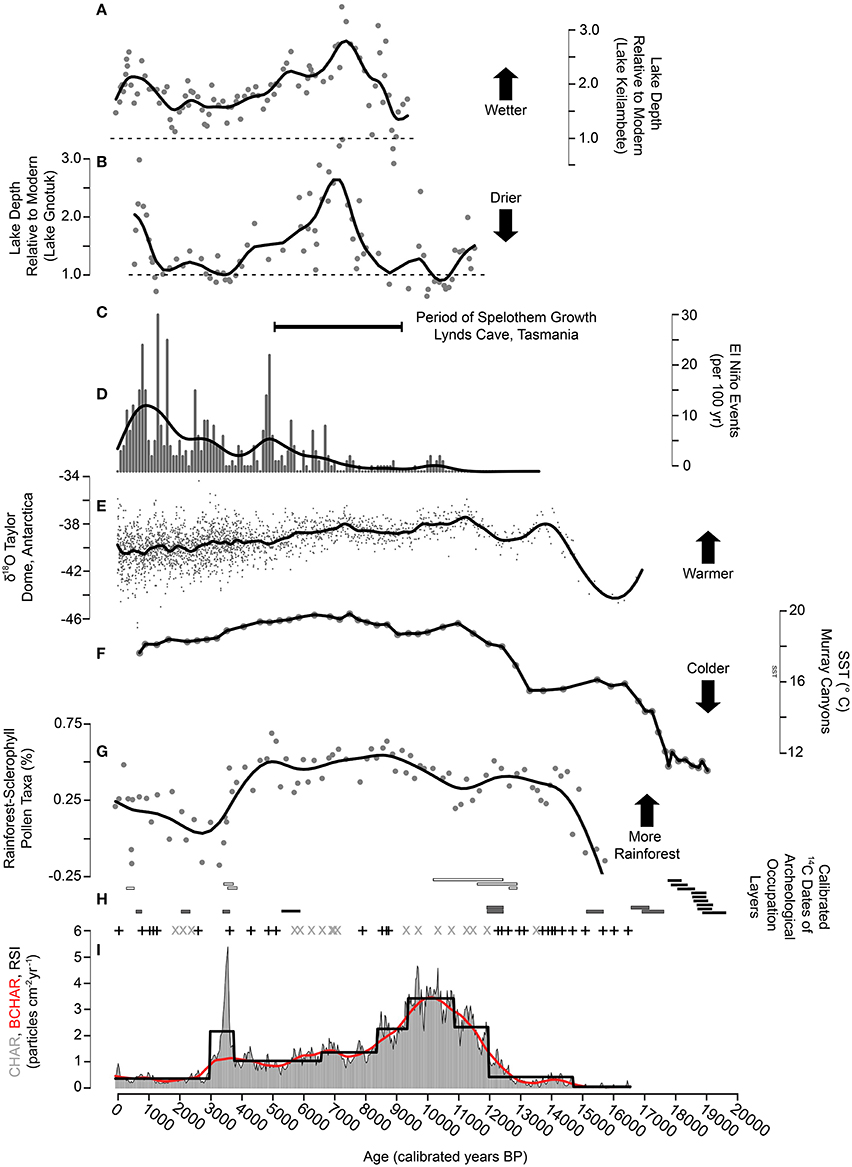
Figure 6. Climate, Fire, Vegetation, and Human Occupation Summary. (A,B) Modeled reconstructions of lake depths relative to modern (modern depth = dashed line) for Lake Keilambete and Lake Gnotuk in southern Victoria, Australia (Wilkins et al., 2013). (C) The stalagmite growth record Lynds Cave, Tasmania. This record spans 5000–9200 cal yr BP (Xia et al., 2001). (D) The number of El Niño events per 100 years (Moy et al., 2002) with smoothing spline (spar = 0.6). (E) Oxygen isotope ratios from Taylor Dome, Antarctica (Grootes et al., 2001) with smoothing spline (spar = 0.6). (F) Alkenone-derived sea surface temperature reconstruction from marine core MD03-2611 at Murray Canyons off the coast of South Australia (Calvo et al., 2007). (G) The ratio of forest pollen taxa to non-forest pollen taxa in the terrestrial pollen record from Wombat Pool, Tasmania with smoothing spline (spar = 0.6). (H) The 1σ calibrated age range of 14C dates on occupation layers at three archeological sites within 35 km of Wombat Pool (Mackintosh Cave = black bars, Warragarra rockshelter = white bars, Parmerpar Meethaner rockshelter = gray bars). (I) Charcoal accumulation rate (gray histogram), background CHAR (red line), regime shift index (black line), charcoal peaks (signal-to-noise ratio >3.0 = +, signal-to-noise ratio <3.0 = ×) from Wombat Pool, Tasmania.
Although an abundance of ethnographic evidence from Sixteenth to eighteenth centuries indicates Aboriginal Tasmanians frequently and adeptly used fire to shape the Tasmanian landscape (Gammage, 2008, 2011), little is known about how and where fire may have been used in earlier time periods. We consider human presence in the Cradle Mountain region as evidence of a potential anthropogenic fire ignition source. The first evidence of people in Tasmania is dated to ca. 39,000 cal yr BP (Allen, 1996). Three archeological sites within 35 km of Wombat Pool have been excavated and radiocarbon dated (Figures 1, 6). Two of these, Parmerpar Meethaner and Warragarra, were first occupied about 35,000 years ago (Cosgrove, 1999; Cosgrove et al., 2010), and the third, Mackintosh Cave ca. 20,500 cal yr BP (Stern and Marshall, 1993). The three sites are located at lower elevations than Wombat Pool (250, 300, and 600 m elevation, respectively, compared with 998 m elevation). Warragarra rockshelter has evidence of sporadic, light occupation in the early and late Holocene. Parmerpar Meethaner experienced intense use between ca. 22,000 and 17,000 cal yr BP (Cosgrove, 1995). Intensive occupation occurred at Mackintosh Cave ca. 20,500–18,000–cal yr BP and was followed by apparent abandonment of the site until the late Holocene (Stern and Marshall, 1993). The period of use and occupation of Parmerpar Meethaner rockshelter and Mackintosh Cave mostly predates the beginning of the Wombat Pool record, and the interval of overlap prior to 14,500 cal yr BP (17,000–14,500 cal yr BP) was the time of lowest biomass burning at Wombat Pool (FI 1 and 2; Figure 5). The fire regime during this period is consistent with what would be expected given the climate (Whitlock et al., 2010; McWethy et al., 2013), therefore, if people were using fire in the region at this time it did not result in alternations to fire regime that deviated from the expectations given climate.
