- 1Department of Ecology, Instituto de Botânica, São Paulo, Brazil
- 2Phycology Laboratory, Botany, Museu Nacional, Universidade Federal do Rio de Janeiro, Rio de Janeiro, Brazil
Euglenophyceae (phototrophic euglenids) are an important lineage within the Euglenida, Euglenozoa. Most of the approximately 3000 described species are free-living, phototrophic, unicellular flagellates with one to several plastids of secondary origin, three bounding membranes and chlorophylls a and b; but, the lineage also includes colorless species that lost their photosynthesizing capability. They show a typical cell membrane consisting of parallel proteinaceous strips and microtubules located underneath the plasma membrane, and discoid mitochondrial cristae. Euglenozoa are a monophyletic group that includes, besides Euglenida, the Kinetoplastidea, Diplonemea, and Symbiontida. Since the class Euglenophyceae was proposed, its classification system has undergone several revisions, mainly after the adoption of molecular techniques. This article summarizes recent advances in the phylogeny and classification of the phototrophic euglenids, aiming at understanding the situation of the group within the phylum Euglenozoa, as well as its evolutionary relationships and the changes in its taxonomic classification. The current status of the group, as well as the limitations derived from the lack of inclusion of tropical strains in phylogenetic studies is briefly discussed.
Introduction
The class Euglenophyceae includes phototrophic members of the Euglenida, supergroup Excavata, being a frequent component of the plankton in marine, brackish, and freshwater environments. The class encompasses free-living phototrophic unicellular flagellates, with one to several plastids of secondary origin, with three bounding membranes and chlorophylls a and b; but, it also includes colorless species that lost their capability to photosynthesize as well as the photosensory apparatus associated with cilia (secondary osmotrophic species). The Euglenida (euglenids), together with Kinetoplastea (trypanosomes), Diplonemea (genera Diplonema and Rhynchopus), and Symbiontida, form the phylum Euglenozoa (Cavalier-Smith, 1981, 1993; Yubuki et al., 2009; Breglia et al., 2010). The phylum Euglenozoa is well-supported in molecular analyses. The most prominent common features of this clade are the polycistronic transcription, massive trans-splicing, and more rarely the absence of introns (Flegontov and Lukeš, 2012). The members of Kinetoplastea are free-living and obligatory parasitic protists that have cells with extensive mitochondrial DNA, termed kinetoplast or DNA kinetoplast; whereas Diplonemea or diplonemids may be free-living phagotrophs or facultative parasites, with sack-shaped cells and short, thin flagella (Roy et al., 2007; Adl et al., 2012). The Symbiontida includes organisms living in low-oxygen sediments; their cells possess rod-shaped epibiotic bacteria (Breglia et al., 2007; Adl et al., 2012). All these groups share morphological features including paraxial rods, a regular array of longitudinal subpellicular microtubules, and closed mitosis (Simpson, 1997).
The advent of molecular studies and the increasing of the number of genes and DNA regions used in the phylogenetic studies led to a restructuring of the taxonomy of Euglenophyceae, improved understanding of the phylogenetic positions of the genera and several species, and helped to deduce the phylogenetic relationships within the group.
The present article summarizes the main information on the phylogenetic position and taxonomic classification of the phototrophic euglenids. The current status and limitations derived from the lack of inclusion of tropical strains in phylogenetic studies of this group are also discussed. A glossary of terms used in this review is included at the end of the article.
Phylogenetic Position of Euglenophyceae within Euglenozoa
Euglenida, together with the Kinetoplastea, Diplonemea, and Symbiontida, form the phylum Euglenozoa. Cells with two cilia, inserted apically or subapically in a pocket, characterize the phylum Euglenozoa. The cells have a basic ciliary apparatus pattern consisting of two functional kinetosomes and three asymmetrically arranged microtubular roots, with paddle-shaped, discoid or flat mitochondrial cristae, tubular extrusomes, and closed mitosis with an intranuclear spindle (Simpson, 1997; Roy et al., 2007; Adl et al., 2012).
Euglenozoa are monophyletic (Breglia et al., 2010; Burki, 2014) and their clades are well-supported by morphological and molecular data (Simpson, 1997; Simpson and Roger, 2004). Table 1 summarizes the main differences among Euglenida, Kinetoplastea, Diplonemea, and Symbiontida.
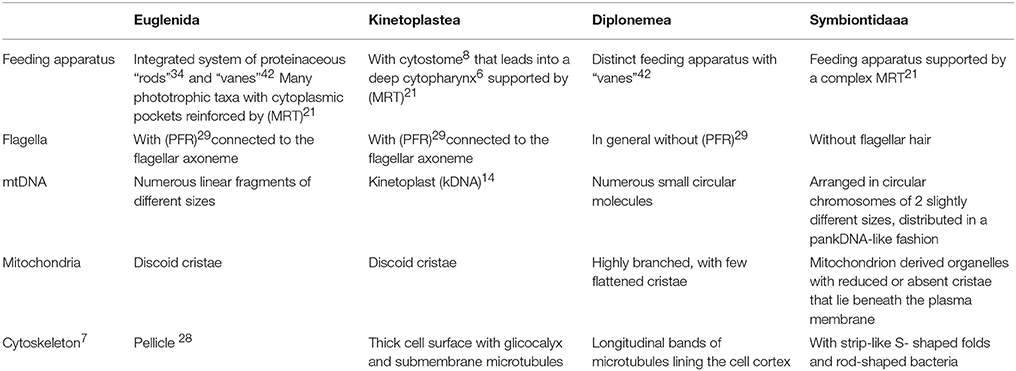
Table 1. Main differences among Euglenida, Kinetoplastea, Diplonemea, and Symbiontida (numbers following some terms correspond to their definitions in the glossary at the end of the text).
The phylum Euglenozoa is a well-defined group, however, its relationship to other protists remains uncertain. The cell structure, especially the presence of discoid mitochondrial cristae, and multigene phylogenetic analyses have suggested that the Euglenozoa are close to the class Heterolobosea, a group represented by heterotrophic amoebae, amoeboflagellates and flagellates (Simpson et al., 2006; Grant and Katz, 2014). Information on ultrastructure and phylogeny suggests that both lineages are members of the supergroup Excavata (Hampl et al., 2009; Yabuki et al., 2011; Figure 1).
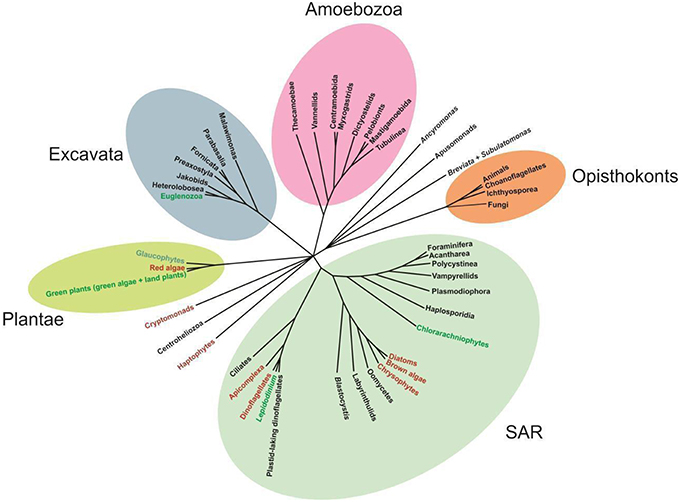
Figure 1. Unrooted phylogenetic tree showing the relationships among representatives of major eukaryote lineages, based on a variety of molecular and morphological data. Green and red text indicates green and red algae derived chloroplast lineages, respectively (Adapted from Katz, 2012).
The Euglenida comprise a group of predominantly free-living organisms characterized by a typical cell cover made of parallel proteinaceous strips and microtubules located underneath the plasma membrane, the pellicle; and encompasses phototrophic, heterotrophic (osmotrophic and phagotrophic), and mixotrophic species (Leander et al., 2007; Yamaguchi et al., 2012).
The Kinetoplastea includes parasitic species and free-living bodonids, which have mitochondrial inclusions of distinctively arranged DNA molecules, termed a kinetoplast DNA or kDNA, which represents an apomorphic character of kinetoplastids (Simpson et al., 2004; Lukeš et al., 2009).
The Diplonemea (diplonemids) encompasses a small group of mostly free-living phagotrophs and some facultative parasites, which exhibit a single highly branched mitochondrion, with a few flat, lamellar cristae, and mitochondrial DNA arranged in circular chromosomes of two slightly different sizes (Marande et al., 2005; Roy et al., 2007).
The Symbiontida is a more recently described clade, which shares typical characters with the Euglenozoa and also shows features not previously described for this group, such as modified (hydrogenosome-like) mitochondria, an “extrusomal pocket,” the highly organized extracellular matrix underneath epibiotic bacteria, and the complex flagellar transition zone (Yubuki et al., 2009).
The phylogenetic relationships among euglenids, diplonemids, kinetoplastids, and symbiontids remain controversial. Studies on morphological features (e.g., shape of the feeding and flagellar apparatuses, mitosis pattern, and cytoskeletal composition) suggest that euglenids and kinetoplastids are more closely related (Triemer and Farmer, 1991). On the other hand, studies combining morphological and molecular phylogenetic data suggest that kinetoplastids and diplonemids are sister groups, with euglenids branching basally to the first two groups (Simpson and Roger, 2004; Marande and Burger, 2007; Kiethega et al., 2011). The discovery of the Symbiontida raised the question of whether diplonemids and symbiontids might be more closely related to euglenids than to kinetoplastids (Yubuki et al., 2013).
A phylogenetic analysis based on SSU rDNA sequences showed the Euglenophyceae as a robust monophyletic clade, with Eutreptia and Eutreptiella as the basal lineages and Rapaza viridis (mixotrophic species) branching as sister lineage (Yamaguchi et al., 2012; Figure 2). Euglenophyceae together with Rapaza form a well-supported clade sister to the clade encompassing heterotrophic groups—Anisonema, Peranema, Dinema, Distigma, Rhabdomonas (with good support), and Peranema trichophorum is basal to this whole clade. Other heterotrophic euglenids (Entosiphon, Pleotia, Petalomonas, Notosolenus) including Symbiontida are grouped together without good support.
