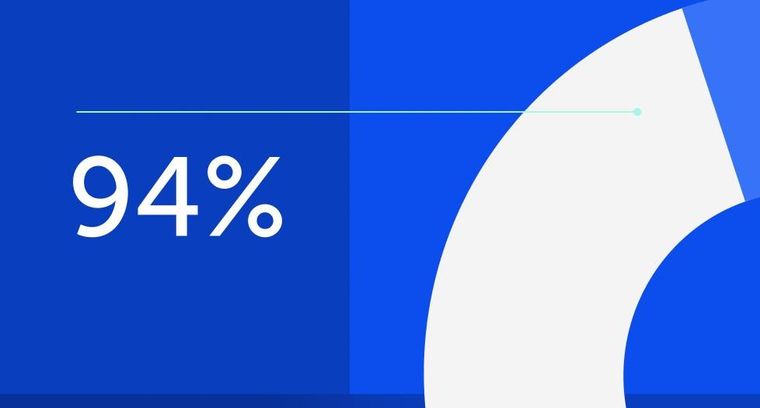
94% of researchers rate our articles as excellent or good
Learn more about the work of our research integrity team to safeguard the quality of each article we publish.
Find out more
ORIGINAL RESEARCH article
Front. Earth Sci., 15 April 2025
Sec. Economic Geology
Volume 13 - 2025 | https://doi.org/10.3389/feart.2025.1589956
The development of clean energy is crucial for the transition to a low-carbon economy, and hydrogen, with its high energy density and environmental friendliness, has become increasingly important. Coal, as a porous medium, provides a possible site for underground storage of hydrogen. The structural evolution of coal during coalification significantly influences hydrogen adsorption capabilities within nanopores, yet comprehensive studies evaluating the hydrogen adsorption and storage potential in coals of different ranks (low, medium, and high) remain limited. Our study innovatively addresses this research gap by investigating the hydrogen storage potential in low-, medium-, and high-rank coal seams, focusing particularly on how molecular structure affects pore structure and hydrogen adsorption mechanisms. Coal samples representing various coal ranks were collected from different basins in China. Multiple experimental techniques, including CO2 and low-temperature N2 adsorption, hydrogen adsorption isotherms, XRD, and HRTEM analyses, were employed to characterize coal structures and hydrogen adsorption properties comprehensively. Results indicate that coal is rich in nanopores. The evolution of coal molecular structure during coalification has a correlation with the microporous evolution characteristics, which determines the hydrogen adsorption capacity. With the increase of coal rank, the hydrogen adsorption capacity showed an obvious four-stage evolution pattern. High-rank coal with strong hydrogen adsorption capacity, faster adsorption rate and lower hydrogen retention after desorption, and is more suitable for underground hydrogen storage. These findings enhance the fundamental understanding of coal-hydrogen interactions and provide crucial guidelines for selecting optimal geological reservoirs for underground hydrogen storage.
The transition towards a low-carbon economy necessitates the development of clean and renewable energy sources, with hydrogen emerging as a promising solution due to its high energy density and environmental benefits (IEA, 2021; Reigstad et al., 2022; Hassan et al., 2023). As a key component in achieving global carbon neutrality, hydrogen energy has been increasingly recognized for its potential in various applications, including fuel cells, industrial processes, and energy storage systems (Van der Spek et al., 2022; Akhtar et al., 2023). China, as the world’s largest producer and consumer of hydrogen, has extensive supply experience and a robust industrial foundation, with an industrial hydrogen production capacity of up to 25 million t/a (Han et al., 2024). Among the key challenges in establishing a robust hydrogen economy is the need for efficient, large-scale, and long-term hydrogen storage solutions (Krevor et al., 2023).
Among the strategies for hydrogen storage, underground hydrogen storage (UHS) offers advantages such as enhanced safety, reduced land use, and minimized environmental impacts (Tarkowski, 2019; Sambo et al., 2022). Geological formations like saline aquifers, depleted oil and gas reservoirs, and abandoned coal seams are considered viable candidates for UHS (Heinemann et al., 2018; Deng et al., 2023; Li et al., 2024). A conceptual map of hydrogen storage in geologic reservoirs is illustrated in Figure 1. Among potential geological formations, coal seams represent a unique opportunity for hydrogen storage due to their abundant nanopore structures and inherent gas adsorption capacity (Karacan et al., 2011; Liu and Liu, 2023).The microporous nature of coal facilitates the physical adsorption of hydrogen molecules, offering a pathway for efficient gas containment (Yang et al., 2023; Zhu et al., 2024). Moreover, coal seams that are unsuitable for mining or already depleted of methane could be repurposed for hydrogen storage, thus adding economic and environmental value (Iglauer et al., 2021).
Figure 1. Schematic illustration of hydrogen storage mechanisms in depleted reservoirs. (modified from Deng et al., 2023).
