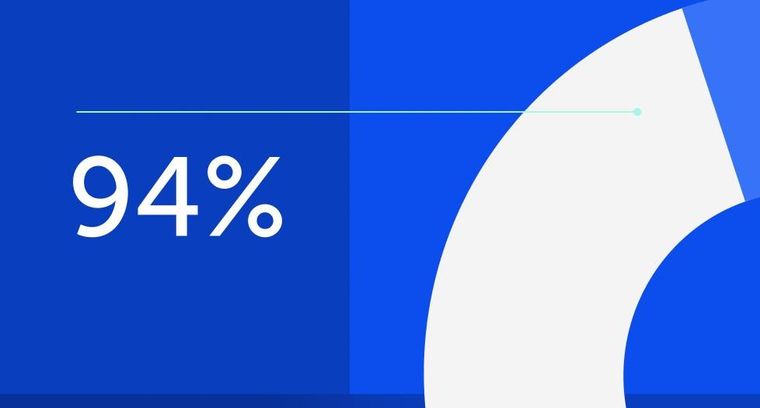
94% of researchers rate our articles as excellent or good
Learn more about the work of our research integrity team to safeguard the quality of each article we publish.
Find out more
ORIGINAL RESEARCH article
Front. Earth Sci., 02 April 2025
Sec. Economic Geology
Volume 13 - 2025 | https://doi.org/10.3389/feart.2025.1570304
This article is part of the Research TopicExploration, Development, and Protection of Earth’s Resources and Environment: Methods, Techniques, Applications, Prospects, Insights, and ProblemsView all 45 articles
Coal seams have been recognized as a promising geological reservoir for lithium, and its enrichment is intimately correlated with the original coal-forming parent materials and sedimentary microfacies. Nevertheless, the influence of coal facies on lithium enrichment has hitherto been scarcely documented. In this research endeavor, with the aim of unraveling the effects of coal facies on the lithium enrichment within coal measure strata, a combination of optical microscope examination, the step-by-step chemical extraction (SCEP) technique, inductively coupled plasma mass spectrometry (ICPMS), and scanning electron microscopy (SEM) was utilized to probe into the occurrence of lithium and the control exerted by coal facies in the Longtan Formation of northern Guizhou. The findings revealed that the coal-forming environment of the Longtan Formation was a humid forest swamp with low-living-water and hydrodynamic conditions, which consequently augmented the salinity and reducibility of the sedimentary microfacies. The Sr/Ba ratios in the Longtan Formation fluctuated from 0.40 to 3.39, averaging 1.63, signifying a marine sedimentary milieu. The SCEP outcomes indicated that lithium in the Longtan Formation was predominantly in the silicate-bound form, constituting 71.67%–93.14% (with an average of 87.32%), wherein clay minerals served as the principal carriers of lithium within the coal. A positive correlation was observed between the tissue preservation index and the lithium content (R2 = 0.82), implying that the lithium content in the coals of the Longtan Formation was closely tied to the enrichment of structured plant tissues during the peat swamp accumulation stage. The lithium content primarily exhibited an upward trend with the intensification of the groundwater influence (GWI), suggesting that more vigorous groundwater dynamic conditions could result in a higher lithium content in coal. In comparison to previous investigations, this study represents an advancement by elucidating the enrichment environments of lithium in coal through the comprehensive cross-analysis of both the coal-forming parent materials and sedimentary microfacies. The results hold substantial significance for comprehending the occurrence mechanism and exploration engineering of lithium in coals.
As a distinctive organic rock, coal often becomes enriched with crucial metal elements during the deposition process. These elements include Li, Ga, Ge, U, V, Se, Sc, Re, Zr, H, Nb, Ta, and REY (as reported by Dai et al., 2020a; 2020b; Hao et al., 2023), presenting an indispensable and significant prospect for emerging industries such as new materials, new energy, information technology, aerospace, national defense, and others (Munir et al., 2018; Di et al., 2023). As one of the geological carriers for critical mineral resources, the coal-forming parent materials and sedimentary microfacies are capable of concentrating certain rare elements, potentially leading to deposits with an even higher industrial grade compared to traditional strategic ones (Dai et al., 2012, 2014). Li is among the lightest metal elements in nature, possessing unique physical and chemical characteristics and playing a vital role in fields like new energy vehicles, lithium batteries, high-performance medical devices, biomedicine, and controllable nuclear fusion (Sun et al., 2010; Talan and Huang, 2022). In the past, Li in coal was not regarded as an exploitable metal for an extended period. However, recent research has shown that coal seams can contain Li with a content exceeding the boundary grade (Wang et al., 2008). Consequently, coal has come to be regarded as a promising source for Li.
Northern Guizhou is abundant in coal resources, with a reserve amounting to 241.963 billion tons, which encompasses spinning bituminous coal, anthracite, and lignite. These coal resources are extensively utilized in various industries such as electric power, metallurgy, chemical engineering, building materials, etc., and thus play a crucial part in the local economic and social development. The available exploration data reveal that the lithium content in the Late Permian coal within the Northern Guizhou coalfield is generally higher than 83 μg/g, surpassing the boundary grade of 80 μg/g and even attaining the industrial grade of 120 μg/g in most regions based on National standard GB/T 41042 - 2021 “Guidance for utilization and classification of content of valuable elements in coal.” The occurrence and dispersion of Li are closely associated with the coal-forming paleogeography, paleoenvironment, and coal-forming materials, all of which are determined by the coal facies. Therefore, the distribution of Li is either influenced or even derived from the coal microscopic facies (Jiu et al., 2023; Zhang et al., 2023). The research on the genesis of coal seams can be traced back to the early part of the last century. Stops and Wheeler initiated related research in 1918 (Stach et al., 1982). Subsequently, in the 1950s, Remchuzhnikov first introduced the term “coal facies,” categorizing the accumulation environment of peat into four types: low-lying water swamp facies, forest swamp facies, circulating swamp facies, and swamp lake facies (Teichmiller, 1989). The coal facies analysis is capable of highlighting the composition of coal precursors and the paleoenvironment of peat swamps (Zhang et al., 2010), which is beneficial for understanding the coal formation process and holds significance for the development of coal resources (Petersen and Ratanasthien, 2011).