Late-Glacial to Early-Holocene Transition (ca. 14,500–11,500 cal yr BP)
Alpine and moorland communities were replaced by forests at the end of the late-glacial period. Phyllocladus was dominant between 14,500 and 11,900 cal yr BP. This early successional species is one of the few bird-dispersed plants in the Cradle Mountain region (Kirkpatrick, 1977). Phyllocladus also expanded in other parts of western Tasmania during the late-glacial period, including at Mt. Field and Lake Vera (Macphail, 1979; Markgraf et al., 1986). Modern analysis has shown that Phyllocladus pollen is over-represented in the regional pollen rain, typically comprising 4–12% of the total terrestrial pollen in open moorland and alpine communities (Fletcher and Thomas, 2007). The initial rise at Wombat Pool could be from extralocal or distant populations; however, by 14,500 cal yr BP, values were high enough to indicate local presence (Fletcher and Thomas, 2007). The wide environmental tolerance of Phyllocladus combined with its bird-dispersed seeds (Barker and Kirkpatrick, 1994; Barker, 1995) help explain the early preponderance of Phyllocladus in a variety of montane and alpine settings.
Warming during this period is inferred from marine cores from the Australian region and Antarctic air-temperature records (Figure 6; Grootes et al., 2001; EPICA Members., 2004). In addition, sea-surface temperature reconstructions in the Murray Basin northwest of Tasmania increased substantially after 13,000 cal yr BP (Calvo et al., 2007), and suggest an increase in adjacent land temperatures (Cook et al., 2000). Biomass burning increased considerably at ca. 12,000 cal yr BP (i.e., FI 3; Figure 5) and coincided with a brief resurgence in Poaceae pollen. Climate conditions would have favored both fires and grass expansion. Human use and occupation in the Cradle Mountain region is documented at Warragarra rockshelter at ca. 13,000–11,000 cal yr BP (Lourandos, 1983; Allen and Porch, 1996), and at Parmerpar Meethaner rockshelter at ca. 17,000–12,000 cal yr BP (Cosgrove, 1995). People may have provided an ignition source during this period that contributed to the observed increase in charcoal influx. Increases in temperatures during this period best explain the increase and dominance of Phyllocladus and would have also promoted fire activity.
Early Holocene (ca. 11,500–9000 cal yr BP)
At the beginning of the Holocene, warming coupled with increased aridity favored increased fires and a brief resurgance of grass. The interval of highest fire activity between 10,900 and 9400 cal yr BP was also the period with highest pollen percentages of Gymnoschoenus, Leptospermum-Baeckea, and Banksia. Buttongrass is highly flammable (i.e., burns in a wide variety of conditions; Pyrke and Marsden-Smedley, 2005), and it vegetatively reproduces allowing it to regenerate vigorously after fire (Burrows, 2002). The arrival of Banksia and Leptospermum-Baeckea in this period likely provided a local woody fuel source that increased the amount of biomass burned compared with previous periods when herbaceous taxa were dominant. A positive feedback with woody shrubs and fires may have operated during this period. The high flammability of the shrub component created conditions for its persistence and only after the climate shifted toward effectively moister conditions at 9000 cal yr BP did this relationship breakdown (Jackson, 1968).
Regional rainforest development continued in the early Holocene (Figure 6). An increase in fires at the same time as rainforest expansion is recorded at Wombat Pool as well as other Australian records (i.e., Haberle, 2005; Fletcher et al., in review). The appearance of the woody, sclerophyllous vegetation community at this time provided a local fuel source while rainforest taxa expanded regionally (Macphail, 1979; Hopf et al., 2000; Anker et al., 2001) and locally. Fires may have occurred along the rainforest-sclerophyll woodland margin and microclimate conditions prevented spread into the rainforest (i.e., Haberle 2005). Increased fire activity and increased rainforest taxa were both likely responding to warming climate but were occurring in spatially disparate areas of the regional landscape. After 11,900 cal yr BP, pollen of Phyllocladus declined and those of Athrotaxis-Diselma rose and remained abundant until 10,700 cal yr BP, probably occurring around the lake edge and on nearby south-facing slopes. During the early Holocene, steadily increasing amounts of N. cunninghammii pollen suggest its increasing abundance in the Cradle Mountain region.
Early-Holocene aridity in western Tasmania has been explained by weakened Southern Hemisphere Southwesterly Wind (SWW) flow (Fletcher and Moreno, 2012; Rees et al., 2015). During years when the SWW are positioned south of the island and/or are weak, less precipitation falls in the Cradle Mountain region (Gillett et al., 2006; Gupta and England, 2006). The weakening during the early Holocene is inferred from chironomid records from western Tasmania (Rees et al., 2015) and low lake levels in southwestern Victoria (Figure 6; Wilkins et al., 2013). The effects of higher-than-present winter and annual insolation (Berger and Loutre, 1991) may have also contributed to aridity in this period by promoting drier summers, milder winters and longer effective spring across the Southern Hemisphere mid-latitudes (Whitlock et al., 2007). Increased biomass burning during the early Holocene has been recorded at other cool-temperate western Tasmanian sites (Fletcher et al., 2015; Rees et al., 2015) and observed in many Patagonian records (Whitlock et al., 2007; Iglesias and Whitlock, 2014), suggesting the importance of large-scale climatic controls on fire activity. Evidence of a human influence on Tasmanian fire regimes is less compelling. After ca. 11,000 cal yr BP, two of the three archeological sites near Wombat Pool contain no evidence of human occupation at this time (Stern and Marshall, 1993; Allen and Porch, 1996). The third site, Parmerpar Meethaner rockshelter, showed low intensity human occupation in the early Holocene (Cosgrove, 1995).
Middle Holocene (ca. 9000–4500 cal yr BP)
Montane rainforest in the Cradle Mountain region reached its greatest Holocene extent during the middle Holocene (Figure 6). The highest levels of pollen percentages of N. cunninghammii indicate its presence at Wombat Pool between ca. 9000 and 6000 cal yr BP. In addition, N. gunnii and Athrotaxis were intermittently important during middle Holocene. Large stepwise declines in biomass burning occurred at ca. 9400 and ca. 8400 cal yr BP, and a smaller decline was recorded ca. 6600 cal yr BP (Figures 5, 6). High-resolution charcoal records from Frenchmans Cap (Fletcher et al., in review) and Mount Field (Rees et al., 2015) also indicate a Holocene minimum in fires during this period. Increased effective moisture from ca. 9000–6500 cal yr BP likely favored the expansion of rainforest taxa and reduced landscape flammability. After ca. 6500 cal yr BP, increased variability among the montane rainforest taxa occurred, suggesting canopy opening and/or fluctuating abundances of rainforest tree species possibly as a result of intermittent wet and dry intervals.
