- Wildlife Conservation Research Unit, Department of Zoology, The Recanati-Kaplan Centre, University of Oxford, Tubney, UK
The societies of group-living carnivores that neither hunt nor interact cooperatively may arise due to ecological drivers and/or constraints. In this study we evaluate whether group-living may be intrinsically associated with fossoriality; a link that is well supported in other taxa, but hitherto under-evaluated in the Carnivora. We make two over-arching predictions: (i) that fossoriality will be associated with carnivoran sociality; and (ii) that this association will be most evident in those species making extended use of subterranean dens. From a meta-analysis of key behavioral, ecological, ontological, and trophic traits, we demonstrate that three quarters of carnivore species exhibit some reliance on underground dens. Congruence between life-history traits and metrics of fossoriality evidenced that: (1) there are phylogenetic, and morphological constraints on wholly fossorial life-histories; (2) fossoriality correlated positively with the extent of offspring altriciality, linked to the use of natal dens; (3) burrow use increased with latitude; and (4) insectivorous carnivores were more fossorial than predatory carnivores. Corroborating work in the Rodentia, fossorial traits associated strongly with carnivoran group-living tendencies, where species utilizing subterranean natal dens are 2.5 times more likely to form groups than those that do not. Furthermore, using comparative analyses, we evidence support for an evolutionary relationship between diet, fossoriality, and sociality. We propose that fossorial dens act as a safe haven, promoting fitness benefits, territorial inheritance and cooperative breeding. We conclude that, among smaller (< 15 kg) den-using carnivores, and especially for omnivorous/insectivorous species for which food resource dispersion is favorable, continued cohabitation at natal dens can promote cohabitation among adults; that is, philopatric benefits leading to (not necessarily cooperative) spatial groups.
Introduction
Understanding the factors that enable conspecifics to congregate, or establish cooperative societies is central to socio-ecology (Alexander, 1974; Smith et al., 2012; Macdonald and Johnson, 2015). Axiomatically, group-living is theorized to evolve when the fitness benefits of joining/remaining in a (natal) group outweigh the costs of sharing key resources (e.g., Alexander, 1974; Emlen, 1982; von Schantz, 1984; Bacon et al., 1991; Koenig et al., 1992), and/or when there are strong ecological constraints on reproducing independently of the group (Lindström, 1986; Hatchwell and Komdeur, 2000; Kokko and Ekman, 2002). Among many of the Carnivora, the benefits of group-living manifest through enhanced hunting ability, where packing power facilitates endurance hunting (e.g., gray wolves, Canis lupus: MacNulty et al., 2014), or strategic kills (e.g., lions, Panthera leo: Mosser and Packer, 2009). There are, however, numerous group-living carnivores that do not hunt, or interact co-operatively (reviewed in Gittleman, 1989; Creel and Macdonald, 1995; Macdonald et al., 2004a; Macdonald and Johnson, 2015).
We observe that among gregarious, yet broadly non-cooperative carnivore species, many tend to have a substantial reliance on subterranean dens. There are two provisos to this observation: (i) these carnivores tend to be small; and (ii) the inverse is not true (i.e., not all small, fossorial carnivores are group-living). Understanding how these factors relate to group-living requires deeper enquiry into carnivoran fossoriality (L. to dig) and associated life-history traits.
In this study we examine two over-arching predictions: (i) that fossoriality will associate with carnivoran sociality; and (ii) that this association will be most evident in those carnivores with extended use of subterranean dens. Our primary set of questions thus pertain to life-history traits associated with fossoriality; particularly how morphological and energetic traits influence both digging ability and/or reliance upon the subterranean ecotope. For example, burrow size, and the cost of burrowing scale allometrically, depending on substrate cohesion (McNab, 1979; Vleck, 1981; White, 2005), suggesting the action of body size constraints on carnivoran fossoriality. Furthermore, although interlinked with body-size, there is support for an evolutionary relationship between longevity and fossoriality across a wide range of taxa (Healy et al., 2014; Williams and Shattuck, 2015), a factor of potential influence in the Carnivora. In addition, carnivoran young are born along a continuum of altriciality to precociality (Derrickson, 1992), altriciality requiring a secure environment for rearing neonates (Case, 1978a,b; Wolff, 1997). Although natal dens can be as simple as a hollow log (e.g., Carter et al., 2012), increased investment in natal den construction can enhance conditions of warmth and security for dependent, altricial young (Kinlaw, 1999; McClellan et al., 2008). This indicates that the extent of neonate altriciality, and parental investment are likely to influence fossorial tendancies. As an extension of this, the extended use of pre-excavated natal dens into adulthood can influence use of the subterranean ecotope, and make a substantial contribution to group formation (Lindström, 1986; Koenig et al., 1992; Kokko and Ekman, 2002). Furthermore, in line with predictions of the Expansible Burrow Hypothesis (see below), those carnivores that experience a substantial predation threat into adulthood will tend to be more fossorial (Alexander et al., 1991; Ebensperger and Blumstein, 2006). There is also evidence for a link between climate and the extent of fossoriality (Nevo, 1999). While endothermy allows carnivores to exploit cold regions (Grigg et al., 2004), fossoriality can provide a means of alleviating untolerable temperature thresholds in colder environments (e.g., Fløjgaard et al., 2009).
These factors are likely to interact with species' evolutionary trajectories however, where carnivoran fossoriality evolved around the Eocene/Oligocene boundary (ca. 35 Mabp: Andersson, 2004) for the excavation, or lithe pursuit, of burrowing prey (Martin, 1989; Andersson, 2004). The most fossorial carnivores tend to exhibit morphological adaptations evolved to dislodge substrate (Hildebrand, 1985; Andersson, 2004); species we define here as primary excavators (Kinlaw, 1999). Notably, these fossorial exaptations (“pre-adaptations,” sensu Gould and Vrba, 1982) permitted subsequent selection for further intrinsic (reproductive/physiological) benefits (Hypothesis of Phylogenetic Constraints, HPC: Burda et al., 2000; Šumbera et al., 2007).
The involement of life-history traits linked to fossoriality has received little attention in explaining carnivore social systems. This is especially apparent in contrast to the substantial support for a link between fossoriality and the evolution of rodent societies (e.g., hystricognath rodents: Ebensperger and Blumstein, 2006; Sobrero et al., 2014, and various marmot species: Barash, 1989; Hoogland, 1995). Smorkatcheva and Lukhtanov (2014) draw attention to three hypotheses, which resonate with pre-established models of carnivoran sociality: (i) the Territorial Defense Hypothesis (TDH: Nevo, 1979) proposes that the low productivity of the subterranean ecotope leads to strong resource competition, militating for solitary social systems. Similarly, the prey of small predatory carnivores tend to be localized and indivisible; conditions associated with competition (Macdonald et al., 2004a) and territorial defense (Waser, 1981); (ii) the Aridity Food Distribution Hypothesis (AFDH: reviewed in Jarvis et al., 1994) highlights patchy and unpredictable resources as a driver of sociality in subterranean mole-rats (family Bathyergidae). This is consistent with the Resource Dispersion Hypothesis (RDH: Macdonald, 1983; reviewed in Macdonald and Johnson, 2015), which proposes that groups will arise independent of cooperative benefits where the dispersion, or renewal rate, of resources result in a territory that can viably accommodate additional individuals; and (iii) the Expansible Burrow Hypothesis (EBH: Alexander et al., 1991) proposes that predator-free subterranean refuges militate for philopatry, cooperation, and thus sociality. Crucially, while small rodents are vulnerable to predation throughout their lives, for large(r), predatory carnivores, this is only a substantial (or greater) risk for their altricial young; hence the protective benefits of a secure natal den for carnivore neonates. Potentially, as with the EBH, some interaction between natal den use and philopatry may also explain components of carnivore sociality (e.g., Greenwood, 1980; Lindström, 1986; Blackwell and Bacon, 1993).
We also evaluate the role fossoriality may play in relation to promoting group formation from a socio-ecological perspective. This leads to our subsequent set of questions on behavioral, developmental, and trophic traits, relating to why some species can remain philopatric as they mature, leading to the formation of adult groups, vs. those that disperse into solitary territories. We propose that group formation in non-co-operatively hunting carnivores will be more evident among omnivorous/insectivorous species, in a suitable RDH-scape, in those species that extend the use of fossorial natal dens into adulthood, which serve as a “central-place” facilitating societal congregation. By way of “exceptions that prove the rule” this framework yields two further postulates. First, that predatory fossorial carnivores, for which the dispersion of their food supply is not suitable, will not form stable adult groups; and second, that omnivorous/insectivorous species that do not use subterranean dens, will tend not to form stable adult groups.
Shifting vulnerable life-cycle stages below ground permits a degree of independence from variable external conditions during critical periods (Kinlaw, 1999; Ebensperger and Blumstein, 2006). This can benefit longevity (Healy et al., 2014; Williams and Shattuck, 2015), energy budgets (Noonan et al., 2014, 2015), and facilitate extended periods of hibernation (Davenport, 1992), aiding the maximization of fitness (Nevo, 1999). Depending on the relative importance of burrow construction, often substantial energetic investments are made in den construction/maintenance (Vleck, 1981). Furthermore, burrows are important for secondary heterospecific competitors, which comandeer burrows (referred to as “kleptoclaustromy,”; e.g., Mills, 1982; Kaneko et al., 1998; Kowalczyk et al., 2008). As such, pre-excavated/actively maintained burrows are valuable resources, often inherited by offspring (sensu Lindström, 1986).
