- Department of Biology, Lund University, Lund, Sweden
Fatty acids (FA) have crucial functions in animals, affecting e.g., inflammatory responses, thermoregulation, and cell membrane fluidity. Diet and ambient temperature affect animals' FA composition, which, in turn, may influence these physiological processes. Great tits (Parus major)—common in both urban and rural habitats—are mainly granivorous during winter and insectivorous during summer. These diets show pronounced differences in FA composition. Such variation has context-dependent effects on physiology, because the thermal environment, food availability, and levels of pro-inflammatory environmental stressors differ between urban and rural areas. Thus, we investigated how great tit plasma FA composition varied between urban and rural habitats and across seasons. Eight FAs differed between urban and rural birds. Among these, arachidonic acid [omega (ω)-6 polyunsaturated FA] with thermoregulatory and pro-inflammatory properties was more abundant in urban than rural birds in winter, whereas ω-3 FAs with anti-inflammatory properties were more abundant in rural birds. The difference in pro- and anti-inflammatory FAs suggest that the negative health effects that urban birds suffer from being exposed to higher levels of pollutants might be enhanced by an elevated inflammatory response. Eight FAs differed between winter and summer birds. This variation reflected the diet change: FAs common in seeds, e.g., oleic- and linoleic acid, were present in higher amounts in winter birds, whereas ω-3 polyunsaturated FAs that are common in caterpillars were more abundant in summer birds. Overall, a larger seasonal variation was seen among the urban birds. This study is the first to reveal a difference in FA composition between urban and rural populations for all animals studied to date. Future experiments should unravel the physiological implications of this variation, and ultimately, link its effects to fitness of animals with different physiological and dietary requirements in urban and rural environments.
Introduction
Urbanization is a global concern for the health and persistence of many wild animal populations. Apart from the major problems of habitat loss and fragmentation, urbanization is associated with a number of other factors that threaten wildlife, including higher pollution levels and predation by pets (Marzluff et al., 2001; Isaksson, 2015). In addition, there are also enticements that lure animals into the urban habitats, such as high availability of anthropogenic food sources and higher temperatures. Thus, animals that enter an urban habitat might gain some immediate benefits, however, the urban inhabitants might suffer in the long run from reduced health, survival and/or lifetime reproductive output. In temperate regions, food availability and environmental temperature are two factors with great impact on animal survival during the cold winter months. What is perhaps less known is the impact of diet and ambient temperature on the fatty acid (FA) composition of animal tissues, which is important for a wide range of physiological processes including oxidative stress, inflammation, thermoregulation, exercise performance, and cell membrane fluidity (Hazel, 1995; Larsson et al., 2004; Pierce et al., 2005; Ben-Hamo et al., 2011; Hulbert and Abbott, 2012). These physiological processes, in turn, are affected by or regulated in response to extrinsic stressors, such as pollutant levels, pathogen infections, and temperature, which all vary on both temporal (e.g., between seasons) and spatial (e.g., between urban and rural habitats) scales (Tinoco, 1982; Hulbert and Else, 1999; Larsson et al., 2004; McWilliams et al., 2004; Cherian et al., 2009; Hulbert and Abbott, 2012). Thus, variation in FA composition is likely to affect animal populations differently, depending on both season and the degree of urbanization. In addition, by studying variation in animal FA content across habitats and seasons, we can gain insights into variation in animals' diets and pinpoint individual FAs that might constrain or facilitate any context-dependent physiological responses to the environment.
Birds and other animals that live in urban areas have higher oxidative stress and inflammation, as compared to animals in rural habitats (Isaksson, 2010, 2015). This difference has largely been explained by the higher pollution levels in urban areas (e.g., Han and Naeher, 2006; Stroh et al., 2007). However, the potential contribution of fatty acid nutrition to oxidative stress and inflammation has largely been neglected. In particular, a high dietary intake of omega-6 polyunsaturated FAs (ω-6 PUFAs) relative to omega-3 polyunsaturated FAs (ω-3 PUFAs) (i.e., ω-6/ω-3) increases inflammatory responses and oxidative stress via production of eicosanoids and reactive oxygen species, respectively (Larsson et al., 2004; Cherian, 2007; Gomes et al., 2012). Thus, a high intake of ω-6 PUFAs in urban environments might further enhance the already high levels of oxidative stress caused by inhalation of pollutants (Isaksson et al., 2005; Isaksson, 2010, 2015). Moreover, anthropogenic winter feeding of birds with seeds and nuts with a high content of the monounsaturated FA (MUFA) oleic acid and the ω-6 PUFA linoleic acid (Beare-Rogers et al., 2001; Becker, 2008), is common across northern temperate latitudes. The density of such anthropogenic food sources is obviously higher in habitats with more human settlements than in e.g., rural forests (Robb et al., 2008). Despite the detrimental effect of poor diet in urban habitats, no study has investigated potential differences in FA profiles between urban and rural bird populations, only the total levels of free fatty acids and triglycerides in plasma (Fokidis et al., 2011; Davies et al., 2013).
Furthermore, since average ambient temperatures generally are higher in cities (Oke, 1973; Hu and Brunsell, 2015), habitat-specific differences in anthropogenic winter feeding might also have habitat-specific physiological consequences, because both oleic acid and arachidonic acid—biosynthesized from linoleic acid (Larsson et al., 2004)—play important roles in thermoregulation (Lerner et al., 1972; Ben-Hamo et al., 2011). For instance, the content of oleic acid in the liver was negatively correlated to body temperature during fasting-induced rest-phase hypothermia in Japanese quail, Coturnix japonica (Ben-Hamo et al., 2011). Thus, a high content of oleic acid during winter might allow birds to save more energy by maintaining a lower body temperature during rest-phase hypothermia (though this might be associated with other costs; e.g., Nord et al., 2011, 2013), although the higher ambient temperature in urban environments lowers the energy cost for thermoregulation.
Apart from the differences in diet and ambient temperatures between urban and rural habitats, the seasonal differences in these two parameters are even greater for many species in temperate regions. Indeed, seasonal variation in FA composition has been found in the tissues of several bird species (Barnett, 1970; Palokangas and Vihko, 1972; Conway et al., 1994; Egeler and Williams, 2000; Pierce and McWilliams, 2005), and the FA content of ingested food items affects the FA composition of the birds (Austin, 1993; Crespo and Esteve-Garcia, 2001; Pierce et al., 2004; Ben-Hamo et al., 2011; but see West and Meng, 1968). In addition to diet, seasonal variation in birds' FA composition might be influenced by selective mobilization of FAs (Raclot, 2003). This occurs to a great extent in e.g., migratory birds to meet context-dependent physiological demands (Price et al., 2008).
Ambient temperature also influences the FA composition of animal tissues. For instance, the ratio of saturated fatty acids (SFA) to unsaturated FAs in cell membranes is often reduced in response to decreasing ambient temperature in order to maintain membrane fluidity, a mechanism known as “homeoviscous adaptation” (Sinensky, 1974; Hazel, 1995). This mechanism is used by ectothermic vertebrates (Cossins and Macdonald, 1989; Hazel, 1995), but might also be important for birds (see Ben-Hamo et al., 2011, 2013, and references therein) where many species are temporally heterothermic (known as daily torpor or rest-phase hypothermia) during the inactive part of the day in cold ambient conditions (Haftorn, 1972; McKechnie and Lovegrove, 2002; Schleucher, 2004; Nord et al., 2009, 2013; Seebacher et al., 2010). In support of this hypothesis, the proportion of SFA in depot fat increased with ambient temperature from 4 to 30°C in starlings (Sturnus vulgaris) and rock partridges (Alectoris chucar), irrespective of diet (Yom-Tov and Tietz, 1978). In addition, ambient temperature alters the proportion of individual FAs in birds, including both SFAs and unsaturated FAs (Balnave, 1973; Ben-Hamo et al., 2013). Still, the independent effects of seasonal variation in ambient temperature and co-occurring seasonal differences in diet composition on birds' FA composition have hitherto been difficult to disentangle in natural populations.
To shed light on habitat differences in FA composition in relation to urbanization, and the extent to which any such differences vary between seasons, we measured FA composition in urban and rural populations of great tits (Parus major Linnaeus 1758) during both winter and summer. This species is an appropriate model for these research questions, because it is common in both urban and rural areas across its European range, and regularly visits bird feeders. Moreover, great tits show a pronounced seasonal change in diet, with seeds (high oleic- and linoleic acid content; Table 1) being the main food source during winter, and caterpillars (rich in the ω-3 PUFA α-linolenic acid, αLNA, due to leaf feeding; Table 1) being the staple food item during late spring to early summer (Perrins, 1991; Gosler, 1993). It was previously shown that great tits living in habitats with low or high caterpillar abundance show marked differences in FA composition of blood plasma (Isaksson et al., 2015). In the present study, we also analyzed FAs in plasma, using sensitive gas chromatography/mass spectrometry. This tissue requires less invasive sampling and its FA composition more strongly reflects dietary intake of FAs as compared to other tissues (Hulbert and Abbott, 2012).
