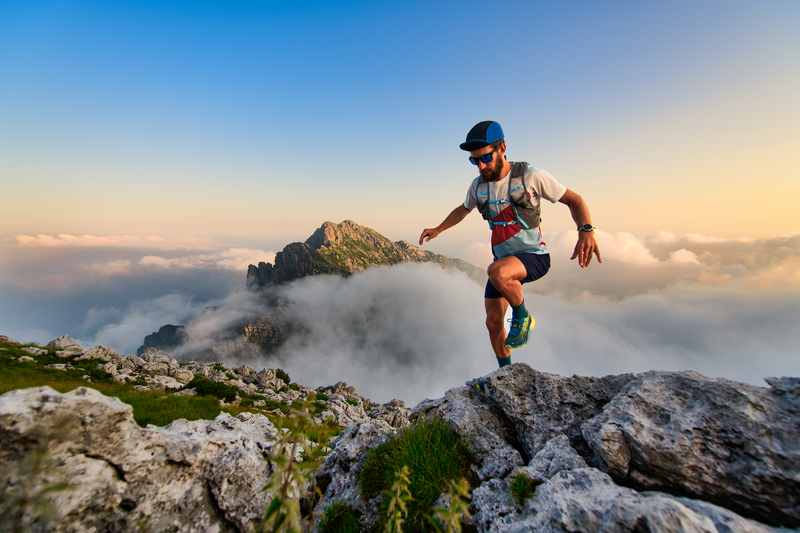
95% of researchers rate our articles as excellent or good
Learn more about the work of our research integrity team to safeguard the quality of each article we publish.
Find out more
REVIEW article
Front. Earth Sci. , 19 March 2025
Sec. Solid Earth Geophysics
Volume 13 - 2025 | https://doi.org/10.3389/feart.2025.1569128
This article is part of the Research Topic When The Earth Records Cycles View all articles
Cyclic fluctuations in the frequency and intensity of volcanic activity are recorded during periods of global climate change. Volcano-sedimentary successions (e.g., in near-coastal environments) may reveal the interplay of glacio-eustatic fluctuations, controlling erosional vs. aggradational processes, and the pattern of volcanic activity. However, the idea of a causal link between Earth’s climate and volcanism is still debated, also because many prior studies have focused on a single glacial cycle. The strongest evidence for a connection between orbitally driven climate variations and volcanism lies in the observed periodicity of volcanic activity on a time-scale of 103–104 years parallel to glacial-interglacial climate fluctuations. This has suggested that volcanism may be influenced indirectly by Earth’s orbital factors, through their effects on climate and the resulting changes in the distribution of continental ice and seawater masses. The hypothesis of a glacio-eustatic control specifically connects Milankovitch cycles—such as the 100,000-year eccentricity cycle, and the 41,000-year obliquity cycle—to the frequency and intensity patterns of volcanic eruptions, as a result of crustal stress changes driven by the redistribution of ice masses and sea level fluctuations. The alternative hypothesis suggests a direct gravitational effect on the crustal stress field driven by orbital oscillations in Earth’s inclination and rotation. This would result into periodic intensifications of volcanic activity and related greenhouse gas emission, thus in turn influencing the intensity of Milankovitch periodicities on a global scale. Here, we present an overview of the ongoing debate on the cause-and-effect relationships of Earth’s orbital factors, periodic climate changes and volcanism. On these grounds, we point out possible research perspectives.
Volcanism is a complex geodynamic process influenced by a variety of internal and external factors. While the primary drivers of volcanic activity stem from magmatic and tectonic processes, the role of external influences, such as climate variations, glacial cycles, and gravitational forcing due to tidal and Earth’s orbital factors has gained increasing attention in recent years (Cooper et al., 2018; Dumont et al., 2022a; Dumont et al., 2022b; Lambert and Sottili, 2019; Le Mouël et al., 2023; Ma et al., 2024; Schindlbeck et al., 2018; Sottili et al., 2021; Wang and Lin, 2015). The key question that we address in this review is: do Milankovitch cycles modulate global volcanic activity? And, if so, is volcanism tuned by orbitally driven climate variations, or directly by the gravitational effects of orbital factors? This question underscores the need for a multidisciplinary approach to understanding the possible connections of Earth’s long-term climate dynamics and/or gravitational-orbital factors to volcanic processes. Here, we explore the current state of knowledge on the intersection of Milankovitch cycles and global volcanism, examining the theoretical foundations, empirical data from different geodynamic contexts, and potential feedback mechanisms.
Volcano-sedimentary successions in near-coastal environments may reveal the interplay of sea level fluctuations, controlling erosional vs. aggradational processes, and the patterns of tectonic and volcanic activities. Integrated geo-volcanological, geochronological and environmental research, also in light of Unconformity-Bounded Stratigraphic Units (UBSU) stratigraphy (Salvador, 1987; Salvador, 1994; Palladino et al., 2010) and tephrochronology (e.g., Monaco et al., 2022a; Monaco et al., 2022b; Bulian et al., 2024) approaches, puts temporal constraints for the reconstruction of the morpho-climatic evolution, i.e., temporal changes in the landscape (morphology) and climate, on regional and ultra-regional scale, and sets the groundwork for assessing possible mutual cause-and-effect interactions among these diverse processes.
Previous studies demonstrate a multifaceted relationship among volcanic activity, environmental factors, such as glacial-interglacial cycles, sea-level fluctuations, and climate events, and Earth’s orbital factors over various timescales. Since the seventies, these studies highlight the intricate interactions among tectonic processes, volcanic eruptions, and Earth’s climate, emphasizing the significant roles played by astronomical cycles and surface load variations in shaping climate dynamics and volcanic activity (e.g., Grove 1974; Kennett and Thunell, 1975; Hall, 1982). The diverse factors explored up to now include tidal stresses, glacial unloading, sea level changes, celestial alignments, and climate over different time scales. Then, we underscore the need for further investigations to decipher the mechanisms of interactions among volcanism, Earth’s climate, and gravitational-orbital effects (gravitational-orbital forcing).
Volcanic activity has long been recognized as a major player in shaping Earth’s climate. Clusters of large eruptions can inject vast quantities of aerosols and gases, such as sulphur dioxide, into the stratosphere, where they can cause abrupt, severe, and long-lasting cooling by reflecting sunlight away from the Earth (Bray, 1976; Miller et al., 2012; Prueher and Rea, 1998). Over longer timescales, volcanic activity can release carbon dioxide, contributing to the greenhouse effect and potentially driving warming trends (e.g., Huybers and Langmuir, 2009).
The relationship between volcanic activity and climate is bidirectional, however. While volcanic eruptions can influence climate, it is plausible that changes in Earth’s climate, particularly those driven by Milankovitch cycles, might in turn influence volcanic activity (Aubry et al., 2022). Orbital changes, which modulate the Earth’s temperature, ice cover, and sea levels, also imply gravitational effects that may affect tectonic stresses, mantle dynamics, and magma production, all of which play a key role in influencing volcanic processes.
The Earth’s climate has experienced significant variability across geological timescales, including changes in temperature, precipitation, and atmospheric composition. These climate dynamics are largely governed by Earth’s orbital factors, under the gravitational influence of the Sun, Moon, and other celestial bodies. The Milankovitch cycles, named after the Serbian mathematician Milutin Milankovitch, refer to periodic variations in Earth’s orbit and axial tilt that influence the planet’s climate system (Milankovitch, 1941). These cycles, which span thousands to hundreds of thousands of years, are modulated by three primary periodicities: eccentricity, obliquity, and precession.
• Eccentricity refers to the shape of the Earth’s orbit around the Sun, ranging from nearly circular to a variably flattened ellipse. Eccentricity has two main periodicities, i.e., of ∼100,000 years on average, and ∼413,000 years. Changes in eccentricity affect the distance between Earth and Sun, which, in turn, alters the amount of solar radiation received by different parts of the planet.
• Obliquity is the tilt of Earth’s axis relative to its orbital plane. This axial tilt varies between 22.1° and 24.5° on a 41,000-year cycle. Changes in obliquity influence the intensity of seasonal climate variations, with a greater tilt resulting in more pronounced seasonal changes.
• Precession is the wobble of Earth’s axis, which makes the timing of seasons to shift over a 26,000-year period, with effects on the distribution of solar energy across the globe that controls the timing and intensity of seasonal contrasts.
The interplay of these three cycles, which produces a complex, long-term pattern of climate variability, is often referred to as orbital forcing. Specifically, at low latitudes, the solar radiation is primarily influenced by the combined effects of eccentricity and precession, while, at higher latitudes, it is mainly controlled by changes in obliquity. In fact, the Earth’s tilt makes more energy to be received at the equator than at the poles, as solar energy at higher latitudes travels through the atmosphere at a shallower angle and is spread over a wider area; thus, an increased tilt amplifies the seasonal differences, while a decreased tilt reduces them.
The most well-known example of climate change driven by Milankovitch cycles is the occurrence of glacial-interglacial cycles during the Pleistocene Epoch, implying the advance and retreat of ice sheets, shifts in sea level, and alterations in the global carbon cycle. Although the Milankovitch theory of climate accounts for several important features of paleoclimate data -especially the frequency and phase distribution of ice sheet cycles influenced by orbital variations-it still faces significant unresolved issues: 1) the large amplitude of the ∼100,000-year ice age cycles, 2) the shift from 41,000-year to 100,000-year glacial cycles around 0.9 million years ago, 3) the absence of notable spectral power at 413,000 years in the past 1.2 million years, 4) changes in the duration of glacial cycles over the last 800,000 years, and 5) the presence of non-orbital spectral peaks in the climate record.