This leads us to posit whether fossorial dens support a “benefit-driven” basis for philopatry, as an extension of the EBH and the benefits of philopatry hypothesis (Brown and Brown, 1984; Pen and Weissing, 2000). Models of philopatry are typically based on cost-driven criteria, such as the greater mortality risk for dispersing individuals (Lucas et al., 1994), competition for breeding opportunities (Kokko and Lundberg, 2001), and habitat saturation (Emlen, 1982)—i.e., the Ecological Constraints Hypothesis (ECH: Pen and Weissing, 2000). In contrast, we examine whether dens promote inclusive fitness (Herre and Wcislo, 2011), territorial inheritance (Zack and Stutchbury, 1992), and cooperative breeding (Koenig et al., 1992) by acting as a “safe haven” (sensu Kokko and Ekman, 2002). Conditions where there are strong ecological constraints on dispersal from natal territories, limiting independent breeding, favor the perpetuation of natal groups to form persistent (sub-)adult groups (Emlen, 1982; Macdonald and Carr, 1989; Creel and Macdonald, 1995; Waser, 1996; Isbell, 2004).
Nevertheless, in many predatory, fossorial carnivores, prey availability becomes a limiting factor as litters develop and begin to forage independently (von Schantz, 1984; Clutton-Brock and Lukas, 2012). Thus, while maternal-offspring groups may persist temporarily (e.g., Dahle and Swenson, 2003; Kaneko et al., 2014), these groups often dissolve as offspring mature. For omnivorous and/or insectivorous species, however, the dispersion of trophic resources is often such that they are not monopolized by primary territory holders and, provided secondary individuals can tolerate inconsistent food supply (Newman et al., 2011; Macdonald and Johnson, 2015), their nutritional needs may still be met as they mature—leading to persistent adult groups (Macdonald, 1983).
We test these sets of questions linking life-history, socio-ecological, and evolutionary components of fossoriality to the propensity for group-living, by compiling a database of key ontological, ecological, and trophic variables across the Carnivora. We then apply Bayesian modeling to conduct a comparative analysis.
Materials and Methods
We applied our analyses to the 281 extant species of Carnivora listed by Wilson et al. (2009); the 36 pinnipeds were excluded a priori due to their predominantly aquatic life-histories. Additionally, no, or too few, data were available for 100 less well-known species, where we could access only anecdotal records, or derive metrics from museum specimens. The resultant database thus comprized a mix of complete and partial data for 145 species (Table S1). Two families (Nandiniidae, and Prionodontidae) were excluded totally from our database because too few data were available for extant species—consequently analyses were conducted across 11 families (Ailuridae, Canidae, Eupleridae, Felidae, Herpestidae, Hyaenidae, Mephitidae, Mustelidae, Procyonidae, Ursidae, Viverridae). Reference sources for these data are listed in Table S1, and detailed in Appendix 1 (Supplementary Material).
We define three response variables: group-living propensity, fossorial propensity and burrowing class.
Group-living Propensity
Due to intra-specific variation in the level of social integration, we rejected a Boolean, solitary/group-living classification, instead classifying group-living typologies according to Johnson et al. (2000) as: (i) solitary: species with predominantly solitary life-histories; (ii) pair-living: species with predominantly pair-living life-histories; (iii) facultative groups: species with both solitary and group-living life-histories, variable across populations; and (iv) group-living: species exhibiting predominantly obligate groups.
Fossorial Propensity
In accord with Nevo (1999), we differentiate here between truely “subterranean” species, which spend the majority of their lives below ground, only emerging occassionally, and “fossorial” species, which use the underground space for distinct activities (e.g., shelter or reproduction), but forage above ground. We therefore catagorize fossorial activities into the function of burrows as a:
(1) natal den: during parturition and the rearing of young;
(2) weather refugia: during hibernation and/or to avoid inclement weather;
(3) refuge from predation: for predator avoidance;
(4) food storage facility: used to store/cache food;
(5) residence: resting and/or sleeping site.
Several species utilize dens according to one or more of the functions listed above, on an opportunistic basis, as an alternative to epigean options (e.g., hollow logs, rock crevices, man-made structures, etc.,). We therefore quantified subterranean den use for each of the categories listed above as: (0) little or none; (0.5) facultative; (1) predominant.
The summation of these five uses yielded a measure of fossorial propensity, ranging on a scale from 0 to 5.
Burrowing Class
To account for the energetic investment each species makes in its burrow, we classified burrowers according to Kinlaw (1999) as:
(1) primary excavators: strong burrowers, for which digging plays a major role in their life-history;
(2) secondary modifiers: species that inhabit and modify burrows dug by primary excavators;
(3) tertiary occupants: species that play no role in digging burrows, but take advantage of burrows dug by other species.
In certain instances, species overlap these burrowing categories (i.e., able to dig their own burrow, but also making use of existing burrows); these were treated as intermediate between categories. Consequently, we quantified burrowing class as: (0) no burrow use; (1) tertiary occupants; (2) secondary modifier; and (3) primary burrowers; with intermediate species categorized as the mean of their respective burrowing classes (i.e., 2.5 for primay/secondary burrowers, and 1.5 for secondary/tertiary). This yielded a measure of burrowing class on a scale from 0 to 3.
Predictive Traits; Life-History, Socio-Ecology, and Phylogeny
We explored a diverse initial range of morphometric, ontological, and ecological traits inferred to influence fossorial propensity and burrowing class (with units in parentheses): (1) length: mean adult head-body length, excluding tail (mm ± 0.1 mm); (2) height: mean adult shoulder height (mm ± 0.1 mm); (3) female mass: mean mass of adult females (kg ± 100 g); (4) male mass: mean mass of adult males (kg ± 100 g); (5) adult mass: mean combined mass of adult males and females (kg ± 100 g); (6) age eyes open: mean duration from birth, until the neonates' eyes open (days); (7) duration of lactation: mean length of time, from birth, until the neonate becomes nutritionally independent of lactation (days); (8) age of independence: mean duration, from birth, until the neonates typically disperse from the natal territory or, in philopatric group-living species, become independent of parental food provisioning (days); (9) gestation length: mean length of time between implantation and parturition (days); (10) litter size: mean number of offspring produced per parturition; (11) age of sexual maturity: mean age at which offspring becomes physiologically capable of conception, averaged across sexes (months); (12) absolute longevity: age of the oldest living individual recorded in the wild; longevity records of individuals living in captivity were disregarded (months); (13) home range: mean combined home range of adult males and females across their geographic range (km2); (14) predators: number of known species that predate the adult life-stage; (15) latitude of biogeographical distribution: the northern-most distribution of the species (degrees north or south of the equator; source IUCN Red List species database) as a proxy for surface temperature, and seasonality therein (Fricke and O'Neil, 1999; although limited by local variation in altitude and continentality: Driscoll and Fong, 1992). Data and distributions concerning expatric, introduced populations were not included beyond their native range. Furthermore, as a categorical predictor we included: (16) diet: a species' main food source, defined as constituting at least 60% of the diet (Gittleman, 1985); classified as: carnivorous, insectivorous, omnivorous, frugivorous/herbivorous) as a proxy for trophic RDH compatibility (see Macdonald and Johnson, 2015).
Many of the traits we initially considered proved inter-correlated. To avoid the ineffectual inclusion of variables in our models, we applied a principal component analysis (PCA) across our continuous trait data to generate independent variables. Two significant correlates of adult mass (female mass; and male mass; Pearson's r > 0.98, corresponding to a p < 0.001), were not included in the PCA. Furthermore, height, and age of independence were excluded from the PCA, due to too few data. This procedure resulted in the retention of 3 principal components (PC) as predictive variables (determined using the R package nFactors; Raiche and Magis, 2010), which cumulatively summarized 76.1% of the variance in these ontological/ecological data (Table 1). Factor loadings indicated that body size, and its correlates (home range; longevity; and age of sexual maturity) were the most influential parameters along the axis of PC 1. PC 2 was determined primarily by measures of altriciality, and northernmost latitude, and PC 3 by predation threat. For clarity, in subsequent analyses these components are refered to by descriptive names based on factor loadings (i.e., body mass PC, latitude/altriciality PC, and predation threat PC respectively).
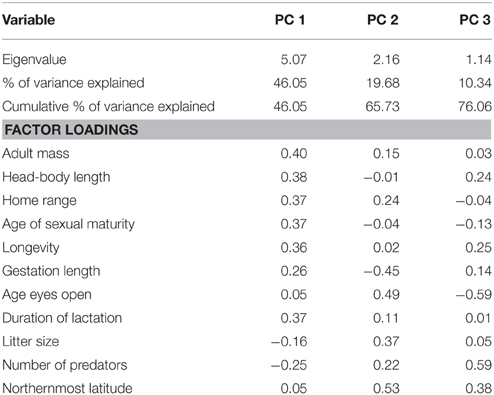
Table 1. Summary of the first three components of a principal components analysis performed over carnivoran ontological/ecological trait data.
Statistical Analyses
All statistical analyses were conducted in the R environment (version 3.0.3; R Core Team, 2014). Due to phylogenetic inertia (Hansen and Orzack, 2005), closely related species may exhibit similiarities in life-history traits from common descent rather than independent evolution, requiring controlled comparisons (Harvey and Pagel, 1991). Accordingly, we did not treat species data records as independent, but rather corrected for this inertia by fitting models using Bayesian phylogenetic mixed models using the R package MCMCglmm (Hadfield, 2010). By including the phylogenetic relationships among species as a random variable, this approach incorporates phylogenetic information into species data, allowing independent evolutionary events to be identified. Phylogenetic relationships were taken from a recent consensus phylogeny of the Carnivora (Agnarsson et al., 2010). For the Canidae, however, we took relationships from Lindblad-Toh et al. (2005), chosen for its completeness. Phylogenetic data were not available for four species in our database (Caracal caracal; Leptailurus serval; Martes martes; and Vulpes velox), consequently we excluded these from our analyses.
To test our primary questions concerning the co-evolution of fossorial propensity, burrowing class, and ontological/ecological traits, the following two models were fitted:
(i) burrowing class = f (body mass PC + latitude/altriciality PC + predation threat PC + diet).
