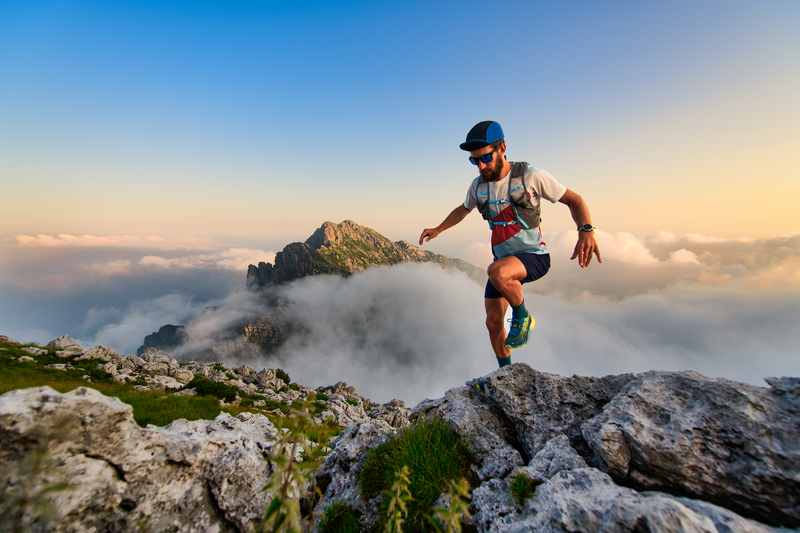
95% of researchers rate our articles as excellent or good
Learn more about the work of our research integrity team to safeguard the quality of each article we publish.
Find out more
ORIGINAL RESEARCH article
Front. Ecol. Evol. , 08 September 2015
Sec. Paleoecology
Volume 3 - 2015 | https://doi.org/10.3389/fevo.2015.00104
Non-ribosomal synthetase-produced cyanopeptoline oligopeptides enables differentiation of subpopulations of the cyanobacterial genus Planktothrix into chemotypes. It is unknown what influences the population structuring of these chemotypes. Sediment cores from seven lakes in southern Norway allowed temporal reconstruction of chemotype diversity from sites where there is only fragmented historical information. Sediment DNA was amplified using primers designed to specify the chemotype variations found within the cyanopeptoline ociB gene cluster. Findings indicate that of the seven lakes studied, only two lakes had Planktothrix populations containing all four of the most common Norwegian chemotypes. We used principal component analysis and Kendall tau analysis to investigate the ability of monitoring data to predict chemotype diversity, and to identify possible biotic or abiotic barriers to chemotype dispersal. The best predictor was a negative relationship between number of chemotypes present in a lake and the concentration of chlorophyll a in the top 0–4 m. At low chlorophyll a concentrations, light penetration is typically deeper, which could allow light tolerant Planktothrix to move deeper into the colder waters. Recent research findings have suggested this allows for a window of opportunity for Planktothrix to escape parasitism. With this added cold, light-constrained niche, more chemotypes might find refuge. The resulting increase in chemotype diversity within Planktothrix populations could present a greater defense against parasitism when conditions varied, such as by seasonal light changes.
The ancient photosynthetic prokaryotes, cyanobacteria, are thought to have been important in the oxygenation of early earth (Agha and Quesada, 2014). However, public concern over the proliferation of cyanobacteria began to rise during the 1960s and 1970s as eutrophication increased, bringing with it a rise of cyanobacterial blooms that were often found to produce toxins. Lake monitoring of cyanobacteria populations began as a result. However, this monitoring was often sporadic and regionally variable. The resulting historical records of cyanobacterial abundance are therefore often inadequate for lake management and a hindrance to ecological research.
One common cyanobacterium genus of concern to lake managers is Planktothrix. This cyanobacterium produces oligopeptides that include several classes of toxins such as microcystins and cyanopeptolins. These oligopeptides are highly diverse in both form and function. Some are capable of producing hepatotoxins while others can produce internal protease inhibitors that are not released until cell death. The oligopeptides are the product of large modules, non-ribosomal peptide synthetases (NRPS) that are formed by gene clusters (Agha and Quesada, 2014).
Recent research has shown that Planktothrix can be subdivided, based on the presence of cyanopeptoline oligopeptide profiles, into chemotypes (Rohrlack et al., 2008). Further research has shown that this subpopulation classification can also be reflected within the cyanopeptoline gene clusters (Sogge et al., 2013). This has led to the use of the cyanopeptoline gene cluster as molecular marker for chemotypes.
A large number of Planktothrix chemotypes have been found throughout central Europe. However, in Norway, only a few chemotypes have been recovered (Welker et al., 2004; Kurmayer and Gumpenberger, 2006; Yéprémian et al., 2007; Rohrlack et al., 2008; Sogge et al., 2013). This might be related to a general pattern of latitudinal decrease in species richness (see for instance Mittelbach et al., 2007), or it might indicate that Planktothrix populations in central Europe are much older and therefore more evolutionarily divergent than the Norwegian strains (Sogge et al., 2013). Although the chemotype variation found in Norway is limited, four primary chemotype profile clusters have been repeatedly identified. Oligopeptide identification and sequencing of the oci gene cluster has primarily used single filament derived clonal cultures and lake filters, obtained from a small set of monitored lakes. These chemotypes have shown stability in this region for more than 40 years (Rohrlack et al., 2008, 2009; Sogge et al., 2013).
Research has suggested that cyanopeptoline oligopeptides might be used as a defense against chytrid fungal parasitism (Sønstebø and Rohrlack, 2011). This is thought to be due to the ability of internal cellular concentrations of oligopeptides to inhibit serine proteases that are expressed by chytrid rhizoids during infection. Multiple chemotypes with oligopeptide variations might place limitations on chytrid adaptation. This limitation due to diversity has been seen in other chytrid parasite—host relationships (De Bruin et al., 2008).