Regional climate records provide evidence for relatively wet and warm conditions during the middle Holocene (Figure 6). See surface temperature (SST) in the Murray Basin reached a Holocene maximum at ca. 8000–5000 cal yr BP with values 2.1°C higher than present between 6000 and 5500 cal yr BP (Calvo et al., 2007). After ca. 5500 cal yr BP, SSTs steadily decline to the present day (Calvo et al., 2007). The chironomid record of summer temperatures from two sites on Mount Field shows a mixed signal, with one site recording the warmest period at ca. 6700 cal yr BP and a second site showing delayed warming at ca. 4600 cal yr BP (Rees and Cwynar, 2010). Evidence from speleothems from Lynds Cave, 25 km west-northwest of Wombat Pond, suggests a Holocene thermal maximum between ca. 7400 and 8000 cal yr BP (Xia et al., 2001).
The evidence for relatively high effective moisture during the middle Holocene comes from multiple sources. Between 9200 and 5000 cal yr BP, increased speleothem growth at Lynds Cave, (Xia et al., 2001) is interpreted as evidence of wetter conditions (Zhao et al., 2001). Higher precipitation has also been inferred in western Tasmania between 7000 and 4500 cal yr BP from the relative abundance of Phyllocladus compared with N. cunninghammii and Atherosperma moschatum (i.e., the Lake Vera paleovegetation index) in a pollen record from the Frenchmans Cap region (Fletcher and Moreno, 2011). Lake levels in southeastern mainland Australia also record a Holocene high-stand at ca. 7200 cal yr BP (Figure 6; Wilkins et al., 2013). High effective moisture during the middle Holocene has been attributed to the increased intensity of moisture-delivering SWW in latitudes north of 50°S (Fletcher and Moreno, 2011, 2012). Aside from a brief period of occupation at Parmerpar Meethaner at ca. 5000 cal yr BP, humans either abandoned the region or were not occupying rockshelters (Cosgrove, 1995). The proxy evidence point to the cool wet conditions of the middle Holocene as the primary cause for reduced fire activity although the apparent absence of people may have resulted in the removal of an important ignition source and contributed to lower biomass burning.
Late Holocene (ca. 4500–Present cal yr BP)
A substantial change in vegetation in the Cradle Mountain region occurred during the late Holocene. Areas of wet, closed montane rainforest became more restricted, while open Eucalyptus woodland communities expanded, after ca. 3600 cal yr BP. The lowest amounts of N. cunninghammii, Athrotaxis, and N. gunnii and highest values of Eucalyptus and Monotoca pollen were recorded between ca. 3600 and 2000 cal yr BP. High amounts of Poaceae and Asteraceae after 3600 cal yr BP provides additional evidence of a shift to more-open canopy structured forests.
A major fire episode occurred at ca. 3620 ± 15 cal yr BP, and the magnitude of the charcoal peak (764.67 particles cm−2 yr−1) was more than 10 times greater than any other in the record, suggesting the occurrence of one or more large or severe fires (i.e., Whitlock et al., 2006; Higuera et al., 2009; Dunnette et al., 2014). After this fire episode, the rainforest taxa in the pollen record dropped to their lowest level of the Holocene and Eucalyptus became dominant (Figure 6). A similar rapid replacement of rainforest by Eucalyptus woodland in the late Holocene is noted at Lake St Clair (Hopf et al., 2000), Mount Field (Macphail, 1979), and Lake Osborne in the Hartz Mountains of south-central Tasmania (Fletcher et al., 2014a). Rainforest elements including N. cunninghammii and Athrotaxis declined substantially at all these sites and were replaced by sclerophyll elements, especially Eucalyptus and Proteaceae. Fire played a substantial role in this transition at Lake Osborne; however, whether or not fire drove this shift at Mount Field or Lake St Clair is currently unknown, as macroscopic charcoal has not been analyzed at these sites.
A significant difference between the records at Lake Osborne (the most detailed and high-resolution of these records) and Wombat Pool, however, is the late Holocene history of Athrotaxis. At Lake Osborne, Athrotaxis collapsed over the last ca. 3000 years. At Cradle Mountain, Athrotaxis declined after the fire episode ca. 3600 years ago, but has rebounded in the last 1000 years. Although large tracts of Athrotaxis forest have been lost from bushfires at the hands of Europeans (Marsden-Smedley, 1998), the pollen record from Wombat Pool indicates that Athrotaxis has become more abundant in the last ca. 1000 years than during the period between ca. 3600 and 1000 cal yr BP. This increase is also observed at Solomons Jewel Lake ~30 km southeast of Wombat Pool on the Central Plateau (Dodson, 2001). Dodson (2001) postulated that the expansion of Athrotaxis in the last 800–900 years was primarily along the stream channels and lakeshores where it grows today, which is the likely habitat of expansion near Wombat Pool as well. At Cradle Mountain, as elsewhere in western Tasmania, Athrotaxis and other montane and subalpine rainforest species are often confined to “topographic refugia” in geographically sheltered, south-facing settings where microclimate conditions are favorable for growth and fires occur infrequently (Wood et al., 2011; Yospin et al., 2015).
Fire activity decreased at Wombat Pool following the extreme fire episode at ca. 3600 cal yr BP. The shift in vegetation composition from montane rainforest to Eucalyptus woodland may have contributed to the decline in charcoal influx. The charcoal record from Lake Osborne shows a similar decrease in macroscopic charcoal (>125 μm) after a vegetation shift from rainforest to Eucalyptus woodland ca. 3000 cal yr BP. The explanation at both sites is likely related to reduced fuel biomass and cooler conditions. Despite being more flammable than rainforest communities (Pyrke and Marsden-Smedley, 2005), Eucalyptus-dominated woodlands have a more open canopy structure, more widely spaced trees, and less overall fuel than the temperate rainforest communities that defined the mid-Holocene vegetation of Wombat Pool (Jackson, 1968, 1999).
