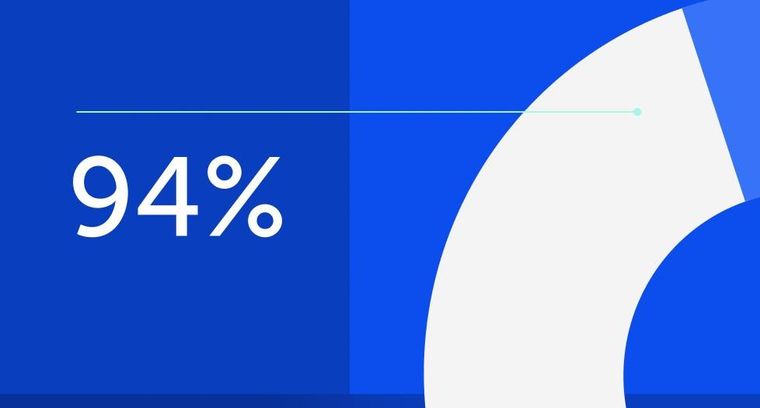
94% of researchers rate our articles as excellent or good
Learn more about the work of our research integrity team to safeguard the quality of each article we publish.
Find out more
REVIEW article
Front. Earth Sci., 06 March 2025
Sec. Biogeoscience
Volume 13 - 2025 | https://doi.org/10.3389/feart.2025.1514255
Since commercial manufacture of plastics started around 1950, plastics have grown more and more important to human society. The ubiquity of plastic particles in the environment and Inefficient waste management have led to the presence of tiny plastic particles in a wide range of natural matrices. Nowadays, finding natural environments with the most potential to archive the past deposition of airborne microplastics is among the hot research topics while investigating plastic pollution across the globe. The capability of peatlands as the most widespread type of wetlands throughout the earth to illustrate natural and anthropogenic deposition of different contaminants has drawn the attention of researchers in recent years. A number of studies have been conducted on the presence and distribution of various pollutants in peatland areas. However, there is still limited information on the presence of microplastics in peatlands. The purpose of this study is to gather the existing data on the occurrence, deposition and distribution of microplastics in peatland areas. We have tried to examine the potential of peatlands as natural archives of atmospheric micro and nano plastics. The research indicates that peatlands serve as a reliable (with some uncertainties) geo-archive for atmospheric micro (nano) plastics. It thoroughly assesses various methods, from sampling to final analyses, to empower researchers in selecting the most effective approach.
− Peatlands can be considered as effective geo-archives of Microplastics
− Ombrotrophic peatlands must be preferred over minerotrophic ones while assessing Microplastics
− 210Pb dating can be viewed as the appropriate age dating method when it comes to assessing Microplastics in peat sequences
− Optimum sampling method depends on the characteristics of the peatland.
Plastic has become one of the most extensively utilized materials in contemporary society. The excessive production of plastics, coupled with their low recycling rates and high environmental persistence, has rendered them a significant emerging environmental concern, characterized by their rapid accumulation in natural ecosystems (Eerkes-Medrano et al., 2015). Although plastics exhibit high durability upon disposal in the environment, they are subjected to a range of processes, including chemical weathering, photoxidation, biodegradation, and mechanical degradation, which induce structural modifications over time. The term “microplastic” was initially introduced by Thompson et al. (2004). The scientific community has not yet reached consensus on a complete and comprehensive definition of the term “microplastic.” The National Oceanic and Atmospheric Administration (NOAA) researchers define microplastics as plastic fragments measuring less than 5 mm in diameter (Arthur et al., 2009). Microplastics can be defined as polymeric particles, either regular or irregular in shape, with dimensions ranging from 1 μm to 5 mm.
Microplastics are recognized as one of the most significant emerging pollutants in all environmental compartments. Microplastics can be introduced into various environmental compartments through numerous sources, including synthetic textiles, tire wear (particularly from automobiles and trucks), household items, waste incineration, construction materials, sewage sludge, landfills, 3D printing processes, and the resuspension of polymer particles in urban dust (Osman et al., 2023). The widespread presence of microplastics in natural environments, coupled with their potential impacts on human health and living organisms, has raised significant concerns among researchers. Considering the presence of microplastic particles in the atmosphere and their deposition influenced by various factors, identifying a natural environment capable of preserving the deposition records of such particles can be important in assessing the extent of plastic pollution over time and presenting optimal strategies to mitigate future pollution levels. Furthermore, these pollutants have been used as “technofossils” in sedimentary cores to evaluate the evolution of plastic types and create a microplastic chronological sequence in the sedimentary record (Chen et al., 2022).
