- Department of Bioscience, Aarhus University, Aarhus, Denmark
Permanently-social spiders share a common suite of traits, including cooperative foraging and brood care, elimination of pre-mating dispersal, and the transition to an inbreeding mating system. Social spiders are confined to tropic and subtropical habitats, suggesting environmental constraints on the evolution of group living in spiders. Because, social spider groups are sedentary and dependent on arrival of insect prey in their capture webs, group living and the associated higher local density is expected to rely on a relatively resource rich environment. We used spatial statistical modeling to explore environmental factors underlying the macro-ecological patterns in the distribution and diversity patterns of social spiders. We found strong support for habitat productivity as a predictor of the distribution of social species, particularly in the Old World. We show that social species are restricted to more productive habitats relative to a set of closely related subsocial sister species with a solitary lifestyle. Within their distribution range, social species richness was higher where precipitation seasonality is lower. These macro-ecological patterns corroborate the underlying biological hypotheses that evolution of group living is facilitated in environments that provide more abundant insect prey and a more continuous supply of food resources.
Introduction
Cooperation includes a wide diversity of traits, for example predator avoidance by warning signals or nest defense, resource acquisition through harvesting, communication or cooperative hunting, or cooperative breeding through provisioning of reproductive females or offspring (Dugatkin, 1997; Davies et al., 2012). Understanding the evolution of sociality and cooperation is a long-standing challenge, not least because both immediate direct benefits and genetic indirect benefits of cooperation may depend on ecological context and therefore vary with different environmental and ecological conditions. For example, cooperative breeding in birds is favored when ecological conditions are harsh and individual reproduction constrained, whereas individuals disperse and attempt own reproduction under benign conditions (Emlen, 1982, 1994). Our understanding of these relationships are, further challenged by a complex set of interactions between life history and environment on the probability of group formation and degree of social organization (Hatchwell and Komdeur, 2000). Geographical patterns of variation in the level of sociality has been documented in a number of social lineages, suggesting a strong influence on ecology, and environment on the evolution and complexity of cooperation, e.g., in social insects (Wilson, 1975; Kaspari and Vargo, 1995; Wcislo, 1997; Schwarz et al., 2007; Gunnels et al., 2008; Kaspari and Weiser, 2011; Kocher et al., 2014), social spiders (Avilés et al., 2007; Purcell, 2011; Majer et al., 2013a), and cooperatively breeding birds (Jetz and Rubenstein, 2011). While these patterns show altitudinal or latitudinal and thereby ecological correlations with degree of sociality, nest size or other social traits, our understanding of the causal relationships between ecological factors and social traits is incomplete.
In a study incorporating variation in ecological conditions into social evolution theory, Van Dyken and Wade (2012) point out that various social traits differ in their ecological function depending on the type of benefits they confer (fecundity/survival/resource acquisition benefits). Given that different ecological roles of cooperative traits are likely to influence selection is different ways, they show how different modes of cooperation evolve under distinct ecological conditions. Traits that contribute to resource acquisition (e.g., provisioning, group hunting, communicating location of food) evolve more readily under strong local competition among social partners, for example when resources are limited, or fecundity and/or survival causes high local crowding. In contrast, altruistic traits that confer survival and/or fecundity benefits, i.e., nest defense or worker altruism, are favored when conditions are reversed and there are abundant resources and weak local competition. If different modes of cooperation evolve under distinct ecological conditions, this framework may provide a useful way of examining the evolution of cooperative traits in different ecological settings (Van Dyken and Wade, 2012).
In the present study, we aim to explore the ecological conditions under which sociality and cooperation in spiders may evolve. We do this by determining the relationship between environmental factors and global geographic distribution of cooperative spiders, and relating the ecological factors that determine the distribution of social spiders to hypotheses of social evolution (Lubin and Bilde, 2007). Permanent cooperation in spiders is rare and known in only approximately 25 of almost 46.000 extant described species (World Spider Catalog, 2015). Interestingly, among these there are at least 20 independent origins of sociality distributed across seven families, and most species show remarkable convergent evolution of a suite of traits associated with their social life style (Lubin and Bilde, 2007; Bilde and Lubin, 2011). Social spiders live in permanent groups and cooperate in web building, prey capture, and brood care. The transition to permanent group-living involves complete elimination of pre-mating dispersal, which results in mating among siblings (family groups) in an obligatory inbreeding mating system. Groups show a female biased primary sex ratio, and new colonies are founded by adult mated females and her offspring. Furthermore, all social species are geographically restricted to subtropical and tropical environments (Avilés, 1997; Agnarsson et al., 2006; Johannesen et al., 2007). The combination of this distribution pattern and the multiple origins of convergent traits suggests that, common ecological factors may underlie or facilitate permanent sociality in spiders.
Benefits of cooperation is spiders appear to be associated with resource acquisition in the form of insect prey (Nentwig, 1985; Ward, 1986; Guevara and Aviles, 2007; Majer et al., 2013b). Social spiders live in sedentary colonies, and as sit-and-wait predators they are entirely dependent on the arrival of insect prey in their capture webs to secure sufficient food to meet demands of higher local densities. This implies that a certain supply of prey in the environment must be present for groups to form (Parrish and Hamner, 1997; Jarvis et al., 1998). Empirical evidence suggests that local competition increases with group size, and that the balance between improved survival but decreased fecundity with increasing group size results in highest fitness (per capita lifetime reproductive success) at intermediate group size (Avilés and Tufiño, 1998; Bilde et al., 2007). Traits that increase capture success, for example cooperative web building and prey capture, or cooperative brood care that facilitates provisioning of young, may be favored under these conditions. The social spider system may therefore follow the predictions of a limited resources situation for the evolution of cooperative traits that contribute to resource-enhancement or resource efficiency (Van Dyken and Wade, 2012).
We collected presence/absence data on a world-wide set of 21 social spider species to explore environmental factors that restrict the distribution of social spider lineages to the subtropics and tropics and identify ecological determinates of their limited distribution ranges. In addition, we performed spatial analysis of species diversity of social species. Comparative spatial analyses of closely related social and solitary sister species in the two most species rich genera, Stegodyphus and Anelosimus (Majer et al., 2013a,b), suggest that social species are confined to lower latitudinal gradients relative to their solitary and subsocial sister species. To corroborate this finding, we also performed spatial analysis of the distribution (presence/absence) on a data set of seven solitary sister species to social species of Stegodyphus and Anelosimus (Supplementary Material). We formulated three not mutually exclusive hypotheses relating to habitat productivity (prey abundance) or seasonality (prey constancy or constant environment), to identify environmental predictors of the distribution of social species.