In particular, a persistent and somewhat enigmatic “100,000-year problem” refers to the fact that the Earth’s climate has oscillated with a dominant 100,000-year periodicity during the late Pleistocene, when ice ages and interglacial periods alternated. In fact, the Milankovitch theory fails to fully explain this 100,000-year cycle, subject of ongoing debate and research in the field of paleoclimatology (Lisiecki and Raymo 2005; Watanabe et al., 2023). Berger and Loutre (1991), in light of modelling studies, provide an explanation for the 100,000-year cycle based on the interaction between eccentricity and precession, which influences the build-up and melting of ice sheets. Another hypothesis is based on the relevance of the Northern Hemisphere in driving glacial-interglacial cycles, as the bulk of Earth’s landmasses, particularly the large continents of North America and Eurasia, lie in the Northern Hemisphere. As a result, even small changes in solar radiation during key times of the year can have a significant impact on the growth and decay of ice sheets (Abe-Ouchi et al., 2013). The interaction between eccentricity and precession can lead to a greater amount of solar radiation during the Northern Hemisphere summer at high latitudes when eccentricity is high, further intensifying the melting of ice sheets (Hays et al., 1976).
Recent studies suggest that the increase in atmospheric CO2 during interglacials may be driven in part by the melting of ice sheets, which releases the carbon stored in ice, as well as by changes in ocean circulation and biological processes (Archer et al., 2000). The feedback between orbital forcing, ice sheet dynamics, and CO2 levels may concur to amplify the 100,000-year climate cycle. Another theory hypothesizes that the 100,000-year cycle is driven by changes in the Earth’s internal dynamics, such as mantle convection and volcanic activity. These processes could influence the amount of CO2 released into the atmosphere and interact with orbital forcing to amplify the climate cycles (Ruddiman, 2003).
As additional data and advanced analytical techniques become available, our understanding of the frequency and phase distribution of glacio-eustatic cycles, controlled by orbital variations, is improved, thus casting new light on the interactions between volcanism and climate on Earth. However, we will explore in the following sections some unresolved issues of the Milankovitch theory of climate, which reflect in our ability to interpret the complex relationship between volcanism and Milankovitch cycles, which in turn involves the complex interactions between geodynamic processes and climate factors. In the following two sections we address the possible response of volcanic activity to climate factors responsible of major changes in cryosphere (e.g., ice distribution on the Planet) and hydrosphere (e.g., sea level changes).
The cryospheric environment, which is dominated by glacial ice but also includes permafrost and annual snow pack, is thought to effect volcanism in different ways: by influencing the variety of eruption styles (both explosive and effusive) within a single eruption cycle, by generating unique morphologies and deposits at the scale of a volcanic edifice, and by modulating the timing and rate of magmatic activity (Edwards et al., 2022). Here, we introduce the concept of decompression melting induced by glacial unloading, which has been extensively studied in volcanic regions like Iceland (Sigmundsson et al., 2010; Eksinchol et al., 2019; Van Vliet-Lanoë et al., 2020), where the retreat of glaciers may significantly impact volcanic activity. The model by Sternai et al. (2016) also attributes to glacial erosion a significant role (comparable to ice melting) on continental unloading, providing new insights into the relationship between glacial processes and melting of the solid Earth. The decompression due to a reduction of the weight of ice on the Earth’s crust may enhance the degree of partial melting in the underlying mantle, with effects on magma chemical and isotopic characteristics (Harðarson and Fitton, 1991; Gee et al., 1998; Schmidt et al., 2013). This process, termed “glacial unloading” has been linked to increased volcanic activity in post-glacial periods (Sigvaldason et al., 1992; Slater et al., 1998). Indeed, the rapid deglaciation following the last Ice Age triggered a sharp increase in volcanic activity, with eruption rates 20 to 30 times higher than today. Jull and McKenzie (1996) suggest that the rapid removal of a thick ice sheet significantly increases the rate of melt production in the mantle, leading to a dramatic surge in volcanic eruptions, i.e., eruption rate up to 30 times higher than normal, the magnitude of the volcanic surge being correlated to the speed of deglaciation. Jull and McKenzie (1996) also modelled the nonlinear effects of glacial unloading, showing that during rapid deglaciation (spanning roughly 1,000 years), a massive increase in melt generation beneath Iceland produced approximately 3,100 km3 of magma. Notably, the melt generated during deglaciation periods slightly differs in chemical signature compared to the steady-state melt, particularly in terms of light rare earth element concentrations, due to the different degrees of partial melting (Schmidt et al., 2013). These findings reinforce the idea that glacial unloading, by reducing pressure on the lithosphere, may play a significant role on volcanism, not only in terms of enhanced magma production in the mantle, and consequent eruptive activity, but also influencing the composition of the primary magma.
Also, the glacial-volcanic feedback mechanism highlights the importance of understanding the physical changes in the Earth’s surface during glaciation and their effects on volcanic dynamics. Hooper et al. (2011) discuss how the retreat of ice caps in Iceland may instead enhance the capture of magma within the crust. In particular, their study of the Kverkfjöll volcanic system shows that stress changes resulting from the ice cap retreat can promote magma storage, rather than eruption, at least in the short term. These studies demonstrate that the relationship between glaciers and volcanism is dynamic, with glacial unloading both influencing magma storage and potentially promoting eruptions. Through a survey of postglacial volcanic activity in Iceland, MacLennan et al. (2002) notice a substantial increase in eruption rates, and changes in lava composition, immediately following deglaciation around 12 kyr BP. They explain the observed eruption rates, up to 100 times higher than in the glacial period and in recent times, as the consequence of the unloading of ice sheets, leading to increased degree of mantle melting. Estimates of melt transport rates in the mantle indicate melt ascent velocities in excess of 50 m/year.
The impact of glacial unloading on volcanic systems is not limited to their mantle magma sources. In addition, a change in the stress state of the crust induced by glacial unloading can directly influence the intensity of volcanic eruptions. The ice removal leads to a process known as isostatic rebound, where the crust undergoes vertical adjustments to compensate for the lost mass. The resulting changes in local stress conditions may influence volcanic processes (McNutt, 1999).
On a global scale, continuous sulphate and sulphur records from Greenland and Antarctic ice cores provide estimations on the intensity, frequency and climatic effects of large volcanic eruptions (Lin et al., 2022). During the deglacial period (16–9 ka b2k), a significant rise in the frequency and magnitude of volcanic events is recorded in Greenland (Lin et al., 2022). However, in Antarctica, the deglacial period cannot be distinguished from other periods, highlighting an asymmetry between the two hemispheres. This suggests that the isostatic unloading of the Northern Hemisphere ice sheets may only be associated with an enhanced volcanic activity in that hemisphere (Lin et al., 2022).
In regions such as Alaska, the interaction between glacial retreat and volcanic activity reflects both in increased eruption frequency (Praetorius et al., 2016) and variations in magma composition (Pagli and Sigmundsson, 2008). Also, Lucas et al. (2022) investigate the role of glaciers on the stability of subglacial volcanoes, with focus on Westdahl Peak, in the Aleutian Arc. By applying thermo-mechanical models, they find that the presence of a thick ice cap increases the repose interval of volcanic systems, particularly in cases where magma flux rates are low. The additional pressure provided by the ice load can slightly delay eruptions, e.g., by around 7 years at Westdahl Peak, thus highlighting the importance of accounting for glacial dynamics in volcanic forecasting.
Over longer time scales, eruptive products from Cascades and Alaska–Aleutian arc volcanoes cluster in time following major deglaciations (Calvert et al., 2014). Based on a compilation of edifice-volumes vs. precise argon ages for over 700 lava events from 16 volcanoes, correlated to marine oxygen isotope stages (MIS 2–8), Calvert et al. (2014) show that deglaciations resulted in depressurization of the magmatic systems, leading to decompression melting and/or reduced compressive stress on crustal magma reservoirs.
A counter-intuitive connection between glaciers and volcanism is reported by Geyer and Bindeman (2011), who point out how eruptive activity in Kamchatka can be instead enhanced by glaciations. In fact, during maximal glacial periods, caldera-forming eruptions are more likely to occur. Numerical modelling illustrates how the weakening of volcanic edifices caused by glacial erosion may enable major volcanic events and caldera collapses.
The study of Jellinek et al. (2004) highlight a glacial signature on volcanic activity even at intermediate latitudes. Their findings on eastern California volcanoes indicate that periods of volcanic activity—both basaltic and silicic—are influenced by changes in the volume of ice, i.e., eruptions tend to cluster during periods of significant ice volume changes, with the eruption frequency proportional to the rate of change in ice volume. The smoothed eruption age distribution shows peaks at 10, 100, 185, 320, and 690 kyr, corresponding to interglacial cycles 1, 5, 7, 9, and 17, thus confirming early observations by Glazner et al. (1999). The mechanism underlying is again glacial unloading, as the removal of ice pressure during glacial retreat reduces the stress acting on the magma chambers, promoting magma ascent and eruption. However, Jellinek et al. (2004) notice variable time lags between glacial unloading and volcanic response, distinct for the different activity styles: silicic volcanism (characterized by more explosive eruptions), shows a time lag of 3.2 ± 4.2 kyr, while basaltic volcanism exhibits a longer lag of 11.2 ± 2.3 kyr. This suggests distinct dynamics triggering silicic vs. basaltic eruptions, with silicic eruptions potentially being related to more complex magma chamber systems.