(ii) fossorial propensity = f (body mass PC + latitude/altriciality PC + predation threat PC + diet).
To test our subsequent questions concerning the socio-ecological association between subterranean life-histories, resource dispersion, and sociality, the following model was fitted:
(i) social class = f (fossorial propensity + burrowing class + diet).
Following Hadfield (2010), we used uninformative, inverse-gamma prior distributions. The number of iterations, thinning interval and the burn-in period were determined using diagnostic tests in the R package coda (Plummer et al., 2006). Convergence between model chains was verified using the Geweke diagnostic (Geweke, 1992).
Results
Of the 145 carnivore species for which we were able to source specific trait data, 113 made at least some use of subterranean space, and 85 of these for more than a singular purpose (Figure 1). Underground burrows were used, in descending order, as natal dens (74.5%, n = 108), residences (57.2%, n = 83), weather refugia (19.3%, n = 28), refuges from predation (19.3%, n = 28), and/or for food storage (10.6%, n = 12).
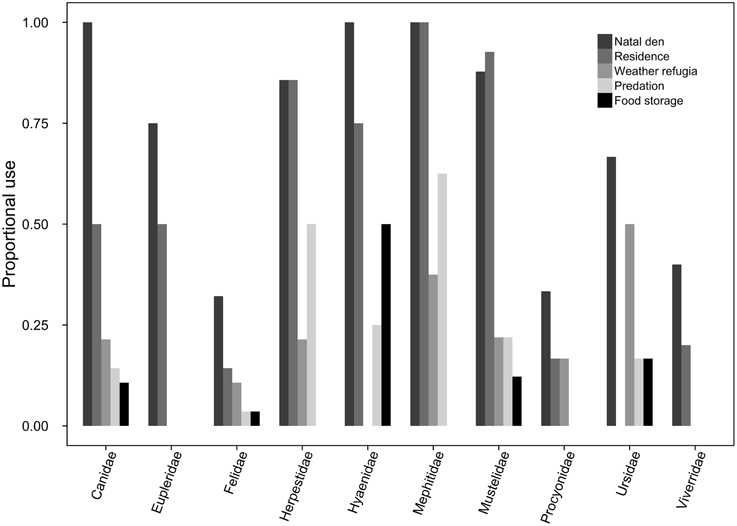
Figure 1. For each carnivore family, the proportion of species exhibiting at least facultative use of subterranean dens for each of the behavioral use categories (i.e., natal dens, residences, weather refugia, predation refugia, and food storage).
Life-history, Socio-ecology, and Fossoriality
Bayesian modeling evidenced that a strong phylogenetic signal exists in carnivoran burrowing class (Table 2), and fossorial propensity (Table 3). Fossoriality was more prevalent in the Caniformia (Figure 2) than in the Feliformia (Figure 3); mephitids, mustelids, and hyaenids being the most fossorial families. None of the felids, procyonids, or viverrids (for which we had data) proved to be primary excavators, and of these families, only two species of felids (Felis margarita; and Felis nigripes) were secondary modifiers, the rest being tertiary occupants. All other families included primary excavators, with the mephitids having the highest proportion (87.5%) of these.
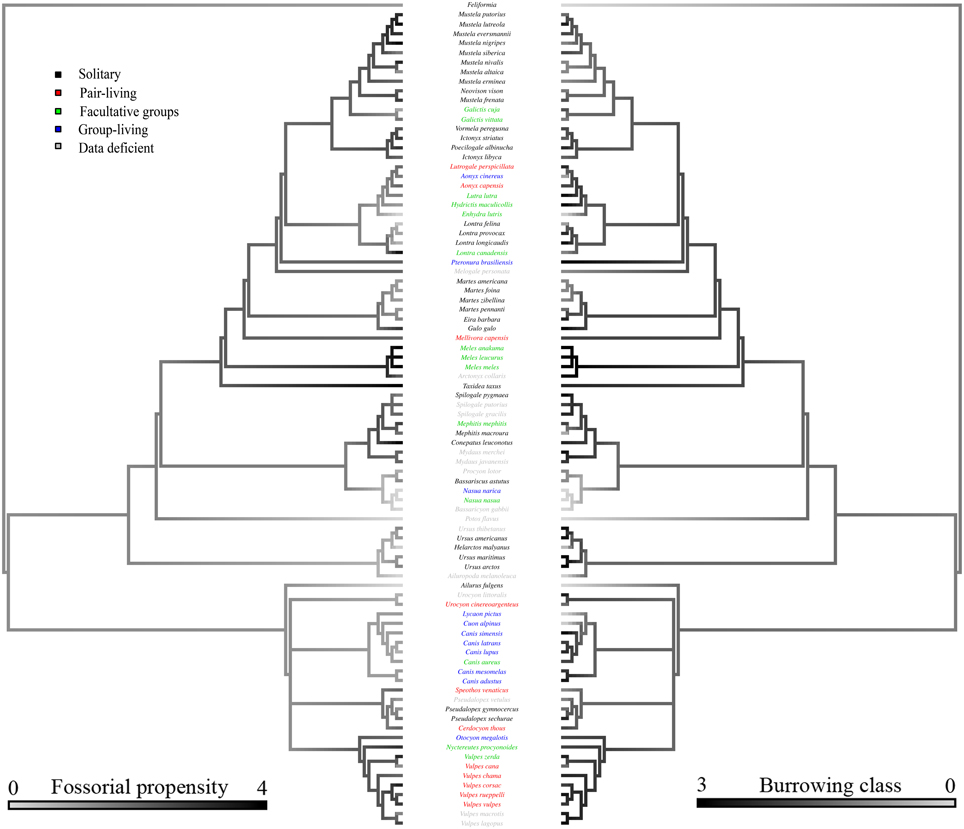
Figure 2. Consensus tree of the Caniformia based on phylogenetic relationships from Agnarsson et al. (2010). Relationships between the Canidae were taken from Lindblad-Toh et al. (2005). For clarity, the Feliformia are collapsed. Shading of the left hand branches depicts fossorial propensity scaled from wholly epigean, to wholly fossorial. Shading of the right hand branch depicts burrowing class scaled from no burrow use, to primary excavators. Coloration of species labels denotes group-living propensity, classified as: (black) solitary: species with predominantly solitary life-histories; (red) pair-living: predominantly pair-living species; (green) facultative groups: species with both solitary and group-living life-histories, variable across populations; and (blue) group-living: species exhibiting obligate groups. Data deficient species are listed in gray.
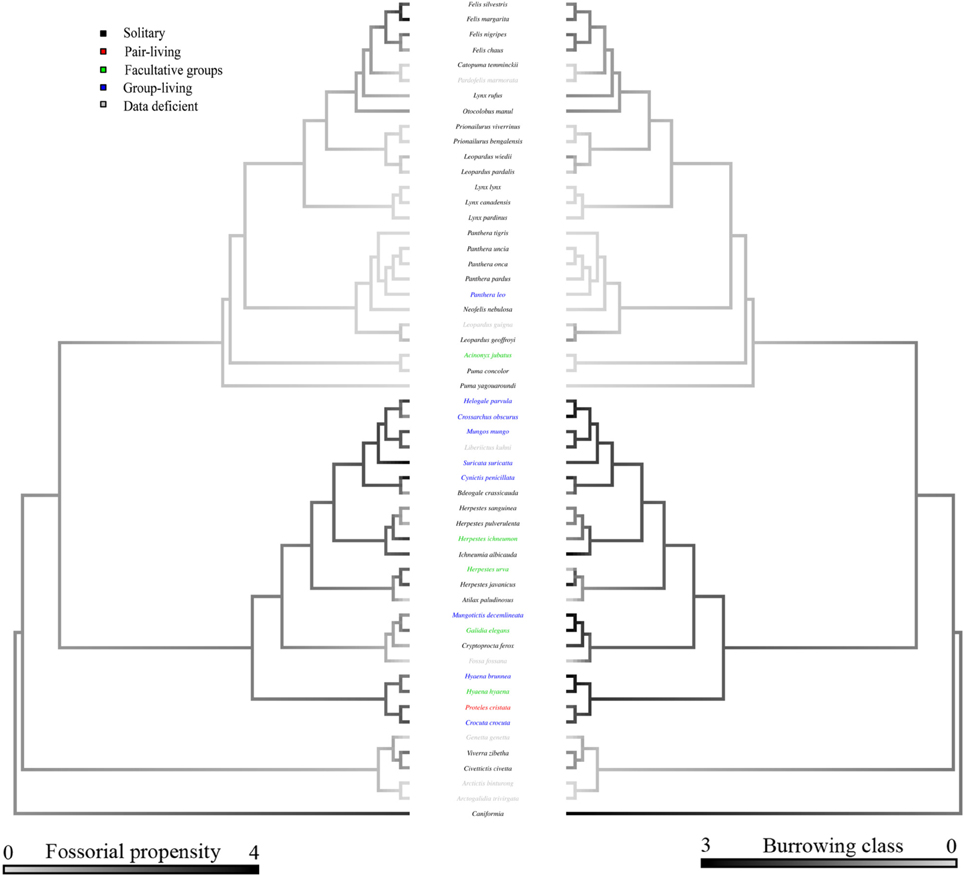
Figure 3. Consensus tree of the Feliformia based on phylogenetic relationships from Agnarsson et al. (2010). For clarity, the Caniformia are collapsed. Shading of the left hand branches depicts fossorial propensity scaled from wholly epigean, to wholly fossorial. Shading of the right hand branch depicts burrowing class scaled from no burrow use, to primary excavators. Coloration of species labels denotes group-living propensity (see Figure 2). Data deficient species are listed in gray.