Typically, there are four crucial parameters available for identifying the coal facies types, namely, petrography, geochemistry, paleobotany, and sedimentology (Zhang et al., 2010). Coal geologists have reached a general agreement that the variation in base level and accommodation space exerts an evident controlling influence on the developmental characteristics of coal facies (Lu et al., 2017). Based on the relationship among swamp type, peat accumulation conditions, and swamp sedimentary environment, Diessel (2010) established coal facies parameters, specifically the gelation index and the structure preservation index, which are correlated with the coal facies and sedimentary environment. This led to the establishment of a microfacies parameter system to elucidate the coal facies and distinguish the sedimentary environment (Diessel and Gammidge, 1998; Calder et al., 1991; Bechtel et al., 2001; 2003; Gruber and Sachsenhofer, 2001; Jiménez et al., 1999). Diessel and Gammidge (1998) suggested that petrographic properties originating from the petrography-paleoenvironment could be focused on lithotypes and microlithotypes. Subsequently, maceral-based facies ratios were introduced and began to be widely employed in facies analysis. A parameter system composed of the tissue preservation index (TPI), gelification index (GI), groundwater influence index (GWI), vegetation index (VI), and structure preservation index (CPI) was established and yielded favorable results (Diessel, 1986; Calder et al., 1991; Jiménez et al., 1999; Bechtel et al., 2001, 2003; Zhang et al., 2010). Nevertheless, there have been ongoing discussions regarding the validity of using maceral distribution to indicate paleodepositional environments (Dehmer, 1995; Moore and Shearer, 2003; Wüst et al., 2001; Scott, 2002). Recent research on the coal-forming paleo-environment has incorporated groundwater level fluctuation parameters, which have demonstrated significant implications for influencing the petrographic properties of coal seams. However, to date, there has been a paucity of reports on the control of coal facies over lithium. The investigation of coal facies in Northern Guizhou holds important theoretical and practical significance for the rational utilization of Li resources and the pursuit of energy conservation and emission reduction goals (Wang J. et al., 2019; Zhou et al., 2021; Sun et al., 2023).
In this present paper, by means of the identification and analysis of the morphological characteristics of macerals, the coal facies as well as the hydrodynamic conditions of the peat swamp were elucidated through the calculation of the facies parameter system. Subsequently, the occurrence of Li was examined using the step-by-step chemical extraction (SCEP) method, inductively coupled plasma mass spectrometry (ICPMS), and scanning electron microscopy (SEM). Eventually, the control of coal facies over the lithium content in the Longtan Formation of northern Guizhou was investigated via correlation analysis. In comparison with previous research efforts, this study represents an advancement as it clarifies the enrichment environments of lithium in coal through the comprehensive cross-analysis of both the coal-forming parent materials and sedimentary microfacies. The results obtained herein are of considerable significance for understanding the occurrence mechanism and exploration engineering of lithium in coals.
The investigation area is structurally positioned within the upper Yangtze block, which belongs to a II-level tectonic unit within the first-level tectonic unit of the Yangtze block. It encompasses two III-level tectonic units, namely, the passive margin fold-thrust belt in the south of the Yangtze block and the Nanpanjiang-Youjiang foreland basin. Moreover, there are three IV-level tectonic units in this area, including the Bijie arc tectonic zone, Liupanshui complex deformation zone, and Zhijin broad fold zone (Figure 1A). Folds are widely developed across this region, which divide the research area into the Baina Syncline, Zhichang Anticline, Zhongba Syncline, and Dadingpo Anticline (Figure 1B).
Figure 1. Location and geological setting of the investigation area. (A)-Location of northern Guizhou [modified from Wang and Yan (2008)]; (B)-Structural outline of northern Guizhou (Ju et al., 2018); (C)-stratigraphic columns of coal-bearing strata (Wang et al., 2024).
The northern Guizhou coalfield is mainly characterized by marine-continental alternating deposits, which consist of mudstone, silty mudstone, and coal seams. The oldest stratum exposed in the coalfield is the Qingshuijiang Formation of the Pre-Sinian system, while the youngest one is the Quaternary. Notably, the Upper Silurian, Devonian, and Lower Permian are absent in this area. The strata that are widely exposed cover the Cambrian, Permian, Triassic, and Jurassic periods. The coal-bearing strata of the Qianbei coalfield incorporate the Xiangbai Formation of the Lower Carboniferous, the Liangshan Formation of the Middle Permian, the Longtan Formation, and the Changxing Formation of the Upper Permian. Among them, the Longtan Formation and the Changxing Formation of the Upper Permian possess the most favorable coal-bearing strata. In other coal-bearing strata, there are no unminable coal seams. The majority of the coal seams are thin ones, with a thickness ranging from 1 to 2 m, and thick coal seams exceeding 3.5 m are rarely found. There are phenomena such as bifurcations and mergers, which give rise to a large number of coal-free zones, and the variations lack regularity (Figure 1C). Due to the absence of typical marker layers, it has hampered the comparison of coal seams. Since the investigation area is situated in western Guizhou, it covers a wide zone and has a complex structure, including the northern Guizhou coalfield, Panxing coalfield, Zhina coalfield, and Liupanshui coalfield.
The samples were mainly obtained from the western region of Guizhou Province. Through meticulous analysis and comparison of previous geological exploration data, mine production records, and relevant research findings, careful consideration was given to various mining areas, coal seams, and coal ranks during the sampling process. Strict compliance was maintained with the stipulations of “Sampling of Coal Seams” (GB/T 428-2008), “Standard Practice for Preparing Coal Samples for Microscopical Analysis by Reflected Light” (ASTM D2797/D2797M-11), and “Standard Test Method for Microscopical Determination of the Vitrinite Reflectance of Coal” (ASTM D2798-11). Samples including coal, parting, roof and floor materials, as well as those from power plants and coal washing plants were collected.
The sampling points within the mines were primarily distributed among the evaluation units of Zhongba-Jinsha, Changchunpu-Maoba, Baixing-Bulang, and Faer-Gemudi. Specifically, within the evaluation units of Zhongba-Jinsha, Changchunpu-Maoba, and Baixing-Bulang, the sampling points were evenly dispersed. In the Faer-Gemudi evaluation unit, however, the sampling points were mostly concentrated and closely arranged along the Shuicheng-Ziyun fault zone. In the Tucheng-Panguan evaluation unit, the sampling points were mainly laid out along the structural unit, though in a relatively small number. In the evaluation units of Dezhuo-Kele, Langdai-Liuzhi, and Qingshan-Qinglong, the sampling points were sparser and more widely scattered (Figure 1B).