The impact of ice-load removal on volcanic activity in subduction zones is also investigated by Rawson et al. (2016). The post-deglaciation eruptive history of Mocho-Choshuenco volcano, Chile, reveals three distinct phases: Phase 1 (13–8.2 ka) featured dacitic and rhyolitic, large explosive eruptions; Phase 2 (7.3–2.9 ka) characterized by basaltic andesite lavas and lower eruption rates; and Phase 3 (since 2.4 ka), with intermediate magma compositions and higher eruption rates. This behaviour is attributed to changes in magma storage conditions, influenced by crustal stress variations: during periods of glacial overload, magma stalls and differentiates; subsequently, glacial unloading promotes large eruptions, followed by less-differentiated eruptions, and eventually a return to more evolved compositions. Similar patterns are observed in other Chilean volcanoes, suggesting a common post-glacial response in volcanic arcs worldwide (Watt et al., 2013; Rawson et al., 2016; Mora and Tassara, 2019; Wilson and Russell, 2020; Singer et al., 2024). However, Conway et al. (2023) point out the limitations in the geochronological and eruption volume datasets in volcanic arc studies, which make it difficult to test whether eruption rates are actually correlated with ice coverage. According to Conway et al. (2023), these major limitations consist in: 1) the potential for biased preservation and exposure of eruptive materials within certain periods of a volcano lifespan; 2) the different resolutions of geochrolonogical data for volcanic eruptions and climate proxy records; 3) the reliance on data only referring to the Last Glacial Termination (∼18 ka), which is rarely compared through the whole Pleistocene to test the reproducibility of eruptive patterns; and 4) the lack of consideration that eruption rates and magma compositions may be also controlled by mantle and crustal processes that operate independently of glacial advance/retreat.
A recent investigation of the Milbanke Sound Volcanoes, British Columbia (Hamilton et al., 2024), also invokes the role of glacial unloading, related to the retreat of the Wisconsinan ice sheet, in promoting magma ascent and eruption due to the lithostatic pressure release on magma chambers. Coonin et al., (2024) state that, in West Antarctica, the rate of glacial unloading effects the cumulative mass discharge of magma and, consequently, the heat released into the ice cap. Also, recent studies on the Pleiades Volcanic Field, Antarctica (Agostini et al., 2024; Rocchi et al., 2024), detail how the glacial load influences volcanic activity and magma differentiation trends. The Pleiades Volcanic Field consist of hawaiitic to trachytic monogenetic volcanic cones formed over the last 900 kyr. It appears that the thick ice cap of Antarctica hindered eruptive activity, favouring prolonged magma residence times, significant crustal assimilation and unusual fractionation patterns in the erupted products, as also evidenced by 87Sr/86Sr and 143Nd/144Nd ratios (Agostini et al., 2024). These findings highlight the surface ice as a key external factor in shaping the eruptive behaviour and the composition of volcanic rock suites. In this regard, alkaline ash fall deposits from the Early to Middle Miocene activity of the Erebus Volcanic Province, Antarctica, reveal the response of explosive activity to glacial cyclicity (Nyland et al., 2013). By taking into account 56 cycles of glacial advance and retreat, it appears that more intense and frequent eruptions occur during ice minimum conditions. From a petrological point of view, trace element (Ba to Hf, Nb, La and Zr) variations in mafic glasses (≥5 wt.% MgO) with time are interpreted as the result of different duration and depth of magma storage, related to changes in the stress state of the crust in response to rapid ice load fluctuations (Nyland et al., 2013).
Correlations between intra-plate volcanism in western Europe and climate patterns during the Quaternary are reported by Nowell et al. (2006). Specifically, by comparing eruption ages in France and Germany with a composite oxygen isotope record, it appears that significant volcanic activity was linked to warming phases at the end of major glacial stages, particularly between 415–400 ka and 175 ka. For example, caldera-forming eruptions in the eastern Eifel, Germany, coincide with the onset of interglacial periods. The distribution of ice sheets during glacial phases, affecting the Earth’s lithosphere through flexural loading, may explain the observed correlation between intra-plate volcanic activity and climate warming Nowell et al. (2006).
With respect to the complex interactions among glacial, volcanic, and climate factors, a key question concerns the effects of increased volcanic activity, implying a concomitant increase of CO2 release in the atmosphere, on global climate during deglaciation periods. Huybers and Langmuir (2009) find that, during the last deglaciation, while ocean ridge volcanism decreased, due to an increase in the hydrostatic pressure related to the sea level rise, subaerial volcanism instead increased globally by two to six times, particularly in deglaciating regions, potentially due to mantle decompression induced by the melting of glaciers and/or changes in eruption timing linked to glacial variability. The increase in subaerial volcanic activity and related CO2 release likely contributed to the 40 ppm rise in atmospheric CO2 observed in ice cores during this period, giving rise to a positive feedback loop that accelerated deglaciation. Huybers and Langmuir (2009) conclude that huge CO2 emission from subaerial volcanism may play a key role in the transition from glacial to interglacial periods, highlighting the importance of long-term volcanic gas emissions in climate dynamics. Recently, the high-resolution terrestrial record of the Middle Eocene Climatic Optimum (MECO) from the Bohai Bay Basin (eastern China; Ma et al., 2024), reveals episodic mercury enrichments, volcanic signatures, and enhanced chemical weathering during the MECO peak warmth. These data indicate that volcanic activity played a role in the gradual rise of atmospheric CO2, while orbital forcing associated with the 405 kyr and 100 kyr eccentricity maxima around 40.27 Ma triggered peak warming and a positive carbon cycle feedback.
Finally, short-term climatic variations, like seasonal snow load changes, can also affect volcanic activity, thus providing additional supporting evidence to long-term impact of ice-load removal on volcanism. For example, Albino et al. (2010) observe that in Icelandic volcanoes like Grímsvötn and Katla, surface unloading events triggered by rapid snowmelt variations, can alter the pressure balance in shallow magma chamber and increase the probability of volcanic eruptions.
One of the most intriguing interactions links volcanic eruptions to short-term sea-level changes resulting from the motion of the surface water mass during the annual hydrological cycle (Mason et al., 2004). McNutt (1999) and McNutt and Beavan (1987) observe a marked seasonal periodicity of eruptions at Pavlof Volcano (Alaska) involving magma volumes ranging from 0.1 to 16 million cubic meters. Their findings indicate that variations in sea level and atmospheric pressure may induce stress changes beneath the volcano, which, in turn, may promote eruptions. Specifically, ocean loading related to sea level rise may produce a compressive strain in the Earth’s crust “…consistent with squeezing out magma, analogous to squeezing a tube of toothpaste” (McNutt and Beavan, 1987). Mason et al. (2004) report on a seasonal pattern of volcanic eruptions observed at individual volcanoes, particularly along the Pacific “Ring of Fire.” Their analysis suggests that the annual motion of the surface water mass, linked to the hydrological cycle, acts as a boundary condition for eruption rates, as the surface deformation induced by regional sea level changes modulates the frequency of eruptions. Also, the modulation of seismicity by tidal and hydrological periodicities has been detected at Campi Flegrei caldera (Petrosino et al., 2018). These studies emphasize the sensitivity of volcanic systems even to minor seasonal fluctuations in sea level that determine relatively small changes in the ambient stress state.
Beyond seasonal periodicities, the interaction between sea-level changes and volcanic activity is addressed in broader regional contexts over millennial time scales, which is referred to as glacio-eustatic fluctuations. For example, Lund and Asimow (2011) suggest that variations in sea level during glacial and interglacial cycles may influence the rate of magma generation beneath mid-ocean ridges, a major contributor to global volcanism. Their model shows that the decompression rate of the mantle source, which controls magma production by partial melting, is highly sensitive to sea-level fluctuations. During periods of rapid sea-level drop, e.g., during the transitions between Marine Isotope Stages (MIS) 5/4 and 3/2, magma flux at mid-ocean ridges increases. Conversely, during periods of sea-level rise, magma flux decreases. Olive et al. (2015) evidence how fluctuations in ridge magma supply caused by sea level changes modulate melt production in the underlying mantle. However, seafloor-shaping processes act as a low-pass filter on variations in magma supply, strongly damping fluctuations shorter than about 100,000 years. Bathymetric data from the Australian-Antarctic ridge show spectral peaks in the rate of changes of bathymetry at Milankovitch periods of 23, 41, and 100 kyr (Crowley et al., 2015). An intriguing observation is that the 100-kyr signal is not detected in the topography of oceanic ridges with fast spreading rates (Crowley et al., 2015). They note that a 100-kyr long period of sea level change would result in a negligible rate of pressure variation in the melting zone. Since the background rate of decompression melting is relatively higher at fast spreading rates, the proportional change in magma flux to the ridge axis would therefore be undetectable.
By contrast, other studies evidence the sensitivity of seafloor volcanism to sea level changes due to orbital factors, particularly to the 100 kyr periodicity (Boulahanis et al. 2020). In this regard, the Southern East Pacific Rise represents a key site where faulting is minimal and magmatism is most pronounced (Tolstoy, 2015). However, an analysis of bathymetric data from intermediate, fast, and superfast spreading ridges provides no supporting evidence for the Milankovitch Cycle control on ridge topography (Goff et al., 2018). Also, fluctuations in sea level linked to glacio-eustatic cycles have been hypothesized to influence hydrothermal activity at mid-ocean ridges over the past 50 kyr (Middleton et al., 2016). The deposition of hydrothermal iron and copper is observed to have increased during the Last Glacial Maximum, followed by a rapid decline during the sea level rise associated with deglaciation (Lund et al., 2016; Middleton et al., 2016).