We found support for an evolutionary relationship between fossorial life-histories and socio-ecological traits. Posterior coefficient estimates for the latitude/altriciality PC, and the herbivorous/frugivorous dietary class indicated that these factors contributed significantly to our model predictive of burrowing class. Measures of body size and predation threat proved relatively unimportant in this model.
There was also evidence for an evolutionary relationship between fossorial propensity and diet, as well as PCs defined by body mass and latitude/altriciality. Generally, smaller carnivore species, producing large(r) litters, of altricial young were more likely to be fossorial than larger carnivore species producing smaller litters of well developed young. Indeed, no fossorial, primary excavator had an adult mass that exceeded 15 kg. Furthermore, insectivorous carnivores were generally more fossorial than carnivorous or frugivorous/herbivorous species.
Fossoriality and Group-living
Modeling predictive of group-living evidenced that carnivores from different burrowing classes differed significantly in their propensity for group-living (Table 4). Primary excavators tended to form groups significantly more often than other burrowing classes; tertiary burrow users were least likely to form groups. There was also clear support for an evolutionary relationship between diet, fossoriality, and social class. Crucially, in tandem with burrowing class, diet proved highly significant, where carnivorous and frugivorous/herbivorous carnivores tended to form groups significantly less often than insectivorous or omnivorous carnivores.
Discussion
Of extant terrestrial mammals, 447 of 777 genera include species that engage in burrowing behavior (Kinlaw, 1999). These represent diverse mammalian species, from small rodents and soricomorphs (Nevo, 1999) to the largest extant burrowers, aardvarks (Orycteropus afer: Taylor and Skinner, 2003). Reflecting the extent of fossoriality observed across terrestrial mammals generally (Kinlaw, 1999), three quarters of carnivore species exhibited at least some level of reliance on the subterranean ecotope. Congruence between ecological/ontological traits and metrics of fossoriality in the Carnivora accorded with four broad themes: (1) there were phylogenetic, and morphological constraints on wholly fossorial life-histories; (2) fossoriality correlated positively with the extent of offspring altriciality, linked to the use of natal dens; (3) burrow use increased with latitude; and (4) insectivorous carnivores were more fossorial than predatory carnivores. Furthermore, congruent with the strong support for a link between fossoriality and sociality in rodent societies (Barash, 1989; Hoogland, 1995; Ebensperger and Blumstein, 2006; Sobrero et al., 2014), we evidenced a positive evolutionary relationship between the extent of fossoriality and the propensity for group-living. These fossorial traits have, functional, mechanistic, ontogenetic, and phylogenetic components (sensu Tinbergen, 1963), which, in turn, significantly influence species' socio-ecology.
Allometric Constraints
We found an association between fossorial propensity and the PC defined by body size, highlighting allometric constraints on fossoriality (McNab, 1979; Vleck, 1981). Indeed, no fossorial, primary excavator had a mean adult mass greater than 15 kg. Although forelimb supination (see below) can support large body sizes (e.g., 300+ kg ursids: Andersson, 2004), as weight increases, cursoriality becomes more economical (Kram and Taylor, 1990). Consequently, with increasing body size, the opposing effects of relaxed running costs vs. greater burrowing costs (McNab, 1979; Vleck, 1981) can act as significant drivers for, or against, fossorial life-histories.
Although carnivores larger than 15 kg are still able to burrow, smaller species made greater use of underground space. In addition to the energetic cost of burrowing scaling allometrically (sensu Vleck, 1981), burrow size also scales allometrically (White, 2005) and there is an upper limit on burrow size. Simply, even the most consolidated soil types cannot support the large and extensive excavations that would be needed to house, for example, a group of bears. Furthermore, although a wide variety of small (< 1.0 kg) subterranean species appear in the paleontological record (Nevo, 1999), large fossil burrows are rare (e.g., Voorhies and Toots, 1970). Indeed, fossil evidence suggests that larger carnivorans were restricted to the use of cave formations, rather than subterranean burrows, as denning sites (e.g., cave bears, Ursus spelaeus: (Stiner, 1999); and cave hyaenas, Crocuta crocuta spelaea: Diedrich and Žák, 2006).
Energetic Adaptations
Mammals exposed to cold winter conditions cope through two mechanisms; (i) low thermal conductance to minimize heat loss (e.g., thick winter fur and/or insulating fat layer: Shield, 1972; McNab, 1983); or (ii) by reducing their exposure to the cold, through the use of refugia (Noonan et al., 2014, 2015), and/or entering a torpid state therein (Harlow, 1981; Lyman, 2013). Thus, while endothermy allows carnivores to exploit cold regions, and higher latitudes (Grigg et al., 2004), fossoriality provides a means of mitigating temperature thresholds (Shelford, 1931; Pörtner, 2002), permitting the species to live in colder environments (Fløjgaard et al., 2009). Also, small carnivores often utilize the subnivean ecotope directly for hunting (e.g., American martens, Martes americana: (Raine, 1987); least weasels, Mustela nivalis: (Sheffield and King, 1994); black-footed ferrets, Mustela nigripes: (Hillman and Clark, 1980); European polecats, Mustela putorius: Heptner and Sludskii, 2002). As a consequence, we found that the PC defined by latitude was influential in predicting the importance of fossoriality to species across all sizes (irrespective of Bergmann's rule, sensu Blackburn et al., 1999). Smaller predatory carnivores, however, are less suited to hibernation (for example no Martes spp. hibernate whereas the larger Meles spp. do: Newman et al., 2011), and tend not to form groups at high latitude.
High latitudes, and seasonality therein, also place particular ecological constraints (ECH: Pen and Weissing, 2000) on dispersing juveniles, leading to delayed dispersal (Ferreras et al., 2004), due to greater seasonal variation in food supply, shorter productive seasons and increased energetic requirements (Blank, 1992; Noonan et al., 2014). Futhermore, in those species large enough to undergo extended hibernation, group-living may be promoted by the thermoregulatory benefits of huddling in groups within subterranean burrows (e.g., Roper et al., 2001; Hwang et al., 2007; Kitao et al., 2009). These factors are likely influential in the integration of subsequent generations into natal groups (Dahle and Swenson, 2003; Ferreras et al., 2004; Kaneko et al., 2014).
Ontological Adaptations
Fossoriality and burrowing class were related to measures of altriciality. Among the Carnivora, while offspring are born along a continuum of altriciality to precociality (Derrickson, 1992), precocial young are incompatible with large litter sizes (reviewed in Martin and MacLarnon, 1985). This is especially so where pregnant females need to maintain hunting agility (Case, 1978b; Woodroffe and Ginsberg, 1997). Thus, altriciality is likely ancestoral (Hopson, 1973; Case, 1978b). Altriciality however, requires a secure environment for rearing neonates (Case, 1978a,b; Wolff, 1997), and although natal dens can be as simple as a hollow log (e.g., Carter et al., 2012), subterranean burrows are more thermally stable (e.g., Buffenstein, 1984; Nevo, 1999; Moore and Roper, 2003; Kaneko et al., 2010). Thus, bespoke natal dens extend womb-like conditions of warmth and security, while altricial offspring are provisioned with milk (Kinlaw, 1999; McClellan et al., 2008). A similar scenario can be seen in the Leporidae where cursorial, precocious hares (Lepus spp.) are capable of following their mother soon after birth, while burowing, altricial rabbits (Oryctolagus spp.) are born blind and hairless, remaining underground until they are 4 weeks old (Macdonald, 2006).
We found that species utilizing subterranean natal dens tend to form groups roughly 2.5 times more often than those that do not. Natal dens can effectively support philopatric and social behavior (benefits of philopatry hypothesis: Brown and Brown, 1984)—mirroring correlations found in birds where extended parental care leads to delayed offspring dispersal (Brown and Brown, 1984; Ekman and Rosander, 1992; Ekman et al., 1994). Even in the absence of any other benefits of sociality, if local environmental carrying capacity permits, then the continuing philopatric use of natal dens (Isbell, 2004), resulting from delayed dispersal (Kokko and Ekman, 2002), may impose communal aggregation upon individuals (an extention of the EBH).
It is important to note, however, that in group-living species where philopatry accrues fitness benefits through enhanced survival (Kokko and Ekman, 2002), inclusive fitness (Herre and Wcislo, 2011) and cooperative breeding (Koenig et al., 1992), reproductive suppression of subordinates often occurs (Creel and Creel, 1991; Creel and Macdonald, 1995). Indeed, even for those non-cooperative breeders in which offspring always disperse, reproductive opportunities may be just as limited as they are for offspring that remain philopatric (Brown and Brown, 1984). Thus, albeit through different mechanisms, both strategies result in similar ecological constraints (ECH: Pen and Weissing, 2000).
Phylogenetic Exaptations
Paleo-ecological selection pressures favoring fossorial traits arose around the Eocene-Oligocene boundary, circa 35 Mabp (Nevo, 1999). During this transition, the earth underwent a shift to cooler (7–10°C over the Paleogene; Savin, 1977), and increasingly variable conditions (Katz et al., 2008), causing aridization and greater seasonality (Prothero and Berggren, 2014). As a result, forest ecosystems, abundant in the Eocene, gave way to Oligocene grasslands (Vicentini et al., 2008; Prothero and Berggren, 2014), impacting habitat availability and food chains. This drove selection for two adaptive herbivore strategies; (i) plains herbivores (Stebbins, 1981), which grew large and migratory in developing prairies and savannahs; and (ii) smaller rodent species, able to burrow for protection (Nevo, 1999). Prior to this transition, carnivore ancestors tended to be arboreal forest dwellers (Macdonald, 1992), however, in a typical “Red Queen” response (Van Valen, 1973), carnivorans invading this new grassland ecotope underwent selection along four formats: (i) cursorial pursuit predators able to chase fleet-footed ungulates, exemplified in the digitigrade canids (Martin, 1989; Andersson, 2004); (ii) “stalk and ambush” predators, with supinated limbs adapted for grappling, common among extant feliformia (Andersson, 2004); and strategists capable of pursuing prey underground, giving rise to ecomorphs undergoing selection for either; (iii) digging power, represented today by the bear-badger type (with selection for specific forelimb anatomy for digging; see Andersson, 2004; Samuels et al., 2013); and (iv) slender ecomorphs evolved to slip into burrows to capture prey, represented today by the polecat-mongoose type (Martin, 1989).