The Productivity Hypothesis
Social spiders are restricted to the most productive habitats that support more abundant prey. The sedimentary nature of groups of social spiders imply that competition for food resources are high and therefore group living can only evolve in relatively productive habitats. Arthropod biomass correlates positively and linearly with plant biomass and diversity (Borer et al., 2012), therefore, we explore the productivity hypothesis by quantifying the primary productivity of social spider habitats. Within the habitat range of social spiders, we expect areas of higher productivity (Majer et al., 2013a,b) to be more species rich. As a proxy for habitat productivity, we used a globalized difference vegetation index (GVI).
Low Temperature Seasonality Hypothesis
Social spiders are restricted to areas with low temperature seasonality. This prediction is consistent with the hypothesis that less seasonal (more constant) environments favor overlapping generations, which increases opportunities for parental, or sibling care of offspring and may be important for acquiring permanent sociality (Avilés, 1997; Field et al., 2010). The rationale is that the activity seasons of tropical species tend to be longer and the percentage of species active around the year higher, relative to their counterparts at higher latitudes (Wolda, 1988). Within the tropics the trend continues from areas with a pronounced dry season toward the relatively non-seasonal areas. Low seasonality furthermore secures a continuous supply of insect food (Riechert, 1985), which in turn facilitates delayed dispersal from the maternal nest consistent with the subsocial route to permanent sociality (Avilés and Gelsey, 1998; Bodasing et al., 2002; Bilde and Lubin, 2011).
Low Precipitation Seasonality Hypothesis
Social spiders are restricted to environments with continuous growing seasons, determined by low precipitation seasonality. The assumption is, that low precipitation seasonality favors continuity of prey supply, since the phenology of insects may correlate with precipitation patterns—i.e., seasonality in insect abundance is reduced when precipitation seasonality is low (Janzen and Schoener, 1968). We expect higher species richness in areas of lower precipitation seasonality (Majer et al., 2013b).
Methods
Social Spiders
Permanent group living in spiders is considered to have evolved through the subsocial route via the loss of premating dispersal and evolution of cooperative breeding, where non-reproducing sisters in the colony take care of the offspring (Avilés, 1997; Bilde and Lubin, 2011). Permanently social species share a number of traits, including the loss of juvenile dispersal, intra-colony mating, and therefore a continuously inbreeding mating system, a primary female-biased sex ratio, and the establishment of new colonies by postmating propagule dispersal of already mated females (Lubin and Bilde, 2007; Lubin, 2010).
We performed a macro-ecological analysis exploring environmental predictors of sociality in a comprehensive data set of 21 social spider species. Three species do not conform to the most stringent definition of permanently social species (Table 1) as they either do not cooperate in foraging (Diaea ergandros and D. socialis) or do not have highly female biased sex-ratio (Tapinillus sp.), while they share the other characteristics of the social species (Lubin and Bilde, 2007). To ensure that that these three species would not alter any conclusions, we ran a parallel analysis on a reduced data set excluding these three species (N = 18).
To investigate the hypotheses outlined above, we analyzed how species richness of social spiders correlates with environmental variables. We collected presence data for all species from the World Spider Catalog (World Spider Catalog, 2015). Based on the presence of each of the species at the country level, we established a species richness map. Species richness was estimated at the level of “botanical countries,” TDWG level 3 (International Working Group on Taxonomic Databases) (Brummit, 2001), which constituted 339 units in our dataset. “Botanical countries” are units based on the floristic composition of continental regions, which for the most part overlap with the political country borders, except for the largest countries (incl. amongst others Australia, Brazil, South Africa). To account for possible historical climate influences, we divided these botanical countries intro Old World countries (Euroasia and Africa), and New World countries (Americas, Australia and Oceania).
Environmental Predictors
Several climatic variables were obtained from the CliMond dataset (Kriticos et al., 2011). Annual or quarterly means of climatic variables in the dataset were derived from monthly temperature and rainfall (precipitation in mm) values measured at various places in the world in the period 1960–2000. The following variables were included in the analyses: the GVI for the productivity hypothesis, temperature seasonality for the constant environment hypothesis, and precipitation seasonality for the continuous food supply hypothesis. GVI is a measure of the mean annual global Normalized Difference Vegetation Index (NDVI), the most common measurement of the density of plant growth (obtained by the EDIT Geoplatform (Lobo, 2007). NDVI is derived from satellite images over the entire globe over a period of 18 years (1982–2000). Original NDVI real values (from −1 to +1) were rescaled to a range from 1 to 255 (byte format). A yearly average (GVI) was computed for NDVI by averaging the monthly means. For this, cell statistic function in Spatial Analyst was used, setting cell size and extent to one of the monthly layers (NCDC Satellite Data Services Division, 1985-1988). Temperature seasonality was calculated as the coefficient of variation of the temperature monthly means (Kriticos et al., 2011). Likewise, precipitation seasonality was calculated as the coefficient of variation of the precipitation monthly means. All the environmental layers used were in 10′ resolution (18.5 km at the equator).
Statistical Analyses
We analyzed the distribution patterns of permanently-social spider species by using binary logistic regression (non-spatial models), and subsequently accounting for spatial autocorrelation with an auto covariate (spatial models) (Dormann, 2007). Some predictor variables were transformed to obtain normal distribution of residuals (Table 3). Within analyses, variables were not correlated (Spearman's r <0.2; Table S1).
Since, spatial autocorrelation was present in the residuals of closest distance classes, we used an auto-covariate in the set of models that accounted for spatial patterns (spatial models). This is a distance-weighted function of response values in neighbor cells (Smith, 1994). It was computed with maximum neighbor distance of the centroid geographical coordinates of the TDWR botanical countries, with weighting by inverse distance. We then used binary logistic regression with the Akaike Information Criterion for model selection (Johnson and Omland, 2004), choosing models predictors by stepwise selection. The starting model was always the one including area and the categorical variable for Old/New World. Two nested sets of models were run, based either on all 21 spp., or the 18 “truly social” spp (Table 1). We first contrasted areas with one or more social spider species with areas without any social spider species. We subsequently contrasted areas with two or more social spider species with areas with just one social spider species. Very few areas have more than two social spider species, therefore species richness could not be analyzed as a continuous or ordinal variable. After selecting the models and calculating the Akaike weights for each, we computed the multi-model coefficients for each variable of interest. These are products of the standardized coefficient of predictor from each model and the Akaike weight of the same model. From multi-model coefficients we further computed the odds ratios, to determine how much the probability of 1 (or binary presence/ presence of 2+ species) changes with one unit of change of the variable for each odds calculated (Agresti, 2002). Explanatory power of the models was estimated using R2 adjusted by the maximum R2 achievable for the data (Nagelkerke, 1991). The analyses were done in SAM 4.0 (Rangel et al., 2010) and R 2.13.2 (R Development Core Team, 2011). The graphs were plotted in R.