The relationship between sea-level changes and volcanic activity also extends beyond the context of mid-ocean ridges to continental volcanic systems. Coussens et al. (2016) observe that periods of enhanced eruptive activity at Montserrat, Caribbean Sea, coincide with increased instability and large landslides that occur during periods of rapid sea-level rise. In particular, the analysis of tephra fall and volcaniclastic turbidite deposits in the drill cores reveals three heightened periods of volcanic activity at Montserrat (∼930 to ∼900 ka, ∼810 to ∼760 ka, and ∼190 to ∼120 ka) that coincide with periods of increased volcano instability. The interaction between tectonic forces and volcanic activity is complex, and these landslides may represent a feedback mechanism, where changes in the crustal stress due to fluctuating sea levels trigger both volcanic eruptions and landslides. These findings contribute to a growing body of evidence that links sea level changes to volcanic response through the modulation of crustal stress.
Sternai et al. (2017) propose that the marked sea-level fluctuations connected to the Messinian salinity crisis in the Mediterranean Sea (5–6 million years ago) may have caused lithospheric unloading that triggered a magmatic pulse in the region. Numerical modelling shows that a kilometre-scale drawdown in sea level affected magmatic systems in the whole Mediterranean region. This case provides additional evidence for the broader impact of sea-level changes on volcanic activity, particularly when combined with other geological processes, such as regional tectonism and lithospheric unloading.
Recently, Satow et al. (2021) report a correlation of the pattern of eruptive activity at Santorini, Aegean Sea, with glacio-eustatic sea-level fluctuations over a 360 kyr-long record. According to their numerical modelling, a drop in sea level induces tensile stresses in the magma chamber, leading to dyke injections and eruptions. This study highlights how the interaction among tectonic forces, magma chamber dynamics, and sea-level fluctuations is crucial in determining the timing and intensity of volcanic eruptions.
These findings support prior evidence reported by McGuire et al. (1997) on the role of sea-level changes on Mediterranean volcanic activity during the late Quaternary. Their statistical analysis, based on the detailed record of marine tephra in deep-sea sediment cores of the central Mediterranean, reveals a nonlinear correlation between the frequency of explosive eruptions in the region and the rate of sea-level change. McGuire et al. (1997) suggest that the growth and decay of large ice sheets may affect volcanic activity through stress-related mechanisms. Also, the volcanic events in Campania, southern Italy, over the past 190 kyr show a 23,000-year periodicity, aligning with changes in magma geochemistry (Paterne et al., 1990). This cycle has been linked to the lifespan of local magma chambers and influenced by precessional forcing and glacio-eustatic pressure variations. De Rita et al. (1994) and de Rita et al., (2002) report another example of the interplay between the occurrence of major explosive eruptions in near coastal volcanoes of central Italy and Quaternary eustatic cycles. The role of glacio-eustatic fluctuations in near-coastal environments is also hypothesised to explain the transition of Mount Etna (Sicily) from a fissure-type shield volcano to a cluster of stratovolcanoes at around 129–126 ka (Stewart, 2017). This transition, previously attributed to tectonic forces, is thought to have been abrupt and coincident with the Eemian sea-level highstand.
The hypothesis that glacio-eustatic cycles may modulate volcanic activity, first observed at individual volcanoes after the last glacial maximum, has led to extensive studies using tephra records across multiple glacial cycles (Kutterolf et al., 2013; 2019; Schindlbeck et al., 2018; Straub et al., 2024). Tephra archives, correlated to climate proxies like δ18O that track sea-level changes, reveal Milankovitch periodicities (i.e., precession, obliquity, and eccentricity) in the occurrence of major volcanic eruptions. Statistical analyses show that enhanced eruptive activity often aligns with deglaciation periods with identified spectral peaks near Milankovitch cycles (41 and 100 kyr), as well as significant correlations with the global δ18O record (Kutterolf et al., 2019). Also, tephra records from IODP Hole U1437B (northwest Pacific) reveal a cyclic pattern of explosive volcanism over the past 1.1 million years (Schindlbeck et al., 2018). Spectral analysis of the data identifies a statistically significant peak at approximately 100 kyr, which aligns with the dominant global climate cycles since the Middle Pleistocene. A time-domain analysis of the full records of eruptions and of the δ18O of benthic foraminifera, used as a climate/sea level proxy, shows that volcanic activity peaks after the glacial maximum, which occurred 13 ± 2 kyr before the δ18O minimum that marks the glacial/interglacial transition. Spectral analysis of volcanic activity from the Pacific Ring of Fire also reveals a significant peak at the obliquity period, a Milankovitch cycle associated with variations in the Earth’s axial tilt (Kutterolf et al., 2013). The volcanic activity in these regions tends to lag behind the highest rate of sea-level rise by approximately 4,000 years (Kutterolf et al., 2013), providing further evidence for a causal link between glacio-eustatism and volcanic activity on a global scale.
Recent evidence from the Kamchatka-Kurile arc provides a link between time-precise records of volcanic activity and climate variations (Straub et al. 2024). In fact, analysis of the Plio-Pleistocene tephrostratigraphy reveals cyclic peaks of eruptive activity corresponding to global ice volume changes, with periodicities that match precession (0.3, 1.0 Myr) and obliquity (1.6, 2.5 and 3.8 Myr) cycles. By assuming a decreasing obliquity variance during the mid-Pleistocene Transition, while precession variance remains constant, the results by Straub et al. (2024) show a strong correlation between ash bed cycles and global benthic δ18O variability (r2 = 0.75), which is interpreted as an example of climate tuning of volcanic activity.
Overall, the above reported examples point to the broader implications of sea-level changes in modulating volcanic systems, beyond isolated cases and across entire volcanic regions. Evidence for a connection between climate variations and volcanism is reported even beyond Plio-Pleistocene. Recent cyclostratigraphic analysis and spectral methods reveal cycles of volcanic activity linked to astronomical orbital periods in the Upper Permian of Southwestern China, during the waning stage of mantle plume activity of the Emeishan Large Igneous Province (Tianyang et al., 2024). The detection of long eccentricity (405 kyr), obliquity (∼33 kyr), and precession (∼20 kyr) cycles thus suggests an astronomical orbital tuning of volcanic activity.
In the previous two sections, we illustrated the wide interest of the scientific community in the multifaceted relationship between volcanic activity and environmental factors, such as glacial-interglacial cycles, sea-level fluctuations (driven by seasonal and glacio-eustatic factors), and other climate events. These processes can create a feedback loop, where climate-driven changes on the Earth’s surface influence volcanic behaviour, which in turn impacts the climate. In this section, we explore the fascinating theme of the direct interactions between volcanism and astronomical factors, including the gravitational actions induced by the dynamics of the Earth’s orbit and of other celestial bodies up to the galactic scale.
A large body of evidence indicates a link between volcanic eruptions and gravitational forces related to solid Earth tides, with recent reviews by Sottili et al. (2021) and Dumont et al. (2022a). Semi-diurnal to fortnightly frequencies of tidal stresses are widely reported to modulate the temporal pattern of eruptive activity of open-conduit volcanoes worldwide (e.g., Stromboli and Etna; Sottili et al., 2007; Sottili and Palladino, 2012), with effects on volcano flank instabilities (Bozzano et al., 2013). Gravitational forces acting on seasonal to multiyear timescales include centrifugal forces from changes in the Earth’s rotation rate (Length of Day variations, LOD) and shifts in the direction of the rotation axis (pole tide) and are considered potential drivers of tectonic, seismic and volcanic activities, from the scale of individual volcanoes (Lambert and Sottili, 2019; 2023) up to the global scale (Riguzzi et al., 2010; Milyukov et al., 2011; Palladino and Sottili, 2014; Sottili et al., 2015; Tuel et al., 2017).
The connection between Earth’s geological and geophysical events and these external forces is further explored by Dumont et al. (2022b) and Le Mouël et al. (2023), with respect to the influence of tidal forces and polar motion on volcanic activity. These studies show that gravitational variations due the motion of the Earth’s rotation axis correlates to global sea levels and volcanic eruptions, indicating that gravitational forces related to solar activity and the motion of large celestial bodies can modulate volcanic behaviour on long timescales. Le Mouël et al. (2023) extend this idea by linking volcanic cycles to solar and planetary interactions, particularly those involving the Jovian planets.
Over millennial time scales, beyond the mediation of glacio-eustatism, some authors provide evidence for a direct influence of orbitally driven gravitational effects (i.e., related to eccentricity, obliquity and precession) on volcanism (Bezverkhnii 2017; Bezverkhnii 2019). Bezverkhnii (2014) suggests that the observed 41 kyr climate periodicity in the Plio-Pleistocene may be an effect of magma degassing and explosive volcanism, in turn linked to obliquity oscillations, with variations in ice volume and albedo further increasing climate variability. Using wavelet and spectral analysis, Bezverkhnii (2014) involves Earth’s orbital factors, rather than solar irradiance, in affecting geodynamic processes and climate changes recorded by geological and ice core data. Wang and Lin (2015) note that paleoclimate variations show the ∼23, ∼40, and ∼100 kyr periodicities typically associated with orbital changes. However, they suggest that oceanic and atmospheric processes may not have enough thermal and dynamic inertia (or “memory”) to fully explain these variations. Using a Sun-Moon gravitation model for a rotating Earth, Wang and Lin (2015) speculate that changes in the orbital obliquity might transfer kinetic energy from the lower mantle into thermal energy, thus affecting the Earth’s heat budget, even though the exact mechanisms—such as slow heat transfer to the surface and/or the release of greenhouse gases like CO2—remain elusive.