Hydrogen storage faces significant challenges due to hydrogen’s low volumetric energy density and high diffusivity (Berta et al., 2018; Han et al., 2017). In geological reservoirs, adsorption greatly influences hydrogen storage efficiency. Coal, a porous and heterogeneous solid with complex physical and chemical structures (Firouzi et al., 2014), has attracted considerable attention for its gas adsorption properties. The adsorption capacity of coal is affected by factors such as coal rank, mineral composition, and pore structure (Han et al., 2024). Research on gas adsorption in coal typically investigates two primary mechanisms: physical adsorption and chemical adsorption. Physical adsorption depends on intermolecular forces, such as van der Waals forces, causing gas molecules to accumulate on the pore surfaces. Hydrogen, lacking a permanent dipole moment, primarily interacts with coal surfaces through dispersion forces or induced dipole effects (Buckingham et al., 1968). Chemical adsorption involves the formation of chemical bonds between gas molecules and reactive sites on the coal surface. Coal contains functional groups like hydroxyl, carboxyl, and carbonyl groups, which can chemically react with hydrogen, affecting adsorption behavior. Molecular simulations conducted by Zhang et al. (2024) demonstrated that pure hydrogen adsorbs more effectively onto kerogen compared to montmorillonite within shale nanopores, emphasizing the role of structural characteristics of the adsorption medium on adsorption selectivity. Tectonic deformation or increased coal maturity changes the microporous and macromolecular structures, which subsequently influences hydrogen adsorption capacity (Liu et al., 2021; Zhu et al., 2025). Experimental results from Liu and Liu (2023) indicate that high-rank coals, such as anthracite, exhibit notably higher hydrogen adsorption capacities than low-rank coals. Additionally, coal seams commonly contain various gases, such as methane (CH4), and carbon dioxide (CO2). Different gas molecules compete for adsorption sites on coal surfaces due to differences in molecular size, polarity, and adsorption affinity (Zhou et al., 2019). Collectively, these studies highlight the complex interplay among pressure, temperature, pore structure, and coal rank in controlling hydrogen adsorption and diffusion processes. Recent advancements in experimental techniques and molecular simulations have provided valuable insights into the adsorption mechanisms and competitive interactions of multi-component gases within coal seams (Wang and Chen, 2024).
Despite recent advances, research on hydrogen adsorption in coal, particularly across different ranks of coalification, remains limited. Understanding the relationship between coal rank, structural characteristics, and hydrogen storage capacity is crucial for optimizing geological reservoir selection and ensuring the long-term stability of stored hydrogen. This study systematically investigates hydrogen adsorption behaviors across various coalification degrees and evaluates coal’s potential as a hydrogen storage medium. By integrating experimental data with theoretical models, this research aims to enhance foundational knowledge, thereby advancing the development of underground hydrogen storage technologies.
China hosts numerous coal-bearing basins with abundant coal resources, primarily formed during the Paleozoic Carboniferous-Permian and Jurassic (Shao et al., 2020). The coal seams have undergone multiple phases of tectonic movements, resulting in a wide range of coal ranks, from low-rank to high-rank coals (Ju et al., 2021). In this study, five coal samples were collected from the Junggar basin, Qinshui basin, South North China basin, and Yong’an basin in China to investigate variations in coal composition, pore structure, and gas adsorption characteristics across different coal ranks (Figure 2a).
Figure 2. Geological survey and lithology histogram of study area. (a) Sampling locations and (b) Stratigraphic columns of coal-bearing strata of Junggar, Southern North China, Qinshui, and Yong’an basins.
The Junggar Basin is a key industrial base for mid-to low-rank coalbed methane in China. The Jimusaer Sag features multiple stratigraphic units overlying Carboniferous folded basement rocks. The coal seams are predominantly developed within the Jurassic Badaowan Formation and Xishanyao Formation (Figure 2b), with a low degree of coal metamorphism (Tao et al., 2018). Sample L-1 was collected from the Lower Jurassic Badaowan Formation and has the average vitrinite reflectance (Ro,m) of 0.41%, classifying it as low-rank coal. The South North China Basin, specifically the Huaibei Coalfield, lies within the Xuhuai Depression, a Carboniferous-Permian coal-accumulating basin dominated by medium-rank coals (Liu et al., 2009). Samples L-2 and M-1 were obtained from coal seam No. 8 of the Lower Permian Shanxi Formation (Figure 2b), with Ro,m of 0.81% and 1.22%, respectively, categorizing them as low-rank and medium-rank coal. The Qinshui Basin exhibits generally high coal rank due to magmatic activity during the Yanshanian period (Qiao et al., 2022). Sample H-1, collected from coal seam No. 3 of the Lower Permian Shanxi Formation (Figure 2b), with Ro,m of 2.94%, indicating high-rank coal. In the Yong’an Basin, the Tongziyan Formation of the Permian system is an important coal-bearing stratum. Due to multiple episodes of magmatic activity during the Yanshanian period, the coals have undergone extensive metamorphism, forming anthracite or coal-based graphite (Xu et al., 2019). Sample H-2, collected from coal seam No. 39 of the Permian Tongziyan Formation (Figure 2b), has a vitrinite reflectance of 5.32%, categorizing it as high-rank coal.
Table 1 shows that the five coal samples with different degrees of coalification are all dominated by carbon elements, ranging from 64.12% to 95.31%. The atomic ratios of H/C, O/C, and N/C gradually decreased with the increase of coal rank, indicating the shedding of heteroatoms in coal with coalification.
CO2 adsorption experiments were conducted following the GB/T 21650.3-2011 standard using an Autosorb IQ automatic surface area and pore size analyzer (Quantachrome Instruments, United States). Coal samples were crushed to particle sizes of 0.245-0.178 mm. Adsorption measurements were performed at 273.1 K under various relative pressures after degassing the samples under vacuum conditions. Adsorption curves were subsequently obtained and analyzed.
Low-temperature nitrogen adsorption experiments were conducted using high-purity nitrogen (>99.999%) as the adsorbate. Coal samples (0.245–0.178 mm) were degassed at 110°C–120°C under vacuum for 12 h to eliminate moisture and volatile components. Nitrogen adsorption-desorption isotherms were then measured at liquid nitrogen temperature (77 K), and the resulting data were analyzed to characterize the pore structures.
The pore structure parameters of micropores, mesopores and macropores of the samples were calculated using DFT (Density function theory) equations, respectively. Compared with other theoretical models, it has very obvious advantages (Ravikovitch et al., 2000): a more accurate characterization of the pore structure in the range of micro- and mesopores; consideration of the adsorption and phase behavior of the fluid in the narrow roar channel of the gas flow; a wider range of measured pore sizes; and a completer and more comprehensive picture of the development of the micro-, meso- and macro-pores.