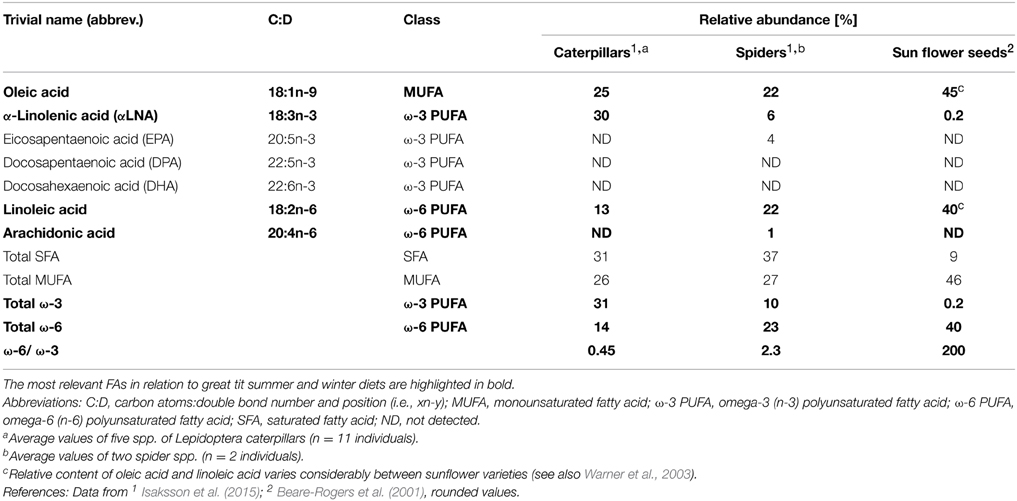
Table 1. Classification and average relative abundance [% of total fatty acid (FA) content] of major fatty acids in common great tit food items.
We hypothesized that the plasma FA composition of great tits would vary between urban and rural habitats, and across seasons due to differences in diet composition and ambient temperature. In relation to seasonal variation, we predicted that (i) winter birds would have higher relative levels of oleic- and linoleic acid that are abundant in seeds. We also predicted that (ii) summer birds would have higher relative levels of ω-3 PUFAs, i.e., αLNA that is abundant in caterpillars, as well as eicosapentaenoic acid (EPA), docosapentaenoic acid (DPA), and docosahexaenoic acid (DHA) that can be biosynthesized from αLNA (Larsson et al., 2004). Due to the seasonal diet change we also predicted (iii) that the physiologically important ratio between total ω-6 PUFAs and total ω-3 PUFAs would be lower in summer birds than in winter birds. Due to seasonal temperature differences, we predicted that (iv) the proportion of total SFA would be lower in birds in winter than in summer.
Due to possible habitat-related differences in diet and temperature we predicted that (v) the FA composition would differ between birds from the urban and rural habitat during a specific season, and (vi) that this variation would lead to differences in the extent of the seasonal variation in plasma FA levels between birds from these two habitat types. In other words, we expected to find a statistical “habitat × season” interaction in great tit FA composition.
Materials and Methods
Bird Populations and Sampling
Fieldwork was performed in the province of Scania in southern Sweden during 2013. The urban birds were caught at four different locations within the city limits of Malmö, the largest city in southernmost Sweden with approximately 300,000 inhabitants (study areas centered around 55° 35′ N, 12° 59′ E). The sites were small to large city parks with established nest box populations of great tits. The parks were characterized by a mixture of conifer (Pinus, Picea, and Larix spp.) and deciduous trees (mainly Betula, Fagus, and Quercus spp.), along with open managed grassland and the urban influences of paved roads and buildings. A satellite image of the urban sites is provided in Supplementary Figure 1, along with an estimation of urbanization i.e., the scores of relative cover of buildings, vegetation and paved surfaces of an area of 1 × 1 km (according to Seress et al., 2014). Rural birds were caught at three different locations between 42 and 54 km E to NE of Malmö (study areas centered around 55° 40′ N, 13° 31′ E). The rural areas were sparsely inhabited by humans (< 5 inhabitants per km2), and comprised of either farmland interspersed by smaller mixed-stand forests (2 sites) or larger continuously forested areas (1 site), largely dominated by the same tree species as the urban parks, though a bias toward conifers (mainly Pinus sylvestris) in the larger forested site. The different rural sites were chosen to capture the heterogeneity of different rural habitats inhabited by great tits. Similar to the urban habitat, satellite images of the rural sites are provided in Supplementary Figure 2 and the level of urbanization estimated. Sampling was conducted in two bouts: winter (14-Feb-2013–28-March-2013) and summer (26-May-2013–09-June-2013). The average ambient daily temperature during winter sampling was −1.0°C in the rural habitat and 0.3°C in the urban habitat. During summer the average daily ambient temperature was 14.1°C in the rural habitat and 15.6°C in the urban habitat (urban weather station: Malmö/centrum, rural station: Sjöbo/Björka; Freiholtz, 2013)1. Thus, urban areas were on average 1.3°C or 1.5°C warmer than rural areas during winter and summer, respectively.
During winter, great tits were caught with mist nets (nurban = 35, nrural = 31; in total 29 males and 37 females), and during summer, birds were caught in nest boxes whilst feeding nestlings (nurban = 30, nrural = 30; in total 29 males and 31 females). All birds were individually ringed, measured (tarsus length, body mass), and sexed according to plumage characteristics (Svensson, 1992). Within 5 min after capture we collected a 110 μl blood sample from the jugular vein with a heparinized syringe and kept it on ice until centrifugation (1800 rpm for 10 min) 0–1 h later. All samples were stored at −80°C until biochemical analyses.
Fatty Acid Extraction and Gas Chromatography/Mass Spectrometry (GC/MS) Analysis
A total lipid extraction of 5 μl plasma was performed for 1 h at room temperature using 50 μl chloroform:methanol (2:1 v/v). The solvent was then evaporated under a gentle N2 stream, and the concentrated lipid extracts were subjected to base methanolysis to convert fatty-acyl moieties into corresponding methyl esters. In this step, 100 μl KOH/methanol (0.5 M) was added to the extracts and the reaction allowed to proceed for 1 h at 40°C, after which 100 μl HCl/methanol was added to terminate the reaction. Resulting fatty acid methyl esters (FAME) were then extracted in 300 μl re-distilled n-hexane. The hexane extract was washed twice with 200 μl H2O and then dried over anhydrous sodium sulfate. Finally, extracts were concentrated under N2 to 80–100 μl before GC/MS analysis.
The FAME extracts were analyzed with an Agilent 5975 mass spectrometer coupled to an Agilent 6890 GC with an HP-88 capillary column [(88%-Cyanopropy)aryl-polysiloxane; 30 m, 0.25 mm id, df 0.20 μm; Agilent]. The oven temperature was set to 80°C for 1 min, then increased by 10°C/min to 230°C and held for 20 min. Helium was used as carrier gas at a constant flow of 1 ml/min. Nineteen FAMEs in plasma samples could be identified by comparing mass spectra and retention times with those of synthetic standards (Supelco 37-Component FAME Mix, Sigma-Aldrich).
Data Analysis
The abundance of each FA was normalized into proportion of total FA by dividing the peak area with the sum of the peak areas of all FAs in each individual. Statistical analyses were performed on the nine FAs that had an average within-individual relative content above 1% of the total FA content during at least one of the seasons (as in e.g., Ben-Hamo et al., 2013; Isaksson et al., 2015). All analyses were performed with general linear models (GLM) using IBM SPSS Statistics v21. The proportion of each FA was logit-transformed, i.e., log(y/[1-y]), prior to analyses according to recommendations for proportional data in Warton and Hui (2011), and then analyzed independently. The proportions of total SFA were analyzed in the same way. The total ω-6/total ω-3 PUFA ratio was analyzed based on log10-transformed values. Factors included in all models were: “season” (winter or summer), “habitat” (rural or urban), and “sex” (male or female). Body condition (residuals from a regression between body mass and tarsus length of all individuals in the study) was included as a covariate, because it has been shown to correlate with the relative abundance of some FAs in great tits (Isaksson et al., 2015). Since there are several different indexes of body condition, we also ran the statistical models using the scaled mass index (Peig and Green, 2009) with outcomes showing nearly identical F and p-values for all predictor variables. This result indicated the robustness of our models using the residual body condition index. Interactions included in all models were “season × habitat” and “body condition × season.” Hence, all models contained the same set of predictor variables to facilitate comparison of habitat-related and seasonal variation of different FAs. We also analyzed FA proportions from the two seasons separately (using factorial GLM) when the season × habitat interaction was significant (i.e., for αLNA, EPA, arachidonic acid, palmitic acid, and stearic acid). Variation in body condition across seasons and habitats was analyzed with factorial GLM with season and habitat, and their interaction, as factors. Only significant (p < 0.05) factors and interactions from the models are reported in the Results.