Peatlands are considered as the most widespread wetlands that are formed due to the decay of plant residues under water saturation (Minayeva et al., 2018). There is no consensus on a specific definition for peatlands and peats. According to Joosten and Clarke (2002), peatlands are ecosystems rich in carbon that have a layer of peat that is more than 30 cm deep and contains at least 30% dry mass of decomposed organic matter. On the contrary, Peat was defined by Burton and Hodgson (1987) as a type of soil that contains at least 50% organic materials. In addition, histosols which are described as soils consisting of at least 20% organic substances or include at least 18% organic materials under water-saturated conditions for 30 consecutive days, are also classified as peatlands in some regions (Michéli et al., 2006). These ecosystems play a significant role in global carbon cycling since they store massive amounts of C in form of peat. According to IPCC (Intergovernmental Panel on Climate Change) (2007), boreal and subarctic peatlands store 270–370 Tg C in form of peat (equivalent to 34%–36% of atmospheric C in the form of CO2). Moreover, tropical peatlands are approximated to retain 52 Tg C (Hooijer et al., 2006). Thus, peatlands not only can be considered as reservoirs of atmospheric CO2, with a crucial influence on the atmospheric fluxes of CO2 and CH4, but also they exert influence on dissolved carbon (DC) fluxes into rivers due to destabilization of peatlands because of global warming (Limpens et al., 2008). Approximately 12% of global peatlands have been drained and damaged, resulting in significant carbon loss (Smith et al., 2024).
The estimation of peatland extent across the globe encompasses huge uncertainties. Based on a meta-analysis (Xu et al., 2018), we created a new GIS map showing the distribution of peatlands worldwide (Figure 1). According to the recently released map of peatlands, there are an estimated 4.23 million km2 of peatlands worldwide, or 2.84% of all land area. The obtained result is consistent with the current Parish et al. (2008) approximation of 4 million km2. Peatlands can be classified based on various parameters such as the nature of water supply, vegetation and hydromorphological structure.
Peatlands can be identified in different ways. “Ombrotrophic” and “minerotrophic” peatlands are the two main classes of peatlands considering the existing water source (Lindsay, 2016). Ombrotrophic peatlands are defined as peatlands that are segregated from all sources of surface or groundwater and fed explicitly by atmospheric wet and dry deposition like ash, dust, rain, snow, and fog (Allen et al., 2021). Sphagnum species predominate in their vegetation, which also includes other bryophytes, monocotyledons, and dwarf shrubs; however, their vegetation varies greatly in other parts of the world, like the southeast Asian tropical peat swamp forests. Sphagnum-dominated peatlands have been investigated more than any other types (Gilbert and Mitchell, 2006; Hughes et al., 2013; Marcisz et al., 2020). These types of peatlands are usually acidic and their pH ranges from 3 to 5.5 (Wheeler and Proctor, 2000). For instance, Aukštumala raised bog, which is located inside the Curonian freshwater lagoon is suspected to contain high levels of plastic pollution. The contamination of plastic particles in the surface beach sand of the Curonian Spit National Park has already been indicated (Esiukova et al., 2020).
In recent years the two terms “Bog” and “Fen” have also been utilized in order to describe the two main classes of peatlands. The former refers to peatlands that are uniquely fed by direct precipitation (ombrotrophic), and the latter is attributed to peatlands that have been saturated by groundwater or surface water (minerotrophic). Minerotrophic peatlands may receive inputs from either groundwater or surface water as well as the atmosphere (Lindsay, 2016) and have a highly diverse range of vegetation types. For instance, the vegetation of certain minerotrophic in northwest Europe is characterized by lack of Sphagnum and an abundance of species such as Molinia caerulea, Menyanthes trifoliata, Carex species, and Equisetum species (Charman, 2002), whereas Sphagnum species and sedges predominate in others (Lamentowicz et al., 2007).
This study aims to advance our understanding of the current status of research on the presence of micro (nano) plastics (MnPs) in peatlands. It attempts to assess the potential of peatlands as natural archives of microplastics. The current study presents a state-of-the-art, comprehensive perspective and benefits and drawbacks concerning to use of peatlands as a geo-archives for atmospheric MnPs. Moreover, it has been tried to comprehensively discuss the key aspects of using peatlands as natural archives of microplastics such as optimum sampling and age dating methods. Additionally, it serves as a valuable resource for researchers, to provide a framework for conducting studies in this field. To our best knowledge, no systematic review article has yet been published on the subject of MnPs occurrence in peatlands and their potential as MnP records.