According to existing studies, hydrogen exhibits a weaker affinity with coal compared to carbon dioxide and methane (Iglauer et al., 2021), and pressure sensors in conventional isothermal adsorption instruments are prone to significant errors during hydrogen adsorption testing. To address this, improvements were made to the pressure sensor in this study to enhance testing accuracy. The static volumetric method was used to conduct hydrogen isothermal adsorption experiments on coal samples, with a test temperature of 50°C and a pressure range of 0–18 MPa. The experimental setup for hydrogen isothermal adsorption, as shown in Figure 3, consists of a gas injection system, sample testing system, and vacuum exhaust system (Han et al., 2024). Each sample was prepared as a powder with particle sizes ranging from 0.245 to 0.178 mm, and approximately 30 g was loaded for each measurement. The samples were first vacuum-dried at 150°C for 6 h, followed by helium gas injection to calibrate the sample volume and the remaining free space in the sample. Since hydrogen is a flammable gas, the instrument’s airtightness was checked before injecting hydrogen. The hydrogen adsorption amount at different pressure values was automatically recorded by the software, and the hydrogen isothermal adsorption curve was plotted using the adsorption data obtained at various pressures.
Figure 3. Experimental set-up of hydrogen isothermal adsorption (modified after Han et al., 2024).
The Langmuir adsorption model is widely used to describe gas adsorption behaviors in porous materials, particularly for gases which interact with coal at the molecular level (Langmuir, 1916; Babatunde et al., 2022). The model assumes a monolayer adsorption process, where gas molecules adsorb onto a finite number of adsorption sites on the coal surface, with each site capable of adsorbing only one molecule. This is particularly suitable for hydrogen sorption in coal, as it reflects the process where hydrogen molecules occupy the available adsorption sites without further interaction with adjacent molecules once the sites are saturated. The Langmuir model is written as shown in Equation 1:
where
X-ray diffraction (XRD) has been widely used for crystal structure studies in coal (Song et al., 2011). XRD provides detailed insights into the crystallographic structure, enabling a deeper investigation into coal metamorphic grades, formation mechanisms, and the distribution of minerals within coal. Specifically, as coal metamorphic grade increases, aromatic layers become larger and more ordered. These changes are reflected in the XRD spectra by stronger peak intensities, narrower peak widths, and corresponding changes in interlayer spacing (Machado et al., 2013). A Panalytical Empyrean X-ray diffractometer from Netherlands was adopted for XRD tests. Copper Kα radiation (40 kV, 40 mA) source was used as an X-ray source. And the X-ray wavelength λ is 0. 1,540,598 nm. X-ray spectrogram was recorded for the scattering range of 5°–80° (2θ) at a scanning speed of 2°/min. The average lateral sizes (La), stacking heights (Lc) and interlayer spacing (d002) of crystallite in coal obtained from XRD test were calculated by Bragg’s equation and Scherrer formula (Takagi et al., 2004). La, Lc, d002 according to Equations 2–4, respectively. The average number of stacked layers (Nc) was calculated by the Equation 5 (Suárez-Ruiz and García, 2007).
where θ002 and θ100 are the diffraction angles corresponding to the (002) peak and (100) peak. The β002 and β100 are the FWHM (full width at half maximum) corresponding to the (002) peak and (100) peak.
High Resolution Transmission Electron microscope (HRTEM) is a powerful means to characterize the spatial structure of coal (Mathews and Sharma, 2012; Pan et al., 2015). HRTEM complements XRD by visually revealing the microstructural morphology of coal and quantitatively analyzing the aromatic layers using advanced computer-aided image processing techniques. Thus, HRTEM serves as a powerful method for characterizing the spatial molecular structure of coal (Wang et al., 2018). The resolution of HRTEM is generally 0.1–0.2 nm, which allows chemical analysis and crystal structure analysis of tiny regions of a few nanometers. HRTEM is able to observe the microscopic morphology of coal at the while quantitatively obtaining the streak structure of aromatic lamellae in coal with the help of advanced computer image processing software. The HRTEM analysis was conducted using a JEM-2100F microscope, manufactured by JEOL, Japan. The powder sample was dispersed in anhydrous ethanol using ultrasonic waves. Subsequently, a droplet of this solution was deposited onto a microgrid using an eyedropper and allowed to dry before HRTEM observation. Initially, the overall morphology of the sample was observed under low magnification, followed by recording the atomic fringe images at high magnification.
Table 2 presents the experimental results of low-temperature CO2 and N2 adsorption analyses. The coal samples demonstrate the most developed micropore structure, with micropores contributing higher total pore volume and significantly greater specific surface area compared to mesopores. CO2 adsorption primarily characterizes monolayer adsorption or micropore filling in pores below 2 nm diameter. DFT model calculations reveal that the five coal samples exhibit pore volumes ranging from 0.039 to 0.087 cm3/g, with a mean value of 0.062 cm3/g. With increasing metamorphic degree, the total micropore volume displays a sequential trend of initial decrease, subsequent increase, and final reduction, while the specific surface area follows an inverse pattern of initial increase, subsequent decrease, and final augmentation.
The CO2 adsorption isotherm curves are shown in Figure 4a. All samples exhibit progressively increasing CO2 adsorption capacities with rising relative pressure. Sample H-1 demonstrates the maximum CO2 adsorption volume of 27.61 cm3/g, indicating superior microporosity development. Figure 4b illustrates the pore size distribution derived from CO2 adsorption data. All samples display pore size distribution curves containing three or more narrow peaks. The primary peaks for samples L-1, L-2, M-1, H-1, and H-2 predominantly occur between 0.35 nm and 0.85 nm, suggesting that coal micropores are principally distributed within this size range. Notably, smaller micropores within this distribution contribute disproportionately greater pore volume.
Figure 4. Pore structure characteristics of coal samples with different metamorphic degrees. (a) CO2 adsorption curves at various rank coals; (b) Structure of 0.3–2 nm pore volumes distribution obtained by CO2 adsorption; (c) Low-pressure N2 adsorption isotherms in coal samples; (d) Structure of 1–100 nm pore volumes distribution obtained by N2 adsorption.