Results
Relative FA Abundance and Variation
In total, 19 FAs could be identified and quantified in all 126 samples (nurban, winter = 35, nrural, winter = 31, nurban, summer = 30, nrural, summer = 30), of which nine FAs had average relative proportions >1% of total FA during at least one of the seasons (indicated below in italics). The 19 FAs included four SFAs (myristic- [14:0], palmitic- [16:0], stearic- [18:0], and arachidic acid [20:0]), four MUFAs (palmitoleic- [16:1n-7], oleic- [18:1n-9], cis-vaccenic- [18:1n-7], and eicosenoic acid [20:1n-9]), four ω-3 PUFAs (αLNA [18:3n-3], EPA [20:5n-3], DPA [22:5n-3], and DHA [22:6n-3]), six ω-6 PUFAs (linoleic- [18:2n-6], gamma (γ)-linolenic- [18:3n-6], eicosadienoic- [20:2n-6], dihomo-γ-linolenic- [20:3n-6], arachidonic- [20:4n-6], and adrenic acid [22:4n-6]), and one ω-9 PUFA (mead acid [20:3n-9]). The five most abundant FAs in all samples were oleic-, linoleic-, arachidonic-, palmitic-, and stearic acid, which all ranged from 11 to 26 % of the total FA content across seasons and habitats. The most abundant ω-3 PUFAs were αLNA and EPA during summer (3–5%), and DHA during winter (1%). Eight FAs (oleic-, linoleic-, αLNA, EPA, DPA, arachidonic-, palmitic-, and stearic acid) differed significantly between winter and summer (see below). Another, largely overlapping, set of eight FAs (linoleic-, αLNA, EPA, DPA, DHA, arachidonic-, palmitic-, and stearic acid) differed between the urban and rural habitat, during one or both of the seasons. In addition, four FAs (oleic-, arachidonic-, stearic acid, and DHA) were either positively or negatively related to body condition, and three of these relationships differed between seasons (i.e., “season × body condition” interaction). The relative abundance of all other FAs showed no association with body condition. Body condition was significantly higher during winter than during summer across habitats [F(1, 118) = 81.3, p < 0.001]. However, the seasonal variation in body condition differed between urban and rural birds [season × habitat interaction: F(1, 118) = 22.5, p < 0.001; Figure 1]. Specifically, body condition was the highest among the urban winter birds, but lowest among the urban summer birds (Figure 1).
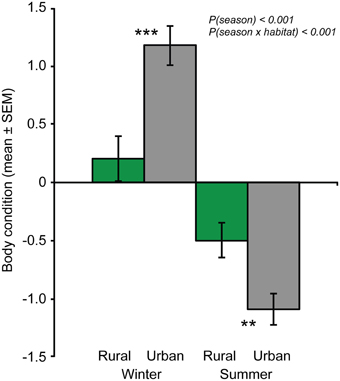
Figure 1. Body condition (i.e., the residuals from a regression between body mass and tarsus length) of great tits in urban and rural habitats during winter and summer (Ntotal = 126). Significant effects from the model are indicated by the p-values. However, due to the significant season × habitat interaction, body condition of birds from the two habitats was also analyzed separately for each season, and significant differences between habitats within a season are indicated by asterisks (**p < 0.01; ***p < 0.001).
Monounsaturated Fatty Acids
Of the four identified MUFAs, statistical analysis was performed on the most abundant one, oleic acid. The proportion of oleic acid was significantly lower in summer than in winter birds [F(1, 119) = 4.11, p = 0.045], but did not differ between rural and urban birds (Figure 2). Furthermore, the proportion of oleic acid showed a positive relationship with body condition in the winter birds, but not in the summer birds [body condition × season interaction: F(1, 119) = 10.93, p = 0.001; Figure 3A].
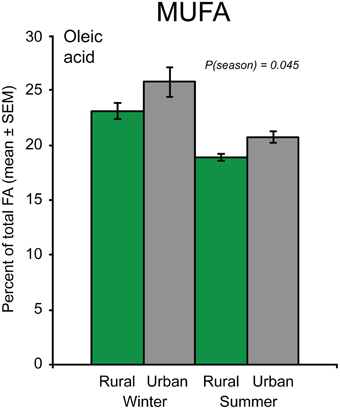
Figure 2. The proportion of the mono-unsaturated fatty acid (MUFA) oleic acid in great tit blood plasma during winter and summer in birds from urban and rural habitats (Ntotal = 126). The significant “season” effect from the model is indicated by the p-value.
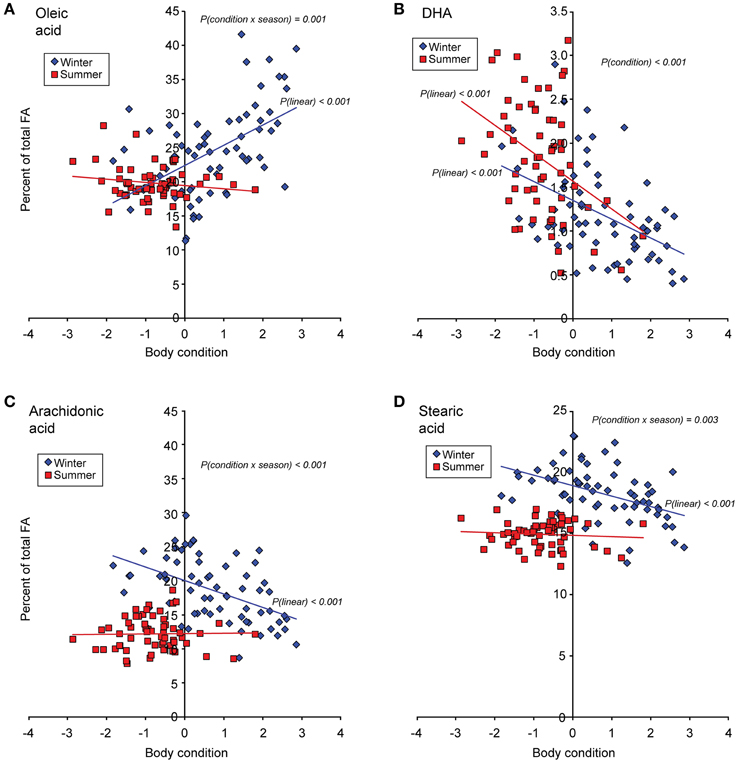
Figure 3. The relationship between body condition (i.e., the residuals from a regression between body mass and tarsus length) and fatty acid (FA) proportions in great tit blood plasma during winter and summer (Ntotal = 126). (A) Oleic acid, (B) docosahexaenoic acid (DHA), (C) arachidonic acid, and (D) stearic acid. Untransformed data are plotted, whereas the statistics and reported p-values are based on logit-transformed proportions (for details, see Materials and Methods). Only significant p-values for main model effects and individual regressions are shown (all p-values for non-significant regressions >> 0.05). The relative FA abundances reported here also underlie the mean values presented in Figure 2 (oleic acid), Figures 4D,F (DHA and arachidonic acid), and Figure 6C (stearic acid). Note that the Y-axis scale differs between figure panels.
Omega-3 Polyunsaturated Fatty Acids
In general, the proportions of the ω-3 PUFAs were low during winter, but up to 30-fold higher during summer. The proportion of the strictly dietary αLNA was significantly higher in birds during summer than during winter [F(1, 119) = 394.9, p < 0.001]. This seasonal increase in αLNA was higher in urban birds [season × habitat interaction: F(1, 119) = 8.54, p = 0.004; Figure 4A]. Subsequent analyses of the two seasons separately showed that the proportion of αLNA in the rural birds was higher than in the urban birds only during winter (Figure 4A).