In both data sets we found very similar results for presence/absence and species richness (full data set of 21 species, and reduced data set of 18 species). We therefore only report the analyses for the 21 species in the main text, results from the reduced data set can be found in the Supplementary Material (Table S1).
Results
Our study formally establishes the global distribution of permanently-social spiders in subtropical and tropical environments (Figure 1). Out of the 335 botanical regions, 50 had one or more social spider species occurring across the territory. Twenty four countries had one social species, while 26 countries had two or more social species. The maximum number of social species (n = 6; or 5 when excluding the “atypical” social Tapillinus spp.) was found in Ecuador (Figure 1).
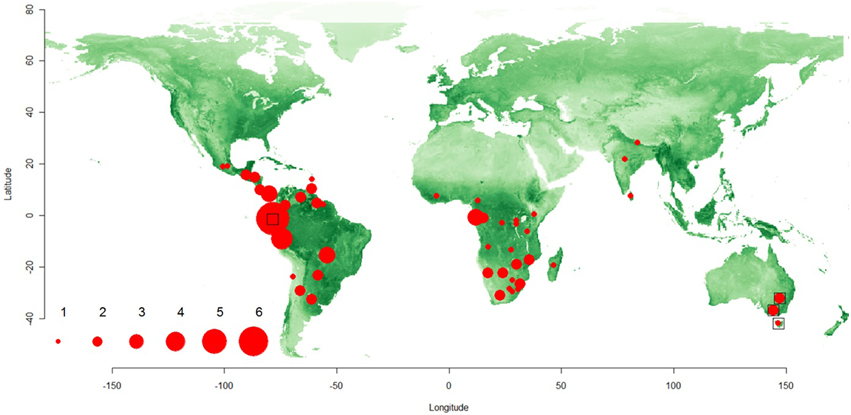
Figure 1. Map of the world showing the species richness of social spiders. The species richness patterns are denoted by filled red circles, whose size represented the species richness in the area (see scale from 1 to 6). The distribution of three “atypical” social species (see Table 1), which were excluded from one part of the analyses, are shown with empty square symbols. The green color represent the global gradient of GVI-values. The symbols were plotted at the lat-long centroids for each botanical country where the social spiders occur (see Methods for details).
The only environmental predictor for the global presence-absence of social spiders with strong support was GVI (Table 2). This implies that social spiders globally occur in more productive environments (Figure 2). However, results differ between the Old and New Worlds, in the Old World, the savannah and not the rainforest is the most species rich with the social species confined to the most productive savannah biome. In the New World, the occurrence of social spiders are in rainforests as the most productive biome. This finding was supported by both the non-spatial and the spatial models (Table 2). Notably, not just the GVI main effect, but also the GVI × Region interaction received strong support. GVI was best supported in both sets of models, and had a much stronger effect on the probability of occurrence of social spiders in the Old World (best modeled with as a quadratic function of GVI, Table 4). This effect of GVI could be attributed to the differences in the proportional occurrences of different species of social spiders across the Old and New World habitats (Figure 3). This effect was much stronger in the non-spatial models without the auto-covariate (Table 4). We cannot rule out that our results could be partly biased by differences in sampling effort between regions/countries, i.e., whether the higher species richness in Ecuador might reflect more intense sampling in this region. Therefore, we repeated the species richness modeling excluding the data from the most species rich region, Ecuador, this analyses reproduced and therefore corroborates our main results (Table S8 in the Supplementary Material).
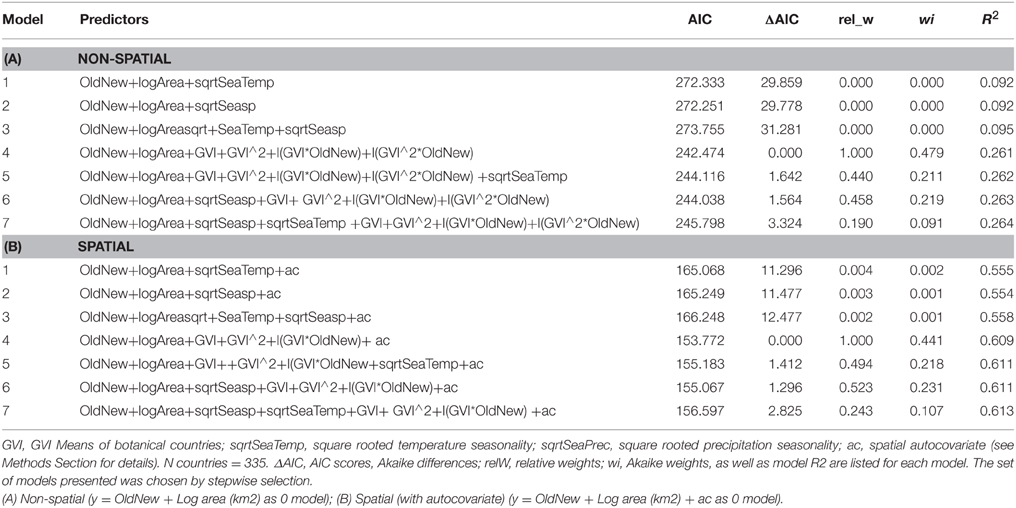
Table 2. Binary logistic regression model summaries and model performance across the data set of presence vs. absence of social spiders (21spp. in 335 botanical countries).