A generally cooler and drier climate and enhanced climate variability were features of the late Holocene in western Tasmania (Figure 6). The cessation of speleothem growth at Lynds Cave at ca. 5000 cal yr BP indicates that effective moisture decreased to the point of precluding of stalagmite growth. A study of Australian dust deposition in New Zealand found that conditions in eastern mainland Australia were generally drier after 4800 BP and also more variable as a result of oscillating dry/wet El Ninao/La Ninaa conditions (Marx et al., 2009). A number of studies from the tropical Pacific indicate changes in ENSO strength and variability in the late Holocene. Records from the western tropical Pacific suggests the onset or strengthening of ENSO ca. 5000 years ago (Shulmeister and Lees, 1995; Wanner et al., 2008), whereas evidence from the eastern tropical Pacific region shows strengthening and/or increased variability occurred at 3000–4000 cal yr BP (Moy et al., 2002; Conroy et al., 2008; Koutavas and Joanidea, 2012; Carré81 et al., 2014). A number of paleoecological records from Tasmania exhibit fluctuations in fire, vegetation or both in the late Holocene (i.e., Macphail, 1979; Fletcher et al., 2014a, 2015), and this variability has been attributed to ENSO influences (Donders et al., 2007; Fletcher et al., 2014a, 2015; Rees et al., 2015; McWethy et al., in revision). Given Wombat Pool's location in the region of northern Tasmania that is influenced by ENSO today (Hill et al., 2009), heightened ENSO variability could explain some of the oscillating vegetation patterns as well as the fuel conditions, including the large fire episode. However, the charcoal and pollen records from Wombat Pool are not of sufficient temporal resolution to show definitive phasing with El Niño and La Niña events.
Archeological evidence from two sites (Warragarra and Parmerpar Meethaner rockshelters) near Wombat Pool documents a resumption of human use ca. 4000 years ago that lasts to the European period (Lourandos, 1983; Cosgrove, 1995; Allen and Porch, 1996). People either repopulated a region that they had abandoned or shifted subsistence strategies back toward the use of rockshelter/cave sites (Cosgrove, 1999; Pike-Tay et al., 2008). In either case, they may have become a significant ignition source that was lacking during the previous ca. 7000 years.
The cause of the large fire episode at 3600 cal yr BP is impossible to determine from the paleoecological record, but the event was likely responsible for triggering a change in vegetation at Wombat Pool from a mixed forest with rainforest elements to a Eucalyptus woodland. The mosaic nature of the vegetation at Cradle Mountain today may be, in part, a legacy of this fire episode. This event appears to have provided an opening for Eucalyptus and other wet sclerophyll forest plants. Cool, dry conditions in the late Holocene limited the ability of subalpine and temperate rainforest species, including Athrotaxis, to be maintained as the dominant vegetation type and thus the shift to more sclerophyll species. The occurrence of a large fire perturbation may have created a threshold that pushed the rainforest vegetation to a more sclerophyll-dominated assemblage.
Conclusions
The Wombat Lake record suggests that variations in climate have been the primary driver of changes in vegetation and fire over the last 17,000 cal years in the Cradle Mountain area. The importance of large-scale changes in the climate system is evident from comparing the pollen and charcoal data at Wombat Pool with paleoclimate proxy data from the region and Southern Hemisphere. In contrast, human occupation intensity near the site declined after 17,000 cal yr BP and was nearly absent by 11,000 cal yr BP. Past changes in vegetation and climate at Wombat Pool show little relationship to times of human activity in the local area.
Deglacial landscapes near Wombat Pool were initially covered by grass and herbs, and woody subalpine shrubs colonized the area at ca. 16,000 cal yr BP. Rainforest trees and shrubs were present at 14,000 cal yr BP when conditions were warmer than before but fires were infrequent. Fire activity increased between 12,500 and 11,000 cal yr BP and the highest fire activity occurred between ca. 10,900 and 9400 cal yr BP. Pollen data indicate a mosaic of wet and dry vegetation types, which suggests that fires primarily burned the fire-prone parts of the landscape covered by wet sclerophyll shrub and buttongrass moorland, while rainforest survived in protected humid settings. High fire activity in the early Holocene is attributed to the effects of high winter and annual insolation during this period, which resulted in higher-than-present winter and annual temperatures that in turn lengthened the growing season and increased levels of fuel biomass. The early Holocene is also a time of high fire activity in southern South America, and warm dry conditions in temperate southern latitudes have been attributed to weaker and more southerly position of the Southern Hemisphere Westerlies than at present.
During the middle Holocene, ca. 8500–6500 cal yr BP, the montane rainforest was well developed near Wombat Pool and at other sites across western Tasmania. A decline in fire activity after ca. 8500 cal yr BP is attributed to high effective moisture and enhanced Southern Westerly flow. The hemispheric temperature record, regional lake levels, and local speleothem growth rates suggest that conditions became drier after ca. 6000 and cooler after ca. 5000 cal yr BP and these trends likely explain the decline in rainforest and subalpine taxa after ca. 6000 cal yr BP at Wombat Pool and across western Tasmania.
In the late Holocene, a large fire event occurred at ca. 3600 cal yr BP and was associated with a major shift in the vegetation from rainforest and subalpine taxa to open sclerophyll taxa. This event has also been noted at other sites in the region, suggesting that severe fires were supported by regionally dry conditions. The open sclerophyll woodland persisted until ca. 2000 cal yr BP when rainforest and subalpine taxa began to increase resulting in a mosaic of wet sclerophyll and rainforest communities. In the last 1000 years, Athrotaxis-Diselma has increased in abundance near Wombat Lake, in the absence of local fires, in contrast to its decline throughout much of western Tasmania. Its local recovery may be cause for some optimism: if old-growth forests can be protected from fire, Athrotaxis may have some capacity to regenerate naturally in the Cradle Mountain region.
Author Contributions
LS, CW, and SH designed the research. LS performed data analysis and wrote the paper. CW and SH contributed to the interpretation of the data, revised for intellectual content, and approved the final version of the manuscript.
Conflict of Interest Statement
The authors declare that the research was conducted in the absence of any commercial or financial relationships that could be construed as a potential conflict of interest.
Acknowledgments
This research was supported by National Science Foundation grant OISE-09664472 to C. Whitlock and Australian Research Council grant DP0664898 to S. Haberle. Support in the field came from K. Allen, C. Burke, J. Chin, L. Fixico, R. Gresswell, S. Nichols, K. Saunders, M. Weingard and G. Yospin. We thank C. Clayton, J. Gay, J. Giskaas, E. Merrell, C. Shama, J. Stevenson and R. Whitau for lab assistance and M. Fletcher, F. Hopf, V. Iglesias, B. Maxwell, D. McWethy, and K. Taylor for intellectual contributions. The comments of two reviewers improved the manuscript and we thank them for their contributions.