Ebensperger and Blumstein (2006) reported that evolutionary trajectories constrained fossoriality in the Rodentia significantly. Similarily, we found that use of the subterranean ecotope was not consistent across all carnivore families. Phylogenetic constraints were apparent, limiting which carnivores are able to dig; inertia consequent of ancestral specialization for cursoriality, scansoriality, and for grasping claws. For example, felids, procyonids, and viverids proved the least fossorial carnivore lineages. Indeed, ichnofossil evidence of burrowing behavior (as distinct from den use, per se) also reflects that ancestral felids, procyonids, and viverids evolved along trajectories incompatible with digging (Macdonald, 1992; Andersson, 2004). The felids are the only wholly carnivorous Carnivora family (Macdonald, 1992; Sicuro and Oliviera, 2011), and possess hook-shaped, retractable claws, adapted for hunting prey (Bryant et al., 1996; Kitchener et al., 2010), which would be damaged by digging. Burrowing species, in contrast, typically have flattened claws adapted for dislodging soil (Hildebrand, 1985). Several epigean viverid species also have retractable claws (Bryant et al., 1996). Similarly, the substantially arboreal Procyonidae have forelimbs adapted to climbing (McClearn, 1992). Coatis (Nasua spp.), being predominantely terrestrial but with arboreal dens, provide an interesting contrast to other procyonids. While they dig holes proficiently to capture invertebrate prey, they do not excavate subterranean burrows, and are the least agile climbers in this family (McClearn, 1992).
Further in line with our findings here, a clear phylogenetic signal for digging ability is evident in the forelimb morphology of carnivores, where shared ancestry significantly constrains kinesiology (Andersson, 2004; Samuels et al., 2013). Forelimb supination is crucial for both scansorial and fossorial carnivores, but is maladaptive for cursorial pursuit predators (Andersson, 2004). Unlike scansoriality however, scratch digging requires elongated humeri and ulnae to provide stable elbow joints (Van Valkenburgh, 1987; Andersson, 2004; Samuels et al., 2013). Due to these phylogenetic constraints, those species that use burrows but are unable to excavate them themselves, rely on kleptoclaustromy, and so burrowing species are “keystone” (Mills and Doak, 1993) to the subterranean ecotope (e.g., Whittington-Jones and Bernard, 2011).
Diet, Trophic Resource Dispersion, and Sociality
Carnivores that evolved along phylogenetic trajectories adaptive for fossorial traits are often those that also exhibit the tendancy to form groups. Here diet proves important: among the 145 species examined, we found a 27.8% overlap (33 species) between the 62 species showing a significant extent of fossoriality and the 62 with omnivorous/insectivorous diets. Of these 33 species, only 3 were solitary; white-tailed mongooses (Ichneumia albicauda); American hog-nosed skunks, (Conepatus leuconotus); and Sechura foxes, (Pseudalopex sechurae).
This substantial interaction between fossoriality and omnivorous/insectivorous diets proves insightful. Although 75% of carnivores utilize fossorial natal dens, species consuming a carnivorous diet tend to disperse as they mature because the resources available in their natal territory cannot support the requirements of multiple adults (von Schantz, 1984). For species that consume a diet where food resources have a dispersion able to support adult groups (typified by omnivory/insectivory; sensu Macdonald, 1983), dispersal may be delayed or obviated. When these species are small enough to cohabit, fossorial dens act as foci leading to the formation of philopatric groups. By increasing the variety of trophic resources available, omnivory (to include insectivory) effectively reduces competition for resources (Gittleman, 1986). Furthermore, the dispersion and renewal characteristics of these alternative food types preclude sufficient resources being monopolized by a primary individual to prevent secondary conspecifics being tolerated in the same range (Macdonald et al., 2004b; Macdonald and Johnson, 2015), leading to range sharing. This is a flexible process; many omnivores form temporary “fission fusion societies” under appropriate but temporary RDH conditions (e.g., raccoons, Procyon lotor; red foxes, Vulpes vulpes; arctic foxes, Vulpes lagopus; Blandford's foxes, Vulpes cana; and kinkajous, Potos flavus; reviewed in Macdonald and Johnson, 2015).
There are nevertheless likely limiations to a fossorial group formation process among the Carnivora. Omnivores/insectivores too big to cohabit will still be driven to disperse, although they may congregate temporarily in rich resources patches, (e.g., ursids at salmon runs: Reimchen, 2000). Furthermore, secondary conspecifics need to have a physiology capable of tolerating secondary food security (Newman et al., 2011; Macdonald and Johnson, 2015). Small, subnivean and/or agile scansorial predators for example, cannot carry sufficient body fat without compromising required hunting athleticism (King, 1989; Newman et al., 2011). Chinese ferret badgers (Melogale moschata) appear to be at the lower body-weight extreme for being able to form burrow-dwelling groups, benefitting from (sub-)tropical levels of productivity (Zhang et al., 2010). Consequently, fossoriality, even in a suitable trophic resource-scape, is unlikely to leverage group-living if these critera are not met.
Conclusions
We evidence that the extent of fossoriality in the Carnivora is an important driver of group-living behavior, resonating with research in the Rodentia (e.g., Ebensperger and Blumstein, 2006; Smorkatcheva and Lukhtanov, 2014; Sobrero et al., 2014). This provides consilient support to an ecological basis to non-cooperative societies (sensu Macdonald, 1983). According to the TDH (Nevo, 1979), under conditions leading to strong resource competition, fossoriality leads to solitary social systems (sensu Gittleman, 1989). Although the role of TDH in the evolution of rodent societies was rejected by Smorkatcheva and Lukhtanov (2014), we observed that extant (semi-) fossorial predatory carnivores that compete for indivisible, small vertebrate prey, which do not simultaneously satisfy RDH sociality criteria (see Macdonald and Johnson, 2015), tend not to be gregarious (e.g., American badgers, Taxidea taxus: Long, 1973; least weasels: Sheffield and King, 1994; black-footed ferrets: Hillman and Clark, 1980; European polecats: Heptner and Sludskii, 2002; wolverines, Gulo gulo: Pasitschniak-Arts and Larivière, 1995; and sand cats, Felis margarita: Sunquist and Sunquist, 2002). Similarly, albeit satisfying dietary RDH criteria, certain epigean carnivores (e.g., tayras, Eira barbara: Presley, 2000; ringtails, Bassariscus astutus: Harrison, 2012; ursids, Ursus spp.: Pasitschniak-Arts, 1993; Larivière, 2001; and African civets, Civettictis civetta: Ray, 1995) proved less gregarious than otherwise ecologically similar fossorial species; that is, fossoriality leverages group formation under marginal circumstances.
Subterranean living is not without its challenges, including hypoxia/hypercapnia, permanent darkness, and conditions of high pathogen infectivity, which can lead to the spread of disease and parasites (Nevo, 1999). Indeed den sharing has been implicated in the spread of Mycobacterium bovis among several social burrowers (e.g., yellow-bellied marmots, Marmota flaviventris: Van Vuren, 1996; brushtail possums, Trichosurus vulpecula: Corner et al., 2003; European badgers, Meles meles: Rogers et al., 2003; wombats, Vombatus ursinus: Skerratt et al., 2004). For those carnivores where the benefits outweigh the costs, we evidence that the extent of fossoriality accords with the TDH, the EBH, and RDH criteria. In tandem with the benefits of philopatry (Brown and Brown, 1984), this can act as a significant precursor for group-living. This also explains why certain carnivores that satisfy the dietary critera of the RDH, but are not strongly fossorial, do not form (semi-) permanent groups. Cohabitation provides the initial step toward group-living. If conditions arise where omnivorous species, living in an environment with suitable resource dispersion (sensu Macdonald, 1983), continue to remain associated with their fossorial natal dens, then a propitious scene is set for the formation of permanent social groups.
Conflict of Interest Statement
The authors declare that the research was conducted in the absence of any commercial or financial relationships that could be construed as a potential conflict of interest.
Acknowledgments
The Peoples Trust for Endangered Species generously supported much of this core study. MN was supported by the Rhodes Trust and an NSERC postgraduate scholarship. In addition, two anonymous reviewers and the Associate Editor provided valuable feedback on an early version of the manuscript.
Supplementary Material
The Supplementary Material for this article can be found online at: http://journal.frontiersin.org/article/10.3389/fevo.2015.00116
References
Agnarsson, I., Kuntner, M., and May-Collado, L. J. (2010). Dogs, cats, and kin: a molecular species-level phylogeny of Carnivora. Mol. Phylogenet. Evol. 54, 726–745. doi: 10.1016/j.ympev.2009.10.033
Alexander, R. D. (1974). The evolution of social behavior. Annu. Rev. Ecol. Syst. 5, 325–383. doi: 10.1146/annurev.es.05.110174.001545
Alexander, R. D., Noonan, K. M., and Crespi, B. J. (1991). “The evolution of eusociality,” in The Biology of the Naked Mole-Rat, eds P. W. Sherman, J. U. Jarvis, and R. D. Alexander (Princeton, NJ: Princeton University Press), 3–44.
Andersson, K. I. (2004). Elbow−joint morphology as a guide to forearm function and foraging behaviour in mammalian carnivores. Zool. J. Linn. Soc. 142, 91–104. doi: 10.1111/j.1096-3642.2004.00129.x
Bacon, P. J., Ball, F., and Blackwell, P. (1991). A model for territory and group formation in a heterogeneous habitat. J. Theor. Biol. 148, 445–468. doi: 10.1016/S0022-5193(05)80231-0
Barash, D. P. (1989). Marmots. Social Behavior and Ecology. Stanford, CA: Stanford University Press.