Low-temperature N2 adsorption results indicate well-developed nanopores (1–100 nm) within the coal samples. Isotherm analyses reveal significant desorption hysteresis in all samples (Figure 4c), with Type H4 hysteresis loops observed in the adsorption-desorption curves, indicative of slit-shaped pore structures. Notably, organic microstructure was identified as a critical factor influencing nitrogen adsorption capacity. Figure 4c illustrates pronounced variations in nitrogen adsorption performance across samples. Quantitative analyses demonstrate that high-rank sample H-2 exhibited the strongest nitrogen adsorption capacity, medium-rank sample M-2 displayed intermediate adsorption volumes, while low-rank samples L-1 and L-2 showed minimal adsorption. These results confirm that adsorbed nitrogen volumes increased progressively with coal rank elevation.
Pore size distributions (PSDs) derived from gas adsorption measurements are plotted as incremental pore volume in Figure 4d. The PSD curves exhibit multiple complex peaks, reflecting highly heterogeneous pore structures. All organic samples contain abundant nanopores spanning 1–100 nm in diameter. A marked increase in incremental pore volume was observed with decreasing pore diameter, particularly within the 1–10 nm range. Significant disparities in PSDs exist between microporous and finer mesoporous size fractions: sample H-2 contains more micropores and finer mesopores (1–10 nm), whereas other samples show reduced proportions of finer mesopores (5–10 nm).
To quantitatively evaluate the evolution of crystal structure of coal, XRD parameters including d002, La, Lc, La/Lc and Nc were are given in Figure 5. As shown in Figure 5b, the d002 parameter (interlayer spacing) decreases progressively with increasing vitrinite reflectance (Ro,m), indicating gradual compaction of aromatic lamellae in coal crystallites during coalification, approaching the ideal graphite interlayer spacing (∼0.335 nm). Concurrently, the lateral crystallite dimension (La) demonstrates sustained growth with elevated Ro,m (Figure 5c), reflecting continuous aromatic layer extension. The stacking height (Lc) exhibits distinct evolutionary phases: gradual enhancement at Ro,m < 2.0%, followed by an initial decline and subsequent resurgence at Ro,m > 2.0% (Figure 5d). The average stacking layer number (Nc) mirrors this trend, increasing from 5 to 11 layers, then decreasing to 9 layers before rising to 12 layers (Figure 7e). These patterns suggest preliminary crystallite stacking during low-to-medium rank coalification, temporary stagnation at high-rank stages, and renewed stacking activity with advanced maturation. The La/Lc ratio, indicative of crystallite growth orientation, rises systematically from 0.47 to 2.56 with increasing Ro,m This trend confirms that crystallite development during coalification predominantly occurs through lateral expansion rather than vertical stacking.
The microscopic morphology of five coal samples, as shown in Figures 6a, d, g, j, m, was analyzed using advanced image processing techniques described by Li et al. (2024) to resolve aromatic layer edge structures (Figures 6b, e, h, k, n). Sample L-1 exhibits a rough surface morphology with pronounced granular texture, characterized by overlapping lattice fringes and irregular particle boundaries. In contrast, samples L-2 and M-3 display finer particle sizes while retaining irregular edge configurations. High-rank samples H-1 and H-2 demonstrate comparatively smoother morphologies with preferential alignment of structural features, where H-2 shows particularly well-defined particle edges.
Figure 6. HRTEM images of five coal samples. (a, d, g, j, m): original observation image; (b, e, h, k, n): edge structure extraction results of aromatic lamellae; (c, f, i, l, o): distribution frequency of aromatic rings of different sizes.
Niekerk and Mathews (2010) proposed a model assuming parallelogram-shaped aromatic fringes in coal, from which they calculated the maximum and minimum lengths of aromatic clusters ranging from naphthalene rings (2 × 2) to 8 × 8 configurations. Due to observational uncertainties in determining whether individual fringes align with their maximum or minimum dimensions, their methodology calculates average cluster lengths and classifies lattice fringes by size. Fringes shorter than 3 Å were treated as noise, thus excluding benzene rings (typically <3 Å) from the classification. Adopting this framework, we statistically categorized lattice fringe lengths extracted from five coal samples. The frequency distributions of aromatic cluster sizes are presented in Figures 6c, f, i, l, o.
Figure 7 reveals stage-dependent evolution of aromatic ring clusters with increasing vitrinite reflectance (Ro,m). Naphthalene rings (2 × 2 clusters) exhibit distinct evolutionary behavior compared to larger aromatic systems. At low coal ranks (Ro,m < 0.9%), naphthalene rings dominate with an initial frequency of 47.47%. This frequency decreases progressively with rising Ro,m, shows transient recovery between Ro,m = 0.9–1.2%, and ultimately declines to 30.87% at higher maturity. This pattern reflects early-stage macromolecular reorganization where naphthalene rings undergo detachment, as corroborated by Liu (2019). During medium-rank stages (Ro,m = 0.9–1.2%), residual carbon structures regenerate naphthalene rings via aromatization, temporarily increasing their abundance. At high ranks (Ro,m>1.2%), naphthalene ring depletion resumes due to condensation into larger aromatic assemblies.
Figure 7. Stage-dependent evolutionary characteristics of aromatic ring clusters across varying sizes with Ro,m.
Smaller aromatic clusters (2 × 2) demonstrate progressive decline, influenced by dual mechanisms: (1) initial detachment during structural rearrangement, and (2) dynamic equilibrium between aromatization-derived regeneration and condensation-driven consumption. Notably, their abundance stabilizes during intermediate coalification stages before marked reduction at advanced maturity. Mid-sized clusters (3 × 3 and 4 × 4) exhibit complementary trends to naphthalene rings, displaying frequency increases that inversely correlate with 2 × 2 cluster depletion. This inverse relationship positions 2 × 2 clusters as transitional units bridging smaller and larger aromatic systems. Larger clusters (5 × 5 to 8 × 8) follow evolutionary trajectories mirroring mid-sized clusters at Ro,m< 1.2%, consistent with condensation-dominated growth during low-to-medium rank stages. However, a phase inversion occurs at Ro,m>5.0%, where mid-sized clusters (3 × 3/4 × 4) become primary precursors for large cluster formation through intensified condensation.