Because research into chemotype subdivision has been developed only recently, there is limited historical data on Planktothrix community structure, distribution, or diversity available for most lakes in Norway. However, sediment core analysis has the potential to allow reconstruction of historical timelines for the distribution and diversity of Planktothrix in individual lakes.
Research has shown that sediment DNA can be amplified using chemotype-specific primers that identify the four main Norwegian Planktothrix chemotypes (Kyle et al., 2015b). These include chemotypes 1, 5, 7, and 9 (cht1, cht5, cht7, and cht9), based on the chemotype nomenclature used by Rohrlack et al. (2009). To study the distribution of these four common Planktothrix chemotypes in Norway, DNA was extracted from sediment cores from seven regional lakes and amplified to analyze Planktothrix chemotype composition and dispersal across lakes over time. While a small set of these lakes have had chemotypes populations identified, it was unknown whether or not these would also be identifiable in the DNA extracted from the sediments. Also, while Planktothrix was identified as present at certain times in all the lakes in the study, chemotype populations had not been identified for all lakes within this set of chemotypes. However, DNA degradation has been found to be a potential problem in the sediment in this region (Kyle et al., 2015a). Therefore, to avoid the potential for DNA degradation in these oxic lakes, only the data from the past 65 years was analyzed. The resulting chemotype information was combined with available monitoring data. The community structure for each lake was compared over time across all lakes to determine possible barriers to chemotype diversity and community composition on a local or regional scale.
The sediment from seven lakes in southern Norway was sampled between 2013 and 2014. These included Årungen, Bjørkelangen, Gjersjøen, Hålandsvatnet, Helgetjern, Kolbotnvannet, and Steinsfjorden. Coordinates for the lake locations along with other general lake information data are given in Table 1 and Figure 1. Monitoring data was obtained from several sources. These included (1) The Norwegian Institute for Water Research (NIVA) monitoring data was used for the majority of lakes in the data set (Haande et al., 2013; Report 6511; in Norwegian); (2) The International Research Institute of Stavanger (IRIS) data was used for information pertaining to Hålandsvatnet (Molversmyr Rapport IRIS—2006/134 and 2010 10/146; Molversmyr, 2006, 2010; in Norwegian); (3) The Norwegian Water Resources and Energy Directorate (NVE) data set was used for general depth and watershed information; and (4) PURA, water quality monitoring report from the River basin district Follo/Oslo (Årsrapport 2008–2010) was used for information about Årungen.
Figure 1. Map of Europe with inset of Norway indicating the locations of the seven Norwegian lakes sampled in this data set.
These lakes were chosen based on a history of Planktothrix blooms reported during the past 20 years and the availability of at least some monitoring data. With the exception of Hålandsvatnet, the lakes are clustered around Oslo in southeastern Norway with the furthest distance between them being 45 km. Hålandsvatnet is approximately 300 km to the west, near the city of Stavanger. Two of the lakes, Gjersjøen and Kolbotnvannet, have a connected watershed, with outflow from Kolbotnvannet flowing into Gjersjøen. The overall average lake area is 3.3 km2 with the smallest lake being Kolbotnvannet (0.3 km2) and the largest is Steinsfjorden (13.9 km2). Maximum depth ranges from 64 m (Gjersjøen) to 3.5 m (Helgetjern). Watersheds differ from 0.9 km2 (Helgetjern) to 85 km2 (Gjersjøen) with main land usage being either agriculture or urban. Most urban areas near these lakes are expanding due to proximity to either Oslo or Stavanger. Overall, the data for the seven lakes can be generalized to describe the lake types. Årungen is a lake with a large agricultural watershed. Bjørkelangen is a shallow lake with a large runoff similar to Årungen. Gjersjøen is a deep lake but with a large watershed that also includes Kolbotnvannet, a small lake that flows into it. A more urbanized area surrounds Kolbotnvannet compared with Gjersjøen. Hålandsvatnet is in close proximity to the ocean, with increased wind mixing compared with the other lakes. It is within close range of a large city with a moderate watershed area. Helgetjern is a shallow lake with a small watershed but with a more moderate residence time than these first four lakes. Steinsfjorden is the largest lake with at least twice the average size and watershed area while being similar to Hålandsvatnet in depth. However, Steinsfjorden also has the longest residence time.
Two sediment cores with an average length of 50 cm were taken from each lake. The cores were taken using a gravity corer in an area of the lake most likely to be representative of the accumulation sediment for the lakes. Table 1 presents a summary of sampling information for the lakes, including core dates, core depths, lake depths at sampling locations, and basic parameters of the lakes including lake areas, watershed areas, and land use. All cores were brought to the lab and stored in the dark at ~10°C for less than 24 h before processing. The cores were sectioned into 1 cm slices, starting at the sediment surface and working downward toward the older layers. Samples were cut using a stainless steel cutting tool which was cleaned after each cut to avoid contamination between sample layers. Samples were either directly placed in scintillation vials for later dating determination, or into single use nuclease free cups prior to DNA sampling. Material from one core was dried at 60°C in scintillation vials and used for dating measurements while the other core was immediately subsampled for DNA extraction and for the determination of dry weight (DW), water content, and organic matter (OM). Following DNA extraction (see extraction method below), the remaining majority of the core sample was weighed, dried at 60°C until all water was evaporated, and reweighed. Then dry samples were crushed, re-weighed, and combusted at 500°C for 2 h and reweighed to determine mass loss on ignition (LOI). This allowed for the determination of sample layer water content and organic matter. Based on these data, DNA concentration was calculated as ng per gram organic matter for each core layer.