A number of studies point to significant periodicities in the order of millions of years or even longer, suggesting that celestial motions superimpose to internal Earth’s dynamics in controlling the cyclicities of volcanic activity, tectonic shifts, climate oscillations and mass extinctions. Boulila et al. (2018), Boulila et al. (2021) examine how internal Earth processes, such as plate tectonics (including subduction processes), are linked to external forces with Milankovitch cyclicity, which govern Earth’s climate on timescales of tens of millions of years. These studies propose that periodicities of 10–36 million years observed in the geological record may be driven by astronomical factors, including the Earth’s motion through the Milky Way and the Sun’s position relative to the Galaxy (Greco and Krasnyy, 2021). In addition, these cycles likely modulate climate and sea-level variations. Gies and Helsel (2005) promote this idea by exploring the Sun’s motion through the Milky Way, particularly its passage through the spiral arms. The increased cosmic-ray exposure during these crossings may influence Earth’s climate by contributing to ice ages. Similarly, Gillman and Erenler (2019) connect the structure of the Milky Way, especially the spiral arms, to major events on Earth, including mass extinctions, suggesting a cyclical pattern of environmental perturbations linked to galactic structures. These findings imply that the periodic nature of galactic events could be a key factor in shaping Earth’s geological history. The role of galactic forces in shaping Earth’s climate and geological processes is also explored by Shaviv et al. (2014) and Zhang et al. (2023), who propose that the Solar System’s motion through the galactic plane may induce periodic climate oscillations. The Solar System’s oscillation, and its impact on the cosmic ray flux, could explain the 30–33 Myrs cycles in volcanic eruptions and mass extinctions (Rampino and Stothers, 1984; Prokoph et al., 2004). This pattern aligns with the Solar System’s oscillation through the galactic plane, suggesting that increased cometary impacts during these periods may have triggered mass extinctions.
Furthermore, Puetz and Condie (2019) show that mantle and crustal processes exhibit periodicities on the scale of tens to hundreds of millions of years. Their analysis of isotopic data reveals cycles of approximately 93.5 and 187 million years, which coincide with global geodynamic processes and the timing of superchrons. These findings suggest that the Earth’s internal dynamics, including the formation of large igneous provinces, which also exhibit cyclic patterns, may be influenced by both terrestrial processes and extraterrestrial factors.
Lastly, Wang and Lin (2015) model the gravitational interaction of Sun and Moon that impart long-term periodic oscillations in Earth’s geophysical fluids, including magma motion, which contribute to the rhythmic climate variability observed in the Earth’s history on timescales of thousands to millions of years.
In summary, these studies collectively point to a complex, multi-scale interaction between Earth’s internal processes and external celestial forces. Whether through the gravitational effects of the Sun, the motion of the Solar System through the galaxy, or tidal and polar forces, these interactions appear to influence key geological and climate events on both short and long timescales. Further exploration of these cyclic patterns could provide valuable insights into the mechanisms driving Earth’s geological and environmental evolution.
The response of volcanic systems to environmental changes involving Earth’s climate, cryosphere, and hydrosphere is multifaceted and complex, through a range of mechanisms that influence magma production and volcanic activity over a variety of timescales. From the seasonal modulation of the field stress beneath individual volcanoes to the large-scale effects of glacial-interglacial cycles, the role of environmental changes on the volcanic behaviour represents an important area of study. Parallel to the acquisition of new data and analytical techniques, our understanding of how these interactions operate is evolving continuously, shedding light on the broader dynamics of the Earth system.
Our present knowledge shows dynamic and intricate, often bidirectional, relationships between volcanic activity and climate, shaped by both internal geodynamic processes and external astronomical forces. Detailed eruptive records, integrated by ice core data, show how volcanic systems worldwide are highly sensitive to orbitally driven, climate fluctuations with Milankovitch cyclicities. Many studies identify the changes in ice load and sea level linked to glacial-interglacial cycles as significant factors modulating the intensity pattern of volcanic activity, by driving tectonic stresses, magma generation, storage and eruption, and related CO2 emissions. Periods of enhanced magma generation and volcanic activity are attributed to pressure variations due to the redistribution of ice and seawater masses, particularly during deglaciation (i.e., decompression melting induced by glacial unloading and sea level drop). In this regard, recent research highlights the possible influence of sea-level fluctuations on ocean ridge magmatism on Milankovitch timescales.
On the one hand, Earth’s orbital variations, particularly obliquity and precession cycles, which control global climate changes, seem to reflect indirectly on volcanism. On the other hand, a growing body of evidence point to a direct interconnection of astronomical factors, including the gravitational actions induced by the dynamics of the Earth’s orbit and of other celestial bodies, and geodynamic processes, including plate tectonics, seismicity and volcanism. Beyond the widely reported effects of tidal forces on the activity patterns of volcanoes on a short timescale, the volcanic response to the Milankovitch cycles strongly suggests a direct gravitational-orbital forcing on volcanism, concomitant with, but essentially independent of, major climate and environmental changes.
Furthermore, other external processes, e.g., including solar activity and polar motion, may act significantly on volcanic and tectonic activities. Ultimately, the numerous studies mentioned in this review emphasize that volcanic behaviour, as well as biodiversity patterns and environmental conditions, are influenced by long-term cyclical processes driven by the interplay of geophysical, astrophysical, and climate factors. Understanding these complex interactions, a common goal across different research disciplines, is essential for advancing our knowledge of Earth’s geological history and eventually improving our ability to predict its future evolution.
GS: Conceptualization, Investigation, Writing–original draft, Writing–review and editing. DP: Conceptualization, Investigation, Writing–original draft, Writing–review and editing.
The author(s) declare that no financial support was received for the research, authorship, and/or publication of this article.
The authors declare that the research was conducted in the absence of any commercial or financial relationships that could be construed as a potential conflict of interest.
The author(s) declare that no Generative AI was used in the creation of this manuscript.
All claims expressed in this article are solely those of the authors and do not necessarily represent those of their affiliated organizations, or those of the publisher, the editors and the reviewers. Any product that may be evaluated in this article, or claim that may be made by its manufacturer, is not guaranteed or endorsed by the publisher.
Abe-Ouchi, A., Saito, F., Kawamura, K., Raymo, M. E., Okuno, J., Takahashi, K., et al. (2013). Insolation-driven 100,000-year glacial cycles and hysteresis of ice-sheet volume. Nature 500 (7461), 190–193. doi:10.1038/nature12374
Agostini, S., Leone, N., Smellie, J. L., and Rocchi, S. (2024). Magma differentiation, contamination/mixing and eruption modulated by glacial load—the volcanic complex of the Pleiades, Antarctica. Geochem. Geophys. Geosyst. 25 (1), e2024GC011509. doi:10.1029/2024GC011509
Albino, F., Pinel, V., and Sigmundsson, F. (2010). Influence of surface load variations on eruption likelihood: application to two Icelandic subglacial volcanoes, Grímsvotn and Katla. Geophys. J. Int. 181 (3), 1510–1524. doi:10.1111/j.1365-246X.2010.04588.x
Archer, D., Winguth, A., Lea, D., and Mahowald, N. (2000). What caused the glacial/interglacial atmospheric pCO2 cycles? Rev. Geophys. 38, 159–189. doi:10.1029/1999RG000066
Aubry, T. J., Farquharson, J. I., Rowell, C. R., Watt, S. F. L., Pinel, V., Beckett, F., et al. (2022). Impact of climate change on volcanic processes: current understanding and future challenges. Bull. Volcanol. 84, 58. doi:10.1007/s00445-022-01562-8
Berger, A., and Loutre, M. F. (1991). Insolation values for the climate of the last 10 million years. Quat. Sci. Rev. 10, 297e317. doi:10.1175/1520-0469(1978)0352.0.CO;2
Bezverkhnii, V. A. (2014). Correlation between 41,000-year rhythms in variations in the inclination of Earth, violent volcanic eruptions, and temperature of deep ocean waters. Izvestiya, Atmos. Ocean. Phys. 50 (6), 657–659. doi:10.1134/S0001433814040124
Bezverkhnii, V. A. (2017). Earth’s obliquity oscillations can influence climate change by driving global volcanic activity. Geoscience Res. 2 (1), 22–26. doi:10.22606/gr.2017.21004
Bezverkhnii, V. A. (2019). On the 100,000-year rhythmicity in geodynamics and the paleoclimate. Izvestiya, Phys. Solid Earth 55 (5), 488–495. doi:10.1134/s1069351319030017
Boulahanis, B., Carbotte, S. M., Huybers, P. J., Nedimović, M. R., Aghaei, O., Canales, J. P., et al. (2020). Do sea level variations influence mid-ocean ridge magma supply? A test using crustal thickness and bathymetry data from the East Pacific Rise. Earth Planet. Sci. Lett. 535, 116121. doi:10.1016/j.epsl.2020.116121
Boulila, S., Haq, B. U., Hara, N., Müller, R. D., Galbrun, B., and Charbonnier, G. (2021). Potential encoding of coupling between Milankovitch forcing and Earth’s interior processes in the Phanerozoic eustatic sea-level record. Earth-Sci. Rev. 220, 103727. doi:10.1016/j.earscirev.2021.103727
Boulila, S., Laskar, J., Haq, B. U., Galbrun, B., and Hara, N. (2018). Long-term cyclicities in Phanerozoic sea-level sedimentary record and their potential drivers. Glob. Planet. Change 165, 128–136. doi:10.1016/j.gloplacha.2018.03.004
Bozzano, F., Gaeta, M., Lenti, L., Martino, S., Paciello, A., Palladino, D. M., et al. (2013). Modeling the effects of eruptive and seismic activities on flank instability at Mount Etna, Italy. J. Geophys. Res. Solid Earth 118, 5252–5273. doi:10.1002/jgrb.50377
Bray, J. R. (1976). Volcanic triggering of glaciation. Nature 260 (5553), 414–415. doi:10.1038/260414a0
Bulian, F., Marra, F., Monaco, L., Palladino, D. M., Scarponi, D., Sevink, J., et al. (2024). 40Ar/39Ar geochronologic and paleoenvironmental constraints to glacial termination III and MIS 7e, 7c, and 7a sea level fluctuations on the Tyrrhenian Sea coast of Italy. Glob. Planet. Change 242, 104594. doi:10.1016/j.gloplacha.2024.104594
Calvert, A. T., Sisson, T. W., Bacon, C. R., and Ferguson, D. J. (2014). Apparent eruptive response of Cascades and Alaska-Aleutian arc volcanoes to major deglaciations. Fall Meeting: American Geophysical Union. Abstract V11B-4710.