References
Allen, J. (1996). “Warreen cave,” in Report of the Southern Forests Archaeological Project: Site Descriptions, Stratigraphies and Chronologies, Vol. 1, ed J. Allen (Melbourne, VIC: La Trobe University Press), 135–167.
Allen, J., and Porch, N. (1996). “Warragarra rockshelter,” in Report of the Southern Forests Archaeological Project: Site Descriptions, Stratigraphies and Chronologies, Vol. 1, ed J. Allen (Melbourne, VIC: La Trobe University Press), 195–218.
Anker, S. A., Colhoun, E. A., Barton, C. E., Petersen, M., and Barbetti, M. (2001). Holocene vegetation and paleoclimatic and Paleomagnetic history from Lake Johnsten, Tasmania. Quatern. Res. 56, 264–274. doi: 10.1006/qres.2001.2233
APSA-Members (2007). The Australasian Pollen and Spore Atlas v1.0. Canberra, ACT: Australian National University. Available online at: http://apsa.anu.edu.au.
Barker, P. C. J. (1995). Phyllocladus aspleniifolius: phenology, germination, and seedling survival. New Zeal. J. Bot. 33, 325–337. doi: 10.1080/0028825X.1995.10412960
Barker, P. C. J., and Kirkpatrick, J. B. (1994). Phyllocladus aspleniifolius: variability in the population structure, regeneration microsite and dispersion patterns in Tasmanian rainforest. Aust. J. Bot. 42, 163–190. doi: 10.1071/BT9940163
Barrows, T. T., Stone, J. O., Fifield, L. K., and Cresswell, R. G. (2002). The timing of the last glacial maximum in Australia. Quatern. Sci. Rev. 21, 159–173. doi: 10.1016/S0277-3791(01)00109-3
Bennett, K. D., and Willis, K. J. (2001). “Pollen,” in Tracking Environmental Change Using Lake Sediments: Terrestrial, Algal, and Siliceous Indicators, Vol. 3, eds J. P. Smol, H. J. B. Birks and W. M. Last (Dordrecht, Kluwer Academic Publishers), 5–32.
Berger, A., and Loutre, M. F. (1991). Insolation values for the climate of the last 10 million years. Quatern. Sci. Rev. 10, 297–317. doi: 10.1016/0277-3791(91)90033-Q
Blaauw, M. (2010). Methods and code for “classical” age-modelling of radiocarbon sequences. Quatern. Geochronol. 5, 512–518. doi: 10.1016/j.quageo.2010.01.002
Blaauw, M., and Christen, J. A. (2011). Flexible paleoclimate age-depth models using an autoregressive gamma process. Bayesian Anal. 6, 457–474. doi: 10.1214/ba/1339616472
Bowman, D. M. J. S. (2000). Australian Rainforests: Islands of Green in a Land of Fire. Cambridge: Cambridge University Press.
Brown, M. J., Kirkpatrick, J. B., and Moscal, A. (1983). The conservation of Tasmanian endemic species found in alpine vegetation. Proc. Ecol. Soc. Aust. 12, 168–169.
Burrows, G. E. (2002). Epicormic strand structure in Angophora, Eucalyptus and Lophostemon (Myrtaceae)—implications for fire resistance and recovery. New Phytol. 153, 111–131. doi: 10.1046/j.0028-646X.2001.00299.x
Calvo, E., Pelejero, C., De Deckker, P., and Logan, G. A. (2007). Antarctic deglacial pattern in a 30 kyr record of sea surface temperature offshore South Australia. Geophys. Res. Lett. 34:L13707. doi: 10.1029/2007GL029937
Carré, M., Sachs, J. P., Purca, S., Schauer, A. J., Braconnot, P., Falcón, R. A., et al. (2014). Holocene history of ENSO variance and asymmetry in the eastern tropical Pacific. Science 345, 1045–1048. doi: 10.1126/science.1252220
Clark, J. S. (1988). Particle motion and the theory of charcoal analysis: source area, trans- port, deposition, and sampling. Quatern. Res. 30, 67–80. doi: 10.1016/0033-5894(88)90088-9
Colhoun, E. A., Pola, J. S., Barton, C. E., and Heijnis, H. (1999). Late Pleistocene vegetation and climate history of Lake Selina, western Tasmania. Quatern. Int. 57–58, 5–23. doi: 10.1016/S1040-6182(98)00046-9
Colhoun, E.A., and Shimeld, P.W. (2012). “Late-Quaternary vegetation history of Tasmania from pollen records,” in: Peopled Landscapes. Archaeological and Biogeographic Approaches to Landscapes, Terra Australis 34, eds S. G. Haberle and B. David (Canberra, ACT: ANU E Press), 297–328.
Colhoun, E. A., and van de Geer, G. (1986). Holocene to middle last glaciation vegetation history at Tullabardine Dam, Western Tasmania. Proc. Roy. Soc. Lond. B Biol. 229, 177–207. doi: 10.1098/rspb.1986.0081
Conroy, J. L., Overpeck, J. T., Cole, J. E., Shanahan, T. M., and Steinitz-Kannan, M. (2008). Holocene changes in eastern tropical Pacific climate inferred from a Galapagos lake sediment record. Quatern. Sci. Rev. 27, 1166–1180. doi: 10.1016/j.quascirev.2008.02.015
Cook, E. R., Buckley, B. M., D'Arrigo, R. D., and Peterson, M. J. (2000). Warm-season temperatures since 1600 BC reconstructed from Tasmanian tree rings and their relationship to large-scale sea surface temperature anomalies. Clim. Dynam. 16, 79–91. doi: 10.1007/s003820050006
Cosgrove, R. (1995). Late pleistocene behavioural variation and time trends: the case from Tasmania. Archaeol. Ocean. 30, 83–104. doi: 10.1002/j.1834-4453.1995.tb00333.x
Cosgrove, R. (1999). Forty-two degrees south: the archaeology of late pleistocene Tasmania. J. World Prehist. 13, 357–402. doi: 10.1023/A:1022310029016
Cosgrove, R., Field, J., Garvey, J., Brenner-Coltrain, J., Goede, A., Charles, B., et al. (2010). Overdone overkill e the archaeological perspective on Tasmanian megafaunal extinctions. J. Archaeol. Sci. 37, 2486–2503. doi: 10.1016/j.jas.2010.05.009
D'Costa, D. M., Edney, P., Kershaw, A. P., and De Deckker, P. (1989). Late quaternary paleoecology or Tower Hill, Victoria, Australia. J. Biogeogr. 16, 461–482.