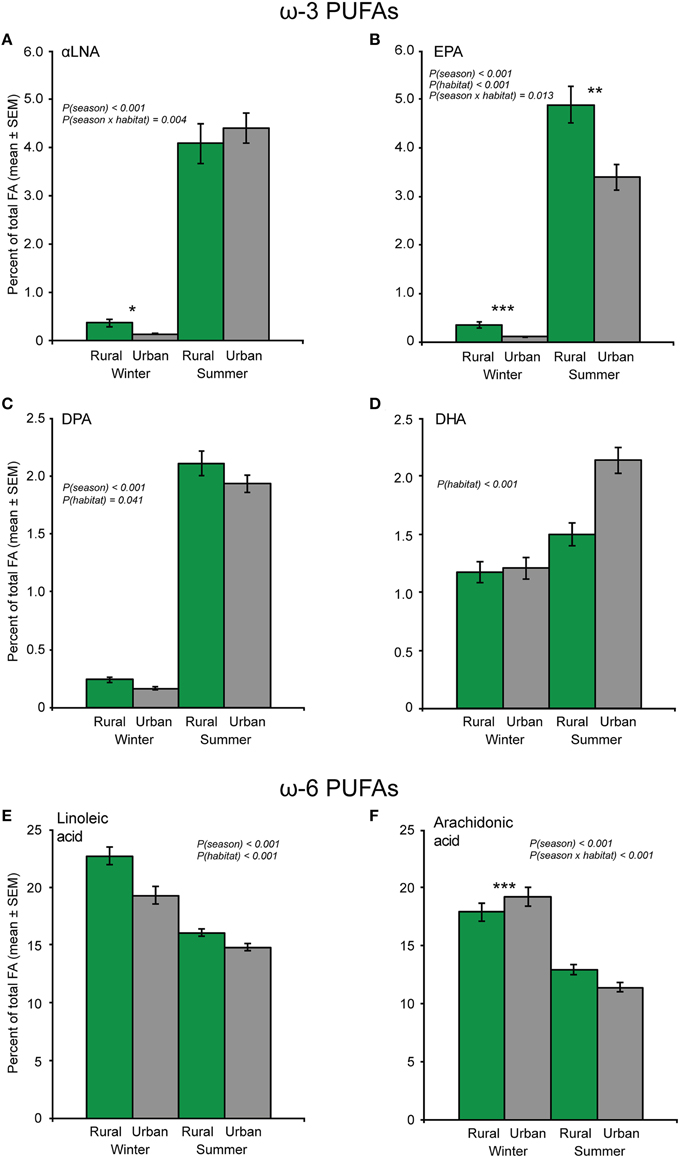
Figure 4. The proportion of ω-3 and ω-6 polyunsaturated fatty acids (PUFA) in blood plasma from urban and rural great tits during winter and summer (Ntotal = 126). (A) α-Linolenic acid (αLNA), (B) eicosapentaenoic acid (EPA), (C) docosapentaenoic acid (DPA), (D) docosahexaenoic acid (DHA), (E) linoleic acid, and (F) arachidonic acid. Significant effects from the models are indicated by the p-values. When the season × habitat interaction was significant, fatty acid (FA) proportions of birds from the two habitats were also analyzed separately for each season, and significant differences between habitats within a season are indicated by asterisks (*p < 0.05; **p < 0.01; ***p < 0.001). Note that the Y-axis scale differs between figure panels.
The proportion of EPA showed a strong seasonal variation [F(1, 119) = 487.1, p < 0.001], with summer birds having higher amounts of this FA than winter birds (Figure 4B). In addition, rural birds had a higher proportion of EPA compared to urban birds across seasons [F(1, 119) = 33.56, p < 0.001]. However, while rural birds had higher plasma levels of EPA during both seasons (Figure 4B), the seasonal variation in EPA content was still larger in urban birds [season × habitat interaction: F(1, 119) = 6.43, p = 0.013; Figure 4B].
The proportion of DPA was significantly higher in birds during summer than during winter [F(1, 119) = 522.3, p < 0.001], and also higher in rural birds across seasons [F(1, 119) = 4.26, p = 0.041; Figure 4C].
In contrast to the three other ω-3 PUFAs, the proportion of DHA only tended to differ between seasons (p = 0.057; Figure 4D), but was significantly higher in urban compared to rural birds [F(1, 119) = 13.14, p < 0.001] especially in summer (Figure 4D). DHA also showed a negative relationship with body condition [F(1, 119) = 24.12, p < 0.001], suggesting that birds in higher condition had lower relative amounts of this FA than did birds in lower condition during both seasons (Figure 3B).
Omega-6 Polyunsaturated Fatty Acids
Two of the ω-6 PUFAs, linoleic- and arachidonic acid, showed relative abundances above 1%. The proportion of the essential linoleic acid was significantly higher in the rural birds as compared to the urban birds [F(1, 119) = 11.90, p < 0.001], and higher in birds during winter than during summer [F(1, 119) = 37.84, p < 0.001; Figure 4E].
The proportion of arachidonic acid was significantly higher in birds during winter than during summer [F(1, 119) = 140.6, p < 0.001], but this seasonal variation was habitat-dependent [season × habitat interaction: F(1, 119) = 24.56, p < 0.001]. Specifically, the proportion of arachidonic acid was significantly higher in urban compared to rural birds during winter, but not during summer. Thus, the seasonal variation in arachidonic acid content was larger in the urban birds (Figure 4F). Furthermore, the proportion of arachidonic acid was negatively related to body condition, but this relationship was present only during winter [body condition × season interaction: F(1, 119) = 11.93, p < 0.001; Figure 3C].
Ratio between Omega-6 and Omega-3 Polyunsaturated Fatty Acids
We also analyzed the physiologically important ratio between total ω-6 (the sum of linoleic-, arachidonic-, γ-linolenic-, eicosadienoic-, dihomo-γ-linolenic-, and adrenic acid) and total ω-3 (the sum of αLNA, EPA, DPA, and DHA) PUFAs. This analysis showed that the ratio of total ω-6/total ω-3 did not differ between urban and rural birds, but was significantly higher during winter than during summer [F(1, 119) = 675.6, p < 0.001]. This result was explained by a seasonal decrease in ω-6 PUFAs (mainly the abundant linoleic- and arachidonic acid) and a simultaneous increase in total ω-3 PUFAs (Figure 5).
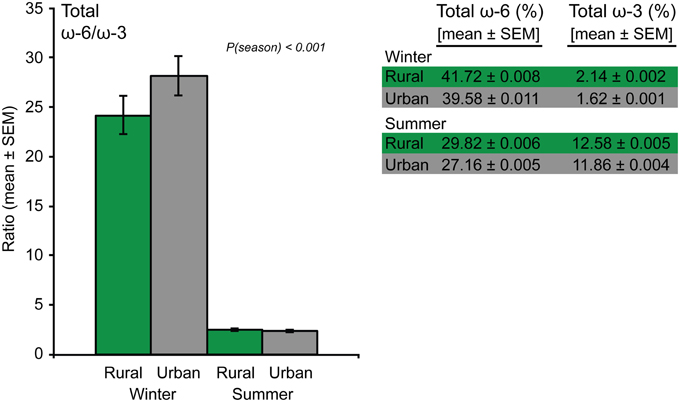
Figure 5. The within-individual ratio between total ω-6 and total ω-3 polyunsaturated fatty acids in blood plasma from urban and rural great tits during winter and summer (left panel). The significant “season” effect from the model is indicated by the p-value. Right panel lists the population-level proportions of total ω-6 and total ω-3 polyunsaturated fatty acids, respectively (Ntotal = 126).
Saturated Fatty Acids
The proportion of total SFA (the sum of myristic-, palmitic-, stearic-, and arachidic acid) was significantly higher in birds during summer than during winter [F(1, 119) = 144.9, p < 0.001], and also significantly higher in urban compared to rural birds [F(1, 119) = 14.48, p < 0.001; Figure 6A].