To obtain core dating and sedimentation rates, samples from the first core were placed into scintillation vials and heated at 60°C until dry. The 137Cs concentration was analyzed for each layer by analyzing 137Cs content of dried sediment samples for 2 h each using a Sodium-Iodine detector (Wallac 1480 Wizard 3″ gamma counter, PerkinElmer) at the Norwegian University of Life Sciences (NMBU) Isotope Laboratory. The 137Cs peak layer depth was converted to age by assuming it represented 1986, the year of the Chernobyl nuclear accident. The distance between the surface and 1986 was used to calculate a sedimentation rate for this period. This sedimentation rate was extrapolated to the layers representing years before 1986. This estimation did not take into account differences in annual sedimentation or any potential sediment compaction that might have occurred.
Originally, Rohrlack et al. (2008) used liquid chromatography-tandem mass spectrometry (LC-MS/MS) to determine the oligopeptide profiles of the four common chemotypes. For our study, relative oligopeptide abundances by chemotypes, according to Rohrlack et al. (2008, Figure 1), were aggregated. We then constructed a heat map of the oligopeptide by chemotype matrix by ordering chemotypes and oligopeptides according to their scores on the first axis of a correspondance analysis (CA) of the matrix. The first CA axis is technically the same as a reciprocal averaging of chemotype and oligopeptide scores, and is not subject to artifact distortions of higher order CA axes. The CA ordination is based on chi square distance, which gives less weight to double zeros than euclidean distance. Analysis was performed using R, version 3.1.1 (R Core Team, 2014).
The second of each lakes cores were maintained at 4°C until DNA extraction. DNA was extracted within 5 days of specific lake coring. PowerSoil® DNA Isolation kit (MoBio Laboratories, Inc., Carlsbad, CA USA; cat.no. 12888) was used for the extraction of the sediment DNA. Samples were taken from the center of each core slice using single-use polypropylene spatulas and between 0.2 and 0.3 g wet weight were added to the extraction solution.
Four separate chemotype standards were developed from Planktothrix cell culture lines from the Norwegian Institute of Water Research—Culture Collection of Algae (NIVA-CCA) representing the four common chemotypes found in Norway. These cultures and associated chemotypes were NIVA-CYA 98 (cht1), NIVA-CYA 407 (cht5), NIVA-CYA 56/3 (cht7), and NIVA-CYA 405 (cht9). All strains were grown in batch culture at 20°C and 3–5 μmol PAR m−2 s−1 light using Z8 medium, and harvested in the exponential growth phase. Filaments were centrifuged and the resulting pellets transferred to the initial tube of the extraction kit for processing using the same method as with the sediment samples. Concentrations were determined for each chemotype standard using Nanodrop® (Thermo Scientific) UV-Vis spectrophotometer and then these standards were diluted to create four point standard curves for the determination of sample concentrations.
Primers and probes for the identification and quantification of the four common Planktothrix chemotypes were previously defined in the Planktothrix cyanopeptoline gene cluster ociB region (Kyle et al., 2015b). Four Planktothrix genotypes corresponding to the classification of chemotypes based on research by Rohrlack et al. (2008) were distinguished by polymorphisms within the ociB gene fragment. Based on work by Sogge et al. (2013) a sequence alignment of strains with known chemotype affiliation was made using BioEdit (version 7.2.0) and uniform regions were identified for each of the four genotypes. Regions of identity within genotypes and maximum sequence variability to the other genotypes were chosen. Primers and probes were tested in silico and in vitro to ensure specificity. BLAST® (Basic Local Alignment Search Tool developed by The National Center for Biotechnology Information) analysis was performed using the four Planktothrix chemotypes primers and probes. Cht1, cht5, cht7, and cht9 were cross-tested using all combinations of primer sets and standards to ensure chemotype specificity.
Amplification of Planktothrix DNA was performed using Fast qPCR MasterMix Plus No ROX dTTP (Eurogentec product number RT-QP2X-03+NRWOUNF) with a BioRad CFX96TM Real-Time PCR Detection System (Bio-Rad, USA). All primer and probe thermal cycling parameters were optimized before measuring the environmental samples. These parameters were set at an initial denaturation of 95°C for 5 min followed by 50 cycles of a two-step protocol, including denaturation at 95°C for 15 and 30 s for the annealing/extension. Optimization results indicated differences in annealing temperature dependent on the target (Table 2). The PCR reaction included 300 nM of each primer, 100 nM probe, 5 μL of template DNA, and 12.5 μL of 2X Master mix per well with a total volume of 25 μL. Bovine serum albumin (BSA, VWR #786006) was added with a final concentration of 0.2 μg μL−1 to limit potential inhibition due to the sediment matrix. All sediment DNA was diluted 1:50 with nuclease free water prior to amplification to avoid possible inhibition.
Table 2. Planktothrix chemotype (C) specific primers in the region of the ociB gene for the four common chemotypes found in Norway with two-step PCR conditions.
Sediment DNA was amplified in duplicate by qPCR for each of the four Planktothrix chemotype primer and probe sets, cht1, cht5, cht7, and cht9 (Table 2). Any amplification beyond 40 cycles was treated as a non-detect since specificity was resolved only to 40 cycles and not beyond. Earlier research for a subset of these lakes found fragmentation of the DNA (Kyle et al., 2015a). Only short 50 base pair fragments amplified in older sediment cores. Therefore, based on the dating analysis, only 1950 to present data were included.
Data analysis and graphical presentation were performed using R software (R Core Team, 2014, version 3.1.1). Principal component analysis (PCA) of water quality parameters was performed on monitoring data aggregated as means for each lake using the R package “vegan,” community ecology package. Chemotype richness was estimated as the total number of chemotypes recorded in each lake. Relationships between chemotype richness and water quality parameters were investigated by Kendall's tau rank correlations, using p-values adjusted by the method of Holm (1979) to correct for Type 1 errors due to multiple testing.
