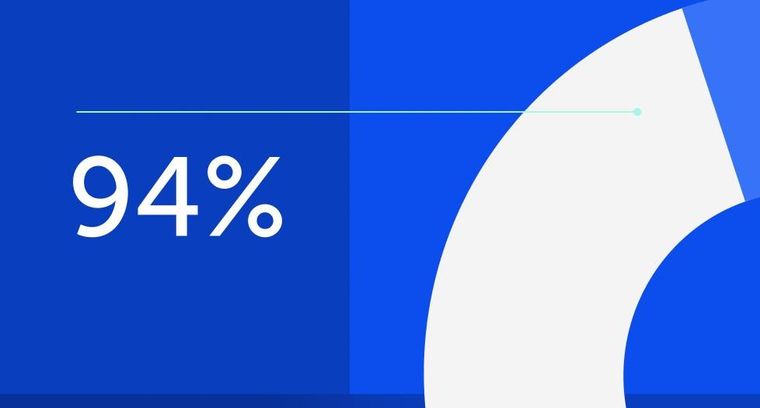
94% of researchers rate our articles as excellent or good
Learn more about the work of our research integrity team to safeguard the quality of each article we publish.
Find out more
ORIGINAL RESEARCH article
Front. Ecol. Evol., 15 May 2015
Sec. Paleoecology
Volume 3 - 2015 | https://doi.org/10.3389/fevo.2015.00046
Understanding the factors controlling the expression of longevity in trees is still an outstanding challenge for tree biologists and forest ecologists. We gathered tree-ring data and literature for broadleaf deciduous (BD) temperate trees growing in closed-canopy old-growth (OG) forests in the Northern Hemisphere to explore the role of geographic patterns, climate variability, and growth rates on longevity. Our pan-continental analysis, covering 25 species from 12 genera, showed that 300–400 years can be considered a baseline threshold for maximum tree lifespan in many temperate deciduous forests. Maximum age varies greatly in relation to environmental features, even within the same species. Tree longevity is generally promoted by reduced growth rates across large genetic differences and environmental gradients. We argue that slower growth rates, and the associated smaller size, provide trees with an advantage against biotic and abiotic disturbance agents, supporting the idea that size, not age, is the main constraint to tree longevity. The oldest trees were living most of their life in subordinate canopy conditions and/or within primary forests in cool temperate environments and outside major storm tracks. Very old trees are thus characterized by slow growth and often live in forests with harsh site conditions and infrequent disturbance events that kill much of the trees. Temperature inversely controls the expression of longevity in mesophilous species (Fagus spp.), but its role in Quercus spp. is more complex and warrants further research in disturbance ecology. Biological, ecological, and historical drivers must be considered to understand the constraints imposed to longevity within different forest landscapes.
Identifying the biological and ecological drivers of forest tree longevity is a long-standing challenge. Exploring species longevity, i.e. the maximum lifespan of trees detected under specific environmental conditions, is an important step in the pursuit of a mechanistic understanding of tree life-history variation and plant geography, as they are related to morphological and physiological traits (e.g., Easdale and Healey, 2009; Stahl et al., 2014). Recent literature has revealed that within-species variation in traits is greater than previously supposed (Richardson et al., 2013), and such large within-species variation is also observed with tree longevity (Di Filippo et al., 2012). Although it is generally considered fixed for each species in ecological modeling, longevity appears to be tightly related to environmental features (e.g., Rotheli et al., 2012). While it has long been known that the age-size relationships are site-dependent (e.g., Suarez et al., 2008), we still have a poor understanding of how a species' longevity varies between forest stands and how it's driven by the environment (Di Filippo et al., 2012; Rohner et al., 2013). A quantitative understanding of the factors controlling tree growth and longevity in different environments is of utmost importance to (1) develop predictive models of tree longevity (Rohner et al., 2013) and forest demography (Stephenson et al., 2011), (2) comprehend ecosystem functioning under climate-change (Michaletz et al., 2014), and (3) develop sound environmental resource management and nature conservation strategies. Tree longevity's ecological counterpart is forest mortality, for which the causes of geographic variation have been explored only in a handful of studies (Stephenson et al., 2011).
Longevity studies are complex as they are intimately connected to the discovery of old-growth (OG) forests, a difficult task in the temperate forest biome where intense human activity has deeply transformed the natural landscape. Fragmentation and use of the forest landscape have affected the presence of OG forests, especially primary ones (Frelich and Reich, 2003). A macroecological approach based on large-scale ecological networks is thus required to better understand the drivers of tree longevity (Di Filippo et al., 2012). Over the last few decades, many dendroecological studies worldwide have generated a large amount of data from different species and environments. These campaigns are invaluable to explore the link between growth dynamics and longevity. Dendroecological studies of OG forests often require substantial scientific efforts, not only due to crossdating difficulties connected to competitive growth suppression and release (Black et al., 2008), but also due to increasing heart rot in older broadleaf tree species (Ranius et al., 2009). These factors limit our full comprehension of tree longevity under natural conditions.
Few meta-analysis studies have attempted to explore the patterns of longevity variation at the biome-scale (e.g., temperate deciduous forest) (Loehle, 1988; Bugmann and Bigler, 2011). In general, the maximum age found among temperate deciduous OG forests can vary widely, and do not seem to differ from other OG temperate and tropical forest types in terms of median age (~300 years) and interquartile age range (200–400 years) (Wirth et al., 2009). Thousand-year old oak, plane, linden and chestnut trees are often reported in the gray literature (e.g., Plietzsch, 2009), even when pollarded in grazed woodland (Rozas, 2005; Drobyshev and Niklasson, 2010; Moir, 2012). In contrast, tree-ring studies have provided evidence that most deciduous tree species rarely exceed 4–5 centuries of age (e.g., OldList: www.rmtrr.org/oldlist.htm; Eastern Oldlist, www.ldeo.columbia.edu/~adk/ oldlisteast; Schweingruber and Wirth, 2009). In effect, studies on tree longevity must focus on measurement of tree age from annual growth rings so that a sound scientific assessment of longevity can be quantified.
Tree longevity patterns within-species can vary according to environmental (e.g., elevational) gradients (Di Filippo et al., 2012; Rotheli et al., 2012). It has long been known that there is a negative correlation between growth rates and longevity in both angiosperm and gymnosperm trees (Schulman, 1954; Black et al., 2008; Bigler and Veblen, 2009; Fritts, 2012). Indeed, this relationship appears to hold, even within-species growing along environmental gradients, as bioclimate and site fertility exert a strong control over tree growth (Di Filippo et al., 2012; Rotheli et al., 2012). Harsher environmental conditions seem to promote tree longevity via reduced tree growth, but the full understanding of their causal relationship, and the role of other life history traits (e.g., wood anatomy or mechanical properties; Loehle, 1988), is still missing. The availability of densely sampled tree-ring networks of widely distributed genera (such as Fagus or Quercus) allows us to investigate how environmental variations affect longevity in trees with similar life-history traits.
The aims of our study are to: (1) make a review of the longevity of the main genera of broadleaf deciduous (BD) trees living in closed-canopy temperate forests of different parts of the Northern Hemisphere using available tree-ring data; (2) identify the mechanisms controlling the expressed range of variation in tree longevity; and (3) explore the role of climate in controlling the expression of the realized maximum lifespan at biome scale.