Figure 8a presents hydrogen adsorption isotherms for five coal samples under varying pressure conditions. All isotherms conform to Type I classification per International Union of Pure and Applied Chemistry (IUPAC) standards, exhibiting monotonic adsorption capacity increases with rising pressure. High-rank coals (H-1, H-2) demonstrate significantly greater hydrogen adsorption capacities than medium-rank (M-1) and low-rank coals (L-1, L-2), with high-rank samples adsorbing approximately threefold more hydrogen than low-rank counterparts. At the target pressure of 18 MPa, adsorption capacities display a complex progression with increasing Ro,m: initial increase, subsequent decrease, renewed increase, final reduction. Notably, sample L-1 shows lower adsorption than L-2, M-1 underperforms L-2, and H-2 exhibits reduced capacity compared to H-1.
Adsorption rates, calculated as hydrogen uptake per unit equilibrium time across pressure intervals (Figure 8b), reveal three distinct phases. 0–1 MPa: Rate decrease due to rapid surface site saturation; 1–4 MPa: Rate increase from macropore filling (large pore volumes); >4 MPa: Rate decline as adsorption transitions to meso-micropores. High-rank coals maintain superior adsorption rates at <4 MPa, with H-2 achieving peak performance. However, beyond 4 MPa, H-2’s rate plummets below H-1’s, while M-1’s rate drops below L-2’s at 18 MPa. This behavior correlates with pore structure parameters: H-2’s inferior microporous specific surface area and total pore volume limit high-pressure adsorption. Similarly, M-1’s microporosity deficit compared to L-2 (explains its rate inversion above 4 MPa.
Coal structural evolution during maturation controls the development of organic nanoporosity in a stage manner. This evolutionary pattern has been partially characterized across coal ranks from lignite to anthracite. Chen et al. (2023) documented phased changes in aromatic fringe lengths within medium-rank coals (Ro,m = 0.68%–1.98%). Adsorptive capacity demonstrates maturation-dependent nonlinearity (Liu et al., 2022), where high-rank coals initially exhibit enhanced adsorption due to optimized pore networks, followed by capacity decline as structural ordering progresses toward graphitization. The process marked by aromatic layer densification and partial micropore occlusion.
Figure 9 presents our proposed model linking organic nanoporosity evolution to hydrogen adsorption capacity across four distinct maturation stages: Stage I (Ro,m = 0.4%–0.9%): Oxygen-functional group elimination and aliphatic side-chain formation dominate, increasing micropore volume through structural disaggregation. Stage II (Ro,m = 0.9%–1.2%): Aromatization of residual carbon structures generates small aromatic clusters (benzene/naphthalene rings), reducing micropore accessibility. Stage III (Ro,m = 1.2%–5.0%): Progressive aromatic condensation reactions create extended microporous networks, significantly enhancing pore volume. Stage IV (Ro,m > 5.0%): Structural alignment approaching graphitic order reduces microporosity through lamellar stacking and pore closure.
Underground hydrogen storage capacity of coal is fundamentally governed by its organic pore types and structural configurations (Zhu et al., 2025). Coal samples of varying metamorphic grades exhibit distinct organic nanostructures and nanoporosity, resulting in divergent gas storage potentials. Figures 4–7 systematically characterize organic pore architectures across coal ranks using gas physisorption, XRD, and HRTEM imaging. Sample-specific variations in specific surface area pore volume, and pore size distribution critically modulate hydrogen adsorption behavior. Micropores (<2 nm), with their high surface area and strong adsorption potential, dominate low-pressure hydrogen uptake (Figure 8). Mesopores (2–50 nm) enhance gas transport through microporous network connectivity, particularly in high-rank coals.
High-rank coals (H-series) exhibit microporosity-dominated systems, enabling rapid hydrogen adsorption at low pressures (<4 MPa). Notably, these coals maintain non-zero adsorption rates even at 18 MPa (Figure 8d), suggesting persistent storage capacity under extreme conditions. Sample H-2 (Ro,m = 5.32%), with its developed mesoporous network, demonstrates superior performance in high-pressure/dynamic cycling regimes due to enhanced gas diffusivity.
During the hydrogen extraction stage post storage, hydrogen is desorbed from the nanoscale pores of the coal as the pressure decreases. However, due to the pore structure limitation and other complex factors, all coal samples have some hydrogen residue in the coal hydrogen, as shown in Figure 10a. The amount of hydrogen residue in different coal samples was calculated as in Figure 10b. In which the low-rank coal retained much more residual hydrogen than the high-rank coal. This suggests that higher rank coals have the least hydrogen loss through adsorption during the extraction process, thus improving the hydrogen recovery efficiency.
Figure 10. Hydrogen desorption characteristics. (a) Schematic of hydrogen desorption residue (b) Residual hydrogen in the sample after desorption.
Despite recent progress, several challenges remain for underground hydrogen storage in coal. Competitive adsorption with co-existing gases significantly reduces hydrogen storage efficiency (Merkel et al., 2015). Additionally, polar molecules such as water (H2O) preferentially occupy oxygen-containing functional groups on coal surfaces, further limiting hydrogen adsorption (Xiang and Lei, 2021). Geological stress-induced permeability reduction represents another substantial challenge (Masoudi et al., 2025). Lastly, microbial activities in coal seams significantly impact hydrogen storage efficacy and introduce economic constraints (Su et al., 2018). Future research should comprehensively address these challenges by systematically characterizing coal heterogeneity, clarifying competitive adsorption mechanisms, modeling permeability evolution under reservoir conditions, mitigating leakage risks (Wood, 2023), and developing microbial control strategies, thereby advancing the practical viability of coal seam hydrogen storage.