In order to acquire adequate information relevant scientific studies have been gathered through online searches in the databases of Science Direct, and Google Scholar. The searched keywords included “microplastic” and “peatlands” or “peatland areas”, or “ombrotrophic peatlands”, or “peat cores” were examined. Moreover, the keywords “nanoplastic” and “peatlands” or “peat cores”, or “ombrotrophic peatlands”, or “peatland areas” were also considered. “Peatlands” and “plastic pollution”, or “plastic accumulation” were also searched. Furthermore, “peatlands” or “ombrotrophic peatlands” and “atmospheric deposition” or “atmospheric microplastics” were investigated as well. At the next stage, non-English articles were omitted. A total of 6 published studies during 2021-2023 were opted and scrutinized. Details about each stage are going to be explained in the next sections of the paper.
When conducting paleoenvironmental research, ombrotrophic peatlands should always be chosen over minerotrophic ones since they can be considered as effective atmospheric particle traps, which makes them a great archive of past events except at high latitudes such as sub-Arctic and Arctic regions, where ombrotrophic peatlands can scarcely found. Consequently, at high latitudes, minerotrophic peat deposits can serve as archives of atmospheric deposits despite being fed by both terrestrial and atmospheric inputs. For example, Shotyk et al. (2003) examined the collected peat cores from a minerotrophic peatland to evaluate the contributions of humans to the accumulation of Pb, Hg, and As in the atmosphere in southern Greenland and Denmark. Ideally, the dome (ombrotrophic peatland) of a bog is its most valuable component since due to the higher elevation, it is exclusively fed by rainfall and provides us with information on atmospheric deposition. Neither surface water runoff from surrounding areas nor groundwater can reach the higher section of the bog since it is above the local groundwater table.
As mentioned before, micro and nano plastics are one of the emerging contaminants on our planet. The versatility, stability, lightweight, and economical production of plastics has made them indispensable in our daily lives microplastics are plastic particles equal to or greater than 1 µm and less than 5 mm in (aerodynamic) diameter (Allen D. et al., 2022). Since these microscopic plastic particles have been detected in a plethora of habitats, including soils, their presence in the environment has drawn the attention of researchers across the globe. In fact, microplastics have been spotted in soils (Hoang et al., 2024; Yang et al., 2021; Wang et al., 2020; Boots et al., 2019), marine environments (Biswas and Pal, 2024; Cole et al., 2011; Nunes et al., 2023; Chen et al., 2023), marine organisms (Terrazas-López et al., 2024; Harmon et al., 2024; Parolini et al., 2023), atmosphere (Nguyen et al., 2024; O'Brien et al., 2023; Gasperi et al., 2018; Sridharan et al., 2021) and even pristine and remote environments (Abbasi et al., 2021; Mishra et al., 2021; Trainic et al., 2020; Allen S. et al., 2022). Archiving microplastic records from the past to the present will provide us with a comprehensive understanding of changes in the abundance of microplastics, which can help policymakers adopt more efficient mitigation strategies. Table 1 compares peat samples with two common plastic archives (marine/riverine sediments and ice cores).
Temporal records of environmental changes have been obtained from a considerable range of natural archives, such as corals (Williams, 2020), ice cores (Vallelonga et al., 2021), peat sequences (Fracasso et al., 2024), etc. In addition, sporadic records from herbaria (Lavoie, 2013), museum specimens (Schmitt et al., 2019), and the teeth, antlers (Kierdorf et al., 2022), and eggs of birds (Montanari, 2018) have been collected. The ubiquity of different types of peatlands throughout the world has made them a plausible option for monitoring a wide range of environmental contaminants (Figure 1).
Peatlands have been studied extensively as natural repositories of previously deposited airborne potentially toxic elements (PTEs) as these contaminants, specifically Pb, Cd and Hg, are capable of being transferred far away from their local sources due to long-range atmospheric transport (Miszczak et al., 2020; Kylander et al., 2006; Hansson et al., 2015; Talbot et al., 2017; Coggins et al., 2006; Luo et al., 2023). A few studies have also investigated the presence of polycyclic aromatic hydrocarbon (PAHs) in peatlands (Ortiz et al., 2023; Gabov et al., 2020).