A total of 2,548 samples of various types were carefully collected. More specifically, there were 1,373 coal samples. Among them, 1,302 were obtained from mines and wells, 24 were sourced from power plants, and 47 were acquired from coal washing plants. There were 180 parting samples, with 164 being collected from boreholes and mines and 16 from coal washing plants. Additionally, 963 roof and floor samples as well as 32 fly ash samples from power plants were gathered (Figure 2). The coal samples in this paper were collected from the Longtan Formation, which belongs to the coal-bearing deposit of the sea-land interaction. The paleogeographical environment of this coal formation is predominantly the residual plain type, which was formed in the early stage of the Longtan period, on the weathering crust of Maokou limestone. The investigation area is mainly characterized by the residual plain type and the tidal marsh sedimentary system under the influence of transgression. Coal accumulation mainly takes place in the extensive areas of lagoon and tidal flat siltation and shallow swamping. The distribution range of coal seams is wide, mainly consisting of thin and medium-thick coal seams, with high ash and sulfur contents, and there are occasional low-sulfur coals.
The coalfields mainly develop a flower-like delta that features a small scale, a shallow water body, a short development duration, less river input of mud and sand, a sedimentary thickness of approximately 100 m, a fine grain size of debris, and a strong tidal effect. There is no main river channel, and numerous small delta bays are often formed, which constitutes a favorable area for coal accumulation. Here, the ranks of the coal samples mainly include low rank bituminous coal (Ro, max < 1.3), high rank bituminous coal (1.3 < Ro, max < 2.5), and anthracite (Ro, max > 2.5).
In accordance with ASTM standard D6357-19 (2019), the laser ablation inductively coupled plasma mass spectrometer (GeoLas HD Pro, LA-ICP-MS), manufactured by Coherent company in Germany, was employed to determine the trace elements, such as rare earth elements and Y, in the collected samples through the use of an Ar-F gaseous excimer laser. The experimental procedures regarding sample digestion and ICP-MS were referred to the previous reports by Dai et al. (2015a), Dai et al. (2015b). The backscattered images (ABS) and the mineral chemical compositions of the samples were collected by the Thermo Quattro S scanning electron microscope, which was equipped with an EDAX energy spectrum from Czech FEI company. Meanwhile, the microscopic morphological characteristics of the minerals were also investigated. Before observing the samples, the coals were sputtered with gold using a British Quorum Q150T ES sputter coater.
The chemical maps obtained via time-of-flight secondary ion mass spectrometry (TOF-SIMS) analysis can effectively reflect the element distribution, offering valuable information regarding the distribution of trace elements like Li both on the surface and within the particles. The secondary ion image represents the distribution of each element on the surface of the sample. For the element distribution maps, the scale on the right side of each image indicates the ion count, and the ion count represented by the scale increases gradually from bottom to top. When an element becomes enriched at a specific position, a stronger signal intensity will be generated, leading to a larger corresponding ion count.
It should be noted that there is no correlation between two element distribution maps, and the number of ions in these distribution maps is not comparable. Nevertheless, the distribution and enrichment of each element at the same position can still be analyzed relatively. As the number of detected ions increases, the element distribution map undergoes a color change sequence of dark blue-light green-dark green-yellow-light red-red. The red region, where the largest number of ions is detected, represents the element-rich area. Through observing the color variations in each element distribution map, the degree of coincidence in the distribution areas between two elements can be intuitively perceived, and the correlation between the two elements can thus be determined.
The occurrence state of lithium in coal resembles that of most other trace elements in coal, as it can be present in either inorganic minerals or organic matter. In order to quantify the occurrence state of lithium in coal, the SCEP method was adopted for a quantitative analysis. Based on previous extraction methods (Ikeh et al., 2022; Bai et al., 2022), and in combination with multiple experimental results, the optimal extraction step was ultimately selected to conduct the SCEP on the samples from the Northern Guizhou coalfield. The detailed steps are illustrated in Table 1. By applying the six-step sequential chemical extraction protocol, comprehensive information about the six different occurrence characteristics and their corresponding concentrations could be obtained. These include the water-soluble state, ion-exchange state, carbonate-bound state, sulfide-bound state, silicate/aluminosilicate-bound state, and the residual/organic-bound state of lithium in coal. Subsequently, the percentages of trace elements existing in various combined forms relative to the total content of lithium elements in coal were calculated.
The morphological characteristics of the macerals in the coal samples are depicted in Figures 3A–I respectively. The vitrinite group mainly consists of matrix vitrinite, followed by homogeneous vitrinite, with a small amount of structural vitrinite II (characterized by cell cavity deformation, being hollow, or filled with clay), and detrital vitrinite (Figures 3A,F). Specifically, structural vitrinite, homogeneous vitrinite, and matrix vitrinite account for 0.33%–10.12% (with an average of 2.3%), 1.23%–31.22% (with an average of 16.27%), and 6.10%–74.67% (with an average of 45.64%) respectively. Under reflected light, the structural vitrinite appears gray, and its cell wall expands. The homogeneous vitrinite shows a gray reflected light as well. Its composition is uniform without any impurities, and endogenous fissures are developed within it. Moreover, the cell cavity of the homogeneous vitrinite is flattened into a short line, becomes smaller, and is arranged irregularly. Additionally, there is also some detrital vitrinite (ranging from 0% to 51.22%, with an average of 9.10%) in the coal samples collected from this area.
Figure 3. The morphological characters of the macerals in the coal samples. (a) homogenous vitrinite; (b,c) semifusinite in coal; (d) macrinite; (e) oxidized filaments; (f) structural vitrinite; (g) quartz; (h,i) pyrite.
The inertinite group is mainly composed of semi-fusinite, followed by detrital inertinite, and oxidized fusinite can occasionally be spotted under a light microscope. They respectively account for 0%–20.78% (with an average of 3.84%), 0%–13.94% (with an average of 3.35%), and 0%–7.39% (with an average of 2.30%). In addition, small amounts of macrinite (ranging from 0.39% to 4.58%) are developed within these coals. The fusinite appears yellow-white under oil-immersed reflected light, with its high sieve-like cells being well preserved. The semi-fusinite is white, and the plant cell structure within it is poorly preserved (Figures 3B,C). The macrinite maceral belongs to inert components with a particle size greater than 30 m (Figure 3D). It is often irregularly round or blocky and appears bright white or yellowish white under oil-immersed reflected light. The reflected light of the oxidized fusinite is bright white, and its cell structure is well preserved (Figure 3E). The exinite is primarily made up of resinite. The resin bodies are nearly circular, oval, or irregular in shape. They are dark gray under oil-immersed reflected light and light gray under air-reflected light. In the coal of the Longtan Formation, most of the resin bodies are distributed within saprophytic wood bodies and might be derivatives of lipids in plant leaves. Quartz minerals can also be found in some samples, and they usually have a relatively complete crystal structure (Figure 3G). The minerals in the Longtan Formation coals are mainly composed of pyrite, which can occur in the form of strawberry-like or cluster-like aggregate morphologies (Figures 3H,I).