Data used in the determination of sediment dating for individual lakes, obtained by 137Cs analysis, are presented in Data Sheet 1. This dating was then used then to compare each lake to monitoring data and molecular results. Summer monitoring data, primarily obtained from NIVA for all lakes except Hålandsvatnet (IRIS), resulted in some lakes having more data collection points than other lakes (Table 3). In order to best compare monitoring data between lakes, all data points were averaged for each lake prior to statistical analysis. The mean total nitrogen (TN) for all lakes was 1408 μg L−1 (sd = 301). The lake with the highest TN was Årungen (2692 μg L−1) and the lowest was Steinsfjorden with 312 μg L−1. Mean total phosphorus (TP) mean was 30 μg L−1 (sd = 41.3) with Helgetjern having the highest TP (143 μg L−1) and Steinsfjorden the lowest (11 μg L−1). These two lakes also had the highest chlorophyll a concentrations in the top four meters. Helgetjern was 38 μg L−1 chlorophyll a and Steinsfjorden was 7 μg L−1, with an overall mean for all lakes at 13 μg L−1 (sd = 10).
Table 3. Summary of available monitoring data for the major nutrients, total nitrogen (TN) and total phosphorus (TP), and the concentrations of chlorophyll a (chl.a) in the upper four meters of the seven lakes in this study.
Our analysis, based on oligopeptides for the four common Norwegian chemotypes as determined by Rohrlack et al. (2008), is shown in Figure 2. Cht1 and cht9 were more similar to each other than to either cht5 or cht7. Oligopeptide variation increased both within and between cht5 and cht7. The R-script for analysis of the chemotypes can be found in Presentation 1.
Figure 2. Analysis of oligopeptide differences taken with permission from published data reported by Rohrlack et al. (2008; Figure 1). Relative oligopeptide abundances by chemotypes were aggregated (A) indicating a clustering (based on a chi square similarity measure) between and closer relationship for the chemotypes (cht) 1 and 9 than for 5 and 7. A heat map (B) was also constructed of the oligopeptide by chemotype matrix by ordering chemotypes and oligopeptides according to their scores on the first axis of a correspondence analysis of the matrix. Gradients from yellow to red indicate increased similarity within chemotypes.
Previous findings by Rohrlack et al. (2008) indicate that Lake Steinsfjorden single filament clonal cultures included the common four chemotypes, cht1, cht5, cht7, and cht9. Genomic data from Sogge et al. (2013) analyzed three lakes and found Kolbotnvannet had cht1 and cht9 while Gjersjøen and Steinsfjorden had all four common chemotypes. This is in agreement with our sediment findings.
Concentrations of lake sediment chemotypes normalized by organic matter, determined by use of individual culture derived standard curves, are presented in Data Sheet 2. The occurrences or non-detect of the four Planktothrix chemotypes in sediment DNA were compared between lakes (Figure 3). Results showed that two lakes, Steinsfjorden and Gjersjøen, were the only lakes where all four chemotypes were detected at some historical point. However, Gjersjøen sediment amplified cht5 in only one sediment layer, 1976. Chemotype amplification results showed Kolbotnvannet having cht1 and cht9, Helgetjern only cht9, Bjørkelangen cht5 and cht9 (both only in 2013) and cht7, Årungen cht5 and cht9, but again, only in the most recent samples. The two lakes with a shared watershed, Gjersjøen and Kolbotnvannet, showed little similarity with respect to chemotype populations. Helgetjern, the furthest lake from Oslo had only one chemotype present. In general, cht1 was found a total of 58 times in the seven lakes. Cht9 occurred 51 times, cht7 occurred 36 times and cht5 only 10 times. Six lakes had cht9 while four lakes had the other three chemotypes.
Figure 3. Identification of the presence (solid red circle) or non-detect (black +) of four common Norwegian Planktothrix chemotypes (1, 5, 7, 9) from each centimeter of sediment cores obtained from seven Norwegian lakes. These centimeters were converted to year by calculation of a sedimentation rate based on the identification of a 137Cs peak identifying 1986, year of the Chernobyl nuclear accident. Estimates for each sample date is the mid point year for each centimeter. Sampling intervals differ between lakes, depending on the calculated individual lake sedimentation rates. Due to potential in this region for DNA degradation in sediment, core analysis excluded core samples older than 1950.
The PCA analysis of lake characteristics and monitoring data revealed that the first axis was represented by shallow eutrophic lakes on one side and large, deep, and more mesotrophic lakes with high residence times on the other side (Figure 4). The first axis explained 52% of the total variation while the second axis explained 28%. The biotic factors (TN, TP, chl a) appeared clustered in opposition to the abiotic (residence time, lake area, lake maximum depth), with the exception of sedimentation rate, which was associated with the biotic cluster. Helgetjern was associated with high TP and chl a while Årungen was associated with high sedimentation rate and TN. Steinsfjorden clustered on the opposite side with high residence time and lake area. Two lakes, Bjørkelangen and Kolbotnvannet, held a central position. Individual correlations of diversity against environmental parameters can be found in Presentation 2.
Figure 4. Principal component analysis (biplot) of lake characteristics and monitoring data. First axis represents 52% of the total variation while second axis represents 28%.
Relationships between chemotype richness and water quality parameters (Figure 5 and Presentation 2) investigated by Kendall's tau rank correlations indicated that the only family-wise significant relationship (p = 0.048) was between number of different chemotypes in a lake and the monitoring data of chl a concentration, which was the amount of chl a reported in the first four meters of a lake. Results indicated a separation of biotic and abiotic factors with a positive relationship for maximum depth, lake area and watershed while TP, TN, and chl a relationships were negative.