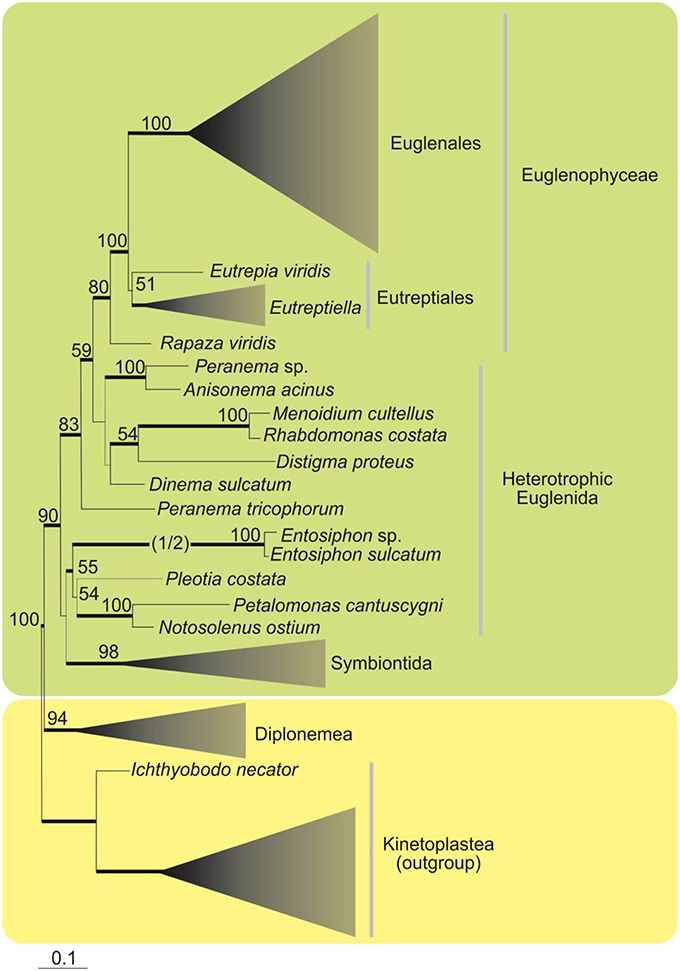
Figure 2. Maximum-likelihood tree inferred from SSU rDNA sequences showing the phylogenetic position of the Euglenophyce within Euglenozoa. Only support values above 50% for the ML bootstrap analysis are shown. Thick branches indicate Bayesian posterior probabilities (PP) over 0.95. The branch leading to the fast-evolving Entosiphon clade has been shortened by one half (indicated by “1/2”; Adapted from Yamaguchi et al., 2012).
Changes in the Taxonomic Classification of Euglenophyceae
Early Studies
The euglenids are part of an extremely diverse lineage of flagellate protists (Marin et al., 2003). Ehrenberg (1830) first attempted to classify euglenids, when he described the genus Euglena. Ehrenberg (1830), keeping within his self-created classification system, placed the new genus among the Polygastrica of family Astasiae, i.e., multi-stomached creatures with no alimentary canal, variable body shape, but no pseudopods or lorica. Subsequently, several investigators added descriptive characteristics for the genus Euglena and established different classification systems for euglenids, based on the presence, number of flagella, and nutrition mode (Dujardin, 1841; Cohn, 1853; Klebs, 1883).
Hollande (1942) accomplished a major revision of these flagellates, grouping them by their shared structural features (e.g., body shape, nutritional mode, flagellar structure, and degree of metaboly) into Peranemoidées, Petalomonadinées, and Euglenidinées. Hollande's (1942) classification was accepted as best reflecting the natural relationships among these organisms, and consequently was widely adopted (Pringsheim, 1956; Leedale, 1967, 1978; Johnson, 1968).
The classification system of Leedale (1967, 1978) expanded Hollande's (1942) scheme by incorporating new physiological data and aspects of their ultrastructure. This classification established six orders (Eutreptiales, Euglenales, Rhabdomonadales, Sphenomonadales, Heteronematales, and Euglenamorphales); considered the reduction in the flagella number as the most important evolutionary event for understanding the phylogeny of the group; and separated the photosynthetic from the colorless forms, with a phagotrophic ancestor involving phototrophic forms through secondary endosymbiosis (Leedale, 1967, 1978). Some taxa included in Leedale's scheme underwent taxonomic changes based on analyses using molecular techniques. These taxa encompass descendants that have independently lost their chloroplasts.
The Leedale scheme of 1978 was used to classify the euglenids until 1986, when Euglena gracilis was sequenced using the SSU rRNA gene (Sogin et al., 1986).
Molecular Studies
Single-Gene Phylogenies
Although E. gracilis was the first phototrophic euglenid to be studied molecularly, based on the SSU rDNA (ribosomal nuclear small subunit) sequences, the phylogenetic results for the group were merely preliminary, since the E. gracilis sequences were compared with only a small number of sequences from other eukaryotes (Sogin et al., 1986). A more-robust molecular study, also based on SSU rDNA sequence data, for 10 species (6 phototrophs and 4 heterotrophs), suggested that euglenids are a distinct monophyletic clade, the phototrophic forms arose after the phagotrophic members, the biflagellate form diverged prior to the uniflagellate, and the genus Euglena is probably paraphyletic (Linton et al., 1999).
Several subsequent studies were also based on the SSU rDNA gene; however, these were combined with morphological characters used for genus delimitation (Linton et al., 2000; Moreira et al., 2001; Müllner et al., 2001; Marin et al., 2003; Nudelman et al., 2003; Kosmala et al., 2005). Marin et al. (2003) provided the first revision of the group in which a diagnosis of Euglenophyceae Schoenichen, 1925 was emended by adding the nuclear-encoded SSU rRNA molecule with A-U as the first base pair of Helix 39 (Marin et al., 2003). Euglenophyta was recovered as a monophyletic group and was divided into the orders Eutreptiales and Euglenales. Within this group, Euglenophyceae also constituted a monophyletic group, with strong support; whereas Petalomonas cantuscygni and P. trichophorum (both phagotrophic) and the Distigma clade (formed by saprophytic species) showed paraphyletic divergence (Marin et al., 2003). An interesting tool used by these authors to separate taxa was the non-homoplasious synapomorphies (NHS), i.e., the characters without known parallels in other taxa.
In addition to these studies are those of Milanowski et al. (2001), who examined the phylogeny of the genus Euglena based on the chloroplast small subunit rDNA (16S rDNA), and others on rbcL genes (Thompson et al., 1995), chloroplast ribosomal large subunit cpLSU rDNA (Kim and Shin, 2008), PAR gene sequences (par1 and par2) (Talke and Preisfeld, 2002), and the cytosolic form of the heat-shock protein 90 gene (hsp90; Breglia et al., 2007).
Multi-Gene Phylogenies
Taxonomic and phylogenetic molecular studies on phototrophic euglenids based on multi-gene analysis combining different molecular markers with morphological characters have increased in number. Examples include studies using nuclear SSU and LSU rDNA (Triemer et al., 2006; Ciugulea et al., 2008); chloroplast SSU rDNA (16S); cytoplasmic SSU rDNA (Milanowski et al., 2006); nuclear SSU, LSU rDNA, and chloroplast SSU rDNA (16S) sequences (Linton et al., 2010); cytoplasmic and chloroplast small subunit rRNA, and the internal transcribed spacer (ITS2) of cytoplasmic rDNA (Zakryś et al., 2002); small-subunit rRNA, large-subunit rRNA α-tubulin, β-tubulin, actin, and cytosolic heat shock protein 90 sequences (Kim et al., 2006); and α-tubulin, β-tubulin, elongation factor 1α (EF-1α), elongation factor 2 (EF-2), cytosolic heat shock protein 70 (HSP70), and cytosolic heat shock protein 90 (HSP90; Simpson et al., 2006). Triemer and Farmer (2007) published a thorough revision of the systematic and molecular phylogeny of the euglenids described up to the year 2006.
Analysis of nuclear SSU rDNA and plastid SSU and LSU rDNA led to splitting of the photosynthetic euglenids into two major monophyletic groups, represented by the clades Euglenaceae and the newfamilyPhacaceae (Kim et al., 2010). Another study, based on nuclear SSU and LSU rDNA, chloroplast SSU rDNA, and two nuclear protein-coding sequences (hsp90 and psbO), indicated the monophyly of the families Euglenaceae and Phacaceae (Karnkowska et al., 2015). More recently, the monophyly of both families was confirmed in a study based on nuclear SSU and LSU rDNA and chloroplast SSU and LSU rDNA (Kim et al., 2015; Figure 3).
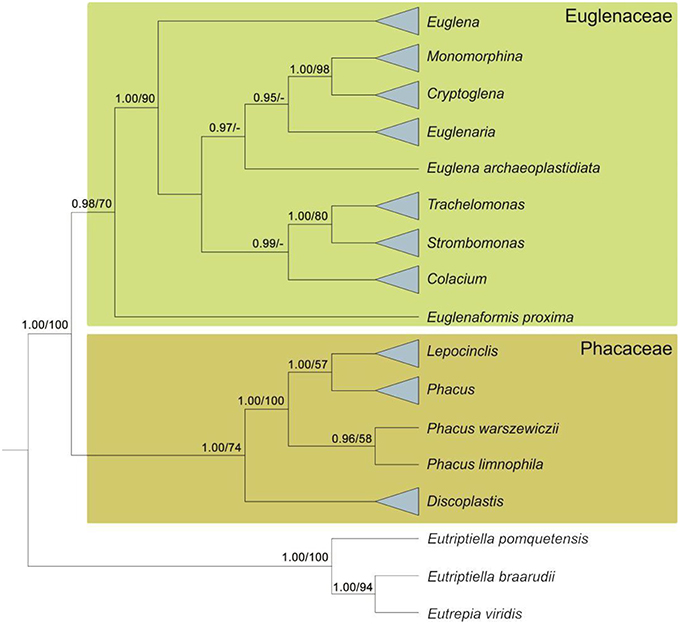
Figure 3. Diagram of a Bayesian tree for the phototrophic euglenids based on mutigenes (nSSU, nLSU, cpSSU, hsp90, and psbO). Numbers at internal nodes represent posterior probability (pp) values followed by bootstrap support (bs) values. Support values below 0.75 pp and below 50 for bs are marked with dashes (adapted from Karnkowska et al., 2015).
A recent analysis based on reconstruction of the inferred ancestral state revealed many well-supported clades defined by apomorphic morphological characters (e.g., the presence of a lorica and mucilaginous stalks), as well as by homoplastic traits (e.g., rigid cells and the presence of large paramylon grains; Karnkowska et al., 2015). This study also revealed that the pyrenoids were lost twice during the evolution of phototrophic euglenids, and that mucocysts, which occur only in the genus Euglena, evolved independently at least twice (Karnkowska et al., 2015).