(1) Coals of varying coalification contain abundant nanopores (<100 nm), primarily micropores (<2 nm), which significantly influence hydrogen adsorption through their large specific surface area.
(2) XRD and HRTEM analyses reveal four distinct stages in the structural evolution of aromatic layers with increasing coalification, demonstrating progressive structural condensation and increased lamellar ordering.
(3) Systematic correlations exist among coal maturity, nanostructure, and hydrogen adsorption. With increasing Ro,m both micropore volume and hydrogen adsorption capacity exhibit a four-stage evolutionary pattern: initial increase, subsequent decrease, renewed growth, and final decline. While enhanced Ro,m (below 5.0%) promotes microporosity development and improves adsorption capacity, structural densification at Ro,m>5.0% reduces micropore volume, thereby diminishing hydrogen adsorption potential.
(4) High-rank coals exhibit superior hydrogen adsorption capacity, rapid adsorption rates, and minimal residual hydrogen post-desorption, making them optimal candidates for underground hydrogen storage compared to lower-rank coals.
These insights represent innovative contributions, deepening our fundamental understanding of coal-hydrogen interactions and providing practical guidance for selecting optimal geological reservoirs for hydrogen storage.
The raw data supporting the conclusions of this article will be made available by the authors, without undue reservation.
LT: Conceptualization, Data curation, Methodology, Writing – original draft, Writing – review and editing. YJ: Conceptualization, Funding acquisition, Resources, Writing – review and editing.
The author(s) declare that financial support was received for the research and/or publication of this article. We acknowledge the financial supports provided by National Key Research and Development Program of China (2023YFF0804304), the National Natural Science Foundation of China (Grant Nos. 42372153).
The authors declare that the research was conducted in the absence of any commercial or financial relationships that could be construed as a potential conflict of interest.
The author(s) declare that no Generative AI was used in the creation of this manuscript.
All claims expressed in this article are solely those of the authors and do not necessarily represent those of their affiliated organizations, or those of the publisher, the editors and the reviewers. Any product that may be evaluated in this article, or claim that may be made by its manufacturer, is not guaranteed or endorsed by the publisher.
Akhtar, M. S., Khan, H., Liu, J. J., and Na, J. (2023). Green hydrogen and sustainable development – a social LCA perspective highlighting social hotspots and geopolitical implications of the future hydrogen economy. J. Clean. Prod., 395, 136438. doi:10.1016/j.jclepro.2023.136438
Babatunde, K. A., Negash, B. M., Jufar, S. R., Ahmed, T. Y., and Mojid, M. R. (2022). Adsorption of gases on heterogeneous shale surfaces: a review. J. Petroleum Sci. Eng. 208, 109466. doi:10.1016/j.petrol.2021.109466
Berta, M., Dethlefsen, F., Ebert, M., Schäfer, D., and Dahmke, A. (2018). Geochemical effects of millimolar hydrogen concentrations in groundwater: an experimental study in the context of subsurface hydrogen storage. Environ. Sci. Technol. 52 (8), 4937–4949. doi:10.1021/acs.est.7b05467
Buckingham, A., Disch, R., and Dunmur, D. (1968). Quadrupole moments of some simple molecules. J. Am. Chem. Soc. 90 (12), 3104–3107. doi:10.1021/ja01014a023
Chen, X., Li, M., Zhang, M., and Shao, Y. (2023). Manipulation of micropores in medium-rank coals by the evolution of aggregated structures during coalification: an HRTEM-based analysis. Fuel 350, 128380. doi:10.1016/j.fuel.2023.128380
Deng, P., Chen, Z., Peng, X., Wang, J., Zhu, S., Ma, H., et al. (2023). Optimized lower pressure limit for condensate underground gas storage using a dynamic pseudo-component model. Energy, 285, 129505. doi:10.1016/j.energy.2023.129505
Firouzi, M., Rupp, E., Liu, C., and Wilcox, J. (2014). Molecular simulation and experimental characterization of the nanoporous structures of coal and gas shale. Int. J. Coal Geol. 121, 123–128. doi:10.1016/j.coal.2013.11.003
Han, J., Bogomolov, A., Makarova, E., Yang, Z., Lu, Y., and Li, X. (2017). Molecular simulations on adsorption and diffusion of CO2 and CH4 in moisture coals. Energy and fuels. Energy Fuels 21 (12), 13528–13535. doi:10.1021/acs.energyfuels.7b02898
Han, S., Wang, J., Huang, J., Jin, Z., Liu, Q., Wan, Y., et al. (2024). Hydrogen adsorption characteristics of coal rock and its geological significance. J. China Coal Soc. 49 (3), 1501–1517. doi:10.13225/j.cnki.jccs.XH23.1523
Hassan, Q., Sameen, A. Z., Salman, H. M., Jaszczur, M., and Al-Jiboory, A. K. (2023). RETRACTED: hydrogen energy future: advancements in storage technologies and implications for sustainability. J. Energy Storage, 72, 108404. doi:10.1016/j.est.2023.108404
Heinemann, N., Booth, M. G., Haszeldine, R. S., Wilkinson, M., Scafidi, J., and Edlmann, K. (2018). Hydrogen storage in porous geological formations – onshore play opportunities in the midland valley (Scotland, UK). Int. J. Hydrogen Energy, 43(45), 20861–20874. doi:10.1016/j.ijhydene.2018.09.149
IEA (2021). Global hydrogen review 2021. Available online at: www.iea.org/t&c/.