Dodson, J. R. (2001). A vegetation and fire history in a subalpine woodland and rainforest region, Solomons Jewel Lake, Tasmania. Holocene 11, 111–116. doi: 10.1191/095968301670253295
Donders, T. H., Haberle, S. G., Hope, G., Wagner, F., and Visscher, H. (2007). Pollen evidence for the transition of the Eastern Australian climate system from the post-glacial to the present-day ENSO mode. Quatern. Sci. Rev. 26, 1621–1637. doi: 10.1016/j.quascirev.2006.11.018
Dunnette, P. V., Higuera, P. E., McLauchlan, K. K., Derr, K. M., Briles, C. E., and Keefe, M. H. (2014). Biogeochemical impacts of wildfires over four millennia in a Rocky Mountain subalpine watershed. New Phytol. 203, 900–912. doi: 10.1111/nph.12828
EPICA Members. (2004). Eight glacial cycles from an Antarctic ice core. Nature 429, 623–628. doi: 10.1038/nature02599
Fletcher, M.-S., Benson, A., Heijnis, H., Gadd, P. S., Cwynar, L. C., and Rees, A. B. H. (2015). Changes in biomass burning mark the onset of an ENSO-influenced climate regime at 42⋅ S in southwest Tasmania, Australia. Quatern. Sci. Rev. 122, 222–232. doi: 10.1016/j.quascirev.2015.05.002
Fletcher, M.-S., and Moreno, P. I. (2011). Zonally symmetric changes in the strength and position of the Southern Westerlies drove atmospheric CO2 variations over the past 14 k.y. Geology 39, 419–422. doi: 10.1130/G31807.1
Fletcher, M.-S., and Moreno, P. I. (2012). Have the Southern Westerlies changed in a zonally symmetric manner over the last 14,000 years? A hemisphere-wide take on a controversial problem. Quatern. Int. 253, 32–46. doi: 10.1016/j.quaint.2011.04.042
Fletcher, M.-S., and Thomas, I. (2007). Modern pollen-vegetation relationships in western Tasmania, Australia. Rev. Palaeobot. Palynol. 146, 146–168. doi: 10.1016/j.revpalbo.2007.03.002
Fletcher, M.-S., Wolfe, B. B., Whitlock, C., Pompeani, D. P., Heijnis, H., Haberle, S. G., et al. (2014a). The legacy of mid-Holocene fire on a Tasmanian montane landscape. J. Biogeogr. 41, 476–488. doi: 10.1111/jbi.12229
Fletcher, M.-S., Wood, S. W., and Haberle, S. G. (2014b). A fire-driven shift from forest to non-forest: evidence for alternative stable states? Ecology 95, 2504–2513. doi: 10.1890/12-1766.1
Gammage, B. (2008). Plain facts: Tasmania under aboriginal management. Landsc. Res. 33, 241–254. doi: 10.1080/01426390701767278
Gammage, B. (2011). The Biggest Estate on Earth: How Aborigines Made Australia. Sydney, FC: Allen and Unwin.
Gedye, S. J., Jones, R. T., Tinner, W., Ammann, B., and Oldfield, F. (2000). The use of mineral magnetism in the reconstruction of fire history: a case study from Lago di Origlio, Swiss Alps. Palaeogeogr. Palaeoclimatol. 164, 101–110. doi: 10.1016/S0031-0182(00)00178-4
Gillett, N. P., Kell, T. D., and Jones, P. D. (2006). Regional climate impacts of the Southern Annular Mode. Geophys. Res. Lett. 33:L23704. doi: 10.1029/2006GL027721
Grimm, E. C. (1988). “Data analysis and display,” in Vegetation History, eds B. Huntley and T. Webb III (Dordrecht: Kluwer Academic), 43–76.
Grimm, E. C. (1990). TILIA and TILIA. GRAPH: PC spreadsheet and graphics software for pollen data (Version 2.0.b.4). INQUA – commission for the study of the holocene, working-group on data-handling methods. Newsletter 4, 5–7.
Grootes, P. M., Steig, E. J., Stuiver, M., Waddington, E. D., and Morse, D. L. (2001). The Taylor Dome Antarctic 18O record and globally synchronous changes in Climate. Quatern. Res. 56, 289–298. doi: 10.1006/qres.2001.2276
Gupta, A. S., and England, M. H. (2006). Coupled ocean-atmosphere-ice response to variations in the Southern Annular Mode. J. Clim. 19, 4457–4486. doi: 10.1175/JCLI3843.1
Haberle, S. G. (2005). A 23,000-yr pollen record from Lake Euramoo, Wet Tropics of NE Queensland, Australia. Quatern. Res. 64, 343–356. doi: 10.1016/j.yqres.2005.08.013
Harris, S., and Kitchener, A. (2005). From Forest to Fjaeldmark: Descriptions of Tasmania's Vegetation. (Hobart, HZ: Department of Primary Industries, Water and Environment).