Multi-gene phylogenetic approaches revealed that all phototrophic euglenids form a monophyletic lineage in which the chloroplast was acquired through secondary endosymbiosis, i.e., a phagotrophic euglenid host engulfed and enslaved a eukaryotic green alga. Studies based on the chloroplast genome demonstrated that the genus Pyramimonas, a green marine prasinophyte, is the closest relative of the euglenid chloroplasts (Turmel et al., 2009; Hrdá et al., 2012).
Chloroplast Genome
Early vs. late origin of plastids hypothesis
Although our understanding of the origin of the diversity and of the evolution of the cpGenomes in euglenids remains unclear, most investigators accept the hypothesis of a late plastid to explain the origin of chloroplasts in the group (Hallick et al., 1993; Vesteg et al., 2010; Bennett et al., 2012; Hrdá et al., 2012). Under this hypothesis, chloroplasts in the Euglenophyceae originated from a secondary endosymbiosis event with a green alga similar to Pyramimonas, which occurred after the separation of the heterotrophic lineage of Peranema, but before the segregation of the basal lineages Eutreptiella and Eutreptia (Leander et al., 2001).
Donor of chloroplast
Comparative analyses between the genomes of the chloroplasts (cpGenomes) generated new understandings of evolutionary questions among the euglenids and with their ancestor, similar to Pyramimonas, although the origin of the diversity and evolution of the cpGenomes in the group still require further clarification. Complete cpGenome sequences of euglenids have been reported for 13 phototrophic species (Sheveleva and Hallick, 2004; Hrdá et al., 2012; Pombert et al., 2012; Wiegert et al., 2012, 2013; Bennett et al., 2014; Bennett and Triemer, 2015). Results of these studies revealed an intense loss of genes occurring in the chloroplast genomes from the common ancestor of Pyramimonas parkeae and E. gracilis, to the present representatives of E. gracilis, as a consequence of secondary endosymbiosis (Turmel et al., 2009); and that these gene losses may have been accompanied by the loss of photosynthesis capacity, for example in Euglena longa (Gockel and Hachtel, 2000; Busse and Preisfeld, 2003). The organization of the cpGenome proved to be diversified in the lineages of E. gracilis and Eutreptiella gymnastica, and also in the lineage that originated Euglena (Hrdá et al., 2012; Wiegert et al., 2012).
Origin and evolution of introns
An analysis of the cpGenome of Monomorphina aenigmatica supported the late-intron hypothesis and the occurrence of two distinct and independent acquisitions of introns in the cpGenomes of the euglenids (Pombert et al., 2012).
The identification of six insertion sites, with an intermediate state in the appearance of twintrons, led to the supposition that the first twintron (group III twintron) that appeared in the cpGenomes of the euglenids is that found in the gene that codes for psbC (Pombert et al., 2012). Chloroplast psbC gene was also recorded in other phototrophic euglenids, e.g., E. gracilis, E. gymnastica, and Eutreptia viridis (Hrdá et al., 2012; Wiegert et al., 2012).
The presently available evidence suggests that the introns found in cpDNAs of euglenids were acquired after the secondary endosymbiosis. The presence of a single intron in P. parkeae, unrelated to those found in the DNAs of the chloroplasts of euglenids, as well as the small number of introns in the cpGenome of E. gymnastica (basal lineage of the euglenids) support the idea that the supposed ancestor of the group was poor in introns; and also that the cpGenome of the ancestor already possessed the psbC intron (Doetsch et al., 1998) and that other introns were acquired later in the course of evolution. However, remains unclear how the increase in the number of introns occurred, and what alterations in their physical form occurred in the euglenids (Pombert et al., 2012).
Gene content and synteny among species
Instances of synteny in the gene contents were identified between the cpGenomes of E. gymnastica vs. E. gracilis, and E. viridis vs. E. gracilis (Hrdá et al., 2012; Wiegert et al., 2012).
A more recent analysis of the cpGenomes of Cryptoglena skujai, E. gracilis var. bacillaris, E. viridis, Euglenaria anabaena, Monomorphina parapyrum, and Trachelomonas volvocina showed that these genomes, generally, do not have the very conserved gene order within the Euglenaceae, and also that strains of E. gracilis and E. viridis have marked synteny, indicating that synteny is a distinctive feature of the taxon (Bennett and Triemer, 2015). A surprising aspect was that different genera (C. skujai, E. anabaena, M. aenigmatica, M. parapyrum, and Strombomnas acuminata) share the same synteny, a feature that was previously known only among species of the same genus (Bennett and Triemer, 2015). Other new and important aspects noted were: (i) unusually high variability among the genomes in the family Euglenaceae, unlike their gene contents, which are extremely conservative; (ii) absence of a pattern of intron numbers in the cpGenome in the Euglenaceae; and (iii) the presence or absence of twintrons is apparently not specific to species, but rather, to genera (Bennett and Triemer, 2015).
Mitochondrial Genomes
Few studies on the mitochondrial genome (mtGenome) in phototrophic forms have been conducted. Most studies have concentrated on the mitochondrial genome of E. gracilis (Ray and Hanawalt, 1965; Crouse et al., 1974; Nass et al., 1974; Tessier et al., 1997; Yasuhira and Simpson, 1997; Roy et al., 2007).
The mtGenome of E. gracilis comprises numerous DNA dispersed in different-sized linear fragments, as well as some circular fragments, two fragments of SSU and two of LSU of the rRNA (Roy et al., 2007; Flegontov and Lukeš, 2012; Moreira et al., 2012). Moreover, various dispersed fragments of three protein-coding genes (cox1, cox2, and cox3) have also been identified in the mitochondrial genome of E. gracilis (Spencer and Gray, 2011).
The regions adjacent to the gene fragmentation lack the classical organelle intron signature in the E. gracilis mitochondrial DNA (Moreira et al., 2012), and its significant fraction is non-coding and contains many repetitions, interspersed with fragments of a handful of protein-coding genes (Flegontov and Lukeš, 2012). Dobáková et al. (2015) study revealed not only the existence of the cytochrome B gene (gene cob) as well as the presence of nad1, nad4, and nad5 genes in mt-genome of E. gracilis. Genes encoding subunits of ATP synthase were not found in the mt-Genome of E. gracilis (Dobáková et al., 2015).
The structure and general organization of the mtGenome of E. gracilis are extremely close to those observed in the dinoflagellates, whose mtGenome also contains three protein-coding genes (cob, cox1, and cox3) in the form of numerous fragments of various sizes, thus reinforcing the hypothesis of cascades of convergent evolution between the two lineages (Lukeš et al., 2009; Spencer and Gray, 2011; Flegontov and Lukeš, 2012). According to this hypothesis, although the dinoflagellates and euglenozoans belong to phylogenetically distinct lineages, they share innumerable characters in addition to the mtGenome, e.g., the presence of a paraxonemal/paraflagellar rod, nucleus with trans-splicing, and a secondary chloroplast with three surrounding membranes, among others (Lukeš et al., 2009; Flegontov and Lukeš, 2012).
Nuclear Genomes
Approaches based on the nuclear genome and its components have been used to elucidate evolutionary processes among the prokaryotic and eukaryotic lineages, as well as phylogenies of different groups of organisms. In general, studies on nuclear genomes in euglenids are few. Among the phototrophic euglenids, most studies have dealt with E. gracilis, which even though its genomes has been only partly studied, was found to have unusual features such as the presence of rRNA genes located extrachromosomically, and by thousands of copies of circular molecules (Cook and Roxby, 1985). The single molecule of 28S rRNA was replaced by 13 short RNA molecules (Schnare and Gray, 1990), and a spice leader is transferred to the 5′-end of the majority of the pre-mRNA in the process depending on spliceosome-trans-splicing (Milanowski et al., 2014). A unique aspect was the presence of three types of introns in the nuclear genome of E. gracilis: (i) conventional, (ii) nonconventional, and (iii) intermediate (Breckenridge et al., 1999; Canaday et al., 2001; Vesteg et al., 2010; Milanowski et al., 2014). These introns have been studied in order to improve understanding of the evolution of the euglenids and their respective genomes, as well as to reconstruct the evolution of the gene architecture in eukaryotes (Rogozin et al., 2012).
Analysis of the tuba and tubb genes in 20 species of euglenids revealed different patterns of distribution of conventional and nonconventional introns. Whereas, the position of conventional introns is conservative, the nonconventional introns are unique to species or groups of closely related species (Milanowski et al., 2014).
Eleven events of losses of conventional introns, compared with 15 other events of acquisition of nonconventional introns, were identified in phototrophic forms by Milanowski et al. (2014). These results suggest that nonconventional introns were more commonly acquired during the course of their evolution than were the conventional introns, and that the two types of introns must have had different origins (Milanowski et al., 2014).
An important aspect to consider in the evolution of the genome and its components is the process of horizontal gene transfer (HGT), interpreted as an important directing force in the evolution of many groups of prokaryotes and eukaryotes, as well as their genomes (Keeling and Palmer, 2008). HGTs also constitute potential sources of information on phylogeny and on the adaptive evolution of species (Schönknecht et al., 2014; Katz, 2015). Most of the information on HGTs is on the prokaryotes, although an increasing number of studies have provided evidence of a concentration of large numbers of genes in the eukaryote nuclear genomes that are derived from organelles, by means of endosymbiotic gene transfer (EGT; Timmis et al., 2004; Schönknecht et al., 2014). The formation of organelles, accompanied by massive transfers of genes with subsequent evolution to the genomes of the host, has transferred complex functional systems, such as photosynthesis, leading to important evolutionary adaptations (Schönknecht et al., 2014). In phototrophic euglenids, some studies have indicated that HGT is a possible directing force of some evolutionary processes.