Iglauer, S., Abid, H., Al-Yaseri, A., and Keshavarz, A. (2021). Hydrogen adsorption on sub-bituminous coal: implications for hydrogen geo-storage. Geophys. Res. Lett., 48(10), e2021GL092976. doi:10.1029/2021GL092976
Ju, Y., Yu, K., Wang, G., Li, W., Zhang, K., Li, S., et al. (2021). Coupling response of the Meso–Cenozoic differential evolution of the North China Craton to lithospheric structural transformation. Earth Sci. Rev., 223, 103859. doi:10.1016/j.earscirev.2021.103859
Karacan, C. Ö., Ruiz, F. A., Cotè, M., and Phipps, S. (2011). Coal mine methane: a review of capture and utilization practices with benefits to mining safety and to greenhouse gas reduction. Int. J. Coal Geol., 86(2), 121–156. doi:10.1016/j.coal.2011.02.009
Krevor, S., de Coninck, H., Gasda, S. E., Ghaleigh, N. S., de Gooyert, V., Hajibeygi, H., et al. (2023). Subsurface carbon dioxide and hydrogen storage for a sustainable energy future. Nat. Rev. Earth Environ. 4 (2), 102–118. doi:10.1038/s43017-022-00376-8
Langmuir, I. (1916). The constitution and fundamental properties of solids and liquids. Part I. solids. J. Am. Chem. Soc. 38 (11), 2221–2295. doi:10.1021/ja02268a002
Li, X., Huo, T., Wei, K., Yan, Z., Zhu, L., and Xue, Q. (2024). The feasibility of hydrogen storage in aquifers: a molecular dynamics simulation. Fuel 367, 131469. doi:10.1016/j.fuel.2024.131469
Liu, A., and Liu, S. (2023). Hydrogen sorption and diffusion in coals: implications for hydrogen geo-storage. Appl. Energy 334, 120746. doi:10.1016/j.apenergy.2023.120746
Liu, D., Qiu, F., Liu, N., Cai, Y., Guo, Y., Zhao, B., et al. (2022). Pore structure characterization and its significance for gas adsorption in coals: a comprehensive review. Unconv. Resour. 2, 139–157. doi:10.1016/j.uncres.2022.10.002
Liu, D., Yao, Y., Tang, D., Tang, S., Che, Y., and Huang, W. (2009). Coal reservoir characteristics and coalbed methane resource assessment in Huainan and Huaibei coalfields, Southern North China. Int. J. Coal Geol., 79(3), 97–112. doi:10.1016/j.coal.2009.05.001
Liu, Y., Liu, S., Zhang, R., and Zhang, Y. (2021). The molecular model of Marcellus shale kerogen: experimental characterization and structure reconstruction. Int. J. Coal Geol. 246, 103833. doi:10.1016/j.coal.2021.103833
Liu, Y. (2019). Effect of coal vitrinite macromolecular structure evolution on methane adsorption capacity at molecular level. Environ. Sci. Technol. Doctoral dissertation. China University of Mining and Technology. (in Chinese).
Machado, A., Mexias, A., Vilela, A., and Osorio, E. (2013). Study of coal, char and coke fines structures and their proportions in the off-gas blast furnace samples by X-ray diffraction. Fuel. 114, 224–228. doi:10.1016/j.fuel.2012.07.064
Masoudi, M., Nooraiepour, M., Blom, R., Xi, K., Cerasi, P., and Hellevang, H. (2025). Impact of hydration on hydrogen sorption in clay minerals and shale caprocks: implications for hydrogen energy and waste storage. Int. J. Hydrogen Energy 99, 661–670. doi:10.1016/j.ijhydene.2024.12.247
Mathews, J. P., and Sharma, A. (2012). The structural alignment of coal and the analogous case of Argonne Upper Freeport coal. Fuel, 95, 19–24. doi:10.1016/j.fuel.2011.12.046
Merkel, A., Gensterblum, Y., Busch, A., and Krooss, B. M. (2015). Competitive sorption of CH4, CO2 and H2O on natural coals of different rank. Int. J. Coal Geol. 150-151, 181–192. doi:10.1016/j.coal.2015.09.006
Niekerk, D. V., and Mathews, J. P. (2010). Molecular representations of Permian-aged vitrinite-rich and inertinite-rich South African coals. Fuel 89, 73–82. doi:10.1016/j.fuel.2009.07.020
Pan, J., Wang, S., Ju, Y., Hou, Q., Niu, Q., Wang, K., et al. (2015). Quantitative study of the macromolecular structures of tectonically deformed coal using high-resolution transmission electron microscopy. J. Nat. Gas Sci. Eng., 27, 1852–1862. doi:10.1016/j.jngse.2015.11.012
Qiao, P., Ju, Y., Yu, K., Ju, L., Xiao, L., Feng, H., et al. (2022). Nanoscale quantitative characterization of microstructure evolution of partly graphitized high rank coal: evidence from AFM and HRTEM. Fuel, 324, 124802. doi:10.1016/j.fuel.2022.124802
Ravikovitch, P. I., Vishnyakov, A., Russo, R., and Neimark, A. V. (2000). Unified approach to pore size characterization of microporous carbonaceous materials from N2, Ar, and CO2 adsorption isotherms. Langmuir 16 (5), 2311–2320. doi:10.1021/la991011c
Reigstad, G. A., Roussanaly, S., Straus, J., Anantharaman, R., de Kler, R., Akhurst, M., et al. (2022). Moving toward the low-carbon hydrogen economy: experiences and key learnings from national case studies. Adv. Appl. Energy, 8, 100108. doi:10.1016/j.adapen.2022.100108
Sambo, C., Dudun, A., Samuel, S. A., Esenenjor, P., Muhammed, N. S., and Haq, B. (2022). A review on worldwide underground hydrogen storage operating and potential fields. Int. J. Hydrogen Energy, 47(54), 22840–22880. doi:10.1016/j.ijhydene.2022.05.126
Shao, L., Wang, X., Wang, D., Li, M., Wang, S., Li, Y., et al. (2020). Sequence stratigraphy, paleogeography, and coal accumulation regularity of major coal-accumulating periods in China. Int. J. Coal Sci. and Technol. 7 (2), 240–262. doi:10.1007/s40789-020-00341-0