We created a dataset of maximum documented lifespan of BD trees from closed-canopy temperate forests in the Northern Hemisphere using tree-ring data and information from scientific literature or online databases. Original data consisted of tree-ring series already available in the laboratories of the coauthors of this manuscript (e.g., Piovesan et al., 2005; Di Filippo et al., 2007; Kitamura et al., 2007; Pederson, 2010; Martin-Benito and Pederson, 2015). Online data were retrieved by different sources, such as: OLDLIST (Brown, 1996; www.rmtrr.org/oldlist.htm); Eastern OLDLIST (www.ldeo.columbia.edu/~adk/oldlisteast); the International Tree-Ring Data Bank (ITRDB) (www.ncdc.noaa.gov/data-access/paleoclimatology-data/datasets/tree-ring).
The criteria used to include a tree into the dataset can be summarized as follows:
- Age data came exclusively from tree-ring series developed through dendrochronological standards (Stokes and Smiley, 1996). Where samples without pith had estimated ages for those missing years, we excluded the estimated data. As heart rot is frequent in very old deciduous trees (Ranius et al., 2009), this restriction excludes ages derived through extrapolation rather than direct observation.
- Priority was given to trees living in OG forests (certified by our own field research or by the selected scientific literature). OG forests contain old trees because they have not experienced recent stand-replacing disturbances. The identification of OG status is characterized by natural canopy tree mortality. Because wood decay often prevents the measurement of tree-rings on dead trees, maximum age from a sufficiently replicated dendroecological sampling can be used as a surrogate of maximum tree lifespan (i.e., longevity) within that forest. The occurrence of natural mortality connects maximum lifespan to local environmental conditions, and allows us to analyze longevity variations across different environments.
- When the OG status couldn't be certified, we used the oldest tree in sampling sites reported in online databases. Given that in warmer sites tree longevity is lower due to faster growth rates (Di Filippo et al., 2012) and turnover rates (Stephenson and van Mantgem, 2005), we used two different age thresholds for “old” trees to streamline the online database search: 400 years (i.e., above the 75th percentile in Wirth et al., 2009) in cool temperate forests and 200 years (i.e., above 25th percentile in Wirth et al., 2009) in warm temperate ones. Warm-cool temperate climate distinction was based on threshold values (mean annual temperature above 12°C or mean temperature of the warmest month above 21°C) reported in Holdridge's Life Zone system (Adams, 2010) and Box and Fujiwara (2015). Trees in the two climatic regimes with ages below these two thresholds (200 and 400 years) were not considered in our analyses.
- All data came from dendroecological studies, which require that samples are replicated enough to efficiently report a certain ecological information, e.g., the climatic signal or disturbance history (Stokes and Smiley, 1996). Tree-ring sampling generally requires large core replication (usually ≥20 cores per species) covering most of the study forest area. When multiple species were present in the same area, each species' maximum age was analyzed separately.
- When available, we retrieved the ring-width series of the oldest tree to characterize its growth history.
The website Dendrobox (www.dendrobox.org) was used as a tool to locate potential tree-ring studies involving BD trees in the Northern Hemisphere (Zang, 2015).
We assessed the role of bioclimate (i.e., annual precipitation; mean temperature of the year, and of the coldest and the warmest month) in controlling the expression of maximum lifespan found at each site. To explore the lifespan-climate relationship, climate data for each site were retrieved via different methods, depending on data availability. In our initial screening, climate data was collected from either (1) climatic time series that were readily available for the site (all US and most European study sites) or (2) the nearest available weather station, which limited us to yearly or monthly climate averages.
Of the 136 sites, 30 (roughly 22%) of our sites did not have high-resolution climatic data. These sites were mostly comprised of those retrieved from literature, online databases and some Japanese sites. In these cases, we corrected for the difference in elevation between the study site and the meteorological station by applying a lapse rate of −0.55°C/100 m. Rolland (2003) reported for the European Alps an observed mean temperature lapse rate between −0.54 and −0.58°C/100 m. In Italy, considering an area spanning 12° latitude, the mean annual temperature lapse rate varied between −0.54°C/100 m in Northern Italy and -0.57°C/100 m in Southern Italy (Brunetti et al., 2014).
For the US and European (Italy and Austria) sites, we used high-resolution (30-arcsecond) temperature and precipitation (1981–2010) monthly climate normals. For the US, we used climate normals provided by the PRISM Group (Daly et al., 2008), based on a climate–elevation regression estimated for each 30-arcsecond digital elevation model (DEM) grid cell, where stations entering the regression are assigned weights based on the physiographic similarity between weather stations and the grid cell. Corresponding to the exact forest location, we estimated the local vertical gradient (using 21 × 21 PRISM grid boxes, about 400 km2, centered on each site) of each meteorological variable and extrapolated its value to the site elevation. For Italy and Austria, normal climate data were obtained using a similar approach (e.g., Efthymiadis et al., 2006; Brunetti et al., 2012, 2014). In this case, thanks to the direct availability of source station data, the relationship between the meteorological variable and elevation was directly reconstructed for each site location using the nearby stations rather than surrounding grids (Brunetti et al., 2014). Both procedures provided reconstructed climate information with associated mean absolute error ranging between 0.5–1.0°C for mean monthly temperature and between 3 and 14 mm for total monthly precipitation, depending on the month considered (Daly et al., 2008; Brunetti et al., 2012, 2014).
The comparison among sites was based on annual temperature and precipitation, and mean temperature of the coldest/warmest months, as these variables are those generally reported in literature for describing site conditions.
The relationships between maximum tree lifespan and potential explanatory variables were first explored using a linear regression analysis for all species within our dataset. Next, we evaluated the most represented genera in our collection (Fagus, Quercus, Liriodendron) separately. When evaluating the maximum lifespan-climate relationships, we focused on Quercus and Fagus as both cover more continents. Since growth suppression can be an important factor promoting tree longevity (Black et al., 2008; Bigler and Veblen, 2009; Di Filippo et al., 2012), lifespan data were analyzed separately for shade-tolerant and shade-intolerant or intermediate species (see Table 1). In forests with many coexisting species, all species were considered separately when assessing the relationship between longevity and growth rate. However, only the oldest species was kept when assessing the temperature control over longevity. Given the established focus of the manuscript, our analyses excluded long-living trees of Mediterranean open dry woodland/shrubland (e.g., Quercus douglasii, Q. canariensis), which grow in severely moisture-stressed environmental conditions and may present a semi-deciduous habit.
Table 1. The oldest tree found for each deciduous species in temperate forest of the Northern Hemisphere.
Our final dataset contained 136 dendrochronologically analyzed trees covering North America, Europe, and Asia, representing 25 species and 12 genera. Trees in this dataset covered a wide range of climatic conditions, with mean annual temperature spanning 4–18°C and mean annual precipitation varying between ~700 and 3000 mm (Figure 1). About 2/3 of our study sites came from our own research with the remaining being derived from literature or online databases (Table S1).
Figure 1. Precipitation–temperature space occupied by the temperate deciduous forests analyzed. Symbols-colors refer to different genus-species. Convex hulls for Quercus, Fagus, and Liriodendron are drawn in red, blue and orange, respectively. Acronyms in Table 1.