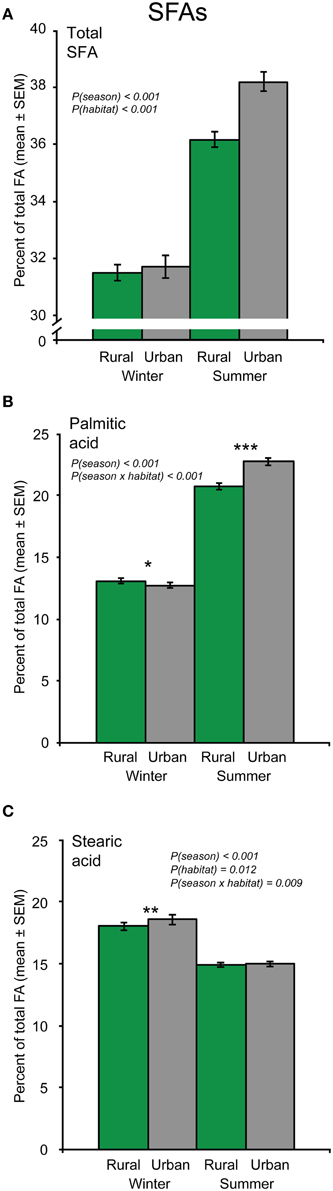
Figure 6. The proportion of saturated fatty acids (SFA) in great tit blood plasma sampled during winter and summer in the urban and rural habitats (Ntotal = 126). (A) Total SFA (i.e., the sum of myristic-, palmitic-, stearic-, and arachidic acid), (B) palmitic acid, and (C) stearic acid. Significant effects from the models are indicated by the p-values. In case of significant season × habitat interactions, fatty acid (FA) proportions of birds from the two habitats were also analyzed separately for each season, and significant differences between habitats within a season are indicated by asterisks (*p < 0.05; **p < 0.01; ***p < 0.001). Note that the Y-axis scale differs between figure panels.
Palmitic- and stearic acid were two of the most abundant of all FAs. These SFAs showed different patterns of variation between seasons and habitats. Across habitats, the relative amount of palmitic acid was significantly higher in summer than in winter [F(1, 119) = 786.0, p < 0.001; Figure 6B]. However, seasonal variation in palmitic acid content differed depending on habitat [season × habitat interaction: F(1, 119) = 27.28, p < 0.001]. Specifically, rural birds had a higher relative amount of this SFA than urban birds during winter, whereas the opposite pattern was found during summer (Figure 6B). Thus, the seasonal variation in palmitic acid was larger for the urban birds.
In contrast, the proportion of stearic acid was significantly higher in birds during winter than during summer [F(1, 119) = 128.8, p < 0.001], and in urban compared to rural birds [F(1, 119) = 6.59, p = 0.012]. As for palmitic acid, the seasonal variation in stearic acid differed between the two habitats [season × habitat interaction: F(1, 119) = 7.05, p = 0.009], with urban birds having higher levels of stearic acid than rural birds only during winter (Figure 6C). Accordingly, the seasonal variation in stearic acid was larger in urban birds. The proportion of stearic acid was negatively related to body condition, but only during winter [body condition × season interaction: F(1, 119) = 9.28, p = 0.003; Figure 3D].
Discussion
This is the first study to investigate seasonal variation in FA composition in animals from an urbanization perspective. To this end, we demonstrate that most FAs with known physiological functions differ in relative abundance in great tit plasma between birds from urban and rural habitats and on a seasonal basis. We discuss these findings in relation to our predictions concerning seasonality (i–iv) and habitat differences (v–vi), and conclude by discussing the physiological implications of the observed variation and give directions for future experiments.
Seasonal Variation (Predictions I–IV)
The relative amount of eight of the nine analyzed FAs in great tit blood plasma differed between seasons in both urban and rural habitats. In accordance with our first prediction (i), birds sampled during winter had higher relative amounts of oleic- and linoleic acid, which are abundant in their main winter food (seeds and nuts, Beare-Rogers et al., 2001; Table 1). In particular, the large seasonal variation in plasma levels of linoleic acid is likely to be explained by dietary intake because this FA cannot be biosynthesized (Larsson et al., 2004). In addition, we found a higher relative content of arachidonic acid in winter, which is likely a result of biosynthesis from linoleic acid (Sprecher, 2002), possibly induced by the lower ambient temperature in this season (Ben-Hamo et al., 2013). This hypothesis is in line with the observation that the arachidonic acid level in muscles of C. japonica increased after birds were fed a diet devoid of arachidonic acid, but rich in linoleic acid (Ben-Hamo et al., 2011).
During late spring to early summer, great tits feed mainly on caterpillars (Perrins, 1991), which contain a high content of the ω-3 PUFA αLNA (Table 1). Therefore, we predicted (ii) that the relative plasma levels of αLNA, and the longer-chained ω-3 PUFAs that are biosynthesized from αLNA (Sprecher, 2002), would be the highest in summer. In line with this prediction, the relative amounts of three ω-3 PUFAs (αLNA, EPA, and DPA) were much higher during summer than during winter in both urban and rural birds. The higher relative content of the essential αLNA is likely to directly reflect intake from food, whereas the increased levels of EPA and DPA might be explained both by food intake and biosynthesis (Larsson et al., 2004).
The ratio between total ω-6 and ω-3 PUFAs is important for animals because these PUFAs have different capacities to enhance oxidative stress and opposing effects on inflammatory responses. For instance, ω-6 PUFAs are used in the biosynthesis of pro-inflammatory eicosanoids, whereas ω-3 PUFAs are used in the production of anti-inflammatory eicosanoids (Larsson et al., 2004; Cherian, 2007). We predicted (iii) that the total ω-6/ω-3 ratio would be higher in plasma during winter than during summer, because caterpillars have a much lower ω-6/ω-3 ratio than seeds (Table 1). Accordingly, we found a ten-fold decrease in this ratio from winter to summer, which likely is due to the dietary change.
A strong correlation between FA levels in animal plasma and FA levels of ingested food has previously been established (Hulbert and Abbott, 2012). However, although the seasonal differences in plasma FA abundance mostly correlated well with the abundance of the FAs in the winter- or summer diet of great tits in the present study, we cannot rule out the possibility that selective mobilization of FAs also contributes to the variation (Raclot, 2003; Price et al., 2008, 2013). Selective mobilization might occur to meet different physiological demands, such as thermoregulation during winter and reproduction during summer. Future experiments should address the relative contribution of dietary intake and FA mobilization on blood plasma FA composition, and whether the seasonal variation in FA composition has any effect on the above-mentioned physiological processes. In addition, although previous studies on other species have shown that the FA composition of membrane phospholipids in organs also is affected by diet (Von Schacky et al., 1985; Abbott et al., 2010; Ben-Hamo et al., 2011), phospholipid composition appears less responsive to dietary change as compared to the composition of triglycerides in the plasma (Hulbert and Abbott, 2012). Thus, future studies on the great tit are needed to investigate the correlations between plasma and phospholipid FA composition of organs in order to better understand how the physiological processes are affected by the dietary differences of the study species.
Ambient temperature also affects animals' FA composition. In response to decreased ambient temperature, ectotherms incorporate a lower proportion of SFAs and a higher proportion of long-chained PUFAs into their cell membranes to achieve constant membrane fluidity (Sinensky, 1974; Cossins and Macdonald, 1989; Hazel, 1995). Because great tits sometimes lower their body temperature during cold nights (Haftorn, 1972; Nord et al., 2013), we predicted (iv) that the proportion of total SFA in blood plasma would be lower in birds during winter than during summer, assuming that plasma SFA levels also are altered in response to changes in ambient temperature. Our results follow this prediction. Similarly, a lower proportion of SFA in depot fat during winter has been found in several passerine species, including great tits (West and Meng, 1968; Barnett, 1970; Palokangas and Vihko, 1972), suggesting that this is a general phenomenon in birds. In addition to the change in total SFA, Balnave (1973) showed that the ratio between palmitic- and stearic acid increases with ambient temperature in chicken (Gallus g. domesticus). This pattern fits our observation of seasonal trends in the relative abundance of these FAs, and those of Palokangas and Vihko (1972) on seasonal changes in great tit fat depot composition. Unfortunately, Palokangas and Vihko (1972) only analyzed C16 and C18 FAs, making it difficult to further compare the seasonal FA variation in great tit fat tissue (Palokangas and Vihko, 1972) with that in blood plasma (this study). However, while the seasonal differences in SFA levels follows our predictions regarding effects of ambient temperature, we cannot rule out any influence of the diet change (which co-varies with ambient temperature) or selective mobilization of certain FAs due to seasonally different physiological demands.
Variation between Urban and Rural Habitats (Predictions V and VI)
While Fokidis et al. (2011) and Davies et al. (2013) found no significant differences in total levels of plasma triglycerides or free fatty acids, respectively, between urban and dessert populations of two species of passerines, our study revealed that the FA composition differed significantly between urban and rural populations of great tits. In line with our prediction (v), eight of the nine FAs differed between urban and rural birds during both, or one, of the seasons. The relative content of the essential linoleic acid was higher in the rural birds across seasons. This may be explained by a higher diversity or availability of natural seeds that are rich in this particular FA in the rural environment, possibly in combination with seeds from agricultural storages that urban birds do not have access to. However, given that urban birds have access to different seeds at feeding tables during winter, this result was somewhat surprising. In winter, the ω-3 PUFAs αLNA, EPA, and DPA were more abundant in rural birds, whereas the urban birds had a higher relative abundance of the ω-6 PUFA arachidonic acid. This indicates that the rural birds incorporated more insect prey (e.g., overwintering moth larvae or pupae) in their winter diet than did urban birds (cf. Isaksson et al., 2015), whereas urban birds either up-regulated biosynthesis or mobilization of arachidonic acid to a larger extent, or received it from a dietary source (e.g., spiders; Table 1).