Furthermore, peat cores have also been evaluated with the aim of assessing the impacts of air pollution and climate change (Souter and Watmough, 2016; Malawska and Ekonomiuk, 2008). In recent years, peatlands have become a plausible option for archiving microplastics (Table 1; Figure 1) (Nguyen et al., 2022; Allen et al., 2021). Bandekar et al. (2022) indicated that the physicochemical characteristics of submicron microplastics and peat facilitate the adsorption of plastic particles, which implies that plastic particles may be adsorbed by peatland ecosystems. Moreover, Hagelskajaer et al. (2023a) showed the presence of microplastic particles in sphagnum moss peat cores in a number of sites across the globe. The project is still ongoing however, the recent results demonstrated an increasing trend in atmospheric deposition of airborne microplastics in recent years.
The amount of plastic particles in these ecosystems is yet unknown, and the lack of analytical procedures has made it challenging to monitor the actual level of plastic pollution in peatland areas. In fact, the adsorption of polystyrene (PS) and poly (vinyl chloride) (PVC)-SMPs (sub-micron microplastics) to peat was influenced by their chemical composition. As a result, this affected the sub-micron microplastic accumulation by Sphagnum moss as well as the variety and composition of the microbial communities in peatland. Nevertheless, the use of these natural archives for microplastic records is quite limited. Therefore, there are still serious doubts about the potential of each of these natural archives to prepare microplastic records. Table 2 demonstrates the geographical characteristics for peatland microplastics.
Table 2. Geographic characteristics of sampling locations for microplastics research (also shown in Figure 1).
Different sampling techniques can be used while sampling peats in paleoenvironmental studies. Basically, the optimum technique depends on the depth in which sampling occurs. Superficial layers (50–100 cm) must be cored cautiously in order to obtain a perfectly shaped core, which is a significant foundation for next stages such as the exact approximation of subsample volume. François et al. (2010) described two methods for sampling superficial layers. For coring of the first 50–100 cm (acrotelm and the subjacent part of catotelm) a stainless steel Wardenaar peat corer can be utilized. The purpose of this double-bladed cutter corer is to extract intact peat monoliths. The full description has been provided by Wardenaar (1987). Allen et al. (2021) used a Wardenaar corer to collect a peat core from the selected peat site in order to evaluate the potential of an ombrotrophic peatland for archiving microplastics deposition. Another peat corer that functions on the same principles is known as Malcolm sampler, which has been fully depicted by Cuttle and Malcolm (1979). Despite the Wardenaar sampler, in this apparatus one of the blades is smaller than the other, functioning as a lid that can be removed to reveal the core upon retrieval. It must be noticed that the Wardenaar or Malcolm blades’ cutting edges need to be sharpened, particularly if there are fibrous layers or roots that are difficult to cut through. If such material is not cut efficiently before pushing, the core will be compressed (François et al., 2010).
For deeper layers, another device which is referred to as Belarus corer is typically used. Actually, two versions of this corer are in use: titanium and stainless steel. The same alloy that was used to make the Wardenaar corer was also used to make the titanium corer, which is significantly lighter. Based on different circumstances, other peat corers may also be used during the investigation of peats. For example, Permafrost can be found worldwide in the organic soils of high altitudes and polar regions. In these areas, the thickness of ice is usually more than 1 m. There are extra difficulties when it comes to coring frozen peat, and the aforementioned corers are not appropriate to sample frozen peats. Tommy Nørnberg created and constructed a special peat sampler to collect continuous samples of frozen peat in the Arctic (Nørnberg et al., 2004). The device produced cores that were 70 cm long and 9.7 cm in diameter (Nørnberg et al., 2004). The cores that were recovered using this apparatus were successfully used to evaluate atmospheric mercury deposition in the Arctic on Bathurst Island, Nunavut (Givelet et al., 2004a). Later, to make the extraction of peat cores easier, Teflon was sprayed onto the core barrel. Using peat from the Carey Islands in Greenland, the updated version was utilized to replicate atmospheric mercury and cadmium deposition in the Arctic (Outridge et al., 2016).
Overall, it is rational to prefer ombrotrophic peatlands over minerotrophic ones while conducting research on the presence of microplastics in peatland areas except for regions that are located at high latitudes. The elimination of the inputs from surface or groundwater will not only facilitate the assessment but also provide us with a more accurate understanding of atmospheric deposition. We suggest that both surface and deep sampling are needed while investigating the presence of microplastics in peatlands since small-size microplastics are capable of migrating to deeper layers. For surface sampling modified version of the Wardenaar sampler is proposed which is commercially available in the market since it has shown great success in the uppermost 1 m of surface peat layers, yielding extensive, undamaged monoliths that make precise environmental reconstructions easier. For deep sampling, Belarus corer will be effective.