Analysis of an expressed sequence tag (EST) genetic marker in E. gracilis showed that the nuclear genes in this species have a complex history, and that many aspects of the integration of the nuclear genome of E. gracilis with the genes of the green alga acquired through secondary endosymbiosis remain unclear (Ahmadinejad et al., 2007). Additionally, molecular phylogenies suggest that genes of the “red lineage” are present in the nuclear genome of E. gracilis (Ahmadinejad et al., 2007; Sanchez-Perez et al., 2008). The presence of 14 genes originating from a red alga, based on analysis of an expanded EST survey using the laterally transferred gene mining pipeline, was detected in E. gracilis and in the heterotrophic P. trichophorum (Maruyama et al., 2011). The 14 phylogenetic trees based on proteins showed, for example, that the sequence of E. gracilis is monophyletic with the “red lineage” and that the homogentisate phytyltransferase (HPT) protein family of E. gracilis is intimately related to the group of the Chromalveolata and homologs of red algae (Maruyama et al., 2011). These data suggest that the lineage of Euglenida could have undergone a process of cryptic endosymbiosis with an alga with a red chloroplast, before the present cloroplast from green algae became established (Maruyama et al., 2011). Possibly, these genes were acquired through HGT between eukaryotes, resulting in the complex mosaic pattern in the genome of euglenids, suggesting multiple HGTs beginning with the red-alga lineage in the common ancestor of the euglenids (Maruyama et al., 2011).
Study of the evolutionary history of the enzymes that participate in the Calvin-Benson cycle in 9 heterotrophic and 20 phototrophic euglenids indicated that many of these enzymes were probably derived from processes of HGT and/or EGT. Nuclear-encoded plastid-targeted genes included in the Calvin-Benson cycle arise from different ancestors derived from multiple lineages of Rhodophyta and Chromophyta. Transfer of these genes to the nucleus probably preceded the acquisition of the green chloroplast in the euglenids (Markunas and Triemer, 2015).
Szabová et al. (2014) examined the distribution of paralogous genes encoding the enzymes MAT-MATX (methionine adenosyltransferase) in 21 photoautotrophic euglenids and two heterotrophic forms, R. viridis and Pyramimonas parkae. The phylogenetic trees showed that MATX was present only in the phototrophic forms (sub-clade with 70% bootstrap); both MAT and MATX in two phototrophic species; and only MAT in the heterotrophic euglenids R. viridis and P. parkae. Szabová et al. (2014) noted that these results, added to the incongruence between eukaryotic phylogenies based on MATX and SSU rDNA data, suggest that MATX appeared in the euglenid lineage in a single horizontal gene-transfer event (HGT) that occurred after the secondary endosymbiotic process of the euglenid chloroplast.
How many Clades are there within Euglenophyceae?
Currently, the Euglenophyceae encompasses three groups, represented by Rapaza, Eutreptiales and Euglenales (Adl et al., 2012). Rapaza includes only a single marine species, R. viridis, characterized by mixotrophic cells and a novel feeding apparatus composed of one rod of microtubules, a feeding pocket, and no vanes (Yamaguchi et al., 2012).
The Eutreptiales includes mainly marine and brackish, occasionally freshwater species, in the genera Eutreptia and Eutreptiella, that have phototrophic cells with a flexible pellicle formed by a relatively large number of helical strips, and no conspicuous feeding apparatus (Adl et al., 2012; Yamaguchi et al., 2012).
The Euglenales includes phototrophic and heterotrophic species that occur primarily in freshwater environments and have a flexible or rigid pellicle, frequently formed by a relatively large number of helical strips. Phototrophic forms have a pellicle with a posterior whorl of short strips, i.e., the strips do not reach the posterior end of the cell. The Euglenales is divided into two monophyletic families, Phacaceae and Euglenacaeae (Kim et al., 2010; Adl et al., 2012).
Reassessment of Genera and Species of Phototrophic Euglenids
According to Leedale (1967), the euglenid flagellates pose a series of problems for the taxonomist, including duplication at the species level, circumscription at the genus level, and assemblage at the order level. It is clear that many of Huber-Pestalozzi's (1955) 235 species of Euglena are unacceptable duplications, generated when size variations and ecological forms were described as separate species. For instance, Pringsheim (1956), using controlled culture conditions, reduced Huber-Pestalozzi's 235 species to 50 after removing synonyms and morphological expressions that were insufficiently described and unrecognizable.
Another important aspect raised by Leedale (1967) is the absence of sexuality in the euglenid flagellates, and therefore, the fact that many natural populations are asexual clones, an occurrence that may lead to the conclusion that a clone produced by a cell with a major morphological peculiarity (acquired by mutation or a copy error during cell division) can legitimately be described as a new “species.” Consequently, the species concept in euglenid flagellates remained, in practice, almost entirely morphological, i.e., species were delimited on the basis of morphology alone.
With the advent of and the recent advances in molecular phylogeny, taxonomy of the euglenid photoautotrophic forms changed, with proposals of new taxa and combinations. Marin et al. (2003) is the first taxonomic revision and phylogenetic study of Euglenophyceae using molecular data. In that work, among various taxonomical changes the authors based on nuclear-encoded SSU rRNA and SSU rRNA secondary structure redefined genera, reestablished the genus Monomorphina which is formed by some taxa belonging to traditional genera Phacus and Euglena, and moved a group of species of Euglena with rigid pellicle and many discoid chloroplast without pyrenoid to Lepocinclis (Marin et al., 2003). Many other studies continually improved refinement of genera and species concepts in Euglenophyceae. The genus Euglena remains problematic although some progress has been made, for example, the analysis of the phylogenetic relationship among species of Euglena and the taxonomy of E. viridis, based upon SSU and partial LSU rDNA (Shin and Triemer, 2004), the description of a new genus, Discoplastis, using SSU and LSU rDNA (Triemer et al., 2006), the reevaluation of the taxonomy of species from Euglena with a single axial stellate chloroplast, including the description of two new species based on nuclear SSU sequences and morphological data (Kosmala et al., 2009), the erection of new genus Euglenaria using combined sequences of nuclear SSU and LSU and of chloroplast 16S rDNA (Linton et al., 2010). More recently, the taxonomy of species from Euglena and Euglenaria was revisited, based on SSU rDNA and morphology (Karnkowska-Ishikawa et al., 2012, 2013), and Euglena proxima was moved into the new genus Euglenaformis applying chloroplast genome (Bennett et al., 2014). An investigation based upon SSU and LSU nuclear rDNA sequences, combined with morphological data, reinforced the separation between Trachelomonas and Strombomonas (Ciugulea et al., 2008). Other molecular studies using different genetic markers (nuclear-encoded genes, chloroplast-encoded gene, and nuclear encoded chloroplast gene) confirmed the separation of those two genera (Karnkowska et al., 2015; Kim et al., 2015). The concept of the genus Lepocinclis was broadened by including, among others, Euglena spirogyra, Euglena fusca, Euglena helicoideus, Phacus horridus based onSSU rDNA (Kosmala et al., 2005; Bennett and Triemer, 2012) and Cyclidiopsis acus using LSU rDNA (Bennett and Triemer, 2014). Studies based on combined gene sets also revealed cryptic diversity in some euglenid genera. Kim et al. (2013a) separated the genus Monomorphina into eight species, diagnosed primarily by DNA sequence differences in Helix 17 and the spacer in Helix 23 of the LSU rRNA gene; five of the species that they recognized were new. Kim et al. (2013b) recognized cryptic species in Cryptoglena, using a combined data set of nuclear SSU and LSU and plastid SSU and LSU rRNA genes, resulting in the segregation of the genus into five clades, two of which represented previously known species (Cryptoglena skujai and Cryptoglena pigra), and the other three were designated as new (Cryptoglena soropigra, Cryptoglena similis, and Cryptoglena longisulca). In all strains analyzed, the morphology showed no significant species-specific pattern (Kim et al., 2013b). Kim and Shin (2014), based on four genes from the cytoplasm and pt-encoded rDNA, found seven clades defining seven new species of Phacus (Phacus brevisulca, Phacus claviformis, Phacus hordeiformis, Phacus longisulca, Phacus minimus, Phacus paraorbicularis, and Phacus viridioryza). The new species were well-supported as independent taxa, and showed close relationships with small Phacus species (Kim and Shin, 2014).
Currently, green euglenids (Euglenophyceae) encompass the following 14 genera: Ascoglena, Euglena, Euglenaria, Euglenaformis, Eutreptia, Eutreptiella, Discoplastis, Phacus, Lepocinclis, Monomorphina, Cryptoglena, Colacium, Strombomonas, and Trachelomonas.
Lepocinclis and Discoplastis are circumscribed in the family Phacaceae, and their monophyly was supported in several studies (Milanowski et al., 2006; Triemer et al., 2006; Linton et al., 2010; Kim et al., 2015) as was the monophyly of the genera Monomorphina, Cryptoglena, Colacium, Strombomonas, and Trachelomonas (Kim et al., 2010, 2015; Karnkowska et al., 2015). The genus Phacus was originally recognized as a monophyletic member of the family Phacaceae by Kim et al. (2010). However, more-detailed studies based on multi-gene analyses carried out by Kim and Shin (2014) and Karnkowska et al. (2015) revealed the paraphyletic nature of the genus. In those two studies, Phacus limnophila and Phacus warszewiczii formed a basal lineage to the Lepocinclis clade. Kim et al. (2015) confirmed the monophyly of Phacus with increasing number of taxa as suggested by Kim and Shin (2014) and Karnkowska et al. (2015). Euglenaformis is positioned as a sister genus to all other Euglenaceae (Milanowski et al., 2006; Kim et al., 2010; Bennett et al., 2014). Eutreptia and Eutreptiella, both containing marine species, correspond to the basal lineage of the euglenid phototrophic clade, thus supporting the hypothesis of a marine origin of the photosynthetic euglenids (Leander et al., 2001; Hrdá et al., 2012). The Euglena and Euglenaria clades form a paraphyletic lineage, because Euglena archaeoplastidiata diverges from other members of Euglena and is a sister of Euglenaria (Kim et al., 2015).
Integrating both morphological features and molecular data in studies of euglenid evolution is not an easy task, because of their great plasticity. However, many studies combining both kinds of data have demonstrated that there are many stable and characteristic morphological aspects among the phototrophic lineages, such as cell shape, chloroplast morphology, cell plasticity, paramylon grain diversity, and the presence of mucus bodies, mucilaginous stalks, and loricas. Characters such as the presence of a lorica and mucilaginous stalks (apomorphic), rigid cells, and the presence of large paramylon grains (homoplasic) correspond to well-defined clades (Karnkowska et al., 2015). The classification of loricate genera and the circumscription of Colacium have always relied heavily on the morphology of the lorica and the mucilaginous stalk, respectively (Brosnan et al., 2005; Linton et al., 2010). Lorica ornamentation, ontogeny of the lorica development, and pellicle-strip reduction are apomorphies useful in differentiating Trachelomonas from Strombomonas (Brosnan et al., 2005), while large paramylon granule types and the presence/absence of large granules can be used at the generic level to support major clades and generic relationships (Monfils et al., 2011).