Conway, C. E., Pure, L. R., and Ishizuka, O. (2023). An assessment of potential causal links between deglaciation and eruption rates at arc volcanoes. Front. Earth Sci. 11, 467. doi:10.3389/feart.2023.1082342
Coonin, A. N., Huber, C., Troch, J., Townsend, M., Scholz, K., and Singer, B. S. (2024). Magma chamber response to ice unloading: applications to volcanism in the West Antarctic rift system. Geochem. Geophys. Geosyst. 25, e2024GC011743. doi:10.1029/2024GC011743
Cooper, C. L., Swindles, G. T., Savov, I. P., Schmidt, A., and Bacon, K. L. (2018). Evaluating the relationship between climate change and volcanism. Earth Sci. Rev. 177, 238–247. doi:10.1016/j.earscirev.2017.11.009
Coussens, M., Coussens, M., Wall-Palmer, D., Talling, P. J., Watt, S. F., Cassidy, M., et al. (2016). The relationship between eruptive activity, flank collapse, and sea level at volcanic islands: a long-term (>1 Ma) record offshore Montserrat, Lesser Antilles. Geochem. Geophys. Geosyst. 17 (7), 2591–2611. doi:10.1002/2015GC006053
Crowley, J. W., Katz, R. F., Huybers, P., Langmuir, C. H., and Park, S.-H. (2015). Glacial cycles drive variations in the production of oceanic crust. Science 347 (6227), 1237–1240. doi:10.1126/science.1261508
de Rita, D., Fabbri, M., Mazzini, I., Paccara, P., Sposato, A., and Trigari, A. (2002). Volcaniclastic sedimentation in coastal environments: the interplay between volcanism and Quaternary sea level changes (central Italy). Quat. Int. 95–96, 141–154. doi:10.1016/s1040-6182(02)00035-6
de Rita, D., Milli, S., Rosa, C., Zarlenga, F., and Cavinato, G. (1994). Catastrophic eruptions and eustatic cycles: example of Latium volcanoes. Atti Convegni Lincei 112, 135–142.
Dumont, S., Custódio, S., Petrosino, S., Thomas, A., and Sottili, G. (2022a). “Tides, earthquakes, and volcanic eruptions,” in A tidal journey through Earth’s history. Editors M. Green, and J. C. Duarte (Elsevier), 1–13.
Dumont, S., Petrosino, S., and Neves, M. C. (2022b). On the link between global volcanic activity and global mean sea level. Front. Earth Sci. 10, 845511. doi:10.3389/feart.2022.845511
Edwards, B. R., Russell, J. K., and Pollock, M. (2022). Cryospheric impacts on volcano-magmatic systems. Front. Earth Sci. 10, 871951. doi:10.3389/feart.2022.871951
Eksinchol, I., Rudge, J. F., and Maclennan, J. (2019). Rate of melt ascent beneath Iceland from the magmatic response to deglaciation. Geochem. Geophys. Geosyst. 20 (6), 2585–2605. doi:10.1029/2019GC008222
Gee, M. A., Taylor, R. N., Thirlwall, M. F., and Murton, B. J. (1998). Glacioisostacy controls chemical and isotopic characteristics of tholeiites from the Reykjanes Peninsula, SW Iceland. Earth Planet. Sci. Lett. 164 (1), 1–5. doi:10.1016/s0012-821x(98)00246-5
Geyer, A., and Bindeman, I. (2011). Glacial influence on caldera-forming eruptions. J. Volcanol. Geotherm. Res. 202 (1–2), 127–142. doi:10.1016/j.jvolgeores.2011.02.001
Gies, D. R., and Helsel, J. W. (2005). Ice age epochs and the Sun’s path through the galaxy. Astrophys. J. 626, 844–848. doi:10.1086/430250
Gillman, M., and Erenler, H. (2019). Reconciling the Earth’s stratigraphic record with the structure of our galaxy. Geosci. Front. 10 (6), 2147–2151. doi:10.1016/j.gsf.2019.06.001
Glazner, A. F., Manley, C. R., Marron, J. S., and Rojstaczer, S. (1999). Fire or ice: anticorrelation of volcanism and glaciation in California over the past 800,000 years. Geophys. Res. Lett. 26 (12), 1759–1762. doi:10.1029/1999gl900333
Goff, J. A., Zahirovic, S., and Dietmar, M. R. (2018). No evidence for Milankovitch cycle influence on abyssal hills at intermediate, fast, and superfast spreading rates. Geophys. Res. Lett. 45 (10), 305–310. doi:10.1029/2018gl079400
Greco, F., and Krasnyy, I. V. (2021). The novel pushing gravity model and volcanic activity: is alignment of planets with compact stars a possible cause of natural phenomena? J. Phys. Conf. Ser. 1, 012019. doi:10.1088/1742-6596/2081/1/012019
Grove, E. W. (1974). “Deglaciation—a possible triggering mechanism for recent volcanism,” in Proceedings symposium of andean and antarctic volcanology, 88–97.
Hall, K. (1982). Rapid deglaciation as an initiator of volcanic activity: an hypothesis. Earth Surf. Process. Landforms 7, 45–51. doi:10.1002/esp.3290070106
Hamilton, T. S., Enkin, J. R., Li, Z., Bednarski, J. M., Stacey, C. D., McGann, M. L., et al. (2024). Where ice gave way to fire: deglacial volcanic activity at the edge of the Coast Mountains in Milbanke Sound, BC. Can. J. Earth Sci. 61 (1), 58–85. doi:10.1139/cjes-2023-0080
Harðarson, B. S., and Fitton, J. G. (1991). Increased mantle melting beneath Snaefellsjökull volcano during Late Pleistocene deglaciation. Nature 353, 62–64. doi:10.1038/353062a0
Hays, J. D., Imbrie, J., and Shackleton, N. J. (1976). Variations in the Earth's orbit: pacemaker of the ice ages. Science 194 (4270), 1121–1132. doi:10.1126/science.194.4270.1121
Hooper, A., Ófeigsson, B., Sigmundsson, F., Lund, B., Geirsson, H., and Sturkell, E. (2011). Increased capture of magma in the crust promoted by ice-cap retreat in Iceland. Nat. Geosci. 4 (11), 1038–1041. doi:10.1038/ngeo1269
Huybers, P., and Langmuir, C. (2009). Feedback between deglaciation, volcanism, and atmospheric CO2. Earth Planet. Sci. Lett. 286 (3–4), 479–491. doi:10.1016/j.epsl.2009.07.014
Jellinek, A. M., Manga, M., and Saar, M. O. (2004). Did melting glaciers cause volcanic eruptions in eastern California? Probing the mechanics of dike formation. J. Geophys. Res. Solid Earth 109, B09206. doi:10.1029/2004jb002978
Jull, M., and McKenzie, D. (1996). The effect of deglaciation on mantle melting beneath Iceland. J. Geophys. Res. Solid Earth 101 (B10), 21815–21828. doi:10.1029/96jb01308
Kennett, J. P., and Thunell, R. C. (1975). Global increase in Quaternary explosive volcanism. Science 187 (4176), 497–503. doi:10.1126/science.187.4176.497
Kutterolf, S., Jegen, M., Mitrovica, J. X., Kwasnitschka, T., Freundt, A., and Huybers, P. J. (2013). A detection of Milankovitch frequencies in global volcanic activity. Geology 41 (2), 227–230. doi:10.1130/g33419.1
Kutterolf, S., Schindlbeck, J. C., Jegen, M., Freundt, A., and Straub, S. M. (2019). Milankovitch frequencies in tephra records at volcanic arcs: the relation of kyr-scale cyclic variations in volcanism to global climate changes. Quat. Sci. Rev. 204, 1–16. doi:10.1016/j.quascirev.2018.11.004
Lambert, S., and Sottili, G. (2019). Is there an influence of the pole tide on volcanism? Insights from Mount Etna recent activity. Geophys. Res. Lett. 46, 13730–13736. doi:10.1029/2019GL085525
Lambert, S., and Sottili, G. (2023). Possible role of tidal and rotational forcing on bradyseismic crises and volcanic unrest in the Campi Flegrei and Somma-Vesuvius areas. Front. Earth Sci. 11, 1060434. doi:10.3389/feart.2023.1060434
Le Mouël, J.-L., Gibert, D., Courtillot, V., Dumont, S., de Bremond d’Ars, J., Petrosino, S., et al. (2023). On the external forcing of global eruptive activity in the past 300 years. Front. Earth Sci. 11, 1254855. doi:10.3389/feart.2023.1254855
Lin, J., Svensson, A., Hvidberg, C. S., Lohmann, J., Kristiansen, S., Dahl-Jensen, D., et al. (2022). Magnitude, frequency and climate forcing of global volcanism during the last glacial period as seen in Greenland and Antarctic ice cores (60–9 ka). Clim. Past. 18, 485–506. doi:10.5194/cp-18-485-2022
Lisiecki, L. E., and Raymo, M. E. (2005). A Pliocene-Pleistocene stack of 57 globally distributed benthic δ18O records. Paleoceanography 20, PA1003. doi:10.1029/2004PA001071
Lucas, L. C., Albright, J. A., Gregg, P. M., and Zhan, Y. (2022). The impact of ice caps on the mechanical stability of magmatic systems: implications for forecasting on human timescales. Front. Earth Sci. 7, 868569. doi:10.3389/feart.2022.868569
Lund, D. C., and Asimow, P. D. (2011). Does sea level influence mid-ocean ridge magmatism on Milankovitch timescales? Geochem. Geophys. Geosyst. 12 (12). doi:10.1029/2011GC003693