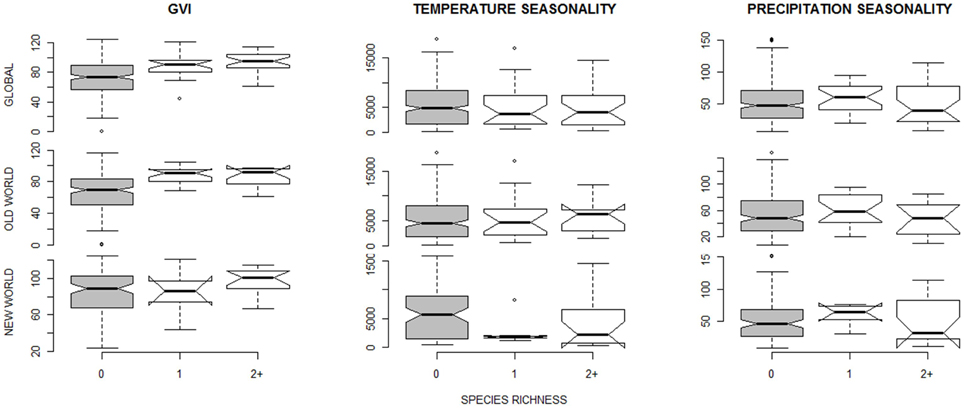
Figure 2. Boxplots of environmental predictors (mean value computed for each botanical country) for species richness equalling zero (gray bars), one or two species. The data are shown for species richness of 21 social spider species (Table 1); for the 18 “true” permanently-social species data see Figure S1 in Supplementary Material. The extremes, the inter-quartile range, and the medians are shown. The top panels show the pooled data, and below the same predictor separated into Old World (middle panels) and New World (lower panels) data sets.
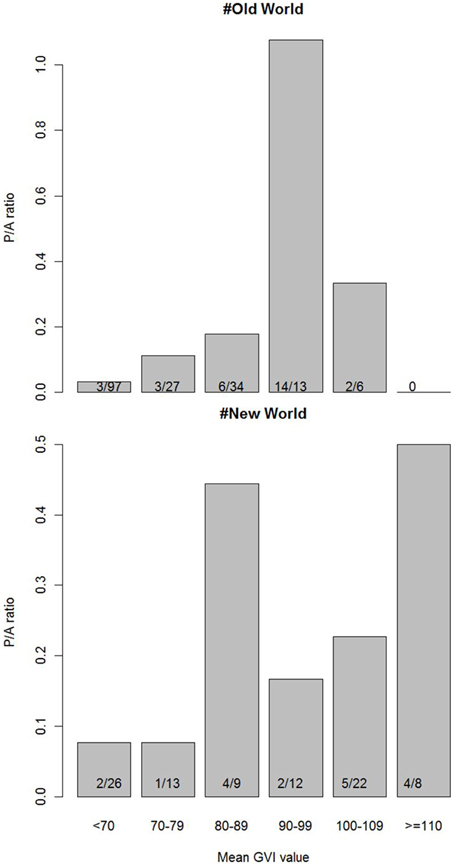
Figure 3. Frequency of occurrence of social spiders across the countries in the Old and New World on the gradient of GVI. Numbers inside the bars are counts of botanical countries with presence/absence of social spiders within the corresponding mean GVI value range. The ratio is higher than one if the number of botanical countries with presence is higher than the number of countries where social spiders are absent, within a given range of GVI values.
In addition, we looked at global predictors of occurrence of solitary living sister species to the New World Anelosimus and Old World Stegodypus spiders (Table S5 in the Supplementary Material). Both sets of models, spatial and non-spatial, showed that subsocial spiders in the genera are constrained to more productive areas (Tables S6, S7 in the Supplementary Material), with productivity being the only supported predictor of their occurrences. However, these effects were much weaker than the predictive power of productivity for the occurrence of social spiders (Table 4), suggesting that resource availability (productivity) indeed is a main global driver of occurrence and distribution patterns of social lineages. Notably, there was no differential effect of productivity as a predictor of subsocial spiders' occurrences in the Old vs. New World. Unlike social Anelosimus spiders, that occur only in the Neotropical rain forests, American subsocial species in the genus occur more north and in much drier environments (e.g., in Mexico).
Where are Social Spiders Most Species Rich within their Overall Range?
The best supported predictor of co-occurrence of two or more social spiders species relative to the occurrence of single species was precipitation seasonality (Table 3), which had a negative effect on the probability of occurrence of two or more species relative to just one species (Table 4 and Figure 2). In other words, two or more social species occurred in areas with lower precipitation seasonality. Again, this finding was supported by both the non-spatial and the spatial models (Table 3).
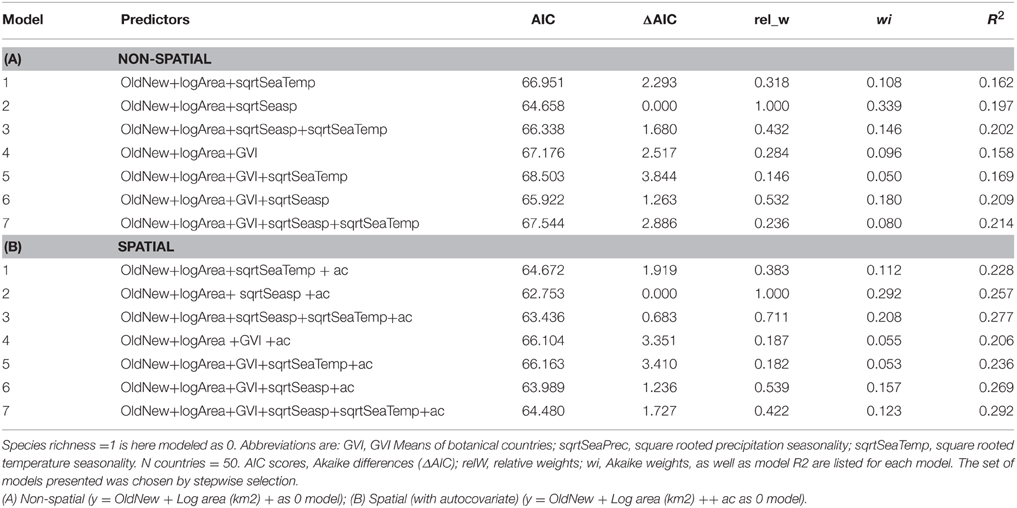
Table 3. Binary logistic regression model summaries and model performance contrasting areas with one social spider spp. with those of two or more spp. (21 spp. from 50 botanical countries included, maximum species richness = 6).