The occurrence state of lithium in coal, along with its six occurrence characteristics (namely, the water-soluble state, ion-exchange state, carbonate-bound state, sulfide-bound state, silicate/aluminosilicate-bound state, and residual/organic-bound state) in coal, can be determined through the application of the six-step sequential chemical extraction method (Zhao et al., 2019; Wang X. et al., 2019). By combining the results of XRD experiments and ICP-MS analysis, the percentages of trace elements in various bound forms relative to the total content of lithium in coal were calculated, thereby enabling the inference of the occurrence carrier of lithium.
37 coal samples collected from the high lithium area were chosen for step-by-step chemical extraction experiments. Based on the results of the SCEP experiment (Figure 4), it was found that lithium in the coal samples was predominantly in the silicate-bound state, accounting for 71.67%–93.14% with an average of 87.32%. The residual/organic fraction came second, accounting for 0.28%–12.63% with an average of 5.81%. The carbonate-bound fraction accounted for 0.21%–25.09% with an average of 5.35%. The remaining occurrence states each accounted for less than 1%. Specifically, the water-soluble state accounted for 0.20%–1.88% with an average of 0.76%. The sulfide-bound fraction accounted for 0.08%–1.90% with an average of 0.48%. The ion exchange state had the lowest proportion, accounting for 0.02%–0.84% with an average of 0.28%. In the gangue sample, the silicate-bound state was also the main occurrence state of lithium, accounting for 92.22%.
Samples with relatively high lithium concentrations within the coal strata of the investigated area were deliberately chosen for micro-regional elemental intensity analysis. In the initial phase, Qinglong M17 (featuring a lithium content of 153 μg/g) and Honglin M16 (with a lithium content of 220 μg/g), both located in the Dadingpo Anticline of the enrichment zone, were identified. The TOF-SIMS technique was purposefully utilized to conduct local ion detection on powdered raw coal specimens. Distribution images of a wide variety of positive secondary ions, including Li, Al, Si, Na, Mg, K, Fe, and Ca, were obtained. Figures 5 and 6 respectively display the elemental distribution maps of the samples Qinglong M17 and Honglin M16 within a precisely defined micro-region measuring 400 μm × 400 μm in the detection domain. In the case of Qinglong M17, the elements Al, Si, and K demonstrated a relatively uniform distribution pattern across the entire region (Figure 5). This indicates that the dominant matrix minerals in this area were clay minerals. Moreover, the spatial distribution of K-rich zones was found to be consistent with that of Al and Si elements, suggesting the potential presence of K-rich illite or illite-smectite mixed layers, which were notably deficient in the Mg element. Simultaneously, in the regions where the Li element appeared black, the Fe element exhibited a pronounced and intense red coloration. Based on the observed lack of coexistence between Li and Fe, it can be convincingly inferred that pyrite did not act as the carrier mineral for Li. Instead, the distribution intensity region of Li was discovered to be in alignment with those of Al, Si, Na, K, Mg, and Ca elements.
Figure 5. TOF-SIMS distribution maps of Li, C, Si, Al, Na, K, Ca, Mg, and Fe in Qinglong M17 sample.
In the case of the Honglin M16 sample, a remarkably strong signal intensity of Li was detected solely within the upper-middle section, which appeared as a vivid bright red color. In the region where Li showed corresponding enrichment, an accumulation of elements such as Al, Si, Na, Mg, and K was noticed (Figure 6). Although elements like Fe and Ca were distributed throughout the Li-enriched zone, their enrichment intensities did not match that of Li, only presenting relatively narrow ranges of elemental intensity. This strongly indicates that the predominant occurrence carrier of Li might potentially be chlorite. In this specific region, the C element was significantly enriched, indicating that the sample was mainly composed of organic matter. Nevertheless, the Li content was only manifested in limited areas where elements like Al and Si were present, suggesting that the organic components did not act as the principal carriers of Li within the coal.
The results described above clearly indicate that the principal occurrence carriers of lithium are aluminosilicates. Furthermore, through XRD experiments, samples with a relatively high clay content were identified. These samples were then subjected to SIMS area scanning analysis in order to verify the different adsorption variances among diverse aluminosilicate minerals, especially clay minerals. The Honglin 35J sample, which had a relatively high lithium content of 255 μg/g, was chosen for the subsequent experiment. Under SEM examination, no obviously extensive and contiguous clay mineral deposits were observed within the bulk sample. The measured lithium ion signal points were found to be dispersed, and a significant difference in lithium distribution was noticed within the region of 100 μm × 100 μm. All of the measured samples demonstrated that the intensity of lithium ions was primarily and closely correlated with the intensities of silicon and aluminum ions, thus suggesting that lithium is predominantly present within aluminosilicate minerals. Clay minerals such as kaolinite, chlorite, and illite are all capable of acting as inorganic mineral carriers for lithium in coal. Subsequently, the planar element high-speed imaging test for lithium and other elements was carried out using the LIBS-LA-ICP-TOFMS technique (Figure 7).
In the regions where the Fe element exhibits a pronounced intensity, the intensities of elements such as Li, Ga, Na, Al, Si, and Ti are nearly imperceptible. This indicates that pyrite does not act as the carrier for these elements (Figure 8). Clay minerals are quite common in coal and are the main carrier minerals for the occurrence of lithium in the coal from western Guizhou in this study. Among the clay minerals in coal, kaolinite is usually the most common. However, in the Late Permian coal in western Guizhou (northern Guizhou), the clay minerals are mainly illite-montmorillonite mixed layers. The authigenic chamosite is relatively common in the Late Permian coal in western Guizhou and is an important component of clay minerals. The clay minerals found in the survey area include: kaolinite, illite, and chamosite. Both kaolinite and illite are common clay minerals in coal. Kaolinite occurs in the form of elliptical aggregates and is distributed along the bedding. This type of kaolinite is usually considered as the characteristic mineral of Tonstein (Figure 8A). A large amount of illite-montmorillonite mixed layers have been found in the Late Permian coal within the study area. These illite-montmorillonite mixed layers either originate from the terrigenous clastic materials formed by the weathering of the Emeishan Basalt or are formed by the precipitation of post-genetic hydrothermal fluids rich in Fe and Mg caused by basic magmatic activities (Figures 8B,C). Chamosite is an oolitic aggregate formed by the marine sedimentation of chlorite. Chlorite is a silicate mineral with a layered structure and is the product of medium- and low-temperature hydrothermal processes, low-grade metamorphism, and sedimentation. Fine quartz particles are distributed in a disseminated pattern within the matrix vitrinite. Sometimes, the authigenic quartz is distributed along the bedding together with clay minerals or pyrite (Figure 8D).