Hendon, H. H., Thompson, D. W. J., and Wheeler, M. C. (2007). Australian rainfall and surface temperature variations associated with the southern hemisphere annular mode. J. Clim. 20, 2452–2467. doi: 10.1175/JCLI4134.1
Higuera, P. E., Brubaker, L. B., Anderson, P. M., Hu, F. S., and Brown, T. A. (2009). Vegetation mediated the impacts of postglacial climate change on fire regimes in the southcentral Brooks Range, Alaska. Ecol. Monogr. 79, 201–219. doi: 10.1890/07-2019.1
Higuera, P. E., Gavin, D. G., Bartlein, P. J., and Hallett, J. (2010). Peak detection in sediment-charcoal records: impacts of alternative data analysis methods on fire-history interpretations. Int. J. Wildland Fire 19, 996–1014. doi: 10.1071/WF09134
Hill, K. J., Santoso, A., and England, M. H. (2009). Interannual Tasmanian rainfall variability associated with large-scale climate modes. J. Clim. 22, 4383–4397. doi: 10.1175/2009JCLI2769.1
Hogg, A. G., Hua, Q., Blackwell, P. G., Niu, M., Buck, C. E., Guilderson, T. P., et al. (2013). SHCal13 southern hemisphere calibration, 0 - 50,000 cal BP. Radiocarbon 55, 1889–1903. doi: 10.2458/azu_js_rc.55.16783
Holz, A. S., Wood, S. W., Veblen, T. T., and Bowman, D. M. J. S. (2015). Effects of high-severity fire drove the population collapse of the subalpine Tasmanian endemic conifer Athrotaxis cupressoides. Glob. Change Biol. 21, 445–458. doi: 10.1111/gcb.12674
Hopf, F. V. L., Colhoun, E. A., and Barton, C. E. (2000). Late-glacial and Holocene record of vegetation and climate from Cynthia Bay, Lake St Clair, Tasmania. J. Quatern. Sci. 15, 725–732. doi: 10.1002/1099-1417(200010)15:7<725::AID-JQS563>3.0.CO;2-8
Iglesias, V., and Whitlock, C. (2014). Fire responses to postglacial climate change and human impact in northern Patagonia (41–43⋅S). Proc. Natl. Acad. Sci. U.S.A. 101, E5545–E5554. doi: 10.1073/pnas.1410443111
Jackson, W. D. (1968). Fire, air, water and earth–An elemental ecology of Tasmania. Proc. Ecol. Soc. Aust. 3, 9–16.
Jackson, W. D. (1999). The Tasmanian legacy of man and fire. Pap. Proc. Roy. Soc. Tasman. 133, 1–14.
Johnson, K., and Marsden-Smedley, J. B. (2002). Fire history of the northern part of the Tasmanian wilderness world heritage area ad its associated regions. Pap. Proc. Roy. Soc. Tasman. 136, 145–152.
Kelly, R. F., Higuera, P. E., Barrett, C. M., and Hu, F. S. (2011). A signal-to-noise index to quantify the potential for peak detection in sediment-charcoal records. Quatern. Res. 75, 11–17. doi: 10.1016/j.yqres.2010.07.011
Kirkpatrick, J. B. (1977). “Native vegetation of the West Coast region of Tasmania,” in Landscape and Man: The Interaction between Man and the Environment in Western Tasmania, eds M. R. Banks and J. B. Kirkpatrick (Hobart, HZ: The Royal Society of Tasmania), 55–80.
Kirkpatrick, J. B. (1984a). Altitudinal and successional variation in the vegetation of the northern part of the West Coast Range, Tasmania. Aust. J. Ecol. 9, 81–91.
Kirkpatrick, J. B. (1984b). Tasmanian High Vegetation II. – Rocky Hill and Pyramid Mountain. Pap. Proc. Roy. Soc. Tasman. 118, 5–20.
Kirkpatrick, J. B., and Balmer, J. (1991). “The vegetation and higher plant flora of the Cradle Mountain-Pencil Pine area, northern Tasmania,” in Aspects of Tasmanian Botany: A Tribute to Winifred Curtis, eds M. R. Banks, S. J. Smith, A. E. Orchard, and G. Kantvilas (Hobart, HZ: The Royal Society of Tasmania), 119–148.
Kirkpatrick, J. B., and Bridle, K. L. (2013). Natural and cultural histories of fire differ between Tasmanian and mainland Australian alpine vegetation. Aust. J. Bot. 61, 465–474. doi: 10.1071/BT13128
Kirkpatrick, J. B., and Fowler, M. (1998). Locating likely glacial forest refugia in Tasmania using palynological and ecological information to test alternative climatic models. Biol. Conserv. 85, 171–182. doi: 10.1016/S0006-3207(97)00144-4
Kirkpatrick, J., and Dickinson, K. (1984). The impact of fire on Tasmanian alpine vegetation and soils. Aust. J. Bot. 32, 613–629. doi: 10.1071/BT9840613
Koutavas, A., and Joanidea, S. (2012). El Ninao–southern oscillation extrema in the holocene and last glacial maximum. Paleoceanography 27:PA4208. doi: 10.1029/2012PA002378
Mackintosh, A. N., Barrows, T. T., Colhoun, E. A., and Fifield, L. K. (2006). Exposure dating and glacial reconstruction at Mt. Field, Tasmania, Australia, identifies MIS 3 and MIS 2 glacial advances and climatic variability. J. Quatern. Sci. 21, 363–376. doi: 10.1002/jqs.989
Macphail, M. K. (1979). Vegetation and climates in southern Tasmania since the last glaciation. Quatern. Res. 11, 306–341. doi: 10.1016/0033-5894(79)90078-4
Markgraf, V., Bradbury, J. P., and Busby, J. R. (1986). Paleoclimates in southwestern Tasmania during the last 13,000 years. Palaios 1, 368–380. doi: 10.2307/3514474
Marsden-Smedley, J. B. (1998). Changes in Southwestern Tasmanian Fire Regimes Since the Early 1800s. Pap. Proc. Roy. Soc. Tasman. 132, 15–29.
Marx, S. K., McGowan, H. A., and Kamber, B. S. (2009). Long-range dust transport from eastern Australia: a proxy for Holocene aridity and ENSO-type climate variability. Earth Planet. Sci. Lett. 282, 167–177. doi: 10.1016/j.epsl.2009.03.013
McWethy, D. B., Higuera, P. E., Whitlock, C., Veblen, T. T., Bowman, D. M. J. S., Cary, C. J., et al. (2013). A conceptual framework for predicting temperate ecosystem sensitivity to human impacts on fire regimes. Glob. Ecol. Biogeogr. 22, 900–912. doi: 10.1111/geb.12038
Moy, C. M., Seltzer, G. O., Rodbell, D. T., and Anderson, D. M. (2002). Variability of El Ninao/Southern Oscillation activity at millennial timescales during the Holocene epoch. Nature 420, 162–165. doi: 10.1038/nature01194
Nicholls, N., and Lucas, C. (2007). Interannual variation of area burnt in Tasmanian bushfires: relationships with climate and predictability. Int. J. Wildland Fire 16, 540–546. doi: 10.1071/WF06125
O'Donnell, R. J. (2010). Modeling of Quaternary Glacier Extent and Climate in Tasmania, Australia. Master's Thesis, Victoria University, Wellington.
Pike-Tay, A., Cosgrove, R., and Garvey, J. (2008). Systematic seasonal land use by late Pleistocene Tasmanian Aborigines. J. Archaeol. Sci. 35, 2532–2544. doi: 10.1016/j.jas.2008.04.007
Pyrke, A. F., and Marsden-Smedley, J. B. (2005). Fire-attributes categories, fire sensitivity, and flammability of Tasmanian vegetation communities. Tasforests 16, 35–46.