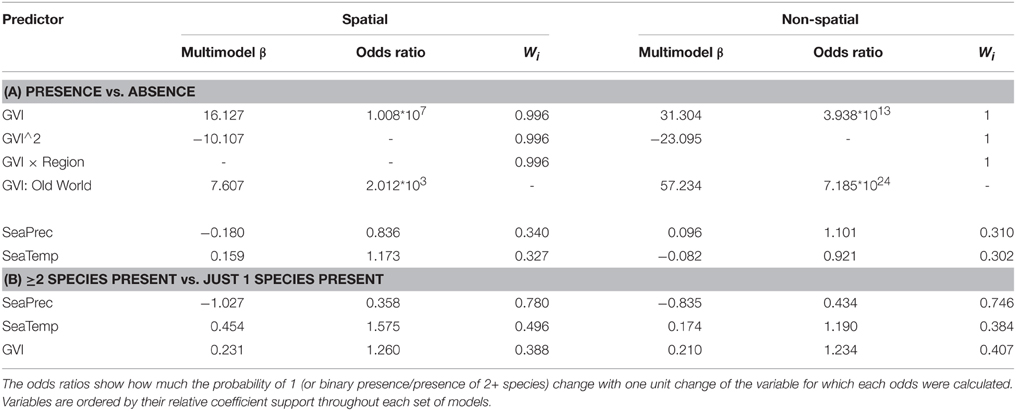
Table 4. Multi-model coefficient estimates β, odds ratios and the coefficient support (Wi, sum of Akaike weights for models including this factor) of all the binary logistic models (Tables 2, 3) containing each of the three environmental variables representing our hypotheses for social spiders' species richness.
Discussion
We analyzed the global patterns of occurrence and species richness of social spiders in relation to the hypotheses of habitat productivity and climatic stability as ecological predictors of social species' distribution ranges. We found strong support for the effect of habitat productivity on the distribution of social spiders. Within the overall range of social spiders, precipitation seasonality was the best supported predictor of species richness (two or more species), i.e., there was a tendency for higher species richness in areas of lower precipitation seasonality. This means that permanently social spiders occur in areas of high habitat productivity, with relatively high species richness concentrated within this range where precipitation is less seasonal. The permanently social species are derived from solitary subsocial sister species (Johannesen et al., 2007), but occupy more limited distribution ranges at lower latitudes and relatively more productive habitats than their subsocial sister species (Majer et al., 2013a,b). Our analyses of seven subsocial sister species confirms this pattern, which suggests that relative to the distribution ranges of close relatives with a solitary life style, the permanently social and derived species are confined to the most productive areas. More productive habitats support higher abundance of prey (Clarke and Gaston, 2006; Majer et al., 2013b) and/or higher abundance of larger prey size (Borer et al., 2012). Food availability is an important ecological driver of group formation (Davies et al., 2012), and has been hypothesized as a main factor restricting social spider species to tropical regions (Nentwig, 1985). Our results support the productivity hypothesis suggesting that food resources is an important driver for the evolution and maintenance of permanent group living.
Latitudinal gradients of decreasing species richness from tropical to extratropical areas is a well-established pattern in biogeography (Pianka, 1966; Willig et al., 2003; Hillebrand, 2004). Our results show increasing diversity of social spider species at lower latitudes and thus, corroborates this pattern, therefore we cannot rule out that higher species richness of social spiders at lower latitudes is a by-product of general higher diversity in these areas. An analyses including distribution ranges of a larger number of solitary species within the social genera would be needed for a more conclusive analysis of the bio-geographical patterns underlying the distribution of social spider species. Nevertheless, while our analyses provided support for the effect of productivity on the occurrence of social spiders on a global scale, there are differences between the New and Old World (Figure 3), and our finding that social species are restricted to lower latitudes relative to their solitary sister species provides confidence in our interpretation. The quadratic relationship in the Old World can be contributed to the occurrence of single social Agelena spp. taxon in central African rainforests (i.e., very productive habitats) vs. several social Stegodyphus spp. in the African dry savannah habitats (i.e., less productive habitats). The differences in the effect of productivity gradients between the Old and New World may reflect the major differences in the ecology of the two most species rich genera, New World Anelosimus and Old World Stegodyphus, where the former occur in the most productive continental biomes, tropical rainforests while the latter occupy drier savannah habitats (Figure 1). Nevertheless, within the distribution range of subsocial Stegodyphus sister species, we find the social derived species in lower latitudes and more productive areas (Majer et al., 2013b). A similar pattern applies to the New World Anelosimus species (Majer et al., 2013a), however, for Anelosimus spiders there was a strong combined effect of productivity with other environmental gradients, in particular precipitation seasonality that influenced the social species' distribution ranges. Therefore, to the extent that we can take the evolutionary relationships of social and subsocial species into account, our analyses confirm the importance of habitat productivity for maintaining sedentary groups of permanently social species.
Within the distribution of social spiders, species richness of social spiders is higher where precipitation seasonality is low, i.e., in aseasonal productive environments such as tropical rain forests. This finding is consistent with the hypothesis that permanent group living is favored not only where the prey supply is relatively high, but also where prey is continuous throughout the seasons. Precipitation patterns have been shown to influence the phenology of insect populations. A set of field studies in the tropical mainland and islands showed that insect populations increased in abundance where rain patterns were more constant (Janzen and Schoener, 1968; Janzen, 1973; Wolda, 1978). This implies that food supply for spiders is also more constant in such areas. The negative effects of precipitation seasonality on species richness inside the tropics might be driven by data on Anelosimus species across the Americas, which have overlapping generations and year-round colony productivity, and which are also the most abundant species in the dataset. Nevertheless, there was no significant effect of the interaction term of Old/New World categorical variable with precipitation. The pattern therefore holds regardless of the fact that the majority of species in the data set are social theridiids (Anelosimus and Parasteatoda spp., Table 1), which occur in the tropical rainforest areas of the Americas and Papua New Guinea.
The lowlands of Amazonian Ecuador harbour six social spiders in total, thus being the most species-rich area (Figure 1). The six species include three Anelosimus spp., 1 dictynid species (Table 1), while the 6th one is the “atypical” social oxyiopid Tapinillus sp. Notably, this is where most of the social theridiid species occur, namely those of the genus Anelosimus. There is a latitudinal pattern of sociality in this genus, with more social species at lower latitudes, which is also paralleled in the altitudinal gradient, as the social species rarely occur above 1500 m (except for A. gucamayos that occurs up to 2000 m) (Avilés et al., 2007). Interestingly, there is also an altitudinal gradient in colony size for two social Anelosimus species (Avilés et al., 2007). Unlike the ants, where colony size increases with latitude (Kaspari and Vargo, 1995), the opposite seems to appear in the spider genus with the most social species. Two patterns have been suggested for these distributional phenomena of social traits in the genus Anelosimus: absence of subsocial congeners in the lowland tropical rainforests, and absence of social congeners in the highlands (Avilés et al., 2007). The authors suggested the prey size and maternal survival contribute to the occurrence and survival of social lineages in the lowlands, where the prey is sufficiently large in size, and where heavy rains affect the spiders in such a way that the survival of offspring is higher in multi-female and cooperative nests. The macro-ecological correlates of sociality in the Anelosimus genus lend support for these biological hypotheses (Majer et al., 2013a), and are in agreement with the results supporting productivity as the most important ecological predictor of the global distribution of social spiders reported here.