Figure 8. Morphological characteristics of clay minerals and quartz under scanning electron microscope. (a) kaolinite in coal; (b) illite in coal; (c) chlorite; (d) quartz.
Remarkably, only the intensity of the Si element shows a significant contrast within the measured area, suggesting a relatively high quartz content, which is consistent with the mineral characteristics observed under the microscope (Figure 8). Moreover, the elemental signal of Li within the measured micro-region is rather weak, strongly suggesting that quartz in coal is not the hosting medium for Li. Meanwhile, there is a close relationship between Li and Al. The results obtained from the aforementioned SEM, TOF-SIMS analysis, and SCEP are mutually confirmatory. Collectively, they imply that aluminosilicate minerals serve as the primary occurrence carriers for lithium. Based on the comprehensive experimental results derived from sequential chemical extraction, mineral phase identification, correlation analysis, TOF-SIMS, and LIBS elemental imaging, it can be inferred that the main carriers of lithium within the coal are clay minerals. It has been established that the clay minerals present in the coal of the investigated area include kaolinite, chlorite, illite, and illite-smectite mixed layers, with kaolinite being the most abundant.
The percentage composition of different macerals in coals of the Longtan formation was illustrated in Figure 9A. It could be deduced that the macerals in the coals of the Longtan formation were preponderantly composed of vitrinite, trailed by minerals. The contents of both inertinite and exinite were comparatively low (Figure 9A). Subsequently, the parameters of coal facies can be computed based on the composition of different macerals:
where V, T, and TC were the vitrinite group, structural vitrinite, and homogeneous vitrinite, respectively. DC, CC, and VD were matrix vitrinite, mass vitrinite, and detrital vitrinite, respectively. The F, Sf, and Ma depicted the fusinite, semi-filamentous body, and coarse grained, respectively. ID, Sp, Cu, and Re were detrital inertinite, sporopollen, cuticle, and resin body. ID was detrital lipid body.
Figure 9. (A)-The percentage composition of different macerals in coals of Longtan formation, (B)-coal facies of Longtan formation, (C)-peat swamp hydrodynamic conditions of Longtan formation, and (D)-relationship between V/I and F/M in coals of Longtan formation.
The coal facies and peat swamp hydrodynamic conditions of the Longtan formation were respectively presented in Figures 9B,C (Equations 1–3). The majority of the samples were attributed to the low moor facies, while a small number of samples belonged to the wetland herb swamp (Figure 9C), implying that the coal-forming environment had diverse water supply types. The peat swamp hydrodynamic conditions of the Longtan formation were in line with the results of coal facies analysis, attesting to the rationality of the methodology employed herein. The relationship between V/I and F/M in the coals of the Longtan formation was illustrated in Figure 3D, which signified that the coals of the Longtan formation were formed within the living water moist forest swamp (Figure 9D) (Equations 4,5). Consequently, the coal-forming environment of the Longtan formation was a low living water moist forest swamp with specific hydrodynamic conditions.
The stronger the hydrodynamic conditions, the higher the content of active oxygen in the water. The more pronounced the off-site accumulation caused by water migration, the higher the content of inertinite and detritus in the coal, leading to an increased ash yield and a greater proportion of dark coal. The swamp plants in a stranded state typically underwent strong gelation, as manifested by the poor preservation of plant remains in the coal. There was generally a higher content of vitrinite (including homogeneous vitrinite and matrix vitrinite). Under the influence of seawater, the salinity and reducibility of the coal-forming swamp were further augmented, resulting in an elevated sulfur content and an altered ratio of vitrinite to inertinite.
There exist distinct occurrence and migration patterns for different trace elements in coals. Consequently, leveraging the geochemical indices of these elements can furnish valuable information regarding the sediment formation environment. The Sr/Ba ratio serves as a reliable indicator to reflect the salinity variation characteristics of ancient water bodies. Specifically, when the Sr/Ba value exceeds 1, it points to a marine saltwater sedimentary environment; when it is less than 0.6, it signals a freshwater sedimentary environment of continental facies; and when it falls between 0.6 and 1, it denotes a brackish water sedimentary environment of transitional facies.
The geochemical index of seawater paleosalinity for the coals of the peat swamp paleosalinity was illustrated in Figure 10. In the Longtan formation, the Sr/Ba value ranged from 0.40 to 3.39, with an average of 1.63, suggesting a predominantly marine sedimentary environment. Notably, sample 26 was influenced by the marine-continental transitional facies sedimentary environment, while sample 43 originated from the continental sedimentary environment. The outcomes related to the peat swamp paleosalinity were in harmony with the preceding analysis of the coal facies of the Longtan formation.
The influences of the coal facies on the Li content in coals of the Longtan Formation were respectively presented in Figures 11A–D. A positive correlation exists between the tissue preservation index and the Li content, with a correlation coefficient of 0.82 (Figure 11A). Given that a higher TPI implies a greater ratio of structured plant tissue to unstructured fine plant debris, this positive relationship indicates that the Li content of coals in the Longtan Formation was tightly linked to the enrichment of structured plant tissue during the peat swamp accumulation period. The results herein perhaps suggest that the high Li content in these coals was induced by the effects of woody plants.Figure 11B illustrates the relationship between the vegetation index and the Li content, and there is a relatively satisfactory linear relationship with a correlation coefficient of 0.57, implying that a higher degree of plant destruction during the coal formation process could lead to a higher Li content.
Figure 11. The influences of the coal facies on the Li content in coals of Longtan formation. (a) relationship between Li content and TPI; (b) relationship between Li content and VI; (c) relationship between Li content and GWI; (d) relationship between Li content and V/I.