Figure 5. Kendal tau rank correlation for the number of Planktothrix chemotypes (ranging from one to four possible chemotype communities) per lake with basic monitoring data including lake area, lake depth, residence time, sediment rate, total phosphorus, total nitrogen, chlorophyll. Red dotted line separates the positive and negative relationships. Family-wise significant correlation (0.05 significance level) is marked with a filled symbol with all p-values presented next to symbols.
Because of the production of the toxin microcystin by Planktothrix, the mcy gene cluster is a common focus in Planktothrix research. However, the cyanopeptoline gene cluster ociB contains sufficient sequence variation to allow for separation of the genus into subpopulation chemotypes for Planktothrix often found in Norway (Sogge et al., 2013). While other variants have been found in Europe, ongoing research over the past 40 years in Norway has failed to detect other chemotypes (Rohrlack et al., 2008; Sogge et al., 2013). We therefore limited the chemotypes tested in this study to only the variants associated with this region. This does leave the possibility that other variants exist in this region and have not been included in this study. Regardless, the use of the most common variants allowed us to study dispersal and diversity in this region.
Research has shown the potential of the oligopeptides to be used as a defense against parasitic chytrid infection (Sønstebø and Rohrlack, 2011; Rohrlack et al., 2013). Therefore, the diversity between the oligopeptide profiles of different chemotypes might be considered a defense against chytrid parasitism. Understanding the regional diversity of these oligopeptide-based chemotypes might shed light on this question. Research on the formation of Planktothrix chemotypes based on oligopeptides has typically utilized either cultures (Rohrlack et al., 2008) or filtered lake samples (Sogge et al., 2013). However, due to the unique capability of sediment to record time, our research allowed for a novel approach to identify chemotype communities where historical data had not been previously available. Within our study, several specific lakes have also been included in the work by Rohrlack et al. (2008) and Sogge et al. (2013). The findings of our sediment data agree with their results of chemotypes and genotypes. Unfortunately, due to dating of the sediments and sedimentation rate differences, matching of the sediment data with the fine scale analysis of their research, as well as monitoring data, was not possible. However, there was no identification of chemotypes found in the sediment that had not been identified in the clonal cultures or lake filters.
The cyanopeptoline oligopeptides were compared between chemotype populations. Data indicated (Figure 2) that the greatest similarity was found within cht1 and between the oligopeptide profiles of cht1 and cht9. These two chemotypes were also found to most often co-occur in the same layer of the sediment. In fact, in every lake either cht1 or cht9, or the combination of the two, occurred at some point in a core. This would suggest that these two chemotypes had the highest competitive abilities of the four, and were able to maintain a continued presence in this region for many decades.
Cht5 and cht7 did not exhibit similar fitness. They were found to co-exist in only one lake, Steinsfjorden, out of the seven lakes sampled, and their overall presence in the sediment layers was less frequent. Sediment data indicates that cht7 and cht5 could be found in Steinsfjorden in the 1960s, but were not detected in other lakes until a decade or more later. Due to DNA degradation, it is possible that they were present but not in detectable concentrations in these lakes. However, our data suggests that either they are less transportable, which is unlikely since the chemotypes are of similar filamentous form, or once transported, they were less capable of securing and maintaining positions in the Planktothrix communities of the other lakes. This might be due to potential selective pressures or competitive disadvantages (Mittelbach, 2012) of the oligopeptide profiles present in these chemotypes compared with those in cht1 or cht9. Little is yet known about competition between these chemotypes in a natural setting.
Regardless, all four Planktothrix chemotypes common to this area of Norway were found within this set of seven lakes. It is possible that there are other chemotypes present in these lakes that were not identified by chemotype specific primers. Genotype determination by Sogge et al. (2013) shows no indication of other chemotypes in three of the lakes studied. However, even if other chemotypes are undetected, it remains interesting to observe the four identifiable chemotypes in terms of dispersal. Only two lakes had communities that were comprised of all four major chemotypes, however chemotype community structures varied over time within these lakes, such that during certain periods, only one or two chemotypes were present out of the four. This suggests that dispersal is more than likely not physically restricted in this area, and both local extinction and reinvasion are possible alternatives. Since not all lakes developed or maintained four-chemotype communities across time, it is conceivable that there are barriers to colonization and persistence in the Planktothrix community structure.
In this lake sediment study, a significant negative correlation was found between chemotype diversity and surface (0–4 m) chlorophyll a concentrations. Typically, a decrease in surface chlorophyll a concentrations is the result of a decrease in phytoplankton cell concentration in the epilimnion. This decrease in phytoplankton density in the surface often leads to a situation of increased light penetration to the deeper layers of the lake, although this is not always the outcome depending on other lake factors such as non-plankton light attenuation due to inorganic turbidity or dissolved inorganic matter. However, when light is capable of penetrating deeper into a lake, Planktothrix are able to move down in the water column while still maintaining positive growth rates. This is the result of highly effective phycobiliprotein light harvesting pigments, phycocyanin, and phycoerythrin. Phycocyanin, present in all Planktothrix strains, absorbs in the 620–630 nm range while phycoerythrin, the red pigmentation found in some strains of Planktothrix, absorbs in the 560–570 nm range (Tomming-Klunderud et al., 2013). The ability to absorb sufficient energy at low light levels and the variation in peak absorbance ranges allow Planktothrix to populate areas that are light limiting to other phytoplankton. At the same time, nutrients in the metalimnion are typically less limiting for growth than in the epilimnion (Sommer et al., 1986). Therefore, there is a competitive advantage for Planktothrix in moving down in the water column when light allows. A non-limiting light-nutrient niche could form that would represent an advantage for Planktothrix in general and for increasing their chemotype diversity. The result of our study, suggesting that for these seven lakes a decrease in epilimnetic phytoplankton resulted in an increase in oligopeptide diversity, could be due to increased niche availability.