On the other hand, the characters that are widely used to circumscribe genera are homoplasic (e.g., lorica shape, presence of a distinctive collar and a tailpiece) and may lead to erroneous identification of taxa on the basis of the morphogenetic process (Brosnan et al., 2005). Some clades and species of Phacus show congruences between molecular phylogeny and morphological characteristics such as cell size, presence, or absence of sulcus, and number and shape of paramylon granules (Kim and Shin, 2014). Other characters such as location, shape and number of chloroplasts and pyrenoids, presence of paramylon caps and mucocysts still need further study in order to form a better evaluation of these characters in the identification of species and a better understanding of the evolutionary context of euglenids (for more details see Triemer and Farmer, 2007; Linton et al., 2010; Karnkowska et al., 2015).
What is the Cutting Edge of Phototrophic Euglenid Biodiversity Studies Today?
Studies on the phylogenetic relationships and morphological evolution among the phototrophic representatives of euglenids have resulted in significant progress, 18 years after molecular techniques were first applied to these questions. New genera and species were described based on single or multigene analyses, and new insights on evolution of phototrophic euglenids and their genomes (plastidial, mitochondrial, and nuclear) have provided more evidence about the organization and distribution of introns and enzymes across the euglenid lineage, supporting the hypothesis that HGTs are an important driving force in the evolution of the group.
One important aspect is the recent application of culture-independent molecular methods for describing new taxa in the group (Bennett and Triemer, 2012; Lax and Simpson, 2013; Łukomska-Kowalczyk et al., 2015). Culture-independent molecular methods open new prospects for better understanding of the diversity of microorganisms at the taxonomic or community level (Giraffa and Neviani, 2001). Since not all organisms can be cultured, the application of methods that are independent of culturing furnishes a truer idea of the diversity of microorganisms in different environments. Thus, the application of these methods will be able to give an idea of the abundance of euglenid taxa in the different environments, and will surely broaden our understanding of the diversity of the group, not only through new discoveries but also through analyses of little-known and/or rare taxa or groups of taxa.
The application of molecular techniques that do not depend on culturing can also broaden our understanding of the biodiversity of the phototrophic euglenids, which is still underestimated, mainly due to the paucity of studies carried out with tropical material.
It is essential to expand the present picture by using polyphasic approaches, i.e., integration of different kinds of data such as from morphology, genetics, and ecology, to reach a better understanding of the phylogenetic relationships among genera, and of the validity of morphological data for species delimitation in phototrophic euglenids.
The number of available sequences used in molecular-biology publications is still insufficient compared to the total estimated number of species in the group. Furthermore, these sequences are almost entirely derived from organisms from Europe, followed by the United States (Northern Hemisphere), and only a few from China and Argentina, which limits the construction of a more robust phylogeny for the group. The present body of knowledge lacks material and/or clones from other parts of the world, particularly the tropics.
The present moment is one of intense reflection worldwide: is it worthwhile to keep on describing new euglenid taxa (genera, species, varieties, and taxonomic formae) on the sole basis of their morphology? How much can molecular-biology data really contribute to the definition of new taxa? Of course, floristic studies must necessarily continue to use the morphological species concept. There is, at present, no other way to proceed. Whereas, a few floras have been published for Asia, Europe and North America (Northern Hemisphere), for the tropics the only published flora is an inventory of the Argentinean phototrophic euglenids (Tell and Conforti, 1986).
Continuing morphology-based work will undoubtedly increase the need for a molecular-biology approach to solve those problems that morphology cannot. In short, floristic studies will provide the underpinning for molecular-biology studies.
Author Contributions
All authors listed, have made substantial, direct and intellectual contribution to the work, and approved it for publication.
Conflict of Interest Statement
The authors declare that the research was conducted in the absence of any commercial or financial relationships that could be construed as a potential conflict of interest.
Acknowledgments
We thank the two anonymous reviewers for their constructive comments and suggestions to the manuscript.
References
Adl, S. M., Simpson, A. G., Lane, C. E., Lukeš, L., Bass, D., Bowser, S. S., et al. (2012). The revised classification of eukaryotes. J. Eukaryot. Microbiol. 59, 429–514. doi: 10.1111/j.1550-7408.2012.00644.x
Ahmadinejad, N., Dagan, T., and Martin, W. (2007). Genome history in the symbiotic hybrid Euglena gracilis. Gene 402, 35–39. doi: 10.1016/j.gene.2007.07.023
Bennett, M. S., and Triemer, R. E. (2012). A new method for obtaining nuclear gene sequences from field samples and taxonomic revisions of the photosynthetic euglenoids Lepocinclis (Euglena) helicoideus and Lepocinclis (Phacus) horridus (Euglenophyta). J. Phycol. 48, 254–260. doi: 10.1111/j.1529-8817.2011.01101.x
Bennett, M. S., and Triemer, R. E. (2014). The genus Cyclidiopsis: an obituary. J. Eukaryot. Microbiol. 61, 166–172. doi: 10.1111/jeu.12094
Bennett, M. S., and Triemer, R. E. (2015). Chloroplast genome evolution in the Euglenaceae. J. Eukaryot. Microbiol. 62, 773–785. doi: 10.1111/jeu.12235
Bennett, M. S., Wiegert, K. E., and Triemer, R. E. (2012). Comparative chloroplast genomics between Euglena viridis and Euglena gracilis (Euglenophyta). Phycologia 51, 711–718. doi: 10.2216/12-017.1
Bennett, M. S., Wiegert, K. E., and Triemer, R. E. (2014). Characterization of Euglenaformis gen. nov. and the chloroplast genome of Euglenaformis [Euglena] proxima (Euglenophyta). Phycologia 53, 66–73. doi: 10.2216/13-198.1
Breckenridge, D. G., Watanabe, Y.-I., Greenwood, S. J., Gray, M. W., and Schnare, M. N. (1999). U1 small nuclear RNA and spliceosomal introns in Euglena gracilis. Proc. Natl. Acad. Sci. U.S.A. 96, 852–856. doi: 10.1073/pnas.96.3.852
Breglia, S. A., Slamovits, C. H., and Leander, B. S. (2007). Phylogeny of phagotrophic euglenids (Euglenozoa) as inferred from hsp90 gene sequences. J. Eukaryot. Microbiol. 52, 86–94. doi: 10.1111/j.1550-7408.2006.00233.x
Breglia, S. A., Yubuki, N., Hoppenrath, M., and Leander, B. S. (2010). Ultra-structure and molecular phylogenetic position of a novel euglenozoan with extrusive episymbiotic bacteria: Bihospite bacati n. gen. et n. sp. (Symbiontida). BMC Microbiol. 10:145. doi: 10.1186/1471-2180-10-145
Brosnan, S., Brown, P. J., Farmer, M. A., and Triemer, R. E. (2005). Morphological separation of the euglenoid genera Trachelomonas and Strombomonas (Euglenophyta) based on lorica development and posterior strip reduction. J. Phycol. 41, 590–605. doi: 10.1111/j.1529-8817.2005.00068.x
Burki, F. (2014). The eukaryotic tree of life from a global phylogenomic perspective. Cold Spring Harb. Perspec. Biol. 6:a016147. doi: 10.1101/cshperspect.a016147
Busse, I., and Preisfeld, A. (2003). Systematics of primary osmotrophic euglenids: a molecular approach to the phylogeny of Distigma and Astasia (Euglenozoa). Int. J. Sys. Evol. Microbiol. 53, 617–624. doi: 10.1099/ijs.0.02295-0
Canaday, J., Tessier, L. H., Imbault, P., and Paulus, F. (2001). Analysis of E. gracilis alpha-, beta- and gamma-tubulin genes: introns and pre-mRNA maturation. Mol. Genet. Genomics 26, 153–160. doi: 10.1007/s004380000403
Ciugulea, I., Nudelman, M. A., Brosnan, S., and Triemer, R. E. (2008). Phylogeny of the euglenoid loricate genera Trachelomonas and Strombomonas (Euglenophyta) inferred from nuclear SSU and LSU rDNA. J. Phycol. 44, 406–418. doi: 10.1111/j.1529-8817.2008.00472.x
Cohn, F. (1853). Untersuchungen über die entwicklungsgeschichte der mikroskopischen algen und Pilze. Nova Acta Acad. Leop Carol. 24, 101–256.
Cook, J. R., and Roxby, R. (1985). Physical properties of a plasmid-like DNA from Euglena gracilis. Biochim. Biophys. Acta 824, 80–83.
Crouse, E. J., Vandrey, J. P., and Stutz, E. (1974). Hybridization studies with RNA and DNA isolated from Euglena gracilis chloroplasts and mitochondria. FEBS Lett. 42, 262–266.
Dobáková, E., Flegontov, P., Skalický, Y., and Lukeš, J. (2015). Unexpectedly streamlined mitochondrial genome of the euglenozoan Euglena gracilis. Genome Biol. Evol. 7, 3358–3367. doi: 10.1093/gbe/evv229
Doetsch, N. A., Thompson, M. D., and Hallick, R. B. (1998). A maturase-encoding group III twintron is conserved in deeply rooted euglenoid species: are group III introns the chicken or the egg? Mol. Biol. Evol. 15, 76–86.
Dujardin, F. (1841). Histoire Naturelle des Zoophytes: Infusoires, Comprenant la Physiologie et la Classification de ces Animaux et la Manière de les Étudier à L'aide du Microscope. Paris: Roret.
Ehrenberg, C. G. (1830). Neue Beobachtungen über blutartige erscheinungen in aegypten, Arabien und Sibirien, nebst einer Übersicht und kritik der früher bekannten. Ann. Phys. Chem. 94, 477–514.
Flegontov, P., and Lukeš, J. (2012). “Mitochondrial genomes of photosynthetic euglenids and Alveolates,” in Mitochondrial Genome Evolution, ed L. Maréchal-Drouard (Stuttgart: Elsevier Ltd.; Academic Press), 127–153.
Giraffa, G., and Neviani, E. (2001). DNA-based, culture-independent strategies for evaluating microbial communities in food-associated ecosystems. Int. J. Food Microbiol. 67, 19–34. doi: 10.1016/S0168-1605(01)00445-7
Gockel, G., and Hachtel, W. (2000). Complete gene map of the plastid genome of the nonphotosynthetic Euglenoid flagellate Astasia longa. Protist 151, 347–351. doi: 10.1078/S1434-4610(04)70033-4
Grant, J. R., and Katz, L. A. (2014). Building a phylogenomic pipeline for the eukaryotic tree of life-addressing deep phylogenies with genome-scale data. PLoS Curr. 6. doi: 10.1371/currents.tol.c24b6054aebf3602748ac042ccc8f2e9
Hallick, R. B., Hong, L., Drager, R. G., Favreau, M. R., Monfort, A., Orsat, B., et al. (1993). Complete sequence of Euglena gracilis chloroplast DNA. Nucleic Acids Res. 21, 3537–3544.