Blackburn, T. M., Gaston, K. J., and Loder, N. (1999). Geographic gradients in body size: a clarification of Bergmann's rule. Divers. Distrib. 5, 165–174. doi: 10.1046/j.1472-4642.1999.00046.x
Blackwell, P., and Bacon, P. J. (1993). A critique of the territory inheritance hypothesis. Anim. Behav. 46, 821–823. doi: 10.1006/anbe.1993.1262
Blank, J. L. (1992). “Phenotypic variation in physiological response to seasonal environments,” in Mammalian Energetics: Interdisciplinary Views of Metabolism and Reproduction, eds T. E. Tomasi and T. H. Horton (Ithaca, NY: Cornell University Press), 186–212.
Brown, J. L., and Brown, E. R. (1984). Parental facilitation: parent-offspring relations in communally breeding birds. Behav. Ecol. Sociobiol. 14, 203–209. doi: 10.1007/BF00299620
Bryant, H. N., Russell, A. P., Laroiya, R., and Powell, G. L. (1996). Claw retraction and protraction in the Carnivora: skeletal microvariation in the phalanges of the Felidae. J. Morphol. 229, 289–308.
Buffenstein, R. (1984). The importance of microhabitat in thermoregulation and thermal conductance in two namib rodents—a crevice dweller, Aethomys namaquensis, and a burrow dweller, Gerbillurus paeba. J. Therm. Biol. 9, 235–241. doi: 10.1016/0306-4565(84)90002-0
Burda, H., Honeycutt, R. L., Begall, S., Locker-Grütjen, O., and Scharff, A. (2000). Are naked and common mole-rats eusocial and if so, why? Behav. Ecol. Sociobiol. 47, 293–303. doi: 10.1007/s002650050669
Carter, A., Luck, G. W., and Wilson, B. P. (2012). Ecology of the red fox (Vulpes vulpes) in an agricultural landscape. 1. Den-site selection. Aust. Mammal. 34, 145–154. doi: 10.1071/AM11038
Case, T. J. (1978a). Endothermy and parental care in the terrestrial vertebrates. Am. Nat. 112, 861–874.
Case, T. J. (1978b). On the evolution and adaptive significance of postnatal growth rates in the terrestrial vertebrates. Q. Rev. Biol. 53, 243–282.
Clutton-Brock, T. H., and Lukas, D. (2012). The evolution of social philopatry and dispersal in female mammals. Mol. Ecol. 21, 472–492. doi: 10.1111/j.1365-294X.2011.05232.x
Corner, L. A. L., Pfeiffer, D. U., and Morris, R. S. (2003). Social-network analysis of Mycobacterium bovis transmission among captive brushtail possums (Trichosurus vulpecula). Prev. Vet. Med. 59, 147–167. doi: 10.1016/S0167-5877(03)00075-8
Creel, S., and Macdonald, D. W. (1995). Sociality, group size, and reproductive suppression among carnivores. Adv. Stud. Behav. 24, 203–257. doi: 10.1016/S0065-3454(08)60395-2
Creel, S. R., and Creel, N. M. (1991). Energetics, reproductive suppression and obligate communal breeding in carnivores. Behav. Ecol. Sociobiol. 28, 263–270. doi: 10.1007/BF00175099
Dahle, B., and Swenson, J. E. (2003). Factors influencing length of maternal care in brown bears (Ursus arctos) and its effect on offspring. Behav. Ecol. Sociobiol. 54, 352–358. doi: 10.1007/s00265-003-0638-8
Davenport, J. (ed.). (1992). “Sleep, torpor and hibernation,” in Animal Life at Low Temperatures (Dordrecht: Springer), 110–135.
Derrickson, E. M. (1992). Comparative reproductive strategies of altricial and precocial eutherian mammals. Funct. Ecol. 6, 57–65. doi: 10.2307/2389771
Diedrich, C. G., and Žák, K. (2006). Prey deposits and den sites of the Upper Pleistocene hyena Crocuta crocuta spelaea (Goldfuss, 1823) in horizontal and vertical caves of the Bohemian Karst (Czech Republic). Bull. Geosci. 81, 237–276. doi: 10.3140/bull.geosci.2006.04.237
Driscoll, D. M., and Fong, J. M. Y. (1992). Continentality: a basic climatic parameter re−examined. Int. J. Climatol. 12, 185–192. doi: 10.1002/joc.3370120207
Ebensperger, L. A., and Blumstein, D. T. (2006). Sociality in new world hystricognath rodents is linked to predators and burrow digging. Behav. Ecol. 17, 410–418. doi: 10.1093/beheco/arj048
Ekman, J., and Rosander, B. (1992). Survival enhancement through food sharing: a means for parental control of natal dispersal. Theor. Popul. Biol. 42, 117–129. doi: 10.1016/0040-5809(92)90008-H
Ekman, J., Sklepkovych, B., and Tegelstrom, H. (1994). Offspring retention in the Siberian jay (Perisoreus infaustus): the prolonged brood care hypothesis. Behav. Ecol. 5, 245–253. doi: 10.1093/beheco/5.3.245
Emlen, S. T. (1982). The evolution of helping. I. An ecological constraints model. Am. Nat. 119, 29–39. doi: 10.1086/283888
Ferreras, P., Delibes, M., Palomares, F., Fedriani, J. M., Calzada, J., and Revilla, E. (2004). Proximate and ultimate causes of dispersal in the Iberian lynx Lynx pardinus. Behav. Ecol. 15, 31–40. doi: 10.1093/beheco/arg097
Fløjgaard, C., Normand, S., Skov, F., and Svenning, J. C. (2009). Ice age distributions of European small mammals: insights from species distribution modelling. J. Biogeogr. 36, 1152–1163. doi: 10.1111/j.1365-2699.2009.02089.x
Fricke, H. C., and O'Neil, J. R. (1999). The correlation between 18O/16O ratios of meteoric water and surface temperature: its use in investigating terrestrial climate change over geologic time. Earth Planet. Sci. Lett. 170, 181–196. doi: 10.1016/S0012-821X(99)00105-3
Geweke, J. (1992). “Evaluating the accuracy of sampling-based approaches to the calculation of posterior moments,” in Bayesian Statistics 4: Proceedings of the Fourth Valencia International Meeting, eds J. M. Bernardo, J. Berger, A. P. Dawid, and A. F. M. Smith (Oxford: Oxford University Press), 169–193.
Gittleman, J. L. (1985). Carnivore body size: ecological and taxonomic correlates. Oecologia 67, 540–554. doi: 10.1007/BF00790026
Gittleman, J. L. (1986). Carnivore life history patterns: allometric, phylogenetic, and ecological associations. Am. Nat. 127, 744–771. doi: 10.1086/284523
Gittleman, J. L. (ed.). (1989). “Carnivore group living: comparative trends,” in Carnivore Behavior, Ecology, and Evolution (Boston, MA: Springer), 183–207.
Gould, S. J., and Vrba, E. S. (1982). Exaptation-a missing term in the science of form. Paleobiology 8, 4–15.
Greenwood, P. J. (1980). Mating systems, philopatry and dispersal in birds and mammals. Anim. Behav. 28, 1140–1162. doi: 10.1016/S0003-3472(80)80103-5
Grigg, G. C., Beard, L. A., and Augee, M. L. (2004). The evolution of endothermy and its diversity in mammals and birds. Physiol. Biochem. Zool. 77, 982–997. doi: 10.1086/425188
Hadfield, J. D. (2010). MCMC methods for multi-response generalized linear mixed models: the MCMCglmm R package. J. Stat. Softw. 33, 1–22. doi: 10.18637/jss.v033.i02
Hansen, T. F., and Orzack, S. H. (2005). Assessing current adaptation and phylogenetic inertia as explanations of trait evolution: the need for controlled comparisons. Evolution 59, 2063–2072. doi: 10.1554/05-088.1
Harlow, H. J. (1981). Torpor and other physiological adaptations of the badger (Taxidea taxus) to cold environments. Physiol. Zool. 54, 267–275.
Harrison, R. L. (2012). Ringtail (Bassariscus astutus) ecology and behavior in central New Mexico, USA. West. N. Am. Nat. 72, 495–506. doi: 10.3398/064.072.0407
Harvey, P. H., and Pagel, M. D. (1991). The Comparative Method in Evolutionary Biology. Oxford: Oxford University Press.
Hatchwell, B. J., and Komdeur, J. (2000). Ecological constraints, life history traits and the evolution of cooperative breeding. Anim. Behav. 59, 1079–1086. doi: 10.1006/anbe.2000.1394
Healy, K., Guillerme, T., Finlay, S., Kane, A., Kelly, S. B. A., McClean, D., et al. (2014). Ecology and mode-of-life explain lifespan variation in birds and mammals. Proc. Biol. Sci. 281, 20140298–20140298. doi: 10.1098/rspb.2014.0298
Heptner, V. G., and Sludskii, A. A. (2002). Mammals of the Soviet Union. Vol. II, Part 1b, Carnivores (Mustelidae and Procyonidae). Washington, DC: Smithsonian Institution Libraries and National Science Foundation.
Herre, E. A., and Wcislo, W. T. (2011). In defence of inclusive fitness theory. Nature 471, E8–E9. doi: 10.1038/nature09835
Hildebrand, M. (1985). “Digging of quadrupeds,” in Functional Vertebrate Morphology, eds M. Hildebrand, D. M. Bramble, K. F. Liem, and D. B. Wake (Cambridge: Belknap Press of Harvard University Press), 89–109.