Moreover, the effects of core compression during the sampling must also be considered. Although some degrees of compression are inevitable during the sampling process (especially when the surface of the bog is dry), it must be prevented as much as possible. Givelet et al. (2004b) mentioned the effects of core compression on anthropogenic Pb concentrations and the age-depth relationship. The results indicated that the aging or magnitude of changes in anthropogenic Pb concentrations will not be impacted by the compression of the peat core alone. However, in terms of the effect of core compression on microplastic concentration further investigations are needed. Finally, the importance of covering the monoliths with foil bags, while transporting them to the laboratory to avoid any plastic pollution. Figure 2 illustrates the different peat sampling methods.
Because slicing regulates sample thickness, which is crucial for volume estimations, it is considered as a key process. In addition, slicing a peat core with fibrous layers (such as those of Scheuchzeria and Eriophorum spp.) makes the process challenging. There are some techniques in use today, but there is no consensus on an optimum way to slice a peat monolith or core. Whichever option is selected, the greatest amount of caution should be used to reduce material loss and cross-contamination. A precise procedure is to measure the core/monolith section’s total length, freeze it, and then cut it with a band saw. This allows for very accurate measurement of the clean slice dimensions, which is necessary for the accurate derivation of bulk density (François et al., 2010). Basic tools such as titanium knives or scissors may also be practical under special circumstances. Regardless of the instruments utilized, they must be cleaned after every slice is separated. However, plastic tools must be avoided in order to prevent any additional plastic contamination.
The next step, which ensures the reconstruction of high-resolution records and is crucial to sample preparation, is subsampling the previously mentioned slices. Sub-samples must represent the characteristics of the slice from which, they have been cutted. Furthermore, the quantity of the sub-samples must be determined considering the item which is supposed to be analyzed. For instance, Allen et al. (2021) subsampled the collected peat core with 1 cm deep sections in order to examine atmospheric microplastics deposition in ombrotrophic peat.
As mentioned before, peat samples contain considerable amounts of organic matter. The presence of these organic substances may hinder the identification and quantification of microplastics as they can be mistaken for microplastics. Therefore, choosing the most efficient method to remove them is of great significance. Different protocols have been suggested to eliminate organic residues. However, no pre-treatment technique has been unanimously accepted globally. Naidoo et al. (2017) proposed acid digestion for isolation of microplastics in juvenile fish. Enzymatic digestion is another method that has been tested by a number of researchers (Courtene-Jones et al., 2017; Mbachu et al., 2021). Oxidizing is the other procedure which has been used to isolate microplastics (Ziajahromi et al., 2017). Nevertheless, not all digestion processes can eliminate organic matter without damaging polymers. HNO3, for instance, has been shown to degrade polyamide (PA), melt polyethylene terephthalate (PET) and high-density polyethylene (HDPE), and cause polymer yellowing (Dehaut et al., 2016; Karami et al., 2017). Moreover, expense is another important factor that must be taken into account while selecting the optimum strategy. Enzymes are too expensive to be consistently incorporated into microplastic sampling protocols, regardless of their effectiveness. Furthermore, rapid digestion times are typically favored over laborious procedures. Protocols might, for instance, last anywhere from an hour to 24 or 48 h (Catarino et al., 2017; Dehaut et al., 2016; Karami et al., 2017). While thermal digestion can shorten the time required for complete digestion, it also damages polymers, so it should be considered cautiously (Munno et al., 2018).
Prata et al. (2019) investigated the digestion efficiency of five solutions (H2O2, H2O2 + Fe), pH (KOH, HNO3) and a surfactant (SDS) for removing organic substances without damaging microplastics. In order to obtain this goal, two temperature conditions were applied to pools of six organic materials in glass flasks: room temperature (RT, average 25°C) and 50°C temperature (OasisTM Benchtop IR CO2 Incubator, Caron) as well as two-time intervals—one and 6 h. These pools were supplemented with 10 mL of solutions or the control (H2O). Each organic matter sample was exposed to 5 mL of each of the two solutions (KOH and H2O2 + Fe) as well as the control (H2O) for a single test condition: 50°C for 1 h after the results of the first test were analyzed. The next step involved testing the polymers’ resistance to the digestion protocols. Furthermore, resistance of filters on digestion protocols was also evaluated. The highest digestion efficiencies under 1 hour at 50°C were shown by the strong redox (H2O2 + Fe) and high pH (KOH) treatments, at 65.9% and 58.3%, respectively. These treatments were therefore selected for additional testing. When organic matter was tested separately under these circumstances, it was found that KOH was most effective when it came to removing animal tissues, but H2O2 Fe was better suited for removing plant material.