Song, D., Yang, C., Zhang, X., Su, X., and Zhang, X. (2011). Structure of the organic crystallite unit in coal as determined by X-ray diffraction. Min. Sci. Technol. (China), 21(5), 667–671. doi:10.1016/j.mstc.2011.10.004
Su, X., Zhao, W., and Xia, D. (2018). The diversity of hydrogen-producing bacteria and methanogens within an in situ coal seam. Biotechnol. Biofuels 11, 245. doi:10.1186/s13068-018-1237-2
Suárez-Ruiz, I., and García, A. B. (2007). Optical parameters as a tool to study the microstructural evolution of carbonized anthracites during high-temperature treatment. Energy Fuels 21 (5), 2935–2941. doi:10.1021/ef700221r
Takagi, H., Maruyama, K., Yoshizawa, N., Yamada, Y., and Sato, Y. (2004). XRD analysis of carbon stacking structure in coal during heat treatment. Fuel 83 (17), 2427–2433. doi:10.1016/j.fuel.2004.06.019
Tao, S., Chen, S., Tang, D., Zhao, X., Xu, H., and Li, S. (2018). Material composition, pore structure and adsorption capacity of low-rank coals around the first coalification jump: a case of eastern Junggar Basin, China. Fuel, 211, 804–815. doi:10.1016/j.fuel.2017.09.087
Tarkowski, R. (2019). Underground hydrogen storage: characteristics and prospects. Renew. Sustain. Energy Rev., 105, 86–94. doi:10.1016/j.rser.2019.01.051
Van der Spek, M., Banet, C., Bauer, C., Gabrielli, P., Goldthorpe, W., Mazzotti, M., et al. (2022). Perspective on the hydrogen economy as a pathway to reach net-zero CO2 emissions in Europe. Energy Environ. Sci. 15 (3), 1034–1077. doi:10.1039/D1EE02118D
Wang, G., and Chen, W. (2024). Molecular simulations of hydrogen adsorption on coal with different ranks: implications for hydrogen geo-storage. Int. J. Hydrogen Energy, 51, 10–20. doi:10.1016/j.ijhydene.2023.10.173
Wang, S., Tang, Y., Chen, H., Liu, P., and Sha, Y. (2018). Chemical structural transformations of different coal components at the similar coal rank by HRTEM in situ heating. Fuel 218, 140–147. doi:10.1016/j.fuel.2018.01.024
Wood, D. (2023). Variable interaction empirical relationships and machine learning provide complementary insight to experimental horizontal wellbore cleaning results. Adv. Geo-Energy Res. 9 (3), 172–184. doi:10.46690/ager.2023.09.05
Xiang, J., and Lei, L. (2021). Study on influence of coal surface functional groups on methane and carbon dioxide adsorption properties. Coal Sci. Technol. 49 (6), 145–151. doi:10.13199/j.cnki.cst.2021.06.017
Xu, Q., Liu, S., Wang, Z., and Zhang, B. (2019). Provenance of the East Guangdong Basin and Yong’an Basin in southeast China: response to the mesozoic tectonic regime transformation. J. Asian Earth Sci., 185, 104024. doi:10.1016/j.jseaes.2019.104024
Yang, H., Kang, N., Chen, X., and Liu, Y. (2023). Exploring the inhibitory effect of H2O on CO2/CH4 adsorption in coal: insights from experimental and simulation approaches. Energy, 284, 129317. doi:10.1016/j.energy.2023.129317
Zhang, M., Yang, Y., Pan, B., Liu, Z., Jin, Z., and Iglauer, S. (2024). Molecular simulation on H2 adsorption in nanopores and effects of cushion gas: implications for underground hydrogen storage in shale reservoirs. Fuel 361, 130621. doi:10.1016/j.fuel.2023.130621
Zhou, W., Wang, H., Zhang, Z., Chen, H., and Liu, X. (2019). Molecular simulation of CO2/CH4/H2O competitive adsorption and diffusion in brown coal. RSC Adv. 9, 3004–3011. doi:10.1039/c8ra10243k
Zhu, H., Ju, Y., Lu, Y., Yang, M., Feng, H., Qiao, P., et al. (2025). Natural evidence of organic nanostructure transformation of shale during bedding-parallel slip. GSA Bull. doi:10.1130/B37712.1
Keywords: underground hydrogen storage, coal rank, nanopores, hydrogen adsorption, pore structure
Citation: Tao L and Ju Y (2025) Hydrogen storage potential of coal: influence of coal on pore structure and adsorption mechanisms. Front. Earth Sci. 13:1589956. doi: 10.3389/feart.2025.1589956
Received: 08 March 2025; Accepted: 24 March 2025;
Published: 15 April 2025.
Edited by:
Guochang Wang, Saint Francis University, United StatesReviewed by:
Yanjun Lu, Yanshan University, ChinaCopyright © 2025 Tao and Ju. This is an open-access article distributed under the terms of the Creative Commons Attribution License (CC BY). The use, distribution or reproduction in other forums is permitted, provided the original author(s) and the copyright owner(s) are credited and that the original publication in this journal is cited, in accordance with accepted academic practice. No use, distribution or reproduction is permitted which does not comply with these terms.
*Correspondence: Liru Tao, dGFvbGlydTIwMThAMTYzLmNvbQ==; Yiwen Ju, anV5d0B1Y2FzLmFjLmNu
Disclaimer: All claims expressed in this article are solely those of the authors and do not necessarily represent those of their affiliated organizations, or those of the publisher, the editors and the reviewers. Any product that may be evaluated in this article or claim that may be made by its manufacturer is not guaranteed or endorsed by the publisher.
Research integrity at Frontiers
Learn more about the work of our research integrity team to safeguard the quality of each article we publish.