During summer, rural birds had higher relative amounts of EPA than urban birds, while the opposite pattern was found for DHA. Because EPA and DHA are inter-convertible (Larsson et al., 2004), this might reflect habitat-specific biosynthetic regulation of these two FAs. In addition, DHA was negatively related to body condition, which was the lowest in summer, especially in the urban habitat. Potential implications of this relationship and the habitat-specific differences in EPA and DHA content remain to be elucidated. Since αLNA, which is obtained mainly from caterpillars, did not differ between urban and rural birds in the summer, it is unlikely that the habitat differences in EPA and DHA would be due to a difference in caterpillar phenology.
Interestingly, the proportion of total SFA was higher in urban than rural birds, especially during summer. The average ambient temperature in the urban study site was around 1.5°C higher than that in the rural site during both seasons. It is, however, questionable if this small temperature difference underlies the difference in total SFA between habitats. It is also uncertain if such a small difference in average ambient temperature is important for homeoviscous adaptation in a species that exhibits relatively shallow hypothermia. However, the reported average ambient temperatures are measured by weather stations under standardized conditions and, thus, might not accurately predict the actual temperature differences that birds from the two habitats experience. Temperature variation between habitats might be larger, given that many of the rural birds live within dense forests with little sunlight coming through, and urban birds may find shelters during winter that are warmer than the average ambient temperatures. Another, perhaps more plausible, explanation is habitat-related differences in SFA content of diets (Table 1).
Finally, we predicted (vi) that the extent of any seasonal FA variation would differ between urban and rural birds due to habitat-related differences in diet and temperature. In line with this, the relative plasma content of all the five FAs that showed significant season × habitat interactions differed the most between seasons in urban birds. This suggests that ecological factors, such as food availability and diversity, might be more seasonally variable in urban habitats.
Implications for Physiology, Health, and Future Experiments
In line with previous work (e.g., Barnett, 1970; Conway et al., 1994; Egeler and Williams, 2000; Pierce and McWilliams, 2005; Isaksson et al., 2015), we have shown that the FA composition of birds varies between habitats and seasons. We suggest that this variation, to a large extent, is explained by differences in natural and anthropogenic food sources and ambient temperature. Due to the multiple context-dependent physiological effects of FA nutrition (Hulbert and Abbott, 2012), such differences, and perhaps especially those that are non-natural (i.e., occur for anthropogenic reasons), might have a larger impact on urban birds and other animals than previously acknowledged (Orell, 1989; Chamberlain et al., 2009; Harrison et al., 2010; Plummer et al., 2013).
For example, oleic acid and arachidonic acid—the latter synthesized from the dietary linoleic acid- have been shown to affect animals' thermoregulatory physiology. Oleic- and linoleic acid are the two dominating FAs in seeds provided by humans, such as sunflower seeds. In C. japonica, the liver content of oleic acid decreased, whereas liver arachidonic acid content increased, in response to decreased ambient temperature, thus suggesting a role for these FAs in cold acclimation (Ben-Hamo et al., 2013). In line with this, C. japonica with a higher content of oleic acid in the liver and a lower content of arachidonic acid in skeletal muscle reduced body temperature more during fasting-induced rest-phase hypothermia (Ben-Hamo et al., 2011). Interestingly, we found that the proportions of oleic- and arachidonic acid in plasma were positively and negatively related, respectively, to birds' body condition in winter, i.e., when thermoregulation is energetically more demanding. This might suggest that birds in higher condition might be more capable of increasing the depth of rest-phase hypothermia, thereby achieving a more energetically efficient thermoregulation than birds in lower condition. However, Nord et al. (2011) found that high-condition individuals of the closely related blue tit (Cyanistes caeruleus) maintained a higher nocturnal body temperature than did low-condition individuals in the wild, and birds with ad lib. food availability did not enter hypothermia at all when maintained in outdoor enclosures (Nord et al., 2009). These opposing observations in combination with the associations found in the present study suggest that the two FAs affect fasting-induced and natural hypothermic responses differently. Another complicating factor is that we measured body condition when birds were caught in the morning, which might not reflect the body condition during the night when hypothermia takes place. An alternative interpretation for our results is that individuals might be in high condition because their oleic- and arachidonic acid composition improves thermoregulatory performance, which in turn allows for fat reserve retention. Thus, future studies need to experimentally address the effect of dietary oleic- and arachidonic acid levels on body condition and body temperature regulation in temperate zone birds.
Another result with potential health implications is the higher relative abundance of the pro-inflammatory arachidonic acid in urban birds in winter (along with their lower levels of anti-inflammatory ω-3 PUFAs). Because urban birds generally are more exposed to antigens such as pollutants and certain pathogens (e.g., Salmonella and Mycoplasma) (Bradley and Altizer, 2007), an arachidonic acid-mediated inflammatory response could enhance the negative health effects of such antigens (Isaksson, 2015). Importantly, the negative relationship between the proportion of arachidonic acid and body condition suggests that birds in low condition might not only be constrained by reduced thermoregulatory capacity, but could also be more susceptible to any negative effects of a pro-inflammatory response. Finally, the strong seasonal effect on the total ω-6/ω-3 ratio in both habitats suggests that winter birds overall are more sensitive than summer birds to the effects of pro-inflammatory antigens, such as nitrogen oxides and particulate matter from traffic fumes, perhaps especially since birds in winter might have reduced antibody-mediated immunocompetence (Svensson et al., 1998; Nord et al., 2014).
In conclusion, this study reveals large differences in great tit plasma FA composition between urban and rural populations and between winter and summer. Most of this variation seems to follow our predictions about habitat-related and seasonal differences in diet composition (although the change in diet co-varied with ambient temperature). We suggest that it is likely that feeding from human-provided food affects the FA composition also of other bird and animal species that occupy cities; thus the implications of our results extend beyond the study species and the avian taxon. Many of the FAs we identified in great tit blood plasma play crucial roles in evolutionarily conserved physiological processes with links to animal nutrition and survival. Accordingly, any possible interactive effects between seasonal changes in FA composition and other urban stressors should be taken into consideration in future studies on the consequences of urbanization for wildlife. Future studies should also compare FA composition between different populations within urban and rural habitats in order to better quantify the effect of urbanization per se in comparison to FA variation due to within-urban or within-rural habitat heterogeneity.
Author Contributions
CI conceived the study and designed the experiment. AN, CI, and PS performed the fieldwork. CI and MA conducted lab work. HW developed the methodology and contributed to lab work and chemical analysis. MA quantified and analyzed the GC/MS data. MA performed statistical analyses with support from CI. MA drafted the manuscript with CI and AN contributing substantially to the final text; other authors provided editorial and scientific advice.
Conflict of Interest Statement
The authors declare that the research was conducted in the absence of any commercial or financial relationships that could be construed as a potential conflict of interest.
Acknowledgments
We are grateful to Maria von Post for assistance in the laboratory, and Christer Löfstedt for hosting MA and HW. We also acknowledge SYSAV for providing nest boxes, the City of Malmö for permission to establish nest box populations within the city and for access to field localities, Sydvatten AB for permission to work on their premises, and Lennart Blomquist and Ragnar Dyrlund for help with nest boxes and field work in Malmö. CI was funded by a Marie Curie Career Integration Grant (FP7-CIG), Carl Trygger's Foundation, the Royal Physiographic Society in Lund, and the Crafoord Foundation. AN was supported by the Swedish Research Council [grant no. 637-2013-7442]. All applicable institutional and/or national guidelines for the care and use of animals were followed (ethical permit number D454 12:1 and ringing licence 0681 (CI)).
Supplementary Material
The Supplementary Material for this article can be found online at: http://journal.frontiersin.org/article/10.3389/fevo.2015.00093
Footnotes
1. ^Freiholtz, E. (2013). Available online at: http://www.temperatur.nu
References
Abbott, S. K., Else, P. L., and Hulbert, A. J. (2010). Membrane fatty acid composition of rat skeletal muscle is most responsive to the balance of dietary n-3 and n-6 PUFA. Br. J. Nutr. 103, 522–529. doi: 10.1017/S0007114509992133
Austin, J. E. (1993). Fatty acid composition of fat depots in wintering Canada geese. Wilson Bull. 105, 339–347.