In fact, a considerable number of studies have utilized H2O2 in order to digest organic materials in the investigation of microplastic (Allen et al., 2019; Brahney et al., 2020; Radford et al., 2021). The equilibrium between the breakdown of biogenic organic matter and its inefficacy against synthetic polymers has rendered H2O2 a desirable substance for a number of research groups (Hagelskjær et al., 2023b). Nguyen et al. (2022) during their investigation to study the Occurrence and distribution of microplastics in a tropical peatland in the Mekong Delta, Vietnam, used H2O2 to digest organic matter. Allen et al. (2021) in their study to assess peatlands as a natural archive of atmospheric deposition also used H2O2 and density separation (ZnCL2) to process blank samples. The use of H2O2 digestion in conjunction with an appropriate density separation technique may produce satisfactory results regarding the separation of atmospheric plastic particles through microscopic analysis in aerosol, rain, river, and seawater samples where the plastic-to-biogenic organic matter ratio is quite large. As mentioned before, it is well known that adding Fe2+ as a catalyst increases H2O2 digestion efficiency (Walling, 1975; Prata et al., 2019). However, Hagelskjær et al. (2023a) indicated that neither H2O2 nor Fenton reaction could remove enough organic materials in matrices made mostly of refractory vegetal matter. The main components of sphagnum moss and peat are polysaccharides that contain cellulose and fructose, such as galactose, glucose, mannose, arabinose, and xylose (Black et al., 1955). They offered a unique protocol to enhance the efficiency of removal of organic materials in vegetal matrices such as peat samples. NaClO (50 vol%) digested sphagnum moss to a total of 86%–96% mass, while H2O2 (30 vol%) only removed 24%–46% mass of the matrix (Hagelskjær et al., 2023b). The utilization of the innovative purged air-assisted digestion technique resulted in an increase in NaClO efficiency to over 99% mass and a reduction in processing time. Digestion of different synthetic polymer types showed that high concentrations (>12.5 vol%) of NaClO attack polyamide (PA) and polyethylene terephthalate (PET), but have no effect on polymer types including all strains of polyethylene (PE), polypropylene (PP), polystyrene (PS), polyvinyl chloride (PVC), and acrylonitrile butadiene styrene (ABS), which together account for the majority of annual plastic production. Three commercial sphagnum moss samples were analyzed to show that the protocol could separate and identify aerosol-sized microplastics as small as Ø = 0.8 μm. Therefore, this method can be considered among the ideal digestion methods while preparing peat samples for further steps. Figure 3 introduces the four major techniques which have been used to digest organic matters in peat samples.
Although none of the studies, which have investigated the presence of microplastics in peatlands, have elementally examined peat samples, the addition of this stage may be beneficial as it can provide us with invaluable data about the sources of the detected microplastics. However, we highly encourage conducting elemental, mineralogical, and isotopic analysis in conjunction with the investigation of microplastics for a comprehensive understanding.
Givelet et al. (2004a) suggested that before conducting any costly and time-consuming chemical process or analysis, all peat samples can be evaluated for main and trace elements utilizing the non-destructive, rapid and cost-effective X-ray fluorescence (XRF) approach. This method offers helpful geochemical information that facilitates recording the organic geochemical processes taking place in the peat profiles., which may have an impact on the distribution of trace elements. For instance, strontium (Sr) and calcium (Ca) can be used to identify mineral weathering reactions in peat profiles, as well as redox processes involving iron (Fe) and manganese (Mn), as well as atmospheric aerosols of marine origin containing bromine (Br) and selenium (Se). In addition, Shotyk et al. (2003) showed that the distribution and abundance of the conservative lithogenic elements such as titanium (Ti) and zirconium (Zr) demonstrate variations in the concentration of mineral matter within the peat core. Mowreover, an analytical procedure for determining the total mercury (Hg) concentrations in solid peat samples was presented by Roos-Barraclough et al., 2002. They analyzed samples of solid peat using a direct mercury analyzer (LECO AMA 254). To put it briefly, three subsamples that had been previously collected from a freshly chosen section of each slice were air-dried for a whole night in a Class 100 laminar flow clean air cabinet before being evaluated for total mercury content. After that, the three subsamples’ results were averaged. Furthermore, an extensive range of trace elements can be measured for other elements in solid peat samples through instrumental neutron activation analysis (INAA). Additionally, the trace element contents have been identified through the use of ICP-MS, ICP-OES, HG-AAS, and HG-AFS (Krachler et al., 1999; Chen et al., 2003).