A wide variability in maximum age emerged within this large range of climatic conditions, either for the same species in different locations or for different species growing within the same forest (Figure 2). The maximum lifespan of most trees varied generally between 300 and 400 years (interquartile range in Figure 2). However, the full range of observed values was far wider, i.e., between 100 and circa 600 years. Latitude/elevation variations affected longevity (r is 0.36 for latitude and 0.43 for elevation, both p = 0.05; Table 1). The lowest ages were represented by low-elevation beech trees growing in warm temperate forests on highly fertile volcanic soils (e.g., Fagus grandifolia in Mexico or F. sylvatica in central Italy). The longest lifespan was reported in the eastern US for Nyssa sylvatica (679 years), a highly shade-tolerant species growing in wetlands that tolerates long suppression periods and commonly occupies an intermediate canopy position (Sperduto et al., 2000; Abrams, 2007). A distinguishing feature of this species is its extremely low growth rates (see Figure 7; Pederson, 2010). N. sylvatica was slightly older than a 600 year old Swedish oak (Quercus robur) reported by Drobyshev and Niklasson (2010), a 559 year old beech tree of the central Apennines in Italy, both growing in northern or high-mountain cold climates, and a 509 year old yellow poplar (Liriodendron tulipifera) in an acidic, north-facing, cove at low elevation in the Smoky Mountains of the southeastern US (Pederson, 2010). The most replicated genera (Quercus, Fagus, Liriodendron) in our study showed a similar median age. On average, oaks have a slightly higher maximum lifespan. But, the lower bound for the beech 25th percentile showed lower age expectations than for oak.
Figure 2. Boxplots of the maximum age found for all temperate deciduous trees in our dataset and separately for the main genera. Within parentheses, the number of trees in each boxplot. The central bar is the median, the margins of each box are the first and third quartile, the whiskers are at ± 1.57 interquartile range and external points are outliers.
Among different sites, the maximum ages of the oldest trees belonging to each species were strongly and inversely related to their growth rate, as expressed by mean ring-width (Figure 3). The relationship also held for the most replicated genera, analyzed separately. Fagus sylvatica in southern Europe and Liriodendron tulipifera in the eastern US both exhibited highly significant negative growth-lifespan relationships (slopes respectively equal to −0.81 and −0.73, in both cases P < 0.01; see legend in Figure 3). Nyssa was not fully explored across its range in this study. Fagus and Liriodendron exhibited more negative slopes than Quercus (regression slope = −0.28; Figure 3), which was not able to reach the highest lifespan at slow growth rates, as predicted by the 600 years old Swedish oak (growth series not available; Drobyshev and Niklasson, 2010). Interestingly, oaks (especially Quercus alba) had older lifespans than the other species while sustaining growth rates over centuries.
Figure 3. Relationships between the age of the oldest deciduous tree within each temperate old-growth forest and its mean ring-width. Values are represented using log-transformed scales, regression lines were developed for all sites together and separately for the main genera (within parentheses: R2adj, adjusted R2; F, value of the F statistic; P, significance of the F-test). Species code in Table 1 (QUROsl = Quercus robur sensu latu, i.e., the exact species not reported in the literature paper). The oldest NYSY couldn't be plotted because the tree-ring series was not available.
The strongest lifespan relationship with climate was with the temperature of the warmest month (in terms of regression significance and variance explained; Figure 4, Figures S1, S2; Di Filippo et al., 2012). The shade-tolerant Fagus and Acer species showed a consistent, highly significant relationship characterized by a steep decrease of maximum lifespan with increasing temperature. We found a relationship for these two species where trees can be 30 years younger in longevity for each Celsius degree increase during the warmest month of the year (see legend in Figure 4). Nyssa sylvatica and Aesculus flava did not yield significant model fits, which might be due to the limited amount of data across their distributions. For oaks, and in general for shade-intolerant species, the regression was weaker and essentially maintained by the oldest Swedish oak tree of 600 years. Correlation between growth-rates and climate confirmed these results. In fact, among all species used in the regressions of Figure 3, Fagus sylvatica was the only one with available ring-width data that showed a significant correlation with temperature (r = 0.75, P ≤ 0.001).
Figure 4. Relationships between the age of the oldest deciduous tree within each temperate old-growth forest and its mean ring-width. Values are represented using log-transformed scales, regression lines were developed for all sites together and separately for the main genera (within parentheses: R2adj, adjusted R2; F, value of the F statistic; P, significance of the F-test). Species code in Table 1 (QUROsl = Quercus robur sensu latu, i.e., the exact species not reported in the literature paper).
Within the OG Fagus sylvatica forests growing from the Alps to the Southern Apennines studied to date, the oldest trees were significantly smaller in diameter and slow growing in comparison to the larger ones in the same stand (Figure 5). Slower growth rates in the oldest beech trees are generally associated with long and repeated suppression phases (Figure 6). It is remarkable that the oldest beech trees (Figure 6) are found in primary forests of the Central Apennines (see Ziaco et al., 2012), where the longest suppression events are experienced (Di Filippo et al., 2012). In Europe and in the US (Figure 7), the oldest trees found in temperate deciduous forests showed a mean ring-width close to 0.5 mm/year, a threshold generally reported to track suppression phases in ring-width series in temperate trees (Canham et al., 1990). Interesting exceptions were provided by Fagus sylvatica in the Northern Apennines (Figure 6) and Magnolia acuminata and Quercus rubra in the US (Figure 7), where trees experienced long periods of sustained growth, especially in the early stages of life. The oldest Liriodendron tulipifera was truly a surprising and exceptional finding, since this 509 years old tree has faster growth rates in comparison to other species of similar maximum age (Fagus sylvatica and Nyssa sylvatica; Figure 3), and is the tallest L. tulipifera so far measured in the eastern US (N. Pederson, personal communication). This underlines that many ways can exist to attain the maximum lifespan in different tree species and environment.
Figure 5. Comparison between the age, DBH and overall Basal Area Increment (BAI) of the 5 oldest (O) and largest (L) beech trees within high-elevation forests. The difference in mean (thick black lines) was tested by a Welch's t test (***: P ≤ 0.001; **: P ≤ 0.01; *: P ≤ 0.05; “.”: P ≤ 0.1). Stands (represented in different colors) are grouped according to location (A-Alp and I-Alp: Austrian and Italian Alps; N-Ape, C-Ape, S-Ape: northern, central, southern Apennines) and naturalness degree (primary and secondary old-growth forest: OG1, OG2).
Figure 6. Ring-widths series of the oldest Fagus sylvatica trees living in (top to bottom). The eastern Alps, northern, central, and southern Apennines (trees sampled, respectively, in the following National Parks: Kalkalpen NP, Foreste Casentinesi NP, Abruzzi NP, and Pollino NP). The dotted line represents the average ring width of the tree.
Figure 7. Ring-widths series of the oldest species (≥300 years old) found in the eastern US. The dotted line represents the average ring width of the tree. [NYSY, Nyssa sylvatica; LITU, Liriodendron tulipifera; CAOV, Carya ovata; MAAC, Magnolia acuminata; QUAL, Quercus alba; QUMO, Quercus montana; QURU, Quercus rubra; QUMU, Quercus muehelebergii).
Several BD tree species growing in different sites of the temperate biome of the Northern Hemisphere showed longevities of 500–600+ years (Table 1). Amazingly, species from evolutionary distant families and genera—Fagus, Quercus, Nothofagus (Pollmann, 2005), Acer, Magnolia, Nyssa, Liriodendron—converge to similar age limits. These species do not show outstanding commonalities in life history traits (Table 1), suggesting that functional traits (e.g., wood density) may not always be the dominant determinants of longevity (see Loehle, 1988). Our findings suggest that much remains to understand how biological (e.g., wood properties, anatomy) and ecological (e.g., r-K selection) properties can definitively influence tree longevity. Since intraspecific plant trait variation could be driven by the environment (Richardson et al., 2013), future exploration of their influence on the realized tree longevity must also consider their simultaneous variability along wide environmental gradients.