The relationship between the groundwater influence index and the Li content is shown in Figure 11C. It can be deduced that the Li content primarily rises with the increasing GWI, suggesting that stronger groundwater dynamic conditions could result in a higher Li content in coals of the Longtan Formation. There is also a good linear relationship between the ratio of vitrinite to inertinite (V/I) and the Li content of coals in the Longtan Formation, with a correlation coefficient of 0.78 (Figure 11D). Since a V/I ratio higher than 4 indicates a moist forest swamp, this good linear relationship verifies that the enrichment of Li was inclined to occur in the moist forest swamp environment.
The parameter V/I represents the ratio of vitrinite to inertinite content in coal and reflects the redox conditions of coal-forming swamps. Generally, a wet reduction environment favors the formation of vitrinite, whereas a dry oxidation environment promotes the formation of inertinite. In coal-forming swamps, the depth of overlying water significantly impacts the redox properties of peat swamps. Specifically, the larger the V/I value, the deeper the water depth of the coal-forming environment and the more prone the water body is to reduction conditions. The V/I values of all coal samples were greater than 1, indicating a deep water environment and corresponding reduction redox conditions. Lithium is one of the most active elements among metals, with relatively active chemical properties. In an oxidizing environment, Li is readily oxidized to form soluble Li-containing oxides or lithium salts.
The correlation between the coal sample parameter F/M value and the lithium element in the coal was found to be positive. The F/M parameter reflects the strength of the hydrodynamic force. That is, the larger the F/M value, the weaker the hydrodynamic force in the corresponding coal-forming environment at that time, and potentially, the higher the lithium content in the coal. Conversely, the correlation between the GWI value of the coal sample parameter and the lithium element in the coal exhibited a relatively weak negative correlation. The GWI value also mirrors the magnitude of the hydrodynamic strength. Namely, the larger the GWI value, the stronger the hydrodynamic force of the coal-forming environment at that time, which should lead to a lower lithium content in the coal.
In this paper, based on the identification and analysis of the morphological characteristics of the macerals, both the coal facies and the peat swamp hydrodynamic conditions were elucidated by calculating the facies parameter system. Subsequently, the occurrence of Li was examined through the SCEP method, inductively coupled plasma mass spectrometry (ICPMS), and scanning electron microscopy (SEM). Finally, the control of coal facies on the lithium content in the Longtan Formation of northern Guizhou was investigated using correlation analysis.
1) The SCEP results indicated that Li in coal was predominantly in the silicate-bound state, accounting for 71.67%–93.14% with an average of 87.32%, followed by the residual/organic fraction. In light of the comprehensive experimental outcomes obtained from sequential chemical extraction, mineral phase identification, correlation analysis, TOF-SIMS, and LIBS elemental imaging, it can be inferred that the principal carriers of lithium within the coal are clay minerals, among which kaolinite shows the highest abundance. The macerals in coals of the Longtan formation were preponderantly composed of vitrinite, trailed by minerals. The contents of both inertinite and exinite were comparatively low, furnishing the foundation for clarifying the coal facies. Coal facies analysis suggested that the coal-forming environment of the Longtan formation was a low living water moist forest swamp with specific hydrodynamic conditions. The swamp plants in a stranded state typically underwent strong gelation, as manifested by the poor preservation of plant remains in the coal.
2) Correlation analysis was carried out to clarify the control of coal facies on Li enrichment. The results revealed a positive relationship between the tissue preservation index and the Li content, with a correlation coefficient as high as 0.82. This implies that the Li content of coals in the Longtan formation was closely tied to the enrichment of structured plant tissue during the peat swamp accumulation period. It was also found that the Li content primarily rises with the increasing GWI, signifying that stronger groundwater dynamic conditions could lead to a higher Li content in coals of the Longtan formation. Additionally, a V/I ratio higher than 4 indicated the moist forest swamp, corroborating that the enrichment of Li was inclined to occur in such an environment. Compared with previous studies, this work represents an advancement by elucidating the enrichment environments of lithium in coal through the cross-analysis of both the coal-forming parent materials and sedimentary microfacies.
The original contributions presented in the study are included in the article/supplementary material, further inquiries can be directed to the corresponding authors.
XM: Writing–original draft, Writing–review & editing, Conceptualization, Investigation. SY: Writing–review & editing, Writing–original draft. WM: Writing–review & editing, Conceptualization, Investigation, Software. FG: Formal Analysis, Project administration, Validation, Writing–review & editing, Data curation, Funding acquisition, Resources. PZ: Writing–review & editing, Formal Analysis, Project administration, Supervision, Validation.
The author(s) declare that financial support was received for the research and/or publication of this article. The work is supported by the National Natural Science Foundation of China (No. 42472224, 42102210) and National Key R&D Program of China (Grant number: 22024YFC2909400).
The authors declare that the research was conducted in the absence of any commercial or financial relationships that could be construed as a potential conflict of interest.
The author(s) declare that no Generative AI was used in the creation of this manuscript.
All claims expressed in this article are solely those of the authors and do not necessarily represent those of their affiliated organizations, or those of the publisher, the editors and the reviewers. Any product that may be evaluated in this article, or claim that may be made by its manufacturer, is not guaranteed or endorsed by the publisher.
ASTM International (2019). Standard Test Methods for Determination of Trace Elements in Coal, Coke, and Combustion Residues from Coal Utilization Processes by Inductively Coupled Plasma Atomic Emission Spectrometry, Inductively Coupled Plasma Mass Spectrometry, and Graphite Fur. West Conshohocken, PA: ASTM International.
Bai, G., Su, J., Li, X., Guo, C., Han, M., Zhou, X., et al. (2022). Step-by-step CO2 injection pressure for enhanced coal seam gas recovery: A laboratory study. Energy 260, 125197. doi:10.1016/j.energy.2022.125197
Bechtel, A., Gruber, W., Sachsenhofer, R. F., Gratzer, R., Lücke, A., and Püttmann, W. (2003). Depositional environment of the Late Miocene Hausruck lignite (Alpine Foreland Basin): insights from petrography, organic geochemistry, and stable carbon isotopes. Int. J. Coal Geol. 53, 153–180. doi:10.1016/s0166-5162(02)00194-5
Bechtel, A., Gruber, W., Sachsenhofer, R. F., Gratzer, R., and Püttmann, W. (2001). Organic geochemical and stable carbon isotopic investigation of coals formed in low-lying and raisedmireswithin the Eastern Alps (Austria). Org. Geochem. 32, 1289–1310. doi:10.1016/s0146-6380(01)00101-2
Calder, J. H., Gibling, M. R., and Mukhopadhyay, P. K. (1991). Peat formation in a Westphalian B piedmont setting, Cumberland Basin, Nova Scotia, Nova Scotia: implications for the maceral-based interpretation of rheotrophic and raised paleomires. Bull. la Société Géologique Fr. 162 (2), 283–298.