In general, volume reduction is an important stage in preparing samples for chemical analysis. A number of methods are usually used in ordr to reduce the volume of the collected samples including filtration, lyophilisation, nitrogen condensing and desiccation. However, since peat samples contain considerable amounts of organic materials, eliminating them is prior to chemical analysis. Various methods have been suggested for the removal of organic matters, among them utilization of H2O2, KOH, NaOH and Fenton Reagent as digestive agents at maximum 55°C are the most common ones. Munno et al. (2018) examined the effect of temperature and digestion method on microplastics. They deduced that temperatures above 60°C during digestion process can have detrimental impacts on microplastics. They reported that in order to minimize the loss of any constituent microplastics, especially microbeads from personal care products, temperatures must be controlled to avoid temperature spikes during reactions. At or below 60°C, wet peroxide oxidation is still an effective method for digesting samples containing plant matter in freshwater and marine samples (water, sediment), and it may also be applicable to tissues. Evaluations of the prevalence, varieties, origins, and consequences of microplastics could be lacking if some materials are excluded from method-processing conditions. Identification of microplastics is another significant stage, which includes a variety of methods. In general, identification methods can be divided in three categories: visual identification, which encompasses the use of microscope (amongst Nile Red microscopy) for the identification of microplastics, spectroscopy methods, which includes various methods such as Raman spectroscopy (micro-Raman, surface enhanced Raman, Tip-enhanced Raman), FTIR (micro-FTIR, nano-FTIR, atomic force microscopy infrared spectroscopy) and a number of novel methods which have recently been applied by researchers and thermal degradation (pyrolysis, laser desorption/ionization, thermal desorption, etc.). Each of the mentioned methods has its own pros and cons. For instance, thermal degradation may alter the molecular structure of microplastics, resulting in alteration in the chemical composition of detected microplastics. Instead of taking many minutes, the majority of FTIR measurements are completed in a couple of seconds. Furthermore, FTIR significantly increases sensitivity for a variety of reasons. Because of the substantially increased optical throughput and far more sensitive detectors used, there is a significant reduction in noise levels (Dutta, 2017). Besides, the likelihood of a mechanical failure is relatively low. On the other side, Dispersive instruments typically have two beams, while FTIR instruments only have one. When it comes to the non-destructive identification of extremely small microplastics (less than 20 µm), µ-Raman stands out as the preferred technique. It is possible to get over some of the commonly mentioned limitations of Raman techniques, like poor signal and fluorescence interference, by employing more effective detectors, adhering to the proper cleaning protocols, and using baseline removal algorithms. With less operator time required, plastic particle identification is now possible because to the introduction of automated Raman mapping procedures.
A crucial component of paleoenvironmental research is creating high-quality chronologies, particularly for the last several centuries that have seen a great deal of change. In fact, reconstructing the historical rate of organic matter accumulation and exogenous material deposition flux requires accurate peat dating. Furthermore, an authentic and comprehensive chronology is required if peat samples are going to be collated with other natural archives such as polar ice, lake sediments, and bryophytes, including specimens from herbariums. It must be noted that since the peat deposition process is characterized by constant decomposition and compression changes, the relationship between depth and age in a peat core is typically non-linear (Oldfield et al., 1990). Due to developments in dating techniques, particularly 210Pb dating, making use of peat samples, the modern history of atmospheric deposition may now be recreated covering the last 200 years or so. It is of great significance when it comes to probing the presence of microplastics in peatlands. Peat chronologies can be produced using a wide range of techniques, including the examination of fly-ash particles, historical pollen, tephra layers, chronostratigraphic markers, and short-lived radioisotopes (210Pb, 137Cs, 241Am, and 14C bomb pulse). However, the two main procedures are radiocarbon age dating and radiometric age dating.
Radiocarbon (14C) is a naturally occurring radioactive isotope of carbon, which is produced by secondary cosmic rays in the atmosphere (Oldfield et al., 1995). According to Reimer et al. (2004) there are two methods to determine the normalized fraction of 14C (F14C): counting (β-ionization) or accelerator mass spectrometry (AMS) analysis.
This technique includes the counting of electrons, which are 14C’s β-decay products. From the first Libby screen counters (Libby, 1955) to gas proportional counters (GPCs) (Kromer et al., 1992) and liquid scintillation counters (LSCs), the technique has undergone multiple advancements. When β-particles fill the detectors with ionizing gas, they can be detected by GPCs. They can operate with methane or CO2. After being converted to benzene (C6H6), the samples are combined with a scintillation agent such as butyl PBD. Detectable and quantifiable scintillation is produced in the mixture by the β-particles resulting from the decay of the 14C in the sample. For LSCs, samples are usually converted to C6H6 and mixed with butyl PBD (Hajdas et al., 2021). Compared to the AMS method, the procedure is lengthier and requires longer measurement durations. Nevertheless, β counters for handling comparatively large samples are still present in a lot of radiocarbon laboratories across the globe.