The maximum lifespan realized by the BD trees in our overall dataset showed a remarkable variability (100–650 years; see also Wirth et al., 2009). In this study, the lower quartile of age distribution was 300 years, which is higher than the previously reported quartile age of 200 years (see Wirth et al., 2009). This could be related to the exclusive use of rigorously dated tree ages through dendrochronological techniques, or to the fact that we used maximum age rather than other forest age indicators (e.g., mean canopy age, mean age of the oldest 10 trees). According to our results, 300–400 years can be baseline values for most temperate deciduous OG forests. These baseline values hold even when analyzing the most represented genera separately, and verify reported lifespans in tropical forests, where large trees over 300 years old may be relatively abundant (Metcalf et al., 2009).
Different ecological site conditions can affect tree longevity through controls over tree growth rates (Di Filippo et al., 2012). Within and between different species, maximum lifespan decreased with increasing growth rates along continental-scale ecological gradients, from cool temperate to warm temperate. In Fagus sylvatica, the maximum lifespan had a threefold increase from hilly to high-mountain sites (200–600 years along an elevation range of ~1500 m), following a three to 4-fold growth rate decrease (Di Filippo et al., 2012). This relationship was evident in previous studies on different genera such as oak, chestnut, spruce, fir, pine, and beech (Black et al., 2008; Bigler and Veblen, 2009; Briffa and Melvin, 2011; Reynolds and Burke, 2011; Di Filippo et al., 2012; Rotheli et al., 2012; Seim et al., 2012; Cooper et al., 2013). Bioclimate (Di Filippo et al., 2012), soil fertility (e.g., Robichaud and Methven, 1993), and early suppression (Black et al., 2008; Bigler and Veblen, 2009; Di Filippo et al., 2012) have been proposed as prominent ecological factors in conferring longevity to forest trees. The dependence of longevity on growth rates can thus be observed from the community-level to landscape- (e.g., elevation transects) and biome scales.
At the hemispheric level, the meta-analysis conducted for the temperate deciduous forest biome highlighted convergent geographic patterns in maximum lifespan and temperature variability. Latitude/elevation variations can affect longevity (r is 0.36 for latitude and 0.43 for elevation, both p ≤ 0.05; Table 1) by controlling tree growth rates (Rotheli et al., 2012). The dependence of maximum lifespan on temperature is prominent in Fagus forests, which cover large geographic transects, but generally selecting well-drained mesic sites (Peters, 1997). Temperature positively affects Fagus growth, primarily through the growing season length (Alessandrini et al., 2010). The relationship of deciduous Quercus spp. with temperature is instead weaker and more complex to interpret. This interpretation might be related to oaks' capacity to adapt to extremely dry, wet, or unfertile site conditions (Jones, 1959). Deciduous oaks have evolved to occupy the drier and wetter ecological spaces left by other tree species with higher competitive capabilities in mesic conditions, i.e., Fagus or Acer spp. (Ellenberg, 1988; Johnson et al., 2009). Since water balance issues have a great impact on their growth (Martin-Benito and Pederson, 2015), they may assume a central role in controlling their expression of longevity. More broadly, some oak species are well adapted to dry environments, like Quercus douglasii, which can live 500–600 years in warm temperate open woodlands under the Mediterranean climate of California (Stahle et al., 2013), while old oak trees can even be found at the opposite side of the wetness gradient, as the 600 year old Quercus robur, growing in the wet forests of northern Europe (Drobyshev and Niklasson, 2010).
The oldest trees maintain remarkably low growth rates for their entire lifespan (Black et al., 2008; Johnson and Abrams, 2009), especially when young (Bigler and Veblen, 2009). This well-known phenomenon to dendrologists (Schulman, 1954; Loehle, 1988; literature cited in Bugmann and Bigler, 2011) seems to support the metabolic theory of ecology (e.g., Issartel and Coiffard, 2011). For a given species, the oldest trees can thus be found in sites characterized by a colder climate, lower soil fertility, and in advanced OG forests with several, long phases of suppression (Di Filippo et al., 2012). Protracted suppression can contribute to denser and more durable wood in some species (Bigler and Veblen, 2009). Fast-growing trees can instead be subject to trade-offs, such as reduced investment in defenses and a lower mechanical wood strength, which can reduce their life expectancy (Bugmann and Bigler, 2011). Slow growth rates confer smaller dimensions to trees, a factor that can delay the onset of hydraulic limitations imposed by size (Mencuccini et al., 2005). Smaller trees can even cope more efficiently with mechanical damages by sustaining lower repairing costs (Peñuelas and Munné-Bosch, 2010). Multiple suppression and release events are frequent in natural closed-canopy temperate forests (Canham et al., 1990), and the oldest trees found in Europe and the eastern US have consistent suppression phases followed by release even in old age (Figures 6, 7; Black et al., 2008). This demonstrates that these old trees must be characterized by an incredible plasticity to survive under harsh environmental conditions.
Slow growth can also confer reduced size (i.e., DBH), a factor with important ecological implications for survival under different disturbance regimes. In structurally complex forests, the oldest trees are usually not the largest, and maintain significantly slower growth rates. This phenomenon has also been observed in species living in different forest types, such as Sequoiadendron giganteum in California (N. Stephenson, personal communication) or Pinus heldreichii in Italy (Monte Pollino, data not shown). In natural temperate deciduous forests, rapidly growing trees are more likely to reach earlier the “high-risk” (i.e., large) DBH (Melvin, 2004), associated with the U-shaped mortality curve (e.g., Lines et al., 2010). In contrast, protracted growth suppression postpones canopy accession and, potentially, exposure to disturbance. Our results only partially follow the reported effect of temperature on mortality rates within temperate deciduous forests of the eastern US (Lines et al., 2010). This finding might result from the fact that specific mortality rates may not always correlate with the maximum lifespans observed in this study. Correspondence is good for beech, but weaker for Quercus, Liriodendron, Betula, Carya, and greatly underestimated for Nyssa sylvatica. Understanding longevity in trees will require integration of the biological and ecological aspects governing slow growth.
The oldest temperate deciduous trees were mainly found in mountainous and remote areas of USA and Europe. Trees over 500 years were often found where geographical features and historical dynamics have discouraged intensive human activities. Despite millennia of intensive land-use, relict primary OG forests can still be found in Europe and North America (e.g., Carpathian Mountains; Abruzzi National Park in the Apennines; Dinaric Mountains; Appalachian Mountains, Adirondacks Mountains; various wetlands in the eastern US; e.g., Sperduto et al., 2000; Piovesan et al., 2005; Stahle et al., 2006; Trotsiuk et al., 2012). “Remote” can either mean distant from large urban settlements (e.g., Nothofagus spp. in the Andes and Patagonia; Gutiérrez et al., 1991; Pollmann, 2005), or limited to economically marginal sites, like those documented in ecotonal oak forests of the eastern US (Stahle, 1996) and the high-mountain Apennines beech forests (Abruzzi National Park; Piovesan et al., 2005). It is remarkable that European OG beech forests (e.g., Carpathian Mts., eastern Alps, Pyrenees, Dinaric, and Apennines Mts.) contain a large stock of trees 400–600 years old (Piovesan et al., 2011; Trotsiuk et al., 2012). In the last decade, the 500–600 year old trees, often living in small, but widespread patches of primary forests in the Abruzzi National Park, have been an unexpected discovery (Piovesan et al., 2005, 2011). This area is distinguished by hosting some among the oldest montane mesophilous deciduous forests in the Northern Hemisphere. Interestingly, trees living in these primary forests are (on average) 100 years older than those living in secondary OG forests.