Dai, S., Hower, J. C., Finkelman, R. B., Graham, I. T., French, D., Ward, C. R., et al. (2020b). Organic associations of non-mineral elements in coal: A review. Int. J. Coal Geol. 218, 103347. doi:10.1016/j.coal.2019.103347
Dai, S., Liu, J., Ward, C. R., Hower, J. C., Xie, P., Jiang, Y., et al. (2015a). Petrological, geochemical, and mineralogical compositions of the low-Ge coals from the Shengli Coalfield, China: A comparative study with Ge-rich coals and a formation model for coal-hosted Ge ore deposit. Ore Geol. Rev. 71, 318–349. doi:10.1016/j.oregeorev.2015.06.013
Dai, S., Ren, D., Chou, C. L., Finkelman, R. B., Seredin, V. V., and Zhou, Y. (2012). Geochemistry of trace elements in Chinese coals: A review of abundances, genetic types, impacts on human health, and industrial utilization. Int. J. Coal Geol. 94, 3–21. doi:10.1016/j.coal.2011.02.003
Dai, S., Seredin, V. V., Ward, C. R., Jiang, J., Hower, J. C., Song, X., et al. (2014). Composition and modes of occurrence of minerals and elements in coal combustion products derived from high-Ge coals. Int. J. Coal Geol. 121, 79–97. doi:10.1016/j.coal.2013.11.004
Dai, S., Wang, P., Ward, C. R., Tang, Y., Song, X., Jiang, J., et al. (2015b). Elemental and mineralogical anomalies in the coal-hosted Ge ore deposit of Lincang, Yunnan, southwestern China: Key role of N2-CO2-mixed hydrothermal solutions. Int. J. Coal Geol. 152, 19–46. doi:10.1016/j.coal.2014.11.006
Dai, S., Yan, X., Ward, C. R., Hower, J. C., Zhao, L., Wang, X., et al. (2020a). Valuable elements in Chinese coals: A review. Coal Geol. China, 60–90. doi:10.4324/9780429449369-5
Dehmer, J. (1995). Petrological and organic geochemical investigation of recent peats with known environments of deposition. Int. J. Coal Geol. 28, 111–138. doi:10.1016/0166-5162(95)00016-x
Di, S., Dai, S., Nechaev Victor, P., French, D., Graham, I. T., Zhao, L., et al. (2023). Mineralogy and enrichment of critical elements (Li and Nb-Ta-Zr-Hf-Ga) in the Pennsylvanian coals from the Antaibao Surface Mine, Shanxi Province, China: derivation of pyroclastics and sediment-source regions. Int. J. Coal Geol. 273, 104262. doi:10.1016/j.coal.2023.104262
Diessel, C. F. K. (1986). On the correlation between coal facies and depositional environments. Advances in the study of the Sydney Basin. Process 20th Newctle. Symposium, 19–22.
Diessel, C. F. K. (2010). The stratigraphic distribution of inertinite. Int. J. Coal Geol. 81 (4), 251–268. doi:10.1016/j.coal.2009.04.004
Diessel, C. F. K., and Gammidge, L. (1998). Isometamorphic variations in the reflectance and fluorescence of vitrinite: a key to depositional environment. Int. J. Coal Geol. 36, 167–222. doi:10.1016/s0166-5162(98)00003-2
Gruber, W., and Sachsenhofer, R. F. (2001). Coal deposition in the Noric Depression (Eastern Alps): raised and low-lying mires in Miocene pull-apart basins. Int. J. Coal Geol. 48, 89–114. doi:10.1016/s0166-5162(01)00049-0
Hao, H., Li, J., Wang, J., Liu, Y., and Sun, Y. (2023). Distribution characteristics and enrichment model of valuable elements in coal: An example from the Nangou Mine, Ningwu Coalfield, Northern China. Ore Geol. Rev. 160, 105599. doi:10.1016/j.oregeorev.2023.105599
Ikeh, T. J., Sun, B., Liu, C., Liu, Y., Kong, Y., and Pan, X. (2022). Modes of occurrence and enrichment of trace elements in coal from the Anjialing mine, Pingshuo mining district, Ningwu coalfield, Shanxi Province, China. Minerals 12 (9), 1082. doi:10.3390/min12091082
Jiménez, A., Martinez-Tarazona, R., and Suárez-Ruiz, I. (1999). Paleoenvironmental conditions of Puertollano coals (Spain): petrological and geochemical study. Int. J. Coal Geol. 41, 189–211. doi:10.1016/s0166-5162(99)00016-6
Jiu, B., Huang, W., Spiro, B., Hao, R., Mu, N., Wen, L., et al. (2023). Distribution of Li, Ga, Nb, and REEs in coal as determined by LA-ICP-MS imaging: a case study from Jungar coalfield, Ordos Basin, China. Int. J. Coal Geol. 267, 104184. doi:10.1016/j.coal.2023.104184
Ju, W., Yang, Z. B., Qin, Y., Yi, T. S., and Zhang, Z. G. (2018). Characteristics of in-situ stress state and prediction of the permeability in the Upper Permian coalbed methane reservoir, western Guizhou region. SW China. J. Petrol Sci. Eng. 165, 199–211. doi:10.1016/j.petrol.2018.02.037
Lu, J., Shao, L., Yang, M., Zhou, K., Wheeley, J. R., Wang, H., et al. (2017). Depositional model for peat swamp and coal facies evolution using sedimentology, coal macerals, geochemistry and sequence stratigraphy. J. Earth Sci. 28, 1163–1177. doi:10.1007/s12583-016-0942-7
Moore, T., and Shearer, J. (2003). Peat/coal type and depositional environment-are they related? Int. J. Coal Geol. 56, 233–252. doi:10.1016/s0166-5162(03)00114-9
Munir, M. A. M., Liu, G., Yousaf, B., Ali, M. U., Abbas, Q., and Ullah, H. (2018). Enrichment of Bi-Be-Mo-Cd-Pb-Nb-Ga, REEs and Y in the permian coals of the huainan coalfield, anhui, China. Ore Geol. Rev. 95, 431–455. doi:10.1016/j.oregeorev.2018.02.037
Petersen, H. I., and Ratanasthien, B. (2011). Coal facies in a Cenozoic paralic lignite bed, Krabi Basin, southern Thailand: Changing peat-forming conditions related to relative sea-level controlled watertable variations. Int. J. Coal Geol. 87 (1), 2–12. doi:10.1016/j.coal.2011.04.004
Scott, A. (2002). Coal petrology and the origin of coal macerals-a way ahead? Int. J. Coal Geol. 50, 119–134. doi:10.1016/s0166-5162(02)00116-7
Stach, E. M., Mackowsky, M. T., Teichmüller, M., Taylor, G. H., Chandra, D., and Teichmüller, R. (1982). Stach's textbook of coal petrology. Berlin: Gebruder Borntraeger, 535.