From these results, it is apparent that there must be some advantage to chemotypes or chemotype communities when surface phytoplankton biomass decreases. The mechanics of transport and dispersal between lakes are probably equal across all chemotypes, but there may still be fitness differences between the chemotypes. Once transported to a new location, chemotypes must have sufficient fitness to be able to reproduce their genetic code in the new environment in order to develop and maintain a population that can compete for resources. When the lake surface becomes more transparent, the environment of the lake could favor increased chemotype fitness in at least three possible ways. These lake environmental conditions include (1) decreasing phytoplankton completion that favors chemotype selection and diversity, (2) metalimnion release from grazing and parasitism that results in a more stable chemotype population, (3) niche diversification that allows for protection of chemotypes and increased community diversity.
The Plankton Ecology Group (PEG) model by Sommer et al. (1986), as well as the update by Sommer et al. (2012), describes the major successional steps of plankton ecology. In this model, seasonally changing population densities are affected by nutrients such as phosphorus and nitrogen, as well as temperature, light and grazing. Early spring increases in phytoplankton results in grazing pressures that remove edible phytoplankton creating a clear water phase where nutrients are typically less limiting. As the phytoplankton population begins to build again, large-sized, grazing-resistant phytoplankton is an advantage. Additional chemotypes would gain from less competition for nutrients during this period, relative to the more strongly stratified and nutrient limited conditions of summer. In addition, more efficient use of light would allow Planktothrix to utilize the metalimnion, thereby avoiding a stronger nutrient completion found in the epilimnion. Less nutrient competition and nutrient limitation could lead to improved growth rates of the chemotypes.
Minimizing grazing losses are important in maintaining a viable population. Metazoan grazing has limited impact on Planktothrix due to both the filamentous form of the cyanobacterium and the negative effect of oligopeptide toxins on grazers (DeMott et al., 1991). However, host-specific chytrid fungi can effectively parasitize Planktothrix (Sønstebø and Rohrlack, 2011; Kyle et al., 2015b). Planktothrix chemotypes might be able to utilize specific combinations of oligopeptides as a defensive system (Rohrlack et al., 2013). These cyanopeptoline oligopeptides are serine protease inhibitors, which might have the potential to reduce the effectiveness of proteases released by the chytrid rhizoid during the infection cycle (Sønstebø and Rohrlack, 2011).
Chytrids have been shown to have environmental constraints that reduce the number of zoospores. These consist of temperature, light, and host density. While Planktothrix maintain a year-round presence in lakes, chytrids enter into a resting stage during the winter when water temperatures are low. In winter it is not uncommon to see Planktothrix form blooms just under the ice as they are released from parasitic pressure (Halstvedt et al., 2007). Likewise, in the spring chytrids remain constrained until temperature increases sufficiently to build a large enough population to effectively exploit Planktothrix populations. This temperature dependence of chytrid growth allows a window of opportunity to evade parasitism. Similar research has shown that diatoms use this window of opportunity to reproduce in early spring while temperature stress is limiting chytrid reproduction (Gsell, 2013).
Research on diatom-parasitizing chytrids showed that both light limitation and host density limitations controlled chytrid growth. In laboratory studies, Bruning (1991) showed significant decrease in chytrid growth, identified as number of zoospore released, when infecting light limited diatoms. In addition, decreased host density also led to a reduction in zoospores production. They suggested that due to limited internal stores chytrid zoospore can quickly exceed their energy stores, such that there might have been as much as a 50% loss in zoospores due at low host densities, due to inability to locate hosts before energy reserves are exhausted.
Therefore, the combination of low cell density, low light, and temperature restrictions as well as internal oligopeptide stores have the potential to form a cohesive defensive system that allows Planktothrix to form seasonal and depth gradient refuges by niche differentiation. The ability to take advantage of restrictions on parasitism in the metalimnion could be an important advantage to Planktothrix.
Gas vesicles present in Planktothrix allow for light-dependent movement within the water column. When the light is limited to a lake, these vesicles allow Planktothrix to float to the surface. The reverse is also true, and Planktothrix sink deeper into the water column when light increases (Walsby, 2005). Due to diurnal and seasonal light fluctuations, filaments do not remain stable in the water column but continually modify their location depending on light conditions. The filament size controls the rate of movement, dependent on length and width, allowing larger filaments to cover more distance than smaller filaments. This allows some advantages to the larger, faster repositioning filaments, and in particular, allowing the filaments to move from the epilimnion to the metalimnion in a shorter time frame.
This ability to move up or down in the water column dependent on light can lead to increased niche potential and the ability to escape potential parasitic pressures. Increased light penetration could allow sufficient increases in niche space and, when linked with decrease in parasitism, would allow for increasing Planktothrix community diversity. It was shown in work by De Bruin et al. (2008) that chytrid fitness was limited by increasing host diatom diversity. This could mean that if increased niche space led to a larger number of co-existing Planktothrix chemotypes, this increased diversity could constitute an increased defense against chytrid parasitism. Protection of Planktothrix against chytrid parasitism by chemotype diversity has not yet been demonstrated experimentally. However, paleolimnological studies have indicated that for certain periods of time, chemotype diversity seems to be an effective defense against chytrids (Kyle et al., 2015b).