We hypothesized that temperature seasonality should favor the occurrence of social spiders by providing a constant environment, but found no support for this effect, indicating that sociality in spiders overall does not correlate with a thermally constant environment. This seemingly lack of effect of temperature seasonality on the distribution pattern of social spiders is perhaps not so surprising as a subset of all the social spider lineages, the genus Stegodyphus, occur across a set of seasonal habitats, spanning the African continent and Indian subcontinent (Majer et al., 2013b). Social Stegodyphus show a remarkably wide distribution range in terms of temperature gradient, which for example on the Indian subcontinent spans from Himalayas to Sri Lanka (Settepani et al., 2014). Given the strongly inbreeding mating system and very limited genetic variation of social spiders, their wide distribution range in terms of temperature is intriguing and suggests either strong plasticity in habitat tolerance or local adaptation (Settepani et al., 2014).
Evolution of Sociality
Group living in spiders evolves by elimination of pre-mating dispersal, evolution of cooperation and the transition to intra-colony mating and therefore a continuously inbreeding mating system (Lubin and Bilde, 2007). Given the sedentary nature of social groups and their reliance on the arrival of sufficient prey in the capture web, the need for a sufficiently high and constant supply of insect food supports the importance of habitat productivity for the evolution and maintenance of social spider groups (Avilés and Tufiño, 1998; Bilde et al., 2007). We propose that elimination of dispersal in actively hunting predators like spiders can only be favored in environments with ample food supply. The role of cooperative hunting that allows a shift in dietary niche to exploit larger prey may be an important contributing factor to group living in spiders. Cooperative foraging can allow animals to capture larger prey or different types of prey than individuals would be able to, for example cooperative hunting strategies in lions facilitate capture of larger prey (Packer et al., 1990). Thereby, cooperation enables the use of otherwise unavailable resources by increasing dietary niche (Kruuk, 1972; Creel and Creel, 1995; Bonsall and Wright, 2012; Davies et al., 2012; Marino et al., 2012). There is some evidence for this effect in the New World social spiders Anelosimus (Yip and Aviles, 2008), whereas Old World species may not obtain similar foraging benefits from extremely large prey (Riechert, 1985; Majer et al., 2012).
Field studies suggest that social spiders are resource limited: local competition increases with group size and individual fecundity decreases (Avilés and Tufiño, 1998; Bilde et al., 2007). Under conditions with limited resources, the evolution of cooperative traits that contribute to resource-enhancement or resource efficiency may be favored (Van Dyken and Wade, 2012). Indeed, social groups benefit from traits that increase resource enhancement/efficiency through cooperative web building and prey capture, and cooperative brood care that facilitates provisioning of young (Lubin and Bilde, 2007). In this way, cooperative traits in social spiders appear to be driven by environmental constraints similarly to social mammals or birds (Emlen, 1982; Faulkes et al., 1997; Jetz and Rubenstein, 2011). In contrast, many eusocial insects species benefit from altruistic traits that confer high fecundity and/or survival benefits, i.e., nest defense or worker altruism, traits that are favored when there are abundant resources and weak local competition (Van Dyken and Wade, 2012).
Finally, another form of aggregated living in spiders exists where individuals remain territorial but interconnect their capture webs to form large aggregations of individual webs. These interconnected spiders are temporary aggregations around abundant prey sources that benefit from “ricochet effects,” where a prey may bounce from one web and into another web, which means that less prey escapes the aggregated webs (Bilde and Lubin, 2011). Ultimately this reduces variance in prey capture. Whilst these so-called “colonial” spiders do not share a communal nest and are therefore “less” social, their aggregation has also evolved around abundant insect prey resources, supporting the significance of the role of food resources in group formation.
Conflict of Interest Statement
The authors declare that the research was conducted in the absence of any commercial or financial relationships that could be construed as a potential conflict of interest.
Acknowledgments
The authors are most grateful to the members of Spiderlab Aarhus University for the ever friendly and supporting working environment. Thank you to the Ecoinformatics and Biodiversity group at Aarhus University for helpful comments and discussions about the analyses done in this manuscript. Comments of two reviewers helped to improve the manuscript. This study was supported by the grant from The Danish Council for Independent Research to TB (grant number 09-065911).
Supplementary Material
The Supplementary Material for this article can be found online at: http://journal.frontiersin.org/article/10.3389/fevo.2015.00101
References
Agnarsson, I., Avilés, L., Coddington, J. A., and Maddison, W. P. (2006). Sociality in Theridiid spiders: repeated origins of an evolutionary dead end. Evolution 60, 2342–2351. doi: 10.1111/j.0014-3820.2006.tb01869.x
Avilés, L. Gelsey, G. (1998). Natal dispersal and demography of a subsocial Anelosimus species and its implications for the evolution of sociality in spiders. Can. J. Zool. 76, 2137–2147. doi: 10.1139/z98-177
Avilés, L., and Tufiño, P. (1998). Colony size and individual fitness in the social spider Anelosimus eximius. Am. Nat. 152, 403–418. doi: 10.1086/286178
Avilés, L. (1997). “Causes and consequences of cooperation and permanentsociality in spiders,” in The Evolution of Social Behaviour in Insects and Arachnids, eds J. C. Choe and B. J. Crespi (Cambridge: Cambridge University Press), 476–498.
Avilés, I., Agnarsson, P. A., Salazar, J., Purcell, G., Iturralde, E. C., Yip, K. S., et al. (2007). Natural history miscellany - Altitudinal patterns of spider sociality and the biology of a new midelevation social Anelosimus species in Ecuador. Am. Nat. 170, 783–792. doi: 10.1086/521965
Bilde, T., Coates, K. S., Birkhofer, K., Bird, T., Maklakov, A., Lubin, Y., et al. (2007). Survival benefits select for group living in a social spider despite reproductive costs. J. Evol. Biol. 20, 2412–2426. doi: 10.1111/j.1420-9101.2007.01407.x
Bilde, T., and Lubin, Y. (2011). “Group living in spiders: cooperative breeding and coloniality,” in Spider Behaviour: Flexibility and Versatility, ed M. E. Herberstein (Cambridge, UK: Cambridge University Press), 275–306.