Hillman, C. N., and Clark, T. W. (1980). Mustela nigripes. Mamm. Species 126, 1–3. doi: 10.2307/3503892
Hoogland, J. L. (1995). The Black-Tailed Prairie Dog: Social Life of a Burrowing Mammal. Chicago, IL: University of Chicago Press.
Hopson, J. A. (1973). Endothermy, small size, and the origin of mammalian reproduction. Am. Nat. 107, 446–452. doi: 10.1086/282846
Hwang, Y. T., Larivière, S., and Messier, F. (2007). Energetic consequences and ecological significance of heterothermy and social thermoregulation in striped skunks (Mephitis mephitis). Physiol. Biochem. Zool. 80, 138–145. doi: 10.1086/509211
Isbell, L. A. (2004). “Is there no place like home? Ecological bases of female dispersal and philopatry and their consequences for the formation of kin groups,” in Kinship and Behavior in Primates, eds.B. Chapais and C. M. Berman (Oxford: Oxford University Press), 71–108.
Jarvis, J. U., O'Riain, M. J., Bennett, N. C., and Sherman, P. W. (1994). Mammalian eusociality: a family affair. Trends Ecol. Evol. 9, 47–51. doi: 10.1016/0169-5347(94)90267-4
Johnson, D. D. P., Macdonald, D. W., and Dickman, A. J. (2000). An analysis and review of models of the sociobiology of the Mustelidae. Mamm. Rev. 30, 171–196. doi: 10.1046/j.1365-2907.2000.00066.x
Kaneko, Y., Kanda, E., Tashima, S., Masuda, R., Newman, C., and Macdonald, D. W. (2014). The socio-spatial dynamics of the Japanese badger (Meles anakuma). J. Mammal. 95, 290–300. doi: 10.1644/12-MAMM-A-158
Kaneko, Y., Newman, C., Buesching, C. D., and Macdonald, D. W. (2010). Variations in Badger (Meles meles) sett microclimate: differential cub survival between main and subsidiary setts, with implications for artificial sett construction. Int. J. Ecol. 2010, 10. doi: 10.1155/2010/859586
Kaneko, Y., Suzuki, T., Maruyama, N., Atoda, O., Kanzaki, N., and Tomisawa, M. (1998). The “Trace Recorder,” a new device for surveying mammal home ranges, and its application to raccoon dog research. Mammal Study 23, 109–118. doi: 10.3106/mammalstudy.23.109
Katz, M. E., Miller, K. G., Wright, J. D., Wade, B. S., Browning, J. V., Cramer, B. S., et al. (2008). Stepwise transition from the Eocene greenhouse to the Oligocene icehouse. Nat. Geosci. 1, 329–334. doi: 10.1038/ngeo179
King, C. M. (1989). “The advantages and disadvantages of small size to weasels, mustela species,” in Carnivore Behavior, Ecology, and Evolution, ed J. L Gittleman (London; Boston, MA: Springer), 302–334.
Kinlaw, A. (1999). A review of burrowing by semi-fossorial vertebrates in arid environments. J. Arid Environ. 41, 127–145. doi: 10.1006/jare.1998.0476
Kitao, N., Fukui, D., Hashimoto, M., and Osborne, P. G. (2009). Overwintering strategy of wild free-ranging and enclosure-housed Japanese raccoon dogs (Nyctereutes procyonoides albus). Int. J. Biometeorol. 53, 159–165. doi: 10.1007/s00484-008-0199-7
Kitchener, A. C., Van Valkenburgh, B., and Yamaguchi, N. (2010). “Felid form and function,” in Biology and Conservation of Wild Felids, eds D. W. Macdonald and A. Loveridge (Oxford: Oxford University Press), 83–106.
Koenig, W. D., Pitelka, F. A., Carmen, W. J., Mumme, R. L., and Stanback, M. T. (1992). The evolution of delayed dispersal in cooperative breeders. Q. Rev. Biol. 67, 111–150. doi: 10.1086/417552
Kokko, H., and Ekman, J. (2002). Delayed dispersal as a route to breeding: territorial inheritance, safe havens, and ecological constraints. Am. Nat. 160, 468–484. doi: 10.1086/342074
Kokko, H., and Lundberg, P. (2001). Dispersal, migration, and offspring retention in saturated habitats. Am. Nat. 157, 188–202. doi: 10.1086/318632
Kowalczyk, R., Jêdrzejewska, B., Zalewski, A., and Jêdrzejewski, W. (2008). Facilitative interactions between the Eurasian badger (Meles meles), the red fox (Vulpes vulpes), and the invasive raccoon dog (Nyctereutes procyonoides) in BiałorowieŻa Primeval Forest, Poland. Can. J. Zool. 86, 1389–1396. doi: 10.1139/Z08-127
Kram, R., and Taylor, C. R. (1990). Energetics of running: a new perspective. Nature 346, 265–267. doi: 10.1038/346265a0
Larivière, S. (2001). Ursus Americanus. Mamm. Speices 647, 1–11. doi: 10.1644/1545-1410(2001)647<0001:UA>2.0.CO;2
Lindblad-Toh, K., Wade, C. M., Mikkelsen, T. S., Karlsson, E. K., Jaffe, D. B., Kamal, M., et al. (2005). Genome sequence, comparative analysis and haplotype structure of the domestic dog. Nature 438, 803–819. doi: 10.1038/nature04338
Lindström, E. (1986). Territory inheritance and the evolution of group-living in carnivores. Anim. Behav. 34, 1825–1835. doi: 10.1016/S0003-3472(86)80268-8
Lucas, J. R., Waser, P. M., and Creel, S. R. (1994). Death and disappearance: estimating mortality risks associated with philopatry and dispersal. Behav. Ecol. 5, 135–141. doi: 10.1093/beheco/5.2.135
Macdonald, D. W. (1983). The ecology of carnivore social behaviour. Nature 301, 379–384. doi: 10.1038/301379a0
Macdonald, D. W. (ed.). (2006). The Encyclopedia of Mammals, 3rd Edn., (Oxford: Oxford University Press).
Macdonald, D. W., and Carr, G. M. (1989). “Food security and the rewards of tolerance,” in Comparative Socioecology: The Behavioural Ecology of Humans and Other Mammals, eds V. Standen and R. A. Foley (Oxford: Blackwell Scientific Publications), 75–99.
Macdonald, D. W., Creel, S., and Mills, M. G. L. (2004a). “Society,” in The Biology and Conservation of Wild Canids Canid Society, eds D. W. Macdonald and C. Sillero-Zubiri (Oxford: Oxford University Press), 85–106.
Macdonald, D. W., and Johnson, D. D. P. (2015). Patchwork planet: the resource dispersion hypothesis, society, and the ecology of life. J. Zool. 295, 75–107. doi: 10.1111/jzo.12202
Macdonald, D. W., Newman, C., Dean, J., Buesching, C. D., and Johnson, P. J. (2004b). The distribution of Eurasian badger, Meles meles, setts in a high−density area: field observations contradict the sett dispersion hypothesis. Oikos 106, 295–307. doi: 10.1111/j.0030-1299.2004.12879.x
MacNulty, D. R., Tallian, A., Stahler, D. R., and Smith, D. W. (2014). Influence of group size on the success of wolves hunting bison. PLoS ONE 9:e112884. doi: 10.1371/journal.pone.0112884
Martin, L. D. (1989). “Fossil History of the Terrestrial Carnivora,” in Carnivore Behavior, Ecology, and Evolution, ed J. L. Gittleman (Boston, MA: Springer), 536–568.
Martin, R. D., and MacLarnon, A. M. (1985). Gestation period, neonatal size and maternal investment in placental mammals. Nature 313, 220–223. doi: 10.1038/313220a0
McClearn, D. (1992). Locomotion, posture, and feeding behavior of kinkajous, coatis, and raccoons. J. Mammal. 73, 245–261. doi: 10.2307/1382055
McClellan, H. L., Miller, S. J., and Hartmann, P. E. (2008). Evolution of lactation: nutrition v. protection with special reference to five mammalian species. Nutr. Res. Rev. 21, 97–116. doi: 10.1017/S0954422408100749
McNab, B. K. (1979). The influence of body size on the energetics and distribution of fossorial and burrowing mammals. Ecology 60, 1010–1021. doi: 10.2307/1936869
McNab, B. K. (1983). Energetics, body size, and the limits to endothermy. J. Zool. 199, 1–29. doi: 10.1111/j.1469-7998.1983.tb06114.x
Mills, L. S., and Doak, D. F. (1993). The keystone-species concept in ecology and conservation. BioScience 43, 219–224. doi: 10.2307/1312122
Moore, J. A. H., and Roper, T. J. (2003). Temperature and humidity in badger Meles meles setts. Mamm. Rev. 33, 308–313. doi: 10.1046/j.1365-2907.2003.00021.x
Mosser, A., and Packer, C. (2009). Group territoriality and the benefits of sociality in the African lion, Panthera leo. Anim. Behav. 78, 359–370. doi: 10.1016/j.anbehav.2009.04.024
Nevo, E. (1979). Adaptive convergence and divergence of subterranean mammals. Annu. Rev. Ecol. Syst. 10, 269–308. doi: 10.1146/annurev.es.10.110179.001413
Nevo, E. (1999). Mosaic Evolution of Subterranean Mammals: Regression, Progression, and Global Convergence. Oxford: Oxford University Press.