It is possible that Planktothrix communities can take advantage of the metalimnion to increase nutrient availability, decrease competition for nutrients, as well as to reduce the effects of parasitism. However, the metalimnic niche is temporary due to seasonal cycles of temperate lakes. An increase in chemotype diversity, developed in the metalimnion, could act as an epilimnetic defense against parasitism that is more active in the warmer surface layers. If chemotypes are allowed a refuge within which to build biomass, then Planktothrix diversity could become stable and act as a defense against a natural parasite.
Sediment DNA provides an archive that can be amplified with short species-specific primers to reconstruct historical ecological data. This research has the potential to reconstruct historical chemotype variability back to time periods when trophic states were in flux and methods for chemotype detection were not yet available. Our findings compare across specific chemotype populations in seven Norwegian lakes and indicate an increase in Planktothrix chemotype diversity associated with decreasing surface chl a (0–4 m). We suggest that Planktothrix in the epilimnion could utilize diverse chemotype specific oligopeptide profiles as defensive systems against predators. However, with increased light penetration, this might allow Planktothrix to migrate into the metalimnion. This lower temperature environment is likely to result in a refuge from chytrid predation due to a mismatch of environmental conditions between Planktothrix chemotypes and chytrid predators while increasing nutrient availability and competition. Our data suggests that the metalimnetic niche expansion allows for increased chemotype diversity.
The authors declare that the research was conducted in the absence of any commercial or financial relationships that could be construed as a potential conflict of interest.
This work was supported by internal grants from Norwegian Institute for Water Research (NIVA) for SH, MK, and TR as well as internal grants from the Norwegian University of Life Sciences (NMBU) for MK and TR. We also thank Veronika Ostermaier and Jørn Sønstebø for preliminary molecular development of Planktothrix chemotype primers and sequencing and Vladyslava Hostyeva for assistance with cultures from the NIVA Culture Collection of Algae (NIVA-CCA).
The Supplementary Material for this article can be found online at: http://journal.frontiersin.org/article/10.3389/fevo.2015.00104
Data Sheet 1. Decay corrected 137Cs (dpm/g) determinations for each centimeter of each lake core (centimeters from surface) for the seven lakes in this analysis with resulting identification of year depending on the identification of the 137Cs peak indicating the 1986 fallout due to the Chernobyl nuclear accident.
Data Sheet 2. Table reporting the year, the layer, percentage of dry mass, and the organic material found for each core of the seven lakes used in this study. DNA concentrations, normalized by organic content, by lake and year, based on qPCR determination of unknowns using chemotype specific standards.
Presentation 1. Text file (oligopeptides.txt) and R script (Heat map R script) used to analyze variations and similarities between the oligopeptide profiles of the four common Planktothrix chemotypes reported by Rohrlack et al. (2009) (their Figure 2).
Presentation 2. The correlation of chemotype numbers compared to monitoring data, including chl a (chlorophyll a in top four meters), TN (μg L−1, total nitrogen), TP (μg L−1, total phosphorus), area (km2, lake area), max.depth (m, maximum depth), watershed area (km2, watershed area), and sed. rate (mm y−1, sedimentation rate).
Agha, R., and Quesada, A. (2014). Oligopeptides as biomarkers of cyanobacterial subpopulations. Toward an understanding of their biological role. Toxins 6, 1929–1950. doi: 10.3390/toxins6061929
Bruning, K. (1991). Infection of the diatom Asterionella by a chytrid. I. Effects of light on reproduction and infectivity of the parasite. J. Plankton Res. 13, 103–117. doi: 10.1093/plankt/13.1.103
De Bruin, A., Ibelings, B. W., Kagami, M., Mooij, W. M., and Van Donk, E. (2008). Adaptation of the fungal parasite Zygohizidium planktonicum during 200 generations of growth on homogeneous and heterogeneous population of its host, the diatom Asterionella formosa. J. Eukaryot. Microbiol. 55, 69–74. doi: 10.1111/j.1550-7408.2008.00306.x
DeMott, W. R., Zhang, W. X., and Carmichael, W. W. (1991). Effects of toxic cyanobacteria and purified toxins on the survival and feeding of a copepod and three species of Daphnia. Limnol. Oceanogr. 36, 1346–1357. doi: 10.4319/lo.1991.36.7.1346
Gsell, A. S. (2013). The Host, the Parasite and their Environment: How Parasitism and Environmental Variation can Maintain Host Genetic Diversity. Ph.D. thesis, Utrecht University, Utrecht.
Haande, S., Hagman, C., and Skogan, O. A. S. (2013). Monitoring in Lake Gjersjøen and Lake Kolbotnvannet and their Tributaries 1972–2013. NIVA Report 6661-2014, Oslo.
Halstvedt, C. B., Rohrlack, T., Andersen, T., Skulberg, O., and Edvardsen, B. (2007). Seasonal dynamics and depth distribution of Planktothrix spp. in Lake Steinsfjorden (Norway) related to environmental factors. J. Plankton Res. 29, 471–482. doi: 10.1093/plankt/fbm036
Holm, S. (1979). A simple sequentially rejective multiple test procedure. Scandinavian J. Stat. 6, 65–70.
Kurmayer, R., and Gumpenberger, M. (2006). Diversity of microcystin genotypes among populations of the filamentous cyanobacteria Planktothrix rubescens and Planktothrix agardhii. Mol. Ecol. 15, 3849–3861. doi: 10.1111/j.1365-294X.2006.03044.x
Kyle, M., Haande, S., Ostermaier, V., and Rohrlack, T. (2015b). The red queen race between parasitic chytrids and their host, Planktothrix: a test using a time series reconstructed from sediment DNA. PLoS ONE 10:e0118738. doi: 10.1371/journal.pone.0118738
Kyle, M., Haande, S., Sønstebø, J., and Rohrlack, T. (2015a). Amplification of DNA in sediment cores to detect historic Planktothrix occurrence in three Norwegian lakes. J. Paleolimnol. 53, 61–72. doi: 10.1007/s10933-014-9807-1
Mittelbach, G. G., Schemske, D. W., Cornell, H. V., Allen, A. P., Brown, J. M., Bush, M. B., et al. (2007). Evolution and the latitudinal diversity gradient: speciation, extinction and biogeography. Ecol. Lett. 10, 315–331. doi: 10.1111/j.1461-0248.2007.01020.x
Molversmyr, A. (2006). International Research Institute of Stavanger Hålandsvatnet Tilførselsberegninger. Stavanger: Raport IRIS 2006/134.