Balnave, D. (1973). The influence of environmental temperature on the fatty acid composition of liver and carcase lipids in the young male chick fed on equalized and ad lib. intakes. Comp. Biochem. Physiol. A Physiol. 44, 1075–1083. doi: 10.1016/0300-9629(73)90245-4
Barnett, L. B. (1970). Seasonal changes in temperature acclimatization of the house sparrow, Passer domesticus. Comp. Biochem. Physiol. 33, 559–578. doi: 10.1016/0010-406X(70)90371-3
Beare-Rogers, J. L., Dieffenbacher, A., and Holm, J. V. (2001). Lexicon of lipid nutrition (IUPAC Technical Report). Pure Appl. Chem. 73, 685–744. doi: 10.1351/pac200173040685
Becker, R. (2008). “Fatty acids in food cereal grains and grain products,” in Fatty acids in foods and their health implications, ed C. K. Chow (Boca Raton, FL: CRC Press; Taylor & Francis Group), 303–316.
Ben-Hamo, M., McCue, M. D., Khozin-Goldberg, I., Mcwilliams, S. R., and Pinshow, B. (2013). Ambient temperature and nutritional stress influence fatty acid composition of structural and fuel lipids in Japanese quail (Coturnix japonica) tissues. Comp. Biochem. Physiol. A Mol. Integr. Physiol. 166, 244–250. doi: 10.1016/j.cbpa.2013.06.017
Ben-Hamo, M., McCue, M. D., Mcwilliams, S. R., and Pinshow, B. (2011). Dietary fatty acid composition influences tissue lipid profiles and regulation of body temperature in Japanese quail. J. Comp. Physiol. B 181, 807–816. doi: 10.1007/s00360-011-0558-2
Bradley, C. A., and Altizer, S. (2007). Urbanization and the ecology of wildlife diseases. Trends Ecol. Evol. 22, 95–102. doi: 10.1016/j.tree.2006.11.001
Chamberlain, D. E., Cannon, A. R., Toms, M. P., Leech, D. I., Hatchwell, B. J., and Gaston, K. J. (2009). Avian productivity in urban landscapes: a review and meta−analysis. Ibis 151, 1–18. doi: 10.1111/j.1474-919X.2008.00899.x
Cherian, G. (2007). Metabolic and cardiovascular diseases in poultry: role of dietary lipids. Poult. Sci. 86, 1012–1016. doi: 10.1093/ps/86.5.1012
Cherian, G., Bautista-Ortega, J., and Goeger, D. E. (2009). Maternal dietary n-3 fatty acids alter cardiac ventricle fatty acid composition, prostaglandin and thromboxane production in growing chicks. Prostaglandins Leukot. Essent. Fatty Acids 80, 297–303. doi: 10.1016/j.plefa.2009.02.006
Conway, C. J., Eddleman, W. R., and Simpson, K. L. (1994). Seasonal changes in fatty acid composition of the wood thrush. Condor 96, 791–794. doi: 10.2307/1369482
Cossins, A. R., and Macdonald, A. G. (1989). The adaptation of biological membranes to temperature and pressure: fish from the deep and cold. J. Bioenerg. Biomembr. 21, 115–135. doi: 10.1007/BF00762215
Crespo, N., and Esteve-Garcia, E. (2001). Dietary fatty acid profile modifies abdominal fat deposition in broiler chickens. Poult. Sci. 80, 71–78. doi: 10.1093/ps/80.1.71
Davies, S., Rodriguez, N. S., Sweazea, K. L., and Deviche, P. (2013). The effect of acute stress and long-term corticosteroid administration on plasma metabolites in an urban and desert songbird. Physiol. Biochem. Zool. 86, 47–60. doi: 10.1086/667990
Egeler, O., and Williams, T. D. (2000). Seasonal, age, and sex-related variation in fatty-acid composition of depot fat in relation to migration in western sandpipers. Auk 117, 110–119. doi: 10.1642/0004-8038(2000)117[0110:SAASRV]2.0.CO;2
Fokidis, H. B., Hurley, L., Rogowski, C., Sweazea, K., and Deviche, P. (2011). Effects of captivity and body condition on plasma corticosterone, locomotor behavior, and plasma metabolites in curve-billed thrashers. Physiol. Biochem. Zool. 84, 595–606. doi: 10.1086/662068
Gomes, A., Couto, D., Alves, A., Dias, I., Freitas, M., Porto, G., et al. (2012). Trihydroxyflavones with antioxidant and anti−inflammatory efficacy. Biofactors 38, 378–386. doi: 10.1002/biof.1033
Haftorn, S. (1972). Hypothermia of tits in the Arctic winter. Ornis Scand. 3, 153–166. doi: 10.2307/3676222
Han, X., and Naeher, L. P. (2006). A review of traffic-related air pollution exposure assessment studies in the developing world. Environ. Int. 32, 106–120. doi: 10.1016/j.envint.2005.05.020
Harrison, T. J. E., Smith, J. A., Martin, G. R., Chamberlain, D. E., Bearhop, S., Robb, G. N., et al. (2010). Does food supplementation really enhance productivity of breeding birds? Oecologia 164, 311–320. doi: 10.1007/s00442-010-1645-x
Hazel, J. R. (1995). Thermal adaptation in biological membranes: is homeoviscous adaptation the explanation? Annu. Rev. Physiol. 57, 19–42. doi: 10.1146/annurev.ph.57.030195.000315
Hu, L., and Brunsell, N. A. (2015). A new perspective to assess the urban heat island through remotely sensed atmospheric profiles. Remote Sens. Environ. 158, 393–406. doi: 10.1016/j.rse.2014.10.022
Hulbert, A., and Abbott, S. K. (2012). Nutritional ecology of essential fatty acids: an evolutionary perspective. Aust. J. Zool. 59, 369–379. doi: 10.1071/ZO11064
Hulbert, A. J., and Else, P. L. (1999). Membranes as possible pacemakers of metabolism. J. Theor. Biol. 199, 257–274. doi: 10.1006/jtbi.1999.0955
Isaksson, C. (2010). Pollution and its impact on wild animals: a meta-analysis on oxidative stress. Ecohealth 7, 342–350. doi: 10.1007/s10393-010-0345-7
Isaksson, C. (2015). Urbanisation, oxidative stress and inflammation: a question of evolving, acclimatizing or coping with urban environmental stress. Funct. Ecol. 29, 913–923. doi: 10.1111/1365-2435.12477
Isaksson, C., Hanson, M. A., and Burdge, G. C. (2015). The effects of spatial and temporal ecological variation on fatty acid compositions of wild great tits (Parus major). J. Avian Biol. 46, 245–253. doi: 10.1111/jav.00409
Isaksson, C., Örnborg, J., Stephensen, E., and Andersson, S. (2005). Plasma glutathione and carotenoid coloration as potential biomarkers of environmental stress in great tits. Ecohealth 2, 138–146. doi: 10.1007/s10393-005-3869-5
Larsson, S. C., Kumlin, M., Ingelman-Sundberg, M., and Wolk, A. (2004). Dietary long-chain n- 3 fatty acids for the prevention of cancer: a review of potential mechanisms. Am. J. Clin. Nutr. 79, 935–945.
Lerner, E., Shug, A. L., Elson, C., and Shrago, E. (1972). Reversible inhibition of adenine nucleotide translocation by long chain fatty acyl coenzyme A esters in liver mitochondria of diabetic and hibernating animals. J. Biol. Chem. 247, 1513–1519.