Hampl, V., Hug, L., Leigh, J. W., Dacks, J. B., Lang, B. F., Simpson, A. G. B., et al. (2009). Phylogenomic analyses support the monophyly of Excavata and resolve relationships among eukaryotic “supergroups.” Proc. Natl. Acad. Sci. U.S.A. 106, 3859–3864. doi: 10.1073/pnas.0807880106
Hollande, A. (1942). Étude cytologique et biologique de quelques Flagellées libres: volvocales, cryptomonadines, eugléniens, promastigines. Arch. Zoo. Exp. Gén. 83, 1–268.
Hrdá, Š., Fousek, J., Szabová, J., Hampl, V., and Vlček, Č. (2012). The plastid genome of Eutreptiella provides a window into the process of secondary endosymbiosis of plastid in euglenids. PLoS ONE 7:e33746. doi: 10.1371/journal.pone.0033746
Huber-Pestalozzi, G. (1955). “Euglenophyceae,” in Das Phytoplankton des Süβwassers: Systematik und Biologie, Vol. 16, ed G. Huber-Pestalozzi (Stuttgart: E. Schweizerbart'sche Verlagsbuchhandlung), 606.
Johnson, L. P. (1968). “The taxonomy, phylogeny and evolution of the genus Euglena,” in The Biology of Euglena: General Biology and Ultrastructure, Vol. 1 ed D. E. Buetow (New York, NY: Academic Press), 1–24.
Karnkowska, A., Bennett, M. S., Watza, D., Kim, J. I., Zakryœ, B., and Triemer, R. E. (2015). Phylogenetic relationships and morphological character evolution of photosynthetic euglenids (Excavata) inferred from taxon-rich analyses of five genes. J. Eukaryot. Microbiol. 62, 362–373. doi: 10.1111/jeu.12192
Karnkowska-Ishikawa, A., Milanowski, R., Triemer, R. E., and Zakryœ, B. (2012). Taxonomic revisions of morphologically similar species from two euglenoid genera: Euglena (E. granulata and E. velata) and Euglenaria (Eu. anabaena, Eu. caudata, and Eu. clavata). J. Phycol. 48, 729–739. doi: 10.1111/j.1529-8817.2012.01140.x
Karnkowska-Ishikawa, A., Milanowski, R., Triemer, R. E., and Zakryœ, B. (2013). A redescription of morphologically similar species from the genus Euglena: E. laciniata, E. sanguinea, E. sociabilis, and E. splendens. J. Phycol. 49, 616–626. doi: 10.1111/jpy.12072
Katz, L. A. (2012). Origin and diversification of eukaryotes. Ann. Rev. Microbiol. 66, 411–427. doi: 10.1146/annurev-micro-090110-102808
Katz, L. A. (2015). Recent events dominate interdomain lateral gene transfers between prokaryotes and eukaryotes and, with the exception of endosymbiotic gene transfers, few ancient transfer events persist. Philos. Trans. R. Soc. B 370:20140324. doi: 10.1098/rstb.2014.0324
Keeling, P. J., and Palmer, J. D. (2008). Horizontal gene transfer in eukaryotic evolution. Nat. Rev. Genet. 9, 605–618. doi: 10.1038/nrg2386
Kiethega, G. N., Turcotte, M., and Burger, G. (2011). Evolutionarily conserved cox1 trans-splicing without cis-motifs. Mol. Biol. Evol. 28, 2425–2428. doi: 10.1093/molbev/msr075
Kim, E., Simpson, A. G. B., and Graham, L. E. (2006). Evolutionary relationships of apusomonads inferred from taxon-rich analyses of 6 nuclear encoded genes. Mol. Biol. Evol. 23, 2455–2466. doi: 10.1093/molbev/msl120
Kim, J. I., Linton, E. W., and Shin, W. (2015). Taxon-rich multigene phylogeny of the photosynthetic euglenoids (Euglenophyceae). Front. Ecol. Evol. 20:98. doi: 10.3389/fevo.2015.00098
Kim, J. I., and Shin, W. (2008). Phylogeny of the Euglenales inferred from plastid LSU rDNA sequences. J. Phycol. 44, 994–1000. doi: 10.1111/j.1529-8817.2008.00536.x
Kim, J. I., and Shin, W. (2014). Molecular phylogeny and cryptic diversity of the genus Phacus (Phacaceae, Euglenophyceae) and the descriptions of seven new species. J. Phycol. 50, 948–959. doi: 10.1111/jpy.12227
Kim, J. I., Shin, W., and Triemer, R. E. (2010). Multigene analyses of photosynthetic euglenoid and new family Phacaceae (Euglenales). J. Phycol. 46, 1278–1287. doi: 10.1111/j.1529-8817.2010.00910.x
Kim, J. I., Shin, W., and Triemer, R. E. (2013a). Phylogenetic reappraisal of the genus Monomorphina (Euglenophyceae) based on molecular and morphological data. J. Phycol. 49, 82–91. doi: 10.1111/jpy.12018
Kim, J. I., Shin, W., and Triemer, R. E. (2013b). Cryptic speciation in the genus Cryptoglena (Euglenaceae) revealed by nuclear and plastid SSU and LSU rRNA gene. J. Phycol. 49, 92–102. doi: 10.1111/jpy.12032
Klebs, G. (1883). Über die organization einiger flagellatengruppen und ihre beziehungen zu algen und infusorien. Unter. Bot. Insti Tüb. 1, 233–362.
Kosmala, S., Karnkowska, A., Milanowski, R., Kwiatowski, J., and Zakryœ, B. (2005). The phylogenetic and taxonomic position of Lepocinclis fusca comb. nov. (= Euglena fusca) (Euglenaceae): morphological and molecular justification. J. Phycol. 41, 258–267. doi: 10.1111/j.1529-8817.2005.00141.x
Kosmala, S., Karnkowska-Ishikawa, A., Milanowski, R., Kwiatowski, J., and Zakryœ, B. (2009). Phylogeny and systematics of Euglena (Euglenaceae) species with axial, stellate chloroplasts based on morphological and molecular data-new taxa, ememded diagnoses, and epitypifications. J. Phycol. 45, 464–481. doi: 10.1111/j.1529-8817.2009.00653.x
Lax, G., and Simpson, A. G. B. (2013). Combining molecular data with classical morphology for uncultured phagotrophic euglenids (Excavata): a single-cell approach. J. Eukaryot. Microbiol. 60, 615–625. doi: 10.1111/jeu.12068
Leander, B. S., Esson, H. J., and Breglia, S. A. (2007). Macroevolution of complex cytoskeletal systems in euglenids. Bioessays 29, 987–1000. doi: 10.1002/bies.20645
Leander, B. S., Triemer, R. E., and Farmer, M. A. (2001). Character evolution in heterotrophic euglenids. Eur. J. Protistol. 37, 337–356. doi: 10.1078/0932-4739-00842
Leedale, G. F. (1978). Phylogenetic criteria in euglenoid flagellates. Biosystems 10, 183–187. doi: 10.1016/0303-2647(78)90040-0
Linton, E. W., Hittner, D., Lewandowski, C., Auld, T., and Triemer, R. E. (1999). A molecular study of euglenoid phylogeny using small subunit rDNA. J. Eukaryot. Microbiol. 46, 217–223. doi: 10.1111/j.1550-7408.1999.tb04606.x
Linton, E. W., Karnkowska-Ishikawa, A., Kim, J. I., Shin, W., Bennett, M., Kwiatowski, J., et al. (2010). Reconstructing Euglenoid evolutionary relationships using three genes: nuclear SSU and LSU, and chloroplast 16S rDNA sequences and the description of Euglenaria gen. nov. (Euglenophyta). Protist 161, 603–619. doi: 10.1016/j.protis.2010.02.002
Linton, E. W., Nudelman, M. A., Conforti, V., and Triemer, R. E. (2000). A molecular analysis of the Euglenophytes using SSU rDNA. J. Phycol. 36, 740–746. doi: 10.1046/j.1529-8817.2000.99226.x
Lukeš, J., Leander, B. S., and Keeling, P. J. (2009). Cascades of convergent evolution: the corresponding evolutionary histories of euglenozoans and dinoflagellates. Proc. Natl. Acad. Sci. U.S.A. 106, 9963–9970. doi: 10.1073/pnas.0901004106
Łukomska-Kowalczyk, M., Karnkowska, A., Milanowski, R., Łach, Ł., and Zakryś, B. (2015). Delimiting species in the Phacus longicauda complex (Euglenida) through morphological and molecular analyses. J. Phycol. 51, 1147–1157. doi: 10.1111/jpy.12352
Marande, W., and Burger, G. (2007). Mitochondrial DNA as a genomic jigsaw puzzle. Science 318, 415. doi: 10.1126/science.1148033
Marande, W., Lukeš, J., and Burger, G. (2005). Unique mitochondrial genome structure in diplonemids, the sister group of kinetoplastids. Eukaryot. Cell 4, 1137–1146. doi: 10.1128/EC.4.6.1137-1146.2005
Marin, B., Palm, A., Klingberg, M., and Melkonian, M. (2003). Phylogeny and taxonomic revision of plastid-containing Euglenophytes based on SSU rDNA sequence comparisons and synapomorphic signatures in the SSU rRNA secondary structure. Protist 154, 99–145. doi: 10.1078/143446103764928521
Markunas, C. M., and Triemer, R. E. (2015). Evolutionary history of the enzymes involved in the Calvin Benson cycle in euglenids. J. Eukaryot. Microbiol. doi: 10.1111/jeu.12282. [Epub ahead of print].