Deciduous oaks approaching 500 years are currently very rare both in the eastern US and in the European landscape. Given the pristine nature of several oak OG forests in the eastern US (Stahle, 1996), this general lack of very old trees could be ascribed to limits imposed by recurring severe natural disturbance like fires and severe drought spells (e.g., Cook et al., 2007). In central Europe, oak forests are instead mostly remnants of pasture woodlands from the Middle Age, shaped by grazing and impacted by pigs, or hunting reserves (like Fontainbleau Forest). The lack of very old deciduous oaks can be related to historical landscape modifications. However, among hundreds of sub-fossil oak trees extracted from bogs in central Europe, very few samples reached 500–600 years (Figure 8; Leuschner et al., 2002; Spurk et al., 2002; Friedrich et al., 2004). This would lead us to conclude that, at least for a large part of lowlands in west and central Europe, oaks were not able to surpass the limit of 500–600 years even in the Neolithic period. An emblematic example is the Fointainebleau Forest, protected since the late Middle Age, where windstorms have been a major force driving stand dynamics so as beech and oak trees are no older than 400 years (Pontailler et al., 1997). Interestingly, central-western Europe is impacted by a higher risk of windstorms/flood (see Map 11 in Schmidt-Thomé, 2005). Winter storms, flooding and fires can act as major large-scale environmental stressors for longevity.
Figure 8. Ring-width series of the “bog oak Methusalem,” one of the oldest central European sub-fossil bog oaks (3336–2877 BC). The sample has 460 tree rings, but sapwood was missing: estimated age is circa 500 years (courtesy of Dr. Hanns Hubert Leuschner). The dotted line represents the average ring width of the tree.
The 600 year age limit may also be explained by human history and climate change. The Black Death in the mid-14th century contributed to a substantial reduction in the human impact on natural ecosystems (~50% human population died in Western Europe), so that beech and oak forests re-colonized, especially in mountainous areas (Mensing et al., 2013). Similarly, there appeared to be a remarkable pulse of regeneration in the late-1600s across the broadleaf dominated forest of the eastern US (Pederson et al., 2014). A spike in recruitment in a light-limited forest suggests large-scale canopy gaps (Lorimer and Frelich, 1989). Factors that open canopies include windstorms, ice storms, severe fire, and climate variations, specifically drought. Most of these factors are not known to operate at subcontinental scales (Vanderwel et al., 2013). Paleoclimate records suggest dry conditions around this time (Pederson et al., 2014) and that regional to pan-continental droughts have occurred over the last 1000 years in the continental US (Cook et al., 2007). Thus, some of the maximum longevities narrowly exceeding 300 years in this region could also be related to severe disturbance pulses from repeated or intense drought.
In some cases, cultural practices could contribute to the conservation of old trees in different cultural landscapes. Moreover, the discovery of 300 year old trees in hilly beech forests (Foreste Casentinesi National Park and Pollino National Park) have been unexpected. How these old trees have survived at low elevation in one of the cradles of Western cultures is difficult to fully understand. Despite these limitations, there are some clues in the cultural history of the region. Most of the remnant forests survived close to towns as hunting reserves or parkland forests for cattle and pig grazing. Romans had a strong tradition for hunting edible dormouse (Glis glis), considered a delicacy, and this practice has persisted up to recent times in local communities like the Pollino National Park (Aldo Schettino, personal communication). Such a tradition may have contributed to preserve those habitats, containing older trees with hollow trunks (wildlife trees).
The relationship between size (i.e., stem diameter), age, and life expectancy in different forest environments is still poorly understood. Slow growth is the only life-history functional trait clearly linked to longevity, and correlating it with environmental features. The dendrochronologically verified age limit of 600–700 years can be considered a maximum lifespan reference for the deciduous temperate biome. Considering the increased growth rates maintained by trees at great ages, we believe that this is not a biological limit (i.e., meristem senescence; Mencuccini et al., 2014). A central theme for future studies will be to understand how the geography of rare, severe disturbance agents impacts tree survivorship.
The oldest trees were living in subordinate canopy conditions for extended periods and/or within primary forests in European cool temperate mesic environments, generally in harsh areas outside storm tracks characterized by extremely infrequent catastrophic stand replacing disturbance events like hurricanes and fires (Piovesan et al., 2011; Trotsiuk et al., 2012; Nagel et al., 2014). It is difficult to find a single mechanism linking forest net primary productivity (NPP), as inferred from radial growth rates, and mortality rates to climate, edaphic and structural state conditions (Stephenson et al., 2011). Site features that constrain tree growth rates and longevity demonstrated to control the velocity of OG recovery in mesic (Fagus) forests; in this ecosystem the structural cycle is faster in warm, fertile environments, rather than in cool ones (Ziaco et al., 2012).
The ecological importance of the Apennines OG beech sites is testified by their candidature—under the criterion IX—to UNESCO World Heritage List (Knapp and Fichtner, 2011), as already acknowledged for the serial sites of Primeval Beech Forests of the Carpathians and the Ancient Beech Forests of Germany (http://whc.unesco.org/en/list/1133) and Shirakami-Sanchi Fagus crenata forests in Japan (http://whc.unesco.org/en/list/663). The protection of the ecological integrity under UNESCO will guarantee the conservation of some of the oldest BD trees on Earth.
The authors declare that the research was conducted in the absence of any commercial or financial relationships that could be construed as a potential conflict of interest.
The collection of tree-ring series used in this work was possible thanks to the collaboration of numerous foresters, rangers, and students during sampling surveys. Different M.Sc. and Ph.D. students have participated in developing the tree-ring series in our labs. We are grateful to Isabel Dorado, Hanns Hubert Leushaner and Nathan Stephenson for providing tree-ring series from Pietra Porciana beech forest, the old bog oak of Germany and Sequoiadendron giganteum, respectively. We thank Paul J. Krusic for age data on Nyssa sylvatica. Ute Sass-Klaassen and Katarina Čufar provided useful information regarding the European tree-ring data. Thanks to Dan Bishop for constructive comments that improved this manuscript. We wish to thank two reviewers whose comments helped to improve the first version of this manuscript. The following Italian and Austrian National Parks partially supported this research: Abruzzo, Lazio e Molise NP; Pollino NP; Foreste Casentinesi NP; Kalkalpen NP.
The Supplementary Material for this article can be found online at: http://journal.frontiersin.org/article/10.3389/fevo.2015.00046/abstract
Abrams, M. D. (2007). Tales from the blackgum, a consummate subordinate tree. Bioscience 57, 347–359. doi: 10.1641/B570409
Abrams, M. D., Copenheaver, C. A., Terazawa, K., Umeki, K., Takiya, M., and Akashi, N. (1999). A 370-year dendroecological history of an old-growth Abies–Acer–Quercus forest in Hokkaido, northern Japan. Can. J. For. Res. 29, 1891–1899. doi: 10.1139/x99-174
Adams, J. (2010). Vegetation-Climate Interaction. How Plants Make the Global Environment. Chichester, UK: Springer Praxis Book.
Alessandrini, A., Vessella, F., Di Filippo, A., Salis, A., Santi, L., Schirone, B., et al. (2010). Combined dendroecological and normalized difference vegetation index analysis to detect regions of provenance in forest species. Scand. J. For. Res. 25, 121–125. doi: 10.1080/02827581.2010.485776
Bigler, C., and Veblen, T. T. (2009). Increased early growth rates decrease longevities of conifers in subalpine forests. Oikos 118, 1130–1138. doi: 10.1111/j.1600-0706.2009.17592.x
Biondi, F. (1992). Development of a tree-ring network for the Italian Peninsula. Tree Ring Bull. 52, 15–29.