Sun, B., Guo, Z., Liu, C., Kong, Y., French, D., and Zhu, Z. (2023). Lithium isotopic composition of two high-lithium coals and their fractions with different lithium occurrence modes, Shanxi Province, China. Int. J. Coal Geol. 277, 104338. doi:10.1016/j.coal.2023.104338
Sun, Y., Li, Y., Zhao, C., Lin, Y., Wang, J., and Qin, S. (2010). Concentrations of lithium in Chinese coals. Energy Explor. Exploit. 28 (2), 97–104. doi:10.1260/0144-5987.28.2.97
Talan, D., and Huang, Q. (2022). A review study of rare Earth, Cobalt, Lithium, and Manganese in Coal-based sources and process development for their recovery. Miner. Eng. 189, 107897. doi:10.1016/j.mineng.2022.107897
Teichmiller, M. (1989). The genesis of coal from the viewpoint of coal petrology. Int. J. Coal Geol. 12 (12), 1–87. doi:10.1016/0166-5162(89)90047-5
Wang, J., Yamada, O., Nakazato, T., Zhang, Z., Suzuki, Y., and Sakanishi, Y. (2008). Statistical analysis of the concentrations of trace elements in a wide diversity of coals and its implications for understanding elemental modes of occurrence. Fuel 87 (10-11), 2211–2222. doi:10.1016/j.fuel.2007.10.012
Wang, J., Yang, Z., Qin, S., Panchal, B., Sun, Y., and Niu, H. (2019). Distribution characteristics and migration patterns of hazardous trace elements in coal combustion products of power plants. Fuel 258, 116062. doi:10.1016/j.fuel.2019.116062
Wang, Q., and Yan, R. D. (2008). Study on REEs as tracers for late permian coal measures in Bijie City, Guizhou Province. China. J. Rare Earth 26 (1), 121–126.
Wang, X., Wang, X., Pan, Z., Pan, W., Yin, X., Chai, P., et al. (2019). Mineralogical and geochemical characteristics of the Permian coal from the Qinshui Basin, northern China, with emphasis on lithium enrichment. Int. J. Coal Geol. 214, 103254. doi:10.1016/j.coal.2019.103254
Wang, Y., Huang, M., Zhu, Y., Qin, Y., and ShiMineralogy, Y. D. K. (2024). Mineralogy, geochemistry and enrichment mechanism of critical elements (Li and Zr-Nb-Hf-Ta-REY) in the coals from western Guizhou Province, China: Source and sedimentary environment. Ore Geol. Rev. 176, 106402. doi:10.1016/j.oregeorev.2024.106402
Wüst, R., Hawke, M., and Bustin, M. (2001). Comparing maceral ratios from tropical peatlands with assumptions from coal studies: do classic coal petrographic interpretation methods have to be disgarded? Int. J. Coal Geol. 48, 115–132. doi:10.1016/s0166-5162(01)00050-7
Zhang, S., Tang, S., Tang, D., Pan, Z., and Yang, F. (2010). The characteristics of coal reservoir pores and coal facies in Liulin district, Hedong coal field of China. Int. J. Coal Geol. 81 (2), 117–127. doi:10.1016/j.coal.2009.11.007
Zhang, S., Xiu, W., Sun, B., and Liu, Q. (2023). Provenance of multi-stage volcanic ash recorded in the Late Carboniferous coal in the Jungar Coalfield, North China, and their contribution to the enrichment of critical metals in the coal. Int. J. Coal Geol. 273, 104265. doi:10.1016/j.coal.2023.104265
Zhao, S., Pudasainee, D., Duan, Y., Gupta, R., Liu, M., and Lu, J. (2019). A review on mercury in coal combustion process: Content and occurrence forms in coal, transformation, sampling methods, emission and control technologies. Prog. Energy Combust. Sci. 73, 26–64. doi:10.1016/j.pecs.2019.02.001
Zhou, M., Zhao, L., Wang, X., Nechaev, V. P., French, D., Spiro, B. F., et al. (2021). Mineralogy and geochemistry of the Late Triassic coal from the Caotang mine, northeastern Sichuan Basin, China, with emphasis on the enrichment of the critical element lithium. Ore Geol. Rev. 139, 104582. doi:10.1016/j.oregeorev.2021.104582
Keywords: coal facies, Lithium, peat swamp paleosalinity, groundwater dynamic conditions, Longtan formation
Citation: Xiwei M, Yu S, Meng W, Guangjun F and Peiming Z (2025) Lithium occurrence and coal facies control of Longtan formation in Northern Guizhou, China. Front. Earth Sci. 13:1570304. doi: 10.3389/feart.2025.1570304
Received: 03 February 2025; Accepted: 07 March 2025;
Published: 02 April 2025.
Edited by:
Hongjian Zhu, Yanshan University, ChinaReviewed by:
Iuliu Bobos, Faculty of Sciences, University of Porto, PortugalCopyright © 2025 Xiwei, Yu, Meng, Guangjun and Peiming. This is an open-access article distributed under the terms of the Creative Commons Attribution License (CC BY). The use, distribution or reproduction in other forums is permitted, provided the original author(s) and the copyright owner(s) are credited and that the original publication in this journal is cited, in accordance with accepted academic practice. No use, distribution or reproduction is permitted which does not comply with these terms.
*Correspondence: Song Yu, c29uZ3l1MTAwOTQ0ODhAMTI2LmNvbQ==; Zhou Peiming, MTA1MzI5NDEwN0BxcS5jb20=
Disclaimer: All claims expressed in this article are solely those of the authors and do not necessarily represent those of their affiliated organizations, or those of the publisher, the editors and the reviewers. Any product that may be evaluated in this article or claim that may be made by its manufacturer is not guaranteed or endorsed by the publisher.
Research integrity at Frontiers
Learn more about the work of our research integrity team to safeguard the quality of each article we publish.