Lund, D. C., Asimow, P. D., Farley, K. A., Rooney, T. O., Seeley, E., Jackson, E. W., et al. (2016). Enhanced East Pacific Rise hydrothermal activity during the last two glacial terminations. Science 351 (6272), 478–482. doi:10.1126/science.aad4296
Ma, Y., Fan, M., Zhang, C., Grasby, S. E., Yin, R., Lu, Y., et al. (2024). Volcanic and orbitally forced carbon release during the Middle Eocene climatic Optimum. Geology 52 (11), 813–818. doi:10.1130/G52435.1
MacLennan, J., Jull, M., McKenzie, D., Slater, L., and Grönvold, K. (2002). The link between volcanism and deglaciation in Iceland. Geochem. Geophys. Geosyst. 3 (11), 1–25. doi:10.1029/2001GC000282
Mason, B. G., Pyle, D. M., Dade, W. B., and Jupp, T. (2004). Seasonality of volcanic eruptions. J. Geophys. Res. Solid Earth 109 (B4). doi:10.1029/2002JB002293
McGuire, W. J., Howarth, R. J., Firth, C. R., Solow, A. R., Pullen, A. D., Saunders, S. J., et al. (1997). Correlation between rate of sea-level change and frequency of explosive volcanism in the Mediterranean. Nature 389, 473–476. doi:10.1038/38998
McNutt, S. R. (1999). Eruptions of Pavlof Volcano, Alaska, and their possible modulation by ocean load and tectonic stresses: Re-evaluation of the hypothesis based on new data from 1984–1998. Pure Appl. Geophys. 155, 701–712. doi:10.1007/s000240050284
McNutt, S. R., and Beavan, R. J. (1987). Eruptions of Pavlof Volcano and their possible modulation by ocean load and tectonic stresses. J. Geophys. Res. Solid Earth 92 (B11), 11509–11523. doi:10.1029/jb092ib11p11509
Middleton, J. L., Langmuir, C. H., Mukhopadhyay, S., McManus, J. F., and Mitrovica, J. X. (2016). Hydrothermal iron flux variability following rapid sea level changes. Geophys. Res. Lett. 43 (8), 3848–3856. doi:10.1002/2016gl068408
Milankovitch, M. (1941). Canon of insolation and the ice-age problem, 132. Royal Serb. Belgrade: Acad. Spec. Publ., 633.
Miller, G. H., Geirsdottir, A., Zhong, Y., Larsen, D. J., Otto-Bliesner, B. L., Holland, M. M., et al. (2012). Abrupt onset of the Little Ice Age triggered by volcanism and sustained by sea-ice/ocean feedbacks. Geophys. Res. Lett. 39 (2). doi:10.1029/2011GL050168
Milyukov, V. K., Kravchyuk, V. K., Mironov, A. P., and Latynina, L. A. (2011). Deformation processes in the lithosphere related to the nonuniformity of the Earth’s rotation. Izv. Phys. Solid Earth 47, 246–258. doi:10.1134/S1069351311020042
Monaco, L., Leicher, N., Palladino, D. M., Arienzo, I., Marra, F., Petrelli, M., et al. (2022b). The Fucino 250-170 ka tephra record: new insights on peri-Tyrrhenian explosive volcanism, central Mediterranean tephrochronology, and timing of the MIS 8-6 climate variability. Quat. Sci. Rev. 296, 107797. doi:10.1016/j.quascirev.2022.107797
Monaco, L., Palladino, D. M., Albert, P. G., Arienzo, I., Conticelli, S., Di Vito, M., et al. (2022a). Linking the Mediterranean MIS 5 tephra markers to Campi Flegrei (southern Italy) 109-92 ka explosive activity and refining the chronology of MIS 5c-d millennial-scale climate variability. Glob. Planet. Change 211, 103785. doi:10.1016/j.gloplacha.2022.103785
Mora, D., and Tassara, A. (2019). Upper crustal decompression due to deglaciation-induced flexural unbending and its role on post-glacial volcanism at the Southern Andes. Geophys. J. Int. 216 (3), 1549–1559. doi:10.1093/gji/ggy473
Nowell, D. A. G., Jones, M. C., and Pyle, D. M. (2006). Episodic quaternary volcanism in France and Germany. J. Quat. Sci. 21 (6), 645–675. doi:10.1002/jqs.1005
Nyland, R. E., Panter, K. S., Rocchi, S., Di Vincenzo, G., Del Carlo, P., Tiepolo, M., et al. (2013). Volcanic activity and its link to glaciation cycles: single-grain age and geochemistry of Early to Middle Miocene volcanic glass from ANDRILL AND-2A core, Antarctica. J. Volcanol. Geotherm. Res. 250, 106–128. doi:10.1016/j.jvolgeores.2012.11.008
Olive, J. A., Behn, M. D., Ito, G., Buck, W. R., Escartín, J., and Howell, S. (2015). Sensitivity of seafloor bathymetry to climate-driven fluctuations in mid-ocean ridge magma supply. Science 350, 310–313. doi:10.1126/science.aad0715
Pagli, C., and Sigmundsson, F. (2008). Will present-day glacier retreat increase volcanic activity? Stress induced by recent glacier retreat and its effect on magmatism at the Vatnajökull ice cap, Iceland. Geophys. Res. Lett. 35 (9), L09304. doi:10.1029/2008GL033510
Palladino, D. M., Simei, S., Sottili, G., and Trigila, R. (2010). Integrated approach for the reconstruction of stratigraphy and geology of Quaternary volcanic terrains: an application to the Vulsini Volcanoes (central Italy). Geol. Soc. Am. Spec. Pap. 464, 63–84. doi:10.1130/2010.2464(04)
Palladino, D. M., and Sottili, G. (2014). Earth's spin and volcanic eruptions: evidence for mutual cause-and-effect interactions? Terra nova. 26 (1), 78–84. doi:10.1111/ter.12073
Paterne, M., Labeyrie, J., Guichard, F., Mazaud, A., and Maitre, F. (1990). Fluctuations of the Campanian explosive volcanic activity (South Italy) during the past 190,000 years as determined by marine tephrochronology. Earth Planet. Sci. Lett. 98 (2), 166–174. doi:10.1016/0012-821X(90)90057-5
Petrosino, S., Cusano, P., and Madonia, P. (2018). Tidal and hydrological periodicities of seismicity reveal new risk scenarios at Campi Flegrei caldera. Sci. Rep. 8 (1), 13808–13812. doi:10.1038/s41598-018-31760-4
Praetorius, S., Mix, A., Jensen, B., Froese, D., Milne, G., Wolhowe, M., et al. (2016). Interaction between climate, volcanism, and isostatic rebound in Southeast Alaska during the last deglaciation. Earth Planet. Sci. Lett. 452, 79–89. doi:10.1016/j.epsl.2016.07.033
Prokoph, A., Rampino, M. R., and El Bilali, H. (2004). Periodic components in the diversity of calcareous plankton and geological events over the past 230 Myr. Palaeogeogr. Palaeoclimatol. Palaeoecol. 207, 105–125. doi:10.1016/j.palaeo.2004.02.004
Prueher, L. M., and Rea, D. K. (1998). Rapid onset of glacial conditions in the subarctic North Pacific region at 2.67 Ma: clues to causality. Geology 26, 1027–1030. doi:10.1130/0091-7613(1998)026<1027:roogci>2.3.co;2
Puetz, S. J., and Condie, K. C. (2019). Time series analysis of mantle cycles part I: periodicities and correlations among seven global isotopic databases. Geosci. Front. 10 (4), 1305–1326. doi:10.1016/j.gsf.2019.04.002
Rampino, M. R., and Stothers, R. B. (1984). Terrestrial mass extinctions, cometary impacts, and the Sun’s motion perpendicular to the galactic plane. Nature 308 (5961), 709–712. doi:10.1038/308709a0
Rawson, H., Pyle, D. M., Mather, T. A., Smith, V. C., Fontijn, K., Lachowycz, S. M., et al. (2016). The magmatic and eruptive response of arc volcanoes to deglaciation: insights from southern Chile. Geology 44 (4), 251–254. doi:10.1130/g37504.1
Riguzzi, F., Panza, G., Varga, P., and Doglioni, C. (2010). Can Earth’s rotation and tidal despinning drive plate tectonics? Tectonophysics 484, 60–73. doi:10.1016/j.tecto.2009.06.012
Rocchi, I., Tomassini, A., Masotta, M., Petrelli, M., Ágreda López, M., and Rocchi, S. (2024). Textures and chemistry of crystal cargo of the Pleiades volcanic field, Antarctica: potential influence of ice load in modulating the plumbing system. J. Petrol. 65 (4), egae027. doi:10.1093/petrology/egae027
Ruddiman, W. F. (2003). Orbital insolation, ice volume, and greenhouse gases. Quat. Sci. Rev. 22 (15-17), 1597–1629. doi:10.1016/S0277-3791(03)00087-8
Salvador, A. (1987). Unconformity-bounded stratigraphic units. Geol. Soc. Am. Bull. 98, 232–237. doi:10.1130/0016-7606(1987)98<232:usu>2.0.co;2
Salvador, A. (1994). International stratigraphic guide: a guide to stratigraphic classification, terminology and procedure. 2nd ed. Trondheim, Norway: International Subcommission on Stratigraphic Classification of IUGS International Commission on Stratigraphy: Copublished by the International Union of Geological Sciences.