Nowadays, the most customary 14C dating technique is AMS. In AMS, the 14C/13C or 14C/12C ratio can be directly measured in the sample, which leads to significantly shorter measurement times (from days to tens of minutes) and lower sample material requirements (from grams to micrograms of carbon) (Hajdas et al., 2021; Kutshera, 2016)). Carbon ions can be extracted from a processed sample of graphite or CO2 using AMS instruments, which analyze the extracted ion beam as it passes through different filters to eliminate undesirable and disruptive species. Both stable and radioactive carbon isotopes are detected and measured while rapidly transitioning between them (Litherland et al., 2011). Further technological advancements in AMS have allowed for the detection of 14C at much lower energies than previously possible, requiring much lower terminal voltage (Synal et al., 2000; Synal et al., 2013).
Only samples containing carbon, either organic or inorganic, can be dated using radiocarbon dating. Materials beyond 55,000 years cannot be reliably dated using radiocarbon (Hajdas et al., 2021). Therefore, microplastic particles can be detected using this method. For instance, Allen et al. (2021) utilized 14C dating technique (combination with 210Pb dating) to date archive samples with the aim of Temporal Archiving of Atmospheric Microplastic Deposition Presented in Ombrotrophic Peat in central Pyrenees.
Numerous radioisotope dating methods (e.g., Rb-Sr, Sm-Nd, and U-Pb) are available that can yield precise ages over a range of time periods. Generally speaking, the time frame of interest and the amount of dateable material that is available determine the dating method of choice. 210Pb is frequently utilized when environmental natural archives such as peat bogs, lake and marine sediments are being dated (Appleby et al., 1988). Mass spectrometers have advanced to the point where time intervals of less than a million years can now be resolved with previously unheard-of resolution (Amelin, 2020). Thermal Ionization Mass Spectrometry (TIMS), Secondary Ion Mass Spectrometry (SIMS), and Laser Ablation - Inductively Coupled Plasma Mass Spectrometry (LA-ICPMS) are the three mass spectrometers that are most frequently used for geochronological purposes.
Moreover, Zircon has been widely employed to date igneous, metamorphic, and sedimentary rocks. It is a hardy mineral that can occur in a variety of geological settings. Zircon is a practical tool for U-Th-Pb geochronology as it includes both U and Th with trivial Pb abundances and resists against weathering (chemical and mechanical), remelting, and variable sedimentation cycles. Due to the recent easing of dilution protocols and advancements in mass spectrometers (ion probe and LA-ICPMS), zircon-based geochronology has become more popular. This is because zircon’s U-Pb ages can be used to determine the emplacement of different lithologies (Joshi et al., 2021). In addition, Titanite (CaTiSiO5), Apatite ((Ca5(PO4)3), Monazite (Ce, La, Nd, Th) (PO4, SiO4), Rutile (TiO2), Baddeleyite (ZrO2) and some other minerals can also be applied in dating samples. The full explanations have been provided by Joshi et al. (2021).
Overall, it seems that diverse geological samples can yield stronger and comparatively more trustworthy geochronological controls/constraints when a multi-proxy method is applied across a range of sample types. We suggest that peat samples can be dated using a combination of 14C and 210Pb dating methods. As mentioned before, Allen et al. (2021) used 210Pb dating in order to date ombrotrophic peat samples with the aim of investigating the potential of peat samples as natural archives. Among the two existing methods of 14C dating, AMS analysis may be preferred as it needs fewer amounts of samples and shorter times. Concludingly, dating peat samples is essential when it comes to modeling the relationship between depth and microplastic concentration in peat samples. Figure 4 descrides the practical age dating methods, being used to examine environmental samples, including peat samples.
Figure 4. Different age dating techniques for samples which include a considerable amount of organic substances.
It is worth mentioning that new dating methods are also being applied to estimate the age of microplastics (Okubo et al., 2023). Therefore, it can be deduced that the relationship between microplastics and peatland areas is bilateral and dating them (microplastics and peat sequences) can provide details on the history of the two aforementioned compartments (peatlands and microplastics).
To enhance our understanding of the behavior of microplastics in peatlands, some information is provided below regarding their color, shape, size, abundance, and polymer types in peatlands (