Bodasing, M., Crouch, T., and Slotow, R. (2002) The influence of starvation on dispersal in the social spider, Stegodyphus mimosarum (Araneae, Eresidae). J. Arachnol. 30, 373–382. doi: 10.1636/0161-8202(2002)030[0373:TIOSOD]2.0.CO;2
Bonsall, M. B., and Wright, A. E. (2012). Altruism and the evolution of resource generalism and specialism. Ecol. Evol. 2, 515–524. doi: 10.1002/ece3.206
Borer, E. T., Seabloom, E. W., and Tilman, D. (2012). Plant diversity controls arthropod biomass and temporal stability. Ecol. Lett. 15, 1457–1464. doi: 10.1111/ele.12006
Brummit, R. K. (2001). World Geographical Scheme for Recording Plant Distributions. Pittsburgh, PA: Hunt Institute for Botanical Documentation Carnegie Mellon University.
Clarke, A., and Gaston, K. J. (2006). Climate, energy and diversity. Proc. R. Soc. B Biol. Sci. 273, 2257–2266. doi: 10.1098/rspb.2006.3545
Creel, S., and Creel, N. M. (1995). Communal hunting and pack size in African wild dogs, Lycaon pictus. Anim. Behav. 50, 1325–1339. doi: 10.1016/0003-3472(95)80048-4
Davies, B., Krebs, R., and West, A. (2012). “Living in groups,” in An Introduction to Behavioural Ecology, eds J. R. Krebs and N. B. Davies (Chichester: JohnWiley & Sons), 147–177.
Dormann, C. F. (2007). Assessing the validity of autologistic regression. Ecol. Modell. 207, 234–242. doi: 10.1016/j.ecolmodel.2007.05.002
Dugatkin, L. A. (1997). Cooperation among Animals: An Evolutionary Perspective. Oxford, UK: Oxford University Press.
Emlen, S. T. (1982). The evolution of helping. I. An ecological constraints model. Am. Nat. 119, 29–39. doi: 10.1086/283888
Emlen, S. T. (1994). Benefits, constrainsts and the evolution of the family. Trends Ecol. Evol. 9, 282–285. doi: 10.1016/0169-5347(94)90030-2
Faulkes, C. G., Bennett, N. C., Bruford, M. W., O'Brien, H. P., Aguilar, G., Jarvis, J. U. M., et al. (1997). Ecological constraints drive social evolution in the African mole–rats. Proc. R. Soc. Lond. B Biol. Sci. 264, 1619–1627. doi: 10.1098/rspb.1997.0226
Field, J., Paxton, R. J., Soro, A., and Bridge, C. (2010). Cryptic plasticity underlies a major evolutionary transition. Curr. Biol. 20, 2028–2031. doi: 10.1016/j.cub.2010.10.020
Guevara, J., and Aviles, L. (2007). Multiple techniques confirm elevational differences in insect size that may influence spider sociality. Ecology 88, 2015–2023. doi: 10.1890/06-0995.1
Gunnels, C. W., Dubrovskiy, A., and Avalos, A. (2008). Social interactions as an ecological constraint in a eusocial insect. Anim. Behav. 75, 681–691. doi: 10.1016/j.anbehav.2007.07.022
Hatchwell, B. J., and Komdeur, J. (2000). Ecological constraints, life history traits and the evolution of cooperative breeding. Anim. Behav. 59, 1079–1086. doi: 10.1006/anbe.2000.1394
Hillebrand, H. (2004). On the generality of the latitudinal diversity gradient. Am. Nat. 163, 192–211. doi: 10.1086/381004
Janzen, D. H., and Schoener, T. W. (1968). Differences in insect abundance and diversity between wetter and drier sites during a tropical dry season. Ecology 49, 96–110. doi: 10.2307/1933565
Janzen, D. H. (1973). Sweep samples of tropical foliage insects: effects of seasons, vegetation types, elevation, time of day, and insularity. Ecology 54, 687–708. doi: 10.2307/1935359
Jarvis, J. U. M., Bennett, N. C., and Spinks, A. C. (1998). Food availability and foraging by wild colonies of Damaraland mole-rats (Cryptomys damarensis): implications for sociality. Oecologia 113, 290–298. doi: 10.1007/s004420050380
Jetz, W., and Rubenstein, D. R. (2011). Environmental uncertainty and the global biogeography of cooperative breeding in birds. Curr. Biol. 21, 72–78. doi: 10.1016/j.cub.2010.11.075
Johannesen, J., Lubin, Y., Smith, D. R., Bilde, T., and Schneider, J. M. (2007). The age and evolution of sociality in Stegodyphus spiders: a molecular phylogenetic perspective. Proc. R. Soc. B Biol. Sci. 274, 231–237. doi: 10.1098/rspb.2006.3699
Johnson, J. B., and Omland, K. S. (2004). Model selection in ecology and evolution. Trends Ecol. Evol. 19, 101–108. doi: 10.1016/j.tree.2003.10.013
Kaspari, M., and Vargo, E. L. (1995). Colony size as a buffer against seasonality: Bergmann's rule in social insects. Am. Nat. 145, 610–632. doi: 10.1086/285758
Kaspari, M., and Weiser, M. D. (2011). Energy, taxonomic aggregation, and the geography of ant abundance. Ecography 35, 65–72. doi: 10.1111/j.1600-0587.2011.06971.x
Kocher, S. D., Pellissier, L., Veller, C., Purcell, J., Nowak, M. A., Chapuisat, M., et al. (2014). Transitions in social complexity along elevational gradients reveal a combined impact of season length and development time on social evolution. Proc. R. Soc. B Biol. Sci. 281:20140627. doi: 10.1098/rspb.2014.0627
Kriticos, D. J., Webber, B. L., Leriche, A., Ota, N., Macadam, I., Bathols, J., et al. (2011). CliMond: global high-resolution historical and future scenario climate surfaces for bioclimatic modelling. Methods Ecol. Evol. 3, 53–64. doi: 10.1111/j.2041-210X.2011.00134.x
Kruuk, H. (1972). The Spotted Hyena: A Study of Predation and Social Behavior. Chicago, IL: University of Chicago Press.
Lobo, J. M. (2007). EDIT Geoplatform. Available online at: http://edit.csic.es/GISdownloads.html
Lubin, Y. (2010). “Spiders: Social Evolution,” in Encyclopedia of Animal Behavior, Vol. 3, eds M. D. Breed and J. Moore, (Oxford: Elsevier Ltd), 329–334.
Lubin, Y., and Bilde, T. (2007). “The evolution of sociality in spiders,” in Advances in the Study of Behavior, Vol. 37, eds H. J. Brockmann, T. J. Roper, M. Naguib, K. E. Wynne-Edwards, C. Barnard, and J. Mitani (Academic Press), 83–145.