Marzluff, J. M., Bowman, R., and Donnelly, R. E. (2001). Avian Conservation and Ecology in an Urbanizing World. New York, NY: Kluwer. doi: 10.1007/978-1-4615-1531-9
McKechnie, A. E., and Lovegrove, B. G. (2002). Avian facultative hypothermic responses: a review. Condor 104, 705–724. doi: 10.1650/0010-5422(2002)104[0705:AFHRAR]2.0.CO;2
McWilliams, S. R., Guglielmo, C., Pierce, B., and Klaassen, M. (2004). Flying, fasting, and feeding in birds during migration: a nutritional and physiological ecology perspective. J. Avian Biol. 35, 377–393. doi: 10.1111/j.0908-8857.2004.03378.x
Nord, A., Chiriac, S., Hasselquist, D., and Nilsson, J. Å. (2013). Endotoxin injection attenuates rest−phase hypothermia in wintering great tits through the onset of fever. Funct. Ecol. 27, 236–244. doi: 10.1111/1365-2435.12003
Nord, A., Nilsson, J. F., and Nilsson, J.-Å. (2011). Nocturnal body temperature in wintering blue tits is affected by roost-site temperature and body reserves. Oecologia 167, 21–25. doi: 10.1007/s00442-011-1972-6
Nord, A., Nilsson, J. F., Sandell, M. I., and Nilsson, J.-Å. (2009). Patterns and dynamics of rest-phase hypothermia in wild and captive blue tits during winter. J. Comp. Physiol. B 179, 737–745. doi: 10.1007/s00360-009-0357-1
Nord, A., Sköld−Chiriac, S., Hasselquist, D., and Nilsson, J. Å. (2014). A tradeoff between perceived predation risk and energy conservation revealed by an immune challenge experiment. Oikos 123, 1091–1100. doi: 10.1111/oik.01221
Oke, T. R. (1973). City size and the urban heat island. Atmos. Environ. 7, 769–779. doi: 10.1016/0004-6981(73)90140-6
Orell, M. (1989). Population fluctuations and survival of great tits Parus major dependent on food supplied by man in winter. Ibis 131, 112–127. doi: 10.1111/j.1474-919X.1989.tb02750.x
Palokangas, R., and Vihko, V. (1972). Fatty acids in the subcutaneous depot fat tissue of the titmouse (Parus major L.) in winter and in summer. Comp. Biochem. Physiol. B Comp. Biochem. 41, 925–929. doi: 10.1016/0305-0491(72)90104-6
Peig, J., and Green, A. J. (2009). New perspectives for estimating body condition from mass/length data: the scaled mass index as an alternative method. Oikos 118, 1883–1891. doi: 10.1111/j.1600-0706.2009.17643.x
Perrins, C. M. (1991). Tits and their caterpillar food supply. Ibis 133, 49–54. doi: 10.1111/j.1474-919X.1991.tb07668.x
Pierce, B. J., and McWilliams, S. R. (2005). Seasonal changes in composition of lipid stores in migratory birds: causes and consequences. Condor 107, 269–279. doi: 10.1650/7809
Pierce, B. J., McWilliams, S. R., O'Connor, T. P., Place, A. R., and Guglielmo, C. G. (2005). Effect of dietary fatty acid composition on depot fat and exercise performance in a migrating songbird, the red-eyed vireo. J. Exp. Biol. 208, 1277–1285. doi: 10.1242/jeb.01493
Pierce, B. J., McWilliams, S. R., Place, A. R., and Huguenin, M. A. (2004). Diet preferences for specific fatty acids and their effect on composition of fat reserves in migratory Red-eyed Vireos (Vireo olivaceous). Comp. Biochem. Physiol. A Mol. Integr. Physiol. 138, 503–514. doi: 10.1016/j.cbpb.2004.06.014
Plummer, K. E., Bearhop, S., Leech, D. I., Chamberlain, D. E., and Blount, J. D. (2013). Fat provisioning in winter impairs egg production during the following spring: a landscape−scale study of blue tits. J. Anim. Ecol. 82, 673–682. doi: 10.1111/1365-2656.12025
Price, E. R., Armstrong, C., Guglielmo, C. G., and Staples, J. F. (2013). Selective mobilization of saturated fatty acids in isolated adipocytes of hibernating 13-lined ground squirrels Ictidomys tridecemlineatus. Physiol. Biochem. Zool. 86, 205–212. doi: 10.1086/668892
Price, E. R., Krokfors, A., and Guglielmo, C. G. (2008). Selective mobilization of fatty acids from adipose tissue in migratory birds. J. Exp. Biol. 211, 29–34. doi: 10.1242/jeb.009340
Raclot, T. (2003). Selective mobilization of fatty acids from adipose tissue triacylglycerols. Prog. Lipid. Res. 42, 257–288. doi: 10.1016/S0163-7827(02)00066-8
Robb, G. N., Mcdonald, R. A., Chamberlain, D. E., and Bearhop, S. (2008). Food for thought: supplementary feeding as a driver of ecological change in avian populations. Front. Ecol. Environ. 6, 476–484. doi: 10.1890/060152
Schleucher, E. (2004). Torpor in birds: taxonomy, energetics, and ecology. Physiol. Biochem. Zool. 77, 942–949. doi: 10.1086/423744
Seebacher, F., Brand, M. D., Else, P. L., Guderley, H., Hulbert, A. J., and Moyes, C. D. (2010). Plasticity of oxidative metabolism in variable climates: molecular mechanisms. Physiol. Biochem. Zool. 83, 721–732. doi: 10.1086/649964
Seress, G., Lipovits, A., Bókony, V., and Czúni, L. (2014). Quantifying the urban gradient: a practical method for broad measurements. Landsc. Urban Plann. 131, 42–50. doi: 10.1016/j.landurbplan.2014.07.010
Sinensky, M. (1974). Homeoviscous adaptation—a homeostatic process that regulates the viscosity of membrane lipids in Escherichia coli. Proc. Natl. Acad. Sci. U.S.A. 71, 522–525. doi: 10.1073/pnas.71.2.522
Sprecher, H. (2002). The roles of anabolic and catabolic reactions in the synthesis and recycling of polyunsaturated fatty acids. Prostaglandins, Leukot. Essent. Fatty Acids 67, 79–83. doi: 10.1054/plef.2002.0402
Stroh, E., Harrie, L., and Gustafsson, S. (2007). A study of spatial resolution in pollution exposure modelling. Int. J. Health Geogr. 6, 19. doi: 10.1186/1476-072X-6-19
Svensson, E., Råberg, L., Koch, C., and Hasselquist, D. (1998). Energetic stress, immunosuppression and the costs of an antibody response. Funct. Ecol. 12, 912–919. doi: 10.1046/j.1365-2435.1998.00271.x
Tinoco, J. (1982). Dietary requirements and functions of α-linolenic acid in animals. Prog. Lipid Res. 21, 1–45. doi: 10.1016/0163-7827(82)90015-7
von Schacky, C., Fischer, S., and Weber, P. C. (1985). Long-term effects of dietary marine omega-3 fatty acids upon plasma and cellular lipids, platelet function, and eicosanoid formation in humans. J. Clin. Investig. 76, 1626–1631. doi: 10.1172/JCI112147
Warner, K., Vick, B., Kleingartner, L., Isaak, R., and Doroff, K. (2003). “Compositions of sunflower, Nusun (mid-oleic sunflower) and high-oleic sunflower oils,” in Proc. Sunflower Res. Workshop (Fargo, ND).
Warton, D. I., and Hui, F. K. C. (2011). The arcsine is asinine: the analysis of proportions in ecology. Ecology 92, 3–10. doi: 10.1890/10-0340.1
West, G. C., and Meng, M. S. (1968). The effect of diet and captivity on the fatty acid composition of redpoll (Acanthis flammea) depot fats. Comp. Biochem. Physiol. 25, 535–540. doi: 10.1016/0010-406X(68)90364-2
Yom-Tov, Y., and Tietz, A. (1978). The effect of diet, ambient temperature and day length on the fatty acid composition in the depot fat of the European starling (Sturnus vulgaris) and the rock partridge (Alectoris chucar). Comp. Biochem. Physiol. A Physiol. 60, 161–164. doi: 10.1016/0300-9629(78)90222-0
Keywords: bird, blood plasma, diet, inflammation, nutrition, polyunsaturated fatty acid, temperature, urbanization
Citation: Andersson MN, Wang H-L, Nord A, Salmón P and Isaksson C (2015) Composition of physiologically important fatty acids in great tits differs between urban and rural populations on a seasonal basis. Front. Ecol. Evol. 3:93. doi: 10.3389/fevo.2015.00093
Received: 20 May 2015; Accepted: 24 July 2015;
Published: 07 August 2015.
Edited by:
G. Darrel Jenerette, University of California, Riverside, USAReviewed by:
Iryna Dronova, University of California, Berkeley, USAScott Davies, Virginia Polytechnic Institute and State University, USA
Copyright © 2015 Andersson, Wang, Nord, Salmón and Isaksson. This is an open-access article distributed under the terms of the Creative Commons Attribution License (CC BY). The use, distribution or reproduction in other forums is permitted, provided the original author(s) or licensor are credited and that the original publication in this journal is cited, in accordance with accepted academic practice. No use, distribution or reproduction is permitted which does not comply with these terms.
*Correspondence: Caroline Isaksson, Department of Biology, Lund University, Sölvegatan 37, SE-22362 Lund, Sweden,Y2Fyb2xpbmUuaXNha3Nzb25AYmlvbC5sdS5zZQ==
†Present Address: Andreas Nord, Department of Arctic and Marine Biology, Arctic Animal Physiology, Arktisk Biologibygget, University of Tromsø, Tromsø, Norway