Newman, C., Zhou, Y. B., Buesching, C. D., Kaneko, Y., and Macdonald, D. W. (2011). Contrasting sociality in two widespread, generalist, mustelid genera, Meles and Martes. Mammal Study 36, 169–188. doi: 10.3106/041.036.0401
Noonan, M. J., Markham, A., Newman, C., Trigoni, N., Buesching, C. D., Ellwood, S. A., et al. (2014). Climate and the individual: inter-annual variation in the autumnal activity of the European Badger (Meles meles). PLoS ONE 9:e83156. doi: 10.1371/journal.pone.0083156
Noonan, M. J., Markham, A., Newman, C., Trigoni, N., Buesching, C. D., Ellwood, S. A., et al. (2015). A new Magneto−Inductive tracking technique to uncover subterranean activity: what do animals do underground? Methods Ecol. Evol. 6, 510–520. doi: 10.1111/2041-210x.12348
Pen, I., and Weissing, F. J. (2000). Towards a unified theory of cooperative breeding: the role of ecology and life history re-examined. Proc. R. Soc. Lond. B. Biol. Sci. 267, 2411–2418. doi: 10.1098/rspb.2000.1299
Plummer, M., Best, N., Cowles, K., and Vines, K. (2006). CODA: convergence diagnosis and output analysis for MCMC. R News 6, 7–11.
Pörtner, H. O. (2002). Physiological basis of temperature-dependent biogeography: trade-offs in muscle design and performance in polar ectotherms. J. Exp. Biol. 205, 2217–2230.
Presley, S. J. (2000). Eira barbara. Mamm. Species 636. doi: 10.1644/1545-1410(2000)636<0001:EB>2.0.CO;2
Prothero, D. R., and Berggren, W. A. (eds.). (2014). Eocene-Oligocene Climatic and Biotic Evolution. Princeton, NJ: Princeton University Press.
Raiche, G., and Magis, D. (2010). nFactors: Parallel Analysis and Non Graphical Solutions to the Cattell Scree Test. R package version 2.3
Raine, R. M. (1987). Winter food habits and foraging behaviour of fishers (Martes pennanti) and martens (Martes americana) in southeastern Manitoba. Can. J. Zool. 65, 745–747. doi: 10.1139/z87-112
R Core Team. (2014). R: A language and environment for statistical computing. Vienna: R foundation for Statistical Computing.
Reimchen, T. E. (2000). Some ecological and evolutionary aspects of bear-salmon interactions in coastal British Columbia. Can. J. Zool. 78, 448–457. doi: 10.1139/z99-232
Rogers, L. M., Forrester, G. J., Wilson, G. J., Yarnell, R. W., and Cheeseman, C. L. (2003). The role of setts in badger (Meles meles) group size, breeding success and status of TB (Mycobacterium bovis). J. Zool. 260, 209–215. doi: 10.1017/S0952836903003649
Roper, T. J., Ostler, J. R., Schmid, T. K., and Christian, S. F. (2001). Sett use in European badgers Meles meles. Behaviour 138, 173–187. doi: 10.1163/15685390151074366
Samuels, J. X., Meachen, J. A., and Sakai, S. A. (2013). Postcranial morphology and the locomotor habits of living and extinct carnivorans. J. Morphol. 274, 121–146. doi: 10.1002/jmor.20077
Savin, S. M. (1977). The history of the Earth's surface temperature during the past 100 million years. Ann. Rev. Earth Planet. Sci. 5, 319–355. doi: 10.1146/annurev.ea.05.050177.001535
Sheffield, S. R., and King, C. M. (1994). Mustela nivalis. Mamm. Species 454, 1–10. doi: 10.2307/3504183
Shield, J. (1972). Acclimation and energy metabolism of the dingo, Cards dingo and the coyote, Canis latrans. J. Zool. 168, 483–501. doi: 10.1111/j.1469-7998.1972.tb01363.x
Sicuro, F. L., and Oliviera, L. F. B. (2011). Skull morphology and functionality of extant Felidae (Mammalia: Carnivora): a phylogenetic and evolutionary perspective. Zool. J. Linn. Soc. 161, 414–462. doi: 10.1111/j.1096-3642.2010.00636.x
Skerratt, L. F., Skerratt, J. H. L., Martin, R., and Handasyde, K. (2004). The effects of sarcoptic mange on the behaviour of wild common wombats (Vombatus ursinus). Aust. J. Zool. 52, 331–339. doi: 10.1071/ZO02062
Smith, J. E., Swanson, E. M., Reed, D., and Holekamp, K. E. (2012). Evolution of cooperation among mammalian carnivores and its relevance to hominin evolution. Curr. Anthropol. 53, S436–S452. doi: 10.1086/667653
Smorkatcheva, A. V., and Lukhtanov, V. A. (2014). Evolutionary association between subterranean lifestyle and female sociality in rodents. Mammal. Biol. 79, 101–109. doi: 10.1016/j.mambio.2013.08.011
Sobrero, R., Inostroza-Michael, O., Hernández, C. E., and Ebensperger, L. A. (2014). Phylogeny modulates the effects of ecological conditions on group living across hystricognath rodents. Anim. Behav. 94, 27–34. doi: 10.1016/j.anbehav.2014.05.008
Stebbins, G. L. (1981). Coevolution of Grasses and Herbivores. Ann. Missouri Bot. Gard. 68, 75. doi: 10.2307/2398811
Šumbera, R., Chitaukali, W. N., and Burda, H. (2007). “Biology of the silvery mole-rat (Heliophobius argenteocinereus). Why study a neglected subterranean rodent species?,” in Subterranean Rodents (Berlin; Heidelberg: Springer), 221–236.
Taylor, W. A., and Skinner, J. D. (2003). Activity patterns, home ranges and burrow use of aardvarks (Orycteropus afer) in the Karoo. J. Zool. 261, 291–297. doi: 10.1017/S0952836903004217
Tinbergen, N. (1963). On aims and methods of Ethology. Ethology 20, 410–433. doi: 10.1111/j.1439-0310.1963.tb01161.x
Van Valkenburgh, B. (1987). Skeletal indicators of locomotor behavior in living and extinct carnivores. J. Vertebr. Paleontol. 7, 162–182. doi: 10.1080/02724634.1987.10011651
Van Vuren, D. (1996). Ectoparasites, fitness, and social behaviour of yellow-bellied marmots. Ethology 102, 686–694. doi: 10.1111/j.1439-0310.1996.tb01159.x
Vicentini, A., Barber, J. C., Aliscioni, S. S., Giussani, L. M., and Kellogg, E. A. (2008). The age of the grasses and clusters of origins of C4 photosynthesis. Glob. Change Biol. 14, 2963–2977. doi: 10.1111/j.1365-2486.2008.01688.x
Vleck, D. (1981). Burrow structure and foraging costs in the fossorial rodent, Thomomys bottae. Oecologia 49, 391–396. doi: 10.1007/BF00347605
von Schantz, T. (1984). Spacing strategies, kin selection, and population regulation in altricial vertebrates. Oikos 42, 48. doi: 10.2307/3544608
Voorhies, M. R., and Toots, H. (1970). An unusual burrow of a Tertiary vertebrate. Rocky Mt. Geol. 9, 7–8.
Waser, P. M. (1981). Sociality or territorial defense? The influence of resource renewal. Behav. Ecol. Sociobiol. 8, 231–237. doi: 10.1007/BF00299835
Waser, P. M. (1996). “Patterns and consequences of dispersal in gregarious carnivores,” in Carnivore Behavior, Ecology, and Evolution, ed J. L. Gittleman (Ithaca, NY: Springer), 267–295.
White, C. R. (2005). The allometry of burrow geometry. J. Zool. 265, 395–403. doi: 10.1017/S0952836905006473
Whittington-Jones, G. M., and Bernard, R. (2011). Aardvark burrows: a potential resource for animals in arid and semi-arid environments. Afr. Zool. 46, 362–370. doi: 10.3377/004.046.0215
Williams, S. A., and Shattuck, M. R. (2015). Ecology, longevity and naked mole-rats: confounding effects of sociality? Proc. Biol. Sci. 282, 20141664–20141664. doi: 10.1098/rspb.2014.1664
Wilson, D. E., Mittermeier, R. A., and Cavallini, P. (2009). Handbook of the Mammals of the World, Vol 1 – Carnivores, 1st Edn., eds D. E. Wilson and R. A. Mittermeier (Barcelona: Lynx Edicions).
Wolff, J. O. (1997). Population regulation in mammals: an evolutionary perspective. J. Anim. Ecol. 66, 1–13. doi: 10.2307/5959
Woodroffe, R., and Ginsberg, J. R. (1997). “Past and future causes of wild dogs' population decline,” in The African Wild Dog Status Survey and Conservation Action Plan, eds R. Woodroffe, J. R. Ginsberg, D. W. Macdonald (Gland: IUCN, IUCN/SSC Candid Specialist Group), 58–74.
Zack, S., and Stutchbury, B. J. (1992). Delayed breeding in avian social systems: the role of territory quality and “floater” tactics. Behaviour 123, 194–219. doi: 10.1163/156853992X00020
Keywords: burrowing ecology, subterranean mammals, socio-ecology, sociality, philopatry, resource dispersion hypothesis
Citation: Noonan MJ, Newman C, Buesching CD and Macdonald DW (2015) Evolution and function of fossoriality in the Carnivora: implications for group-living. Front. Ecol. Evol. 3:116. doi: 10.3389/fevo.2015.00116
Received: 19 May 2015; Accepted: 22 September 2015;
Published: 13 October 2015.
Edited by:
Dustin R. Rubenstein, Columbia University, USACopyright © 2015 Noonan, Newman, Buesching and Macdonald. This is an open-access article distributed under the terms of the Creative Commons Attribution License (CC BY). The use, distribution or reproduction in other forums is permitted, provided the original author(s) or licensor are credited and that the original publication in this journal is cited, in accordance with accepted academic practice. No use, distribution or reproduction is permitted which does not comply with these terms.
*Correspondence: David W. Macdonald, Wildlife Conservation Research Unit, Department of Zoology, The Recanati-Kaplan Centre, University of Oxford, Tubney House, Abingdon Road, Tubney OX13 5QL, UK,ZGF2aWQubWFjZG9uYWxkQHpvby5veC5hYy51aw==