Majer, M., Agnarsson, I., Svenning, J.-C., and Bilde, T. (2013a). Social spiders of the genus Anelosimus occur in wetter, more productive environments than non-social species. Naturwissenschaften 100, 1031–1040. doi: 10.1007/s00114-013-1106-6
Majer, M., Holm, C., and Lubin, Y. Bilde, T. (2012). Group Living Benefits in the Social Spiders: Optimal Foraging and Silk Saving Properties of Solitary and Social Stegodyphus Species. Doctorate thesis, Aarhus University, Aarhus.
Majer, M., Svenning, J.- C., and Bilde, T. (2013b). Habitat productivity constrains the distribution of social spiders across continents - case study of the genus Stegodyphus. Front. Zool. 10, 9. doi: 10.1186/1742-9994-10-9
Marino, J., Sillero-Zubiri, C., Johnson, P. J., and Macdonald, D. W. (2012). Ecological bases of philopatry and cooperation in Ethiopian wolves. Behav. Ecol. Sociobiol. 66, 1005–1015. doi: 10.1007/s00265-012-1348-x
Nagelkerke, N. J. D. (1991). A note on a general definition of the coefficient of determination. Biometrika 78, 691–692. doi: 10.1093/biomet/78.3.691
NCDC Satellite Data Services Division (1985-1988). Weekly Plate Carreé (uncalibrated) Global Vegetation Index Product from NOAA-9 (APR 1985–DEC 1988). Washington DC: Digital Raster Data on a Geographic (lat/long) 904 × 2500 grid. N. N. C. D. Center.
Nentwig, W. (1985). Social spiders catch larger prey: a study of Anelosimus eximius (Araneae: Theridiidae). Behav. Ecol. Sociobiol. 17, 79–85. doi: 10.1007/BF00299433
Packer, C., Scheel, D., and Pusey, A. E. (1990). Why lions form groups: food is not enough. Am. Nat. 136, 1–19. doi: 10.1086/285079
Parrish, J. K., and Hamner, W. M. (1997). Animal Groups in Three Dimensions. Cambridge, UK: Cambridge University Press.
Pianka, E. R. (1966). Latitudinal gradients in species diversity - a review of concepts. Am. Nat. 100, 33–46. doi: 10.1086/282398
Purcell, J. (2011). Geographic patterns in the distribution of social systems in terrestrial arthropods. Biol. Rev. 86, 475–491. doi: 10.1111/j.1469-185X.2010.00156.x
Rangel, T. F., Diniz-Filho, J. A. F., and Bini, L. M. (2010). SAM: a comprehensive application for Spatial Analysis in Macroecology. Ecography 33, 46–50. doi: 10.1111/j.1600-0587.2009.06299.x
R Development Core Team. (2011). R: A Language and Environment for Statistical Computing. Vienna: R Foundation for Statistical Computing. Available online at: http://www.R-project.org/.
Riechert, S. E. (1985). Why do some spiders cooperate? agelena consociata, a case study. Fla. Entomol. 68, 105–116. doi: 10.2307/3494333
Schwarz, M. P., Richards, M. H., and Danforth, B. N. (2007). Changing paradigms in insect social evolution: insights from halictine and allodapine bees. Annu. Rev. Entomol. 52, 127–150. doi: 10.1146/annurev.ento.51.110104.150950
Settepani, V., Bechsgaard, J., and Bilde, T. (2014). Low genetic diversity and strong but shallow population differentiation suggests genetic homogenization by metapopulation dynamics in a social spider. J. Evol. Biol. 27, 2850–2855. doi: 10.1111/jeb.12520
Smith, P. A. (1994). Autocorrelation in logistic regression modelling of species' distributions. Glob. Ecol. Biogeogr. Lett. 4, 47–61. doi: 10.2307/2997753
Van Dyken, J. D., and Wade, M. J. (2012). Origins of Altruism diversity I: the diverse ecological roles of altruistic strategies and their evolutionary responses to local competition. Evolution 66, 2484–2497. doi: 10.1111/j.1558-5646.2012.01630.x
Ward, P. I. (1986). Prey availability increases less quickly than nest size in the social spider Stegodyphus Mimosarum. Behaviour 97, 213–225. doi: 10.1163/156853986X00603
Wcislo, W. T. (1997). Social interactions and behavioral context in a largely solitary bee, Lasioglossum (Dialictus) figueresi (Hymenoptera, Halictidae). Insectes Soc. 44, 199–208. doi: 10.1007/s000400050041
Willig, M. R., Kaufman, D. M., and Stevens, R. D. (2003). Latitudinal gradients of biodiversity: pattern, process, scale, and synthesis. Annu. Rev. Ecol. Evol. Syst. 34, 273–309. doi: 10.1146/annurev.ecolsys.34.012103.144032
Wolda, H. (1978). Seasonal fluctuations in rainfall, food and abundance of tropical insects. J. Anim. Ecol. 47, 369–381. doi: 10.2307/3789
Wolda, H. (1988). Insect Seasonality - Why. Annu. Rev. Ecol. Syst. 19, 1–18. doi: 10.1146/annurev.es.19.110188.000245
World Spider Catalog. (2015). World Spider Catalog. Bern: Natural History Museum. Available online at: http://wsc.nmbe.ch
Keywords: ecological constraint, macro-ecological modeling, environmental predictors, social evolution, distribution range, species diversity patterns
Citation: Majer M, Svenning J-C and Bilde T (2015) Habitat productivity predicts the global distribution of social spiders. Front. Ecol. Evol. 3:101. doi: 10.3389/fevo.2015.00101
Received: 17 April 2015; Accepted: 13 August 2015;
Published: 15 September 2015.
Edited by:
Patrizia D'Ettorre, Université Paris 13, FranceReviewed by:
Raphael Jeanson, University of Toulouse, FranceHeikki Helanterä, University of Helsinki, Finland
Copyright © 2015 Majer, Svenning and Bilde. This is an open-access article distributed under the terms of the Creative Commons Attribution License (CC BY). The use, distribution or reproduction in other forums is permitted, provided the original author(s) or licensor are credited and that the original publication in this journal is cited, in accordance with accepted academic practice. No use, distribution or reproduction is permitted which does not comply with these terms.
*Correspondence: Trine Bilde, Department of Bioscience, Aarhus University, Ny Munkegade 116, 8000 Aarhus C, Denmark, trine.bilde@biology.au.dk