Black, B. A., Colbert, J. J., and Pederson, N. (2008). Relationships between radial growth rates and lifespan within North American tree species. Ecoscience 15, 349–357. doi: 10.2980/15-3-3149
Box, E. O., and Fujiwara, K. (2015). Warm-Temperate Deciduous Forests around the Northern Hemisphere. Cham: Springer International Publishing.
Briffa, K. R., and Melvin, T. M. (2011). “A closer look at regional curve standardization of tree-ring records: justification of the need, a warning of some pitfalls, and suggested improvements in its application,” in Dendroclimatology: Progress and Prospects, eds M. K. Hughes, T. W. Swetnam, and H. F. Diaz (Dordrecht: Springer), 113–145.
Brown, P. M. (1996). “OLDLIST: a database of maximum tree ages,” in Tree Rings, Environment, and Humanity. Radiocarbon 1996, eds J. S. Dean, D. M. Meko, and T. W. Swetnam (Tucson, AZ), 727–731. Available online at: http://www.rmtrr.org/index.html
Brunetti, M., Lentini, G., Maugeri, M., Nanni, T., Simolo, C., and Spinoni, J. (2012). Projecting North Eastern Italy temperature and precipitation secular records onto a high resolution grid. Phys. Chem. Earth 40–41, 9–22. doi: 10.1016/j.pce.2009.12.005
Brunetti, M., Maugeri, M., Nanni, T., Simolo, C., and Spinoni, J. (2014). High−resolution temperature climatology for Italy: interpolation method intercomparison. Int. J. Climatol. 34, 1278–1296. doi: 10.1002/joc.3764
Bugmann, H., and Bigler, C. (2011). Will the CO2 fertilization effect in forests be offset by reduced tree longevity? Oecologia 165, 533–544. doi: 10.1007/s00442-010-1837-4
Canham, C. D., Denslow, J. S., Platt, W. J., Runkle, J. R., Spies, T. A., and White, P. S. (1990). Light regimes beneath closed canopies and tree-fall gaps in temperate and tropical forests. Can. J. For. Res. 20, 620–631. doi: 10.1139/x90-084
Chokkalingam, U., and White, A. (2000). Structure and spatial patterns of trees in old-growth northern hardwood and mixed forests of northern Maine. Plant Ecol. 156, 139–160. doi: 10.1023/A:1012639109366
Cook, E. R., Seager, R., Cane, M. A., and Stahle, D. W. (2007). North American drought: reconstructions, causes, and consequences. Earth Sci. Rev. 81, 93–134. doi: 10.1016/j.earscirev.2006.12.002
Cooper, A. M. (2011). Age, Forest Structure, and Disturbance History of Five Potential Old-growth Forests in Eastern Kentucky's Cumberland Plateau. Online Theses and Dissertations. Paper 31.
Cooper, R. J., Melvin, T. M., Tyers, I., Wilson, R. J., and Briffa, K. R. (2013). A tree-ring reconstruction of East Anglian (UK) hydroclimate variability over the last millennium. Clim. Dyn. 40, 1019–1039. doi: 10.1007/s00382-012-1328-x
Daly, C., Halbleib, M., Smith, J. I., Gibson, W. P., Doggett, M. K., Taylor, G. H., et al. (2008). Physiographically sensitive mapping of climatological temperature and precipitation across the conterminous United States. Int. J. Climatol. 28, 2031–2064. doi: 10.1002/joc.1688
Di Filippo, A., Biondi, F., Čufar, K., De Luis, M., Grabner, M., Maugeri, M., et al. (2007). Bioclimatology of beech (Fagus sylvatica L.) in the Eastern Alps: spatial and altitudinal climatic signals identified through a tree−ring network. J. Biogeogr. 34, 1873–1892. doi: 10.1111/j.1365-2699.2007.01747.x
Di Filippo, A., Biondi, F., Maugeri, M., Schirone, B., and Piovesan, G. (2012). Bioclimate and growth history affect beech lifespan in the Italian Alps and Apennines. Glob. Chang. Biol. 18, 960–972. doi: 10.1111/j.1365-2486.2011.02617.x
Drobyshev, I., and Niklasson, M. (2010). How old are the largest southern Swedish oaks? A dendrochronological analysis. Ecol. Bull. 53, 155–163.
Easdale, T. A., and Healey, J. R. (2009). Resource-use-related traits correlate with population turnover rates, but not stem diameter growth rates, in 29 subtropical montane tree species. Perspect. Plant Ecol. Evol. Syst. 11, 203–218. doi: 10.1016/j.ppees.2009.03.001
Efthymiadis, D., Jones, P. D., Briffa, K. R., Auer, I., Böhm, R., Schöner, W., et al. (2006). Construction of a 10−min−gridded precipitation data set for the Greater Alpine Region for 1800–2003. J. Geophys. Res. 111. doi: 10.1029/2005JD006120
Ellenberg, H. (1988). Vegetation Ecology of Central Europe. New York, NY: Cambridge University Press.
Fonti, P., Treydte, K., Osenstetter, S., Frank, D., and Esper, J. (2009). Frequency-dependent signals in multi-centennial oak vessel data. Palaeogeogr. Palaeoclimatol. Palaeoecol. 275, 92–99. doi: 10.1016/j.palaeo.2009.02.021
Frelich, L. E., and Reich, P. B. (2003). Perspectives on development of definitions and values related to old-growth forests. Environ. Rev. 11, S9–S22. doi: 10.1139/a03-011
Friedrich, M., Remmele, S., Kromer, B., Hofmann, J., Spurk, M., Kaiser, K. F., et al. (2004). The 12,460-year hohenheim oak and pine tree-ring chronology from central Europe—a unique annual record for radiocarbon calibration and paleoenvironment reconstructions. Radiocarbon 46, 1111–1122.
Gutiérrez, E., Vallejo, V. R., Romana, J., and Fons, J. (1991). The subantarctic Nothofagus forests of Tierra del Fuego: distribution, structure and production. Oecol. Aquat. 10, 351–366.
Issartel, J., and Coiffard, C. (2011). Extreme longevity in trees: live slow, die old? Oecologia 165, 1–5. doi: 10.1007/s00442-010-1807-x
Johnson, P. S., Shifley, S. R., and Rogers, R. (2009). The Ecology and Silviculture of Oaks. Wallingford, CT: CABI Publishing.
Johnson, S. E., and Abrams, M. D. (2009). Age class, longevity and growth rate relationships: protracted growth increases in old trees in the eastern United States. Tree Physiol. 29, 1317–1328. doi: 10.1093/treephys/tpp068
Jones, E. W. (1959). Biological flora of the british isles: Quercus L. J. Ecol. 47, 169–222. doi: 10.2307/2257253
Kitamura, K., Kobayashi, M., and Kawahara, T. (2007). Age structure of wind-felled canopy trees for Siebold's beech (Fagus crenata) in the northernmost population in Karibayama, Hokkaido. J. For. Res. 12, 467–472. doi: 10.1007/s10310-007-0026-8
Knapp, H. D., and Fichtner, A. (2011). Beech Forests: Joint Natural Heritage of Europa, Skripten 297. Bonn: Bundesamt für Naturschutz.