Maruyama, S., Suzaki, T., Weber, A. P. M., Archibald, J. M., and Nozaki, H. (2011). Eukaryote-to-eukaryote gene transfer gives rise to genome mosaicism in euglenids. BMC Evol. Biol. 11:105. doi: 10.1186/1471-2148-11-105
Milanowski, R., Karnkowska, A., Ishikawa, T., and Zakryś, B. (2014). Distribution of conventional and nonconventional introns in Tubulin (α and β) genes of euglenids. Mol. Biol. Evol. 31, 584–593. doi: 10.1093/molbev/mst227
Milanowski, R., Kosmala, S., Zakryś, B., and Kwiatowski, J. (2006). Phylogenetic analysis of photosynthetic Euglenophytes based on combined chloroplast and cytoplasmic SSU rDNA sequences. J. Phycol. 42, 721–730. doi: 10.1111/j.1529-8817.2006.00216.x
Milanowski, R., Zakryś, B., and Kwiatowski, J. (2001). Phylogenetic analysis of chloroplast small-subunit rRNA genes of the genus Euglena Ehrenberg. Int. J. Syst. Evol. Microbiol. 51, 773–781. doi: 10.1099/00207713-51-3-773
Monfils, A. K., Triemer, R. E., and Bellairs, E. F. (2011). Characterization of paramylon morphological diversity in photosynthetic euglenoids (Euglenales, Euglenophyta). Phycologia 50, 156–169. doi: 10.2216/09-112.1
Moreira, D., López-García, P., and Rodríguez-Valera, F. (2001). New insights into phylogenetic position of diplonemids: G + C contents bias, differences of evolutionary rate and a new environmental sequence. Int. J. Syst. Evol. Microbiol. 51, 2211–2219. doi: 10.1099/00207713-51-6-2211
Moreira, S., Breton, S., and Burger, G. (2012). Unscrambling genetic information at the RNA level. Wiley Interdiscip. Rev. RNA 3, 213–228. doi: 10.1002/wrna.1106
Müllner, A. N., Angeler, D. G., Samuel, R., Linton, E. W., and Triemer, R. E. (2001). Phylogenetic analysis of phagotrophic, phototrophic and osmotrophic euglenoids by using the nuclear 18S rDNA sequence. Int. J. Syst. Evol. Microbiol. 51, 783–791. doi: 10.1099/00207713-51-3-783
Nass, M. M. K., Schori, L., Ben-Shaul, Y., and Edelman, M. (1974). Size and configuration of mitochondrial DNA in Euglena gracilis. Biochim. Biophys. Acta 374, 283–291. doi: 10.1016/0005-2787(74)90249-4
Nudelman, M. A., Rossi, M. S., Conforti, V., and Triemer, R. E. (2003). Phylogeny of Euglenophyceae based on small subunit rDNA sequences: taxonomic implications. J. Phycol. 39, 226–235. doi: 10.1046/j.1529-8817.2003.02075.x
Pombert, J.-F., James, E. R., Janouškovec, J., and Keeling, P. J. (2012). Evidence for transitional stages in the evolution of Euglenid group II introns and twintrons in the Monomorphina aenigmatica plastid genome. PLoS ONE 7:e53433. doi: 10.1371/journal.pone.0053433
Pringsheim, E. G. (1956). Contribution towards a monograph of the genus Euglena. Nova Acta Leopol. Nov. Ser. 18, 3–168.
Ray, D. S., and Hanawalt, P. C. (1965). Satellite DNA components in Euglena gracilis cells lacking chloroplasts. J. Mol. Biol. 11, 760–768. doi: 10.1016/S0022-2836(65)80033-X
Rogozin, I. B., Carmel, L., Csuros, M., and Koonin, E. V. (2012). Origin and evolution of spliceosomal introns. Biol. Direct 7, 6150–6157. doi: 10.1186/1745-6150-7-11
Roy, J., Faktorová, D., Lukeš, J., and Burger, G. (2007). Unusual mitochondrial genome structures throughout the Euglenozoa. Protist 158, 385–396. doi: 10.1016/j.protis.2007.03.002
Sanchez-Perez, G. F., Hampl, V., Simpson, A. G. B., and Roger, A. J. (2008). A new divergent type of eukaryotic methionine adenosyltransferase is present in multiple distantly related secondary algal lineages. J. Eukaryot. Microbiol. 55, 374–381. doi: 10.1111/j.1550-7408.2008.00349.x
Schnare, M. N., and Gray, M. W. (1990). Sixteen discrete RNA components in the cytoplasmic ribosome of Euglena gracilis. J. Mol. Biol. 215, 73–83. doi: 10.1016/S0022-2836(05)80096-8
Schönknecht, G., Weber, A. P. M., and Lercher, M. J. (2014). Horizontal gene acquisitions by eukaryotes as drivers of adaptive evolution. Bioessays 36, 9–20. doi: 10.1002/bies.201300095
Sheveleva, E. V., and Hallick, R. B. (2004). Recent horizontal transfer to a chloroplast genome. Nucleic Acids Res. 32, 803–810. doi: 10.1093/nar/gkh225
Shin, W., Brosnan, S., and Triemer, R. E. (2002). Are cytoplasmic pockets (MTR/pocket) present in all photosynthetic euglenoid genera? J. Phycol. 38, 790–799. doi: 10.1046/j.1529-8817.2002.01208.x
Shin, W., and Triemer, R. E. (2004). Phylogenetic analysis of the genus Euglena (Euglenophyceae) with the particular reference to the type species Euglena viridis. J. Phycol. 40, 758–771. doi: 10.1111/j.1529-8817.2004.03142.x
Simpson, A. G. B. (1997). The identity and composition of the Euglenozoa. Arch. Protistenk. 148, 318–328. doi: 10.1016/s0003-9365(97)80012-7
Simpson, A. G. B., Gill, E. E., Callahan, H. A., Litaker, R. W., and Roger, A. J. (2004). Early evolution within kinetoplastids (Euglenozoa), and the late emergence of trypanosomatids. Protist 155, 407–422. doi: 10.1078/1434461042650389
Simpson, A. G. B., Inagaki, Y., and Roger, A. J. (2006). Comprehensive multi-gene phylogenies of excavate protists reveal the evolutionary positions of “primitive” eukaryotes. Mol. Biol. Evol 23, 615–625. doi: 10.1093/molbev/msj068
Simpson, A. G. B., and Roger, A. J. (2004). Protein phylogenies robustly resolve the deep-level relationships within Euglenozoa. Mol. Phylogenet. Evol. 30, 201–212. doi: 10.1016/S1055-7903(03)00177-5
Sogin, M. L., Elwood, H. J., and Gunderson, J. H. (1986). Evolutionary diversity of eukaryotic small-subunit rRNA genes. Proc. Natl. Acad. Sci. U.S.A. 83, 1383–1387. doi: 10.1073/pnas.83.5.1383
Spencer, D. F., and Gray, M. W. (2011). Ribosomal RNA genes in Euglena gracilis mitochondrial DNA: fragmented genes in a seemingly fragmented genome. Mol. Genet. Genomics 285, 19–31. doi: 10.1007/s00438-010-0585-9
Szabová, J., Yubuki, N., Leander, B. S., Triemer, R. E., and Hampl, V. (2014). The evolution of paralogous enzymes MAT and MATX within the Euglenida and beyond. BMC Evol. Biol. 14:25. doi: 10.1186/1471-2148-14-25
Talke, S., and Preisfeld, A. (2002). Molecular evolution of euglenozoan paraxonemal rod genes par1 and par2 coincides with phylogenetic reconstruction based on small subunit rDNA data. J. Phycol. 38, 995–1003. doi: 10.1046/j.1529-8817.2002.02028.x
Tell, G., and Conforti, V. (1986). Euglenophyta pigmentadas de la Argentina. Bibl. Phycol. 75, 1–301.
Tessier, L. H., van der Speck, H., Gualberto, J. M., and Grienenberger, J. M. (1997). The cox1 gene from Euglena gracilis: a protist mitochondrial gene without introns and genetic code modifications. Curr. Genet. 31, 208–213. doi: 10.1007/s002940050197
Thompson, M. D., Copertino, D. W., Thompson, E., Favreau, M. R., and Hallick, R. B. (1995). Evidence for the late origin of introns in chloroplast genes from an evolutionary analysis of the genus Euglena. Nucleic Acids Res. 23, 745–752. doi: 10.1093/nar/23.23.4745
Timmis, J. N., Ayliffe, M. A., Huang, C. Y., and Martin, W. (2004). Endosymbiotic gene transfer: organelle genomes forge eukaryotic chromosomes. Nat. Rev. Genet. 5, 123–135. doi: 10.1038/nrg1271
Triemer, R., and Farmer, M. A. (1991). “The ultrastructural organization of the heterotrophic euglenids and its evolutionary implications,” in The Biology of Free-Living Heterotrophic Flagellates, eds D. Patterson and J. Larsen (Oxford: Clarendon Press), 185–205.
Triemer, R. E., and Farmer, M. A. (2007). “A decade of euglenoid molecular phylogenetics,” in Unraveling the Algae: the Past, Present and Future of Algal Systematics, eds Brodie and J. Lewis (Boca Raton, FL: CRC Press), 315–330.