R Core Team (2015). R: A Language and Environment for Statistical Computing. Vienna: R Foundation for Statistical Computing. Available online at: https://www.R-project.org/
Rees, A. B. H., and Cwynar, L. C. (2010). Evidence for early postglacial warming in Mount Field National Park, Tasmania. Quatern. Sci. Rev. 29, 443–454. doi: 10.1016/j.quascirev.2009.10.003
Rees, A. B. H., Cwynar, L. C., and Fletcher, M.-S. (2015). Southern Westerly Winds submit to the ENSO regime: a multiproxy paleohydrology record from Lake Dobson, Tasmania. Quatern. Sci. Rev. 126. 254–263. doi: 10.1016/j.quascirev.2015.08.022
Risbey, J. S., Pook, M. J., McIntosh, P. C., Wheeler, M. C., and Hendon, H. H. (2009). On the Remote Drivers of Rainfall Variability in Australia. Mon. Weather Rev. 137, 3233–3253. doi: 10.1175/2009MWR2861.1
Rodionov, S. N. (2004). A sequential algorithm for testing climate regime shifts. Geophys. Res. Lett. 31:L09204. doi: 10.1029/2004GL019448
Shimeld, P., Hopf, F., and Pearson, S. (2000). Pollen image management: the Newcastle digital collection initiative. Quaternary Australasia 18, 13–15.
Shulmeister, J., and Lees, B. G. (1995). Pollen evidence from tropical Australia for the onset of an ENSO-dominated climate at c. 4000 BP. Holocene 5, 10–18.
Sikes, E. L., Howard, W. R., Samson, C. R., Mahan, T. S., Robertson, L. G., and Volkman, J. K. (2009). Southern Ocean seasonal temperature and Subtropical Front movement on the South Tasman Rise in the late Quaternary. Paleoceanography 24:2201. doi: 10.1029/2008PA001659
Stern, N., and Marshall, B. (1993). Excavations at Mackintosh 90/1 in western Tasmania: a discussion of stratigraphy chronology and site formation. Archaeol. Ocean. 28, 8–17. doi: 10.1002/j.1834-4453.1993.tb00310.x
Wanner, H., Beer, J., Bütikofer, J., Crowley, T. J., Cubasch, U., Flückiger, J., et al. (2008). Mid- to Late Holocene climate change: an overview. Quatern. Sci. Rev. 27, 1791–1828. doi: 10.1016/j.quascirev.2008.06.013
Whitlock, C., Bianchi, M. M., Bartlein, P. J., Markgraf, V., Marlon, J., Walsh, M., et al. (2006). Postglacial vegetation, climate, and fire history along the east side of the Andes (lat 41–42.5⋅S), Argentina. Quatern. Res. 66, 187–201. doi: 10.1016/j.yqres.2006.04.004
Whitlock, C., Higuera, P. E., McWethy, D. B., and Briles, C. E. (2010). Paleoecological perspective on fire ecology: revisiting the fire regime concept. Open Ecol. J. 3, 6–23. doi: 10.2174/1874213001003020006
Whitlock, C., and Larsen, C. P. S. (2001). “Charcoal as a fire proxy,” in Tracking Environmental Change Using Lake Sediments: Vol. 3 Terrestrial, Algal, and Siliceous Indicators, eds J. P. Smol, H. J. P. Birks and W. M. Last (Dordrecht: Kluwer Academic Publishers), 75–97.
Whitlock, C., Moreno, P. I., and Bartlein, P. (2007). Climatic controls of Holocene fire patterns in southern South America. Quatern. Res. 68, 28–36. doi: 10.1016/j.yqres.2007.01.012
Wilkins, D., Gouramanis, C., De Deckker, P., Fifield, L. K., and Olley, J. (2013). Holocene lake-level fluctuations in Lakes Keilambete and Gnotuk, southwestern Victoria, Australia. Holocene 23, 784–795. doi: 10.1177/0959683612471983
Wood, S. W., and Bowman, D. M. J. S. (2012). Alternative stable states and the role of fire–vegetation–soil feedbacks in the temperate wilderness of southwest Tasmania. Landsc. Ecol. 27, 13–28. doi: 10.1007/s10980-011-9677-0
Wood, S. W., Murphy, B. P., and Bowman, D. M. J. S. (2011). Firescape ecology: how topography determines the contrasting distribution of fire and rain forest in the south-west of the Tasmanian Wilderness World Heritage Area. J. Biogeogr. 38, 1807–1820. doi: 10.1111/j.1365-2699.2011.02524.x
Xia, Q. K., Zhao, J.-X., and Collerson, K. D. (2001). Early-mid Holocene climatic variations in Tasmania, Australia: multi-proxy records in a stalagmite from Lynds Cave. Earth Planet. Sci. Lett. 194, 177–187. doi: 10.1016/S0012-821X(01)00541-6
Yospin, G. I., Wood, S. W., Holz, A., Bowman, D. M. J. S., Keane, R. E., and Whitlock, C. (2015). Modeling vegetation mosaics in sub-alpine Tasmania under various fire regimes. Model Earth Syst. Environ. 1:16. doi: 10.1007/s40808-015-0019-0
Keywords: fire history, vegetation history, pyrogeography, Tasmania, Athrotaxis, Eucalyptus, fire ecology, vegetation state change
Citation: Stahle LN, Whitlock C and Haberle SG (2016) A 17,000-Year-Long Record of Vegetation and Fire from Cradle Mountain National Park, Tasmania. Front. Ecol. Evol. 4:82. doi: 10.3389/fevo.2016.00082
Received: 19 April 2016; Accepted: 22 June 2016;
Published: 07 July 2016.
Edited by:
Marc Macias-Fauria, University of Oxford, UKReviewed by:
Anne Elisabeth Bjune, Uni Research Ltd., Uni Climate, NorwayMinna Maria Väliranta, University of Helsinki, Finland
Copyright © 2016 Stahle, Whitlock and Haberle. This is an open-access article distributed under the terms of the Creative Commons Attribution License (CC BY). The use, distribution or reproduction in other forums is permitted, provided the original author(s) or licensor are credited and that the original publication in this journal is cited, in accordance with accepted academic practice. No use, distribution or reproduction is permitted which does not comply with these terms.
*Correspondence: Laura N. Stahle, laura.stahle@msu.montana.edu