Kose, N., and Guner, H. T. (2012). The effect of temperature and precipitation on the intra-annual radial growth of Fagus orientalis Lipsky in Artvin, Turkey. Turk. J. Agric. For. 36, 501–509. doi: 10.3906/tar-1109-4
Labuhn, I., Daux, V., Pierre, M., Stievenard, M., Girardclos, O., Féron, A., et al. (2014). Tree age, site and climate controls on tree ring cellulose δ18O: A case study on oak trees from south-western France. Dendrochronologia 32, 78–89. doi: 10.1016/j.dendro.2013.11.001
Leuschner, H. H., Sass-Klaassen, U., Jansma, E., Baillie, M. G., and Spurk, M. (2002). Subfossil European bog oaks: population dynamics and long-term growth depressions as indicators of changes in the Holocene hydro-regime and climate. Holocene 12, 695–706. doi: 10.1191/0959683602hl584rp
Lines, E. R., Coomes, D. A., and Purves, D. W. (2010). Influences of forest structure, climate and species composition on tree mortality across the eastern US. PLoS ONE 5:e13212. doi: 10.1371/journal.pone.0013212
Loehle, C. (1988). Tree life history strategies: the role of defenses. Can. J. For. Res. 18, 209–222. doi: 10.1139/x88-032
Lorimer, C. G., and Frelich, L. E. (1989). A methodology for estimating canopy disturbance frequency and intensity in dense temperate forests. Can. J. For. Res. 19, 651–663. doi: 10.1139/x89-102
Martin-Benito, D., and Pederson, N. (2015). Convergence in drought stress, but a divergence of climatic drivers across a latitudinal gradient in a temperate broadleaf forest. J. Biogeogr. 42, 925–937. doi: 10.1111/jbi.12462
Melvin, T. M. (2004). Historical Growth Rates and Changing Climatic Sensitivity of Boreal Conifers. Thesis, University of East Anglia, Norwich. Available online at: http://www.cru.uea.ac.uk/cru/pubs/thesis/2004-melvin/
Mencuccini, M., Martínez-Vilalta, J., Vanderklein, D., Hamid, H. A., Korakaki, E., Lee, S., et al. (2005). Size-mediated ageing reduces vigour in trees. Ecol. Lett. 8, 1183–1190. doi: 10.1111/j.1461-0248.2005.00819.x
Mencuccini, M., Oñate, M., Rico, L., Peñuelas, J., and Munné-Bosch, S. (2014). No signs of meristem senescence in old Scots pine. J. Ecol. 102, 555–565. doi: 10.1111/1365-2745.12219
Mensing, S., Tunno, I., Cifani, G., Florindo, F., Noble, P., Sagnotti, L., et al. (2013). Effects of human impacts and climate variation on forests: the Rieti basin since medieval time. Annali di Botanica, 3, 121–126. doi: 10.4462/annbotrm-10243
Metcalf, C. J., Horvitz, C. C., Tuljapurkar, S., and Clark, D. A. (2009). A time to grow and a time to die: a new way to analyze the dynamics of size, light, age, and death of tropical trees. Ecology 90, 2766–2778. doi: 10.1890/08-1645.1
Michaletz, S. T., Cheng, D., Kerkhoff, A. J., and Enquist, B. J. (2014). Convergence of terrestrial plant production across global climate gradients. Nature 512, 39–43. doi: 10.1038/nature13470
Moir, A. (2012). Dendrochronological Analysis of Oak Trees at Lullingstone County Park. Eynsford: Kent.
Nagano Regional Forest Office. (1989). Forest Management by the Natural Regeneration of Beech. Matsumoto: Fujiwara Insatsu KK.
Nagel, T. A., Svoboda, M., and Kobal, M. (2014). Disturbance, life history traits, and dynamics in an old-growth forest landscape of southeastern Europe. Ecol. Appl. 24, 663–679. doi: 10.1890/13-0632.1
Niinemets, U., and Valladares, F. (2006). Tolerance to shade, drought, and waterlogging of temperate northern hemisphere trees and shrubs. Ecol. Monogr. 76, 521–547. doi: 10.1890/0012-9615(2006)076[0521:TTSDAW]2.0.CO;2
Orwig, D. A., Cogbill, C. V., Foster, D. R., and O'Keefe, J. F. (2001). Variations in old-growth structure and definitions: forest dynamics on Wachusett Mountain, Massachusetts. Ecol. Appl. 11, 437–452. doi: 10.1890/1051-0761(2001)011[0437:VIOGSA]2.0.CO;2
Pederson, N. (2010). External characteristics of old trees in the Eastern Deciduous Forest. Nat. Areas J. 30, 396–407. doi: 10.3375/043.030.0405
Pederson, N., Bell, A. R., Cook, E. R., Lall, U., Devineni, N., Seager, R., et al. (2013). Is an epic pluvial masking the water insecurity of the greater New York City region? J. Clim. 26, 1339–1354. doi: 10.1175/JCLI-D-11-00723.1
Pederson, N., D'Amato, A. W., and Orwig, D. A. (2007). “Central hardwood natural history from dendrochronology: maximum ages of rarely studied species,” in Proceedings of the 15th Central Hardwood Conference, Vol. 27 (Knoxville, TN: University of Tennessee).
Pederson, N., Dyer, J. M., McEwan, R. W., Hessl, A. E., Mock, C. J., Orwig, D. A., et al. (2014). The legacy of episodic climatic events in shaping temperate, broadleaf forests. Ecol. Monogr. 84, 599–620. doi: 10.1890/13-1025.1
Pederson, N., Tackett, K., McEwan, R. W., Clark, S., Cooper, A., Brosi, G., et al. (2012). Long-term drought sensitivity of trees in second-growth forests in a humid region. Can. J. For. Res. 42, 1837–1850. doi: 10.1139/x2012-130
Peñuelas, J., and Munné-Bosch, S. (2010). Potentially immortal? New Phytol. 187, 564–567. doi: 10.1111/j.1469-8137.2010.03360.x
Piovesan, G., Alessandrini, A., Biondi, F., Di Filippo, A., Schirone, B., and Ziaco, E. (2011). “Bioclimatology, growth processes, longevity and structural attributes in an Italian network of old-growth beech forests spreading from the Alps to the Apennines,” in Beech Forests-Joint Natural Heritage of Europe, eds H. D. Knapp and A. Fichtner (Bonn: BfN-Skripten), 173–192.
Piovesan, G., Di Filippo, A., Alessandrini, A., Biondi, F., and Schirone, B. (2005). Structure, dynamics and dendroecology of an old-growth Fagus forest in the Apennines. J. Veg. Sci. 16, 13–28. doi: 10.1658/1100-9233(2005)016[0013:SDADOA]2.0.CO;2
Plietzsch, A. (2009). Die Lebensdauer von Bäumen und Möglichkeiten zur Altersbestimmung. Jahrbuch der Baumpflege 172–188.