Triemer, R. E., Linton, E., Shin, W., Nudelman, A., Monfils, A., Bennett, M., et al. (2006). Phylogeny of the Euglenales based upon combined SSU and LSU rDNA sequence comparisons and description of Discoplastis gen. nov. (Euglenophyta). J. Phycol. 42, 731–740. doi: 10.1111/j.1529-8817.2006.00219.x
Turmel, M., Gagnon, M. C., O'Kelly, C. J., Otis, C., and Lemieux, C. (2009). The chloroplast genomes of the green algae Pyramimonas, Monomastix, and Pycnococcus shed new light on the evolutionary history of prasinophytes and the origin of the secondary chloroplasts of euglenids. Mol. Biol. Evol. 26, 631–648. doi: 10.1093/molbev/msn285
Vesteg, M., Vacula, R., Steiner, J. M., Mateášiková, B., Löffelhardt, W., Brejová, B., et al. (2010). A possible role for short introns in the acquisition of stroma-targeting peptides in the flagellate Euglena gracilis. DNA Res. 17, 223–231. doi: 10.1093/dnares/dsq015
Wiegert, K. E., Bennett, M. S., and Triemer, R. E. (2012). Evolution of the chloroplast genome in photosynthetic euglenoids: a comparison of Eutreptia viridis and Euglena gracilis (Euglenophyta). Protist 163, 832–843. doi: 10.1016/j.protis.2012.01.002
Wiegert, K. E., Bennett, M. S., and Triemer, R. E. (2013). Tracing patterns of chloroplast evolution in euglenoids: contributions from Colacium vesiculosum and Strombomonas acuminata (Euglenophyta). J. Eukaryot. Microbiol. 60, 214–221. doi: 10.1111/jeu.12025
Yabuki, A., Nakayama, T., Yubuki, N., Hashimoto, T., Ishida, K.-I., and Inagaki, Y. (2011). Tsukubamonas globosa n. gen., n. sp., a novel excavate flagellate possibly holding a key for the early evolution in “Discoba.” J. Eukaryot. Microbiol. 58, 319–331. doi: 10.1111/j.1550-7408.2011.00552.x
Yamaguchi, A., Yubuki, N., and Leander, B. S. (2012). Morphostasis in a novel eukaryote illuminates the evolutionary transition from phagotrophy to phototrophy: description of Rapaza viridis n. gen. et n. sp. (Euglenozoa, Euglenida). BMC Evol. Biol. 12:29. doi: 10.1186/1471-2148-12-29
Yasuhira, S., and Simpson, L. (1997). Phylogenetic affinity of mitochondria of Euglena gracilis and kinetoplastids using cytochrome oxidase I and hsp60. J. Mol. Evol. 44, 341–347. doi: 10.1007/PL00006152
Yubuki, N., Edgcomb, V. P., Bernhardt, J. M., and Leander, B. S. (2009). Ultrastructure and molecular phylogeny of Calkinsia aureus: cellular identity of a novel clade of deep-sea euglenozoans with epibiotic bacteria. BMC Microbiol. 9:16. doi: 10.1186/1471-2180-9-16
Yubuki, N., Simpson, A. G. B., and Leander, B. S. (2013). Reconstruction of the feeding apparatus in Postgaardi mariagerensis provides evidence for character evolution within the Symbiontida (Euglenozoa). Eur. J. Protistol. 49, 32–39. doi: 10.1016/j.ejop.2012.07.001
Zakryś, B., Milanowski, R., Empel, J., Borsuk, P., Gromadka, R., and Kwiatowski, J. (2002). Two different species of Euglena, E. geniculata and E. myxocylindracea (Euglenophyceae), are virtually genetically and morphologically identical. J. Phycol. 38, 1190–1199. doi: 10.1046/j.1529-8817.2002.02020.x
Glossary
1. ATP synthase (adenosine triphosphate syntetase): Enzyme that catalyzes the synthesis of ATP from adenosine diphosphate (ADP) and inorganic phosphate (Pi) under aerobic and anaerobic cell growth.
2. Bodonids: Include organisms that form an order of Kinetoplastea.
3. Closed mitosis: A type of cell division in which the nuclear membrane remains intact throughout the mitotic cell cycle.
4. Collar: A sharply defined neck surrounding an apical pore through which the flagellum emerges that occurs in Trachelomonas genus.
5. Conventional intron: Formed by five snRNPs (small nuclear ribonucleoprotein particles), together with numerous less stably associated proteins; the core of the spliceosome is conserved in all well-characterized eukaryotes. The spliceosome interacts with specific sites in the intron and the flanking exons to ensure accurate and efficient splicing. They have canonical intron boundary nucleotides- containing characteristic GT…AG boundary sequences
6. Cytopharynx: A gullet-like canal or groove between the cytostome and the endoplasm, through which food is ingested.
7. Cytoskeleton: Network of microtubules and microfilaments that provide shape, mechanical resistance to deformation, and aid for cell migration; it also aids intracellular transport and movement.
8. Cytostome: The mouth opening into the cytopharynx.
9. Extrusomes: Membrane-bounded extrusible bodies usually located beneath the pellicle, which are ejected under chemical or mechanical stimulation.
10. Flagellar roots: Microtubular or fibrous, sometimes amorphous structures that originate from a protruding basal body of the flagellum.
11. GAPDH (glyceraldehyde-3-phosphate dehydrogenase): This gene encodes a member of the glyceraldehyde-3-phosphate dehydrogenase protein family. The encoded protein has been identified as a moonlighting protein, based on its ability to perform mechanistically distinct functions. The encoded protein was originally identified as a key glycolytic enzyme that converts D-glyceraldehyde 3-phosphate (G3P) into 3-phospho-D-glyceroyl phosphate.
12. Intermediate intron: Characterized by properties of both conventional and nonconventional introns (e.g., shows a “GT” _5_' _SS dinucleotide and terminal base pairing).
13. Intron: A DNA section of a gene that does not encode any part of the protein produced by the gene. The intron is initially transcribed pre-molecule mRNA, but is then eliminated during the RNA maturation process (or splice) before leaving the cell nucleus. Introns exist mainly in eukaryotic cells.
14. Kinetoplast ( = kDNA): DNA molecule within a large mitochondrion (several copies of the mitochondrial genome) attached to the basal bodies.
15. Lorica: A mineralized envelope or shell-like protective outer covering with an apical opening through which the flagellum/flagella emerge: it varies in shape, size, and ornamentation.
16. Mastigonemes: Also termed flagellar hairs, these are fine filamentous appendages associated with the flagella in many flagellates. They are usually arranged in one or more rows of thin, delicate, non-tubular units. In contrast, tubular hairs consist of two distinct regions, one of which is thick and tubular. In the case of euglenids, mastigonemes are non-tubular.
17. MAT (methionine adenosyltransferase): Is an enzyme that catalyzes the synthesis of S- adenosylmethionine, which is the major methyl donor and used as a substrate in a variety of methylation reactions.
18. MATX: Genes encoding a divergent form of MAT.
19. Metaboly: Euglenoid movements with irregular changes in the cell shape through various peristaltic-like motions.
20. Mixotrophic: Feeding which combine phototrophic and heterotrophic nutrition modes.
21. MRT: A complex array of microtubules associated with the feeding apparatus of some phagotrophic and phototrophic flagellates; sometimes understood as a vestigial cytopharynx or vestigial feeding apparatus (Shin et al., 2002).
22. Mucocystis ( = mucus bodies): A kind of ejectile organelle or extrusome located beneath the cell surface, uniform in size, spherical or spindle-shaped, with openings located between periplast folds.
23. NAD (nicotinamide adenine dinucleotide): Ubiquitous coenzyme that occurrs in all living cells, and functions in oxidizing (NAD+) and reduction reactions (NADH); nad1, nad2, nad3 are NADH subunits.
24. NHS (non-homoplasious synapomorphies): Characters without known parallels in any other taxa, “sensu” Marin et al. (2003).
25. Non-conventional intron: Formed by non-canonical and variable dinucleotides at the borders and by the presence of two inverted repeats that are adjacent to each border of the intron. These repeats would have the capacity for base pairing and for bringing together both splice sites in order to constitute a distinct, and probably spliceosome-free mechanism for splicing. They are not similar to GT…AG intron boundary sequences.
26. Osmotrophic: Heterotrophic nutrition in which feeding is by absorption or uptake of dissolved organic molecules by osmosis.
27. Paramylon: It is first of all storage material for euglenids formed by polymerization of a large number of β-1, 3 glucans.
28. Pellicle: The cytoskeletal complex of euglenids, consisting of the plasma membrane, proteinaceous strips, microtubules, and tubular cisternae of the endoplasmic reticulum.
29. PFR (paraxonemal rods): Also termed paraxial rods, are composed of discrete filaments structured in lattice-like arrays, with three distinct domains: proximal, intermediate and distal. Each domain is formed by a complex array of 25 nm-thick filaments intercrossed by 7-nm filaments at an angle of 100°.
30. Phagotrophic: Heterotrophic nutrition in which feeding is by engulfement and digestion of other cells or particles.
31. Polycistronic: A kind of messenger RNA that encodes several proteins and is characteristic of many bacterial and chloroplast mRNAs.
32. Psb: Gene encoding the CP43 protein of photosystem II of photosynthesis.
33. Pyrenoids: Usually roughly spherical and highly refractive bodies associated with a carbon-concentrating mechanism (CCM), which is composed primarily of the Calvin-cycle enzyme Ribulose-1,5-Bis-Phosphate Carboxylase/Oxygenase (RuBisCo), sometimes surrounded by a starch sheath; in euglenids the sheath is formed by accumulation of paramylon.
34. Rods: Cluster of microtubules and amorphous proteinaceous material longitudinally oriented within the cell, present in phagotrophic euglenids. Together with the vanes, the rods form the feeding structures, giving structural support to the cytostome when engulfing prey cells.
35. Spliced leader (SL): Is a gene that generates a functional ncRNA that is composed of two regions: an intronic region of unknown function (SLi) and an exonic region (SLe), which is transferred to the 5′-end of independent transcripts yielding mature mRNAs, in a process known as spliced leader trans-splicing (SLTS).
36. Spliceosome: Formed by five small nuclear RNAs (small nuclear ribonucleoprotein particles) and more than 100 associated proteins that spliced out noncoding introns at the initial transcript from DNA to RNA in the eukaryotic cell nucleus.
37. Splicing: Process that removes introns and exons during RNA transcription. Splicing occurs only in eukaryotic cells, since the DNA of prokaryotic cells lacks introns. The snRNA or ribozyme is responsible for cleaving the bonds between nucleotides.
38. Sulcus: Apical groove that occurs in Phacus genus.
39. Synteny: Set of orthologous genes that have the same local organization in different species, i.e., the sequence conservation among chromosome segments of two or more species.
40. Trans-splicing: Mechanism acting by connecting transcripts of otherwise unrelated genes.
41. Twintron: Special intronic arrangement in which introns of two different types occupy the same gene position.
42. Vanes: A set of membranous folds that surround the cytostome in phagotrophic euglenids. Vanes originate from the rods. As the prey is engulfed, the vanes rotate in a pinwheel-like fashion, creating a space within the feeding apparatus.
Keywords: Euglenophyceae, molecular biology, morphology, phylogeny, taxonomy
Citation: Bicudo CEdM and Menezes M (2016) Phylogeny and Classification of Euglenophyceae: A Brief Review. Front. Ecol. Evol. 4:17. doi: 10.3389/fevo.2016.00017
Received: 11 February 2015; Accepted: 16 February 2016;
Published: 16 March 2016.
Edited by:
Jan Maria Kwiatowski, University of Warsaw, PolandReviewed by:
Alberto G. Sáez, Instituto de Salud Carlos III, SpainAnna Karnkowska, University of British Columbia, Canada
Copyright © 2016 Bicudo and Menezes. This is an open-access article distributed under the terms of the Creative Commons Attribution License (CC BY). The use, distribution or reproduction in other forums is permitted, provided the original author(s) or licensor are credited and that the original publication in this journal is cited, in accordance with accepted academic practice. No use, distribution or reproduction is permitted which does not comply with these terms.
*Correspondence: Carlos E. de M. Bicudo, Y2JpY3Vkb0B0ZXJyYS5jb20uYnI=