Satow, C., Gudmundsson, A., Gertisser, R., Ramsey, C. B., Bazargan, M., Pyle, D. M., et al. (2021). Eruptive activity of the Santorini Volcano controlled by sea-level rise and fall. Nat. Geosci. 14, 586–592. doi:10.1038/s41561-021-00783-4
Schindlbeck, J. C., Jegen, M., Freundt, A., Kutterolf, S., Straub, S. M., Mleneck-Vautravers, M. J., et al. (2018). 100-kyr cyclicity in volcanic ash emplacement: evidence from a 1.1 Myr tephra record from the NW Pacific. Sci. Rep. 13 (1), 4440. doi:10.1038/s41598-018-22595-0
Schmidt, P., Lund, B., Hieronymus, C., Maclennan, J., Arnadóttir, T., and Pagli, C. (2013). Effects of present-day deglaciation in Iceland on mantle melt production rates. J. Geophys. Res. Solid Earth 118 (7), 3366–3379. doi:10.1002/jgrb.50273
Shaviv, N. J., Prokoph, A., and Veizer, J. (2014). Is the solar system’s galactic motion imprinted in the Phanerozoic climate? Sci. Rep. 4, 6150. doi:10.1038/srep06150
Sigmundsson, F., Pinel, V., Lund, B., Albino, F., Pagli, C., Geirsson, H., et al. (2010). Climate effects on volcanism: influence on magmatic systems of loading and unloading from ice mass variations, with examples from Iceland. Philos. Trans. R. Soc. A 368, 2519–2534. doi:10.1098/rsta.2010.0042
Sigvaldason, G. E., Annertz, K., and Nilsson, M. (1992). Effect of glacier loading/deloading on volcanism: postglacial volcanic production rate of the Dyngjufjöll area, central Iceland. Bull. Volcanol. 54 (5), 385–392. doi:10.1007/bf00312320
Singer, B. S., Moreno-Yaeger, P., Townsend, M., Huber, C., Cuzzone, J., Edwards, B. R., et al. (2024). New perspectives on ice forcing in continental arc magma plumbing systems. J. Volcanol. Geotherm. Res. 455, 108187. doi:10.1016/j.jvolgeores.2024.108187
Slater, L., Jull, M., McKenzie, D., and Grönvöld, K. (1998). Deglaciation effects on mantle melting under Iceland: results from the northern volcanic zone. Earth Planet. Sci. Lett. 164 (1–2), 151–164. doi:10.1016/s0012-821x(98)00200-3
Sottili, G., Lambert, S., and Palladino, D. M. (2021). Tides and volcanoes: a historical perspective. Front. Earth Sci. 9, 777548. doi:10.3389/feart.2021.777548
Sottili, G., Martino, S., Palladino, D. M., Paciello, A., and Bozzano, F. (2007). Effects of tidal stresses on volcanic activity at Mount Etna, Italy. Geophys. Res. Lett. 34 (1), L01311. doi:10.1029/2006gl028190
Sottili, G., and Palladino, D. M. (2012). Tidal modulation of eruptive activity at open-vent volcanoes: evidence from Stromboli, Italy. Terra nova. 24 (3), 233–237. doi:10.1111/j.1365-3121.2012.01059.x
Sottili, G., Palladino, D. M., Cuffaro, M., and Doglioni, C. (2015). Earth’s rotation variability triggers explosive eruptions in subduction zones. Earth Planet. Space 67, 208. doi:10.1186/s40623-015-0375-z
Sternai, P., Caricchi, L., Castelltort, S., and Champagnac, J.-D. (2016). Deglaciation and glacial erosion: a joint control on magma productivity by continental unloading. Geophys. Res. Lett. 43, 1632–1641. doi:10.1002/2015gl067285
Sternai, P., Caricchi, L., Garcia-Castellanos, D., Jolivet, L., Sheldrake, T. E., and Castelltort, S. (2017). Magmatic pulse driven by sea-level changes associated with the Messinian Salinity Crisis. Nat. Geosci. 10, 783–787. doi:10.1038/ngeo3032
Stewart, I. S. (2017). Did sea-level change cause the switch from fissure-type to central-type volcanism at Mount Etna, Sicily? Episodes 41 (1), 7–16. doi:10.18814/epiiugs/2018/v41i1/018002
Straub, S. M., Reilly, B., Raymo, M. E., Gómez-Tuena, A., Wang, K.-L., Widom, E., et al. (2024). Patterns of Plio-Pleistocene ice volume variability recorded by the large-magnitude explosive eruptions from the Kamchatka-Kurile volcanic arc. Geochem. Geophys. Geosyst. 25, e2024GC011748. doi:10.1029/2024GC011748
Tianyang, Y., Yulin, S., Lu, L., Jun, J., Yunfei, Z., Lijun, Z., et al. (2024). Milankovitch cycles recorded by the Late Permian volcanic ash layers in southwestern China. Mar. Petrol. Geol. 161, 106671. doi:10.1016/j.marpetgeo.2023.106671
Tolstoy, M. (2015). Mid-ocean ridge eruptions as a climate valve. Geophys. Res. Lett. 42, 1346–1351. doi:10.1002/2014gl063015
Tuel, A., Naveau, P., and Ammann, C. M. (2017). Skillful prediction of multidecadal variations in volcanic forcing. Geophys. Res. Lett. 44 (6), 2868–2874. doi:10.1002/2016GL072234
Van Vliet-Lanoë, B., Bergerat, F., Allemand, P., Innocentd, C., Guillou, H., Cavailhes, T., et al. (2020). Tectonism and volcanism enhanced by deglaciation events in southern Iceland. Quat. Res. 94, 94–120. doi:10.1017/qua.2019.68
Wang, Z. J., and Lin, X. (2015). Astronomy and climate-Earth system: can magma motion under Sun-Moon gravitation contribute to paleoclimatic variations and Earth’s heat? Adv. Astronomy 2015, 1–10. paper ID 536829. doi:10.1155/2015/536829
Watanabe, Y., Abe-Ouchi, A., Saito, F., Kino, K., O’ishi, R., Ito, T., et al. (2023). Astronomical forcing shaped the timing of early Pleistocene glacial cycles. Comm. Earth Environ. 4, 113. doi:10.1038/s43247-023-00765-x
Watt, S. F. L., Pyle, D. M., and Mather, T. A. (2013). The volcanic response to deglaciation: evidence from glaciated arcs and a reassessment of global eruption records. Earth Sci. Rev. 122, 77–102. doi:10.1016/j.earscirev.2013.03.007
Wilson, A. M., and Russell, J. K. (2020). Glacial pumping of a magma-charged lithosphere: a model for glaciovolcanic causality in magmatic arcs. Earth Planet. Sci. Lett. 548, 116500. doi:10.1016/j.epsl.2020.116500
Keywords: volcanism and climate, glacio-eustatism, obliquity and precession forcing, orbital (Milankovitch) cycles, pole tide effects on climate, Earth’s precession-nutation
Citation: Sottili G and Palladino DM (2025) When volcanoes record Milankovitch cycles. Front. Earth Sci. 13:1569128. doi: 10.3389/feart.2025.1569128
Received: 31 January 2025; Accepted: 03 March 2025;
Published: 19 March 2025.
Edited by:
Pierpaolo Zuddas, Sorbonne Universités, FranceReviewed by:
Fernando Lopes, Muséum National d’Histoire Naturelle, FranceCopyright © 2025 Sottili and Palladino. This is an open-access article distributed under the terms of the Creative Commons Attribution License (CC BY). The use, distribution or reproduction in other forums is permitted, provided the original author(s) and the copyright owner(s) are credited and that the original publication in this journal is cited, in accordance with accepted academic practice. No use, distribution or reproduction is permitted which does not comply with these terms.
*Correspondence: Gianluca Sottili, Z2lhbmx1Y2Euc290dGlsaUB1bmlyb21hMS5pdA==
Disclaimer: All claims expressed in this article are solely those of the authors and do not necessarily represent those of their affiliated organizations, or those of the publisher, the editors and the reviewers. Any product that may be evaluated in this article or claim that may be made by its manufacturer is not guaranteed or endorsed by the publisher.
Research integrity at Frontiers
Learn more about the work of our research integrity team to safeguard the quality of each article we publish.