Pollmann, W. (2005). A long-term record of Nothofagus dominance in the southern Andes, Chile. Austral Ecol. 30, 91–102. doi: 10.1046/j.1442-9993.2004.01427.x
Pontailler, J. Y., Faille, A., and Lemée, G. (1997). Storms drive successional dynamics in natural forests: a case study in Fontainebleau forest (France). For. Ecol. Manage. 98, 1–15. doi: 10.1016/S0378-1127(97)00073-X
Poulson, T. L., and Platt, W. J. (1996). Replacement patterns of beech and sugar maple in Warren Woods, Michigan. Ecology 77, 1234–1253. doi: 10.2307/2265592
Ranius, T., Niklasson, M., and Berg, N. (2009). Development of tree hollows in pedunculate oak (Quercus robur). For. Ecol. Manage. 257, 303–310. doi: 10.1016/j.foreco.2008.09.007
Reynolds, D. L., and Burke, K. L. (2011). The effect of growth rate, age, and chestnut blight on American chestnut mortality. Castanea 76, 129–139. doi: 10.2179/10-035.1
Richardson, S. J., Allen, R. B., Buxton, R. P., Easdale, T. A., Hurst, J. M., Morse, C. W., et al. (2013). Intraspecific relationships among wood density, leaf structural traits and environment in four co-occurring species of Nothofagus in New Zealand. PLoS ONE 8:e58878. doi: 10.1371/journal.pone.0058878
Robichaud, E., and Methven, I. R. (1993). The effect of site quality on the timing of stand breakup, tree longevity, and the maximum attainable height of black spruce. Can. J. For. Res. 23, 1514–1519. doi: 10.1139/x93-191
Rohner, B., Bugmann, H., and Bigler, C. (2013). Towards non-destructive estimation of tree age. For. Ecol. Manage. 304, 286–295. doi: 10.1016/j.foreco.2013.04.034
Rolland, C. (2003). Spatial and seasonal variations of air temperature lapse rates in Alpine regions. J. Clim. 16, 1032–1046. doi: 10.1175/1520-0442(2003)016<1032:SASVOA>2.0.CO;2
Rotheli, E., Heiri, C., and Bigler, C. (2012). Effects of growth rates, tree morphology and site conditions on longevity of Norway spruce in the northern Swiss Alps. Eur. J. For. Res. 131, 1117–1125. doi: 10.1007/s10342-011-0583-4
Rozas, V. (2005). Dendrochronology of pedunculate oak (Quercus robur L.) in an old-growth pollarded woodland in northern Spain: establishment patterns and the management history. Ann. For. Sci. 62, 13–22. doi: 10.1051/forest:2004091
Schmidt-Thomé, P. (2005). The spatial effects and management of natural and technological hazards in Europe. Final Rep. Euro. Spat. Plann. Obs. Netw. Proj. 1, 1–197.
Schulman, E. (1954). Longevity under adversity in conifers. Science 119, 396–399. doi: 10.1126/science.119.3091.396
Schweingruber, F. H., and Wirth, C. (2009). “Old trees and the meaning of ‘old’,” in Old-Growth Forests, eds C. Wirth, G. Gleixner, and M. Heimann (Berlin; Heidelberg: Springer Verlag), 35–54. doi: 10.1007/978-3-540-92706-8_3
Seim, A., Büntgen, U., Fonti, P., Haska, H., Herzig, F., Tegel, W., et al. (2012). Climate sensitivity of a millennium-long pine chronology from Albania. Clim. Res. 51, 217–228. doi: 10.3354/cr01076
Sperduto, D. D., Nichols, W. F., Crowley, K. F., and Bechtel, D. A. (2000). Black Gum (Nyssa Sylvatica Marsh) in New Hampshire. Concord, NH: NH Natural Heritage Inventory, Department of Resources and Economic Development.
Spurk, M., Leuschner, H. H., Baillie, M. G. L., Briffa, K. R., and Friedrich, M. (2002). Depositional frequency of German subfossil oaks: climatically and non-climatically induced fluctuations in the Holocene. Holocene 12, 707–716. doi: 10.1191/0959683602hl583rp
Stahl, U., Reu, B., and Wirth, C. (2014). Predicting species' range limits from functional traits for the tree flora of North America. Proc. Natl. Acad. Sci. U.S.A. 111, 13739–13744. doi: 10.1073/pnas.1300673111
Stahle, D. W. (1996). “Tree rings and ancient forest history,” in Eastern Old-Growth Forests, ed M. B. Davis (Washington, DC: Island Press), 321–343.
Stahle, D. W., Cleaveland, M. K., Griffin, R. D., Spond, M. D., Fye, F. K., Culpepper, R. B., et al. (2006). Decadal drought effects on endangered woodpecker habitat. EOS, Trans. Am. Geophys. Union 87, 121–125. doi: 10.1029/2006EO120002
Stahle, D. W., Griffin, R. D., Meko, D. M., Therrell, M. D., Edmondson, J. R., Cleaveland, M. K., et al. (2013). The Ancient Blue Oak Woodlands of California: longevity and hydroclimatic history. Earth Interact. 17, 1–23. doi: 10.1175/2013EI000518.1
Stephenson, N. L., and van Mantgem, P. J. (2005). Forest turnover rates follow global and regional patterns of productivity. Ecol. Lett. 8, 524–531. doi: 10.1111/j.1461-0248.2005.00746.x
Stephenson, N. L., van Mantgem, P. J., Bunn, A. G., Bruner, H., Harmon, M. E., O'Connell, K. B., et al. (2011). Causes and implications of the correlation between forest productivity and tree mortality rates. Ecol. Monogr. 81, 527–555. doi: 10.1890/10-1077.1
Stokes, M. A., and Smiley, T. L. (1996). An Introduction to Tree-ring Dating. Tucson, AZ: University of Arizona Press.
Suarez, M. L., Renison, D., Marcora, P., and Hensen, I. (2008). Age–size–habitat relationships for Polylepis australis: dealing with endangered forest ecosystems. Biodivers. Conserv. 17, 2617–2625. doi: 10.1007/s10531-008-9336-1
Trotsiuk, V., Hobi, M. L., and Commarmot, B. (2012). Age structure and disturbance dynamics of the relic virgin beech forest Uholka (Ukrainian Carpathians). For. Ecol. Manage. 265, 181–190. doi: 10.1016/j.foreco.2011.10.042
Vanderwel, M. C., Coomes, D. A., and Purves, D. W. (2013). Quantifying variation in forest disturbance, and its effects on aboveground biomass dynamics, across the eastern United States. Glob. Chang. Biol. 19, 1504–1517. doi: 10.1111/gcb.12152
Wirth, C., Messier, C., Bergeron, Y., Frank, D., and Fankhänel, A. (2009). “Old-growth forest definitions: a pragmatic view,” in Old-Growth Forests, eds C. Wirth, G. Gleixner, and M. Heimann (Heidelberg;Berlin: Springer), 11–33.
Zang, C. (2015). Dendrobox–An interactive exploration tool for the International Tree Ring Data Bank. Dendrochronologia 33, 31–33. doi: 10.1016/j.dendro.2014.10.002
Keywords: tree longevity, tree rings, old-growth forest, life history traits, temperate deciduous forest
Citation: Di Filippo A, Pederson N, Baliva M, Brunetti M, Dinella A, Kitamura K, Knapp HD, Schirone B and Piovesan G (2015) The longevity of broadleaf deciduous trees in Northern Hemisphere temperate forests: insights from tree-ring series. Front. Ecol. Evol. 3:46. doi: 10.3389/fevo.2015.00046
Received: 02 March 2015; Accepted: 19 April 2015;
Published: 15 May 2015.
Edited by:
Marc Macias-Fauria, University of Oxford, UKReviewed by:
Emad Farahat, Helwan University, EgyptCopyright © 2015 Di Filippo, Pederson, Baliva, Brunetti, Dinella, Kitamura, Knapp, Schirone and Piovesan. This is an open-access article distributed under the terms of the Creative Commons Attribution License (CC BY). The use, distribution or reproduction in other forums is permitted, provided the original author(s) or licensor are credited and that the original publication in this journal is cited, in accordance with accepted academic practice. No use, distribution or reproduction is permitted which does not comply with these terms.
*Correspondence: Gianluca Piovesan, Department of Agriculture, Forest, Nature and Energy, Via SC de Lellis, 01100 Viterbo, Italy,cGlvdmVzYW5AdW5pdHVzLml0
Disclaimer: All claims expressed in this article are solely those of the authors and do not necessarily represent those of their affiliated organizations, or those of the publisher, the editors and the reviewers. Any product that may be evaluated in this article or claim that may be made by its manufacturer is not guaranteed or endorsed by the publisher.
Research integrity at Frontiers
Learn more about the work of our research integrity team to safeguard the quality of each article we publish.