Molversmyr, A. (2010). International Research Institute of Stavanger Hålandsvatnet Tilførselsberegninger. Stavanger: Raport IRIS 2010/146.
R Core Team. (2014). R: A language and environment for statistical computing. Version 3.1.1. R Foundation for Statistical Computing. Vienna. Available online at: http://www.R-project.org/
Rohrlack, T., Christiansen, G., and Kurmayer, R. (2013). Putative antiparasite defensive system involving ribosomal and nonribosomal oligopeptides in cyanobacteria of the genus Planktothrix. Appl. Environ. Microbiol. 79, 2642–2647. doi: 10.1128/AEM.03499-12
Rohrlack, T., Edvardsen, B., Skulberg, R., Halstvedt, C. B., Utkilen, H. C., Ptacnik, R., et al. (2008). Oligopeptide chemotypes of the toxic freshwater cyanobacterium Planktothrix can form subpopulations with dissimilar ecological traits. Limnol. Oceanogr. 53, 1279–1293. doi: 10.4319/lo.2008.53.4.1279
Rohrlack, T., Skulberg, R., and Skulberg, O. M. (2009). Distribution of oligopeptide chemotypes Planktothrix and their persistence in Fennoscandia. J. Phycol. 45, 1259–1265. doi: 10.1111/j.1529-8817.2009.00757.x
Sogge, H., Rohrlack, T., Rounge, T., Sønstebø, Y., Tooming-Klunderud, A., Kristensen, T., et al. (2013). Gene flow, recombination, and selection in cyanobacteria: population structure of geographically related Planktothrix freshwater strains. Appl. Environ. Microbiol. 79, 508. doi: 10.1128/AEM.02417-12
Sommer, U., Adrian, R., De Senerpont Domis, L., Elser, J. J., Gaedke, U., Ibelings, B., et al. (2012). Beyond the Plankton Ecology Group (PEG) model: mechanisms driving plankton succession. Rev. Ecol. Evol. Syst. 43, 429–448. doi: 10.1146/annurev-ecolsys-110411-160251
Sommer, U., Gliwicz, Z. M., Lampert, W., and Duncan, A. (1986). The PEG model of seasonal succession of planktonic events in fresh waters. Arch. Hydrobiol. 106, 433–471.
Sønstebø, J. H., and Rohrlack, T. (2011). Possible implications of chytrid parasitism for population subdivision in freshwater cyanobacteria of the genus Planktothrix. Appl. Environ. Microbiol. 77, 1344–1351. doi: 10.1128/AEM.02153-10
Tomming-Klunderud, A., Sogge, H., Rounge, T. B., Nederbragt, A. J., Lagesen, K., Glöckner, G., et al. (2013). From green to red: horizontal gene transfer of the phycoerythrin gene cluster between Planktothrix strains. Appl. Environ. Microbiol. 79, 6803–6812. doi: 10.1128/AEM.01455-13
Walsby, A. E. (2005). Stratification by cyanobacteria in lakes: a dynamic buoyancy model indicates size limitations met by Planktothrix rubescens filaments. New Phytol. 168, 365–376. doi: 10.1111/j.1469-8137.2005.01508.x
Welker, M., Christiansen, G., and von Döhren, H. (2004). Diversity of coexisting Planktothrix (cyanobacteria) chemotypes deduced by mass spectral analysis of microcystins and other oligopeptides. Arch. Microbiol. 182, 288–298. doi: 10.1007/s00203-004-0711-3
Keywords: Planktothrix chemotypes, sediment DNA, chytrids, diversity, gene flow, dispersal
Citation: Kyle M, Andersen T, Haande S and Rohrlack T (2015) Historical Planktothrix diversity across seven Norwegian lakes implies environmentally driven niche differentiation. Front. Ecol. Evol. 3:104. doi: 10.3389/fevo.2015.00104
Received: 13 April 2015; Accepted: 24 August 2015;
Published: 08 September 2015.
Edited by:
Gael Le Roux, Centre National de la Recherche Scientifique, FranceReviewed by:
Jeffery Robert Stone, Indiana State University, USACopyright © 2015 Kyle, Andersen, Haande and Rohrlack. This is an open-access article distributed under the terms of the Creative Commons Attribution License (CC BY). The use, distribution or reproduction in other forums is permitted, provided the original author(s) or licensor are credited and that the original publication in this journal is cited, in accordance with accepted academic practice. No use, distribution or reproduction is permitted which does not comply with these terms.
*Correspondence: Marcia Kyle, Environmental Sciences, Norwegian University of Life Sciences, Universitetstunet 3, 1430 Ås, Norway,bWFyY2lhLmt5bGVAbm1idS5ubw==
Disclaimer: All claims expressed in this article are solely those of the authors and do not necessarily represent those of their affiliated organizations, or those of the publisher, the editors and the reviewers. Any product that may be evaluated in this article or claim that may be made by its manufacturer is not guaranteed or endorsed by the publisher.
Research integrity at Frontiers
Learn more about the work of our research integrity team to safeguard the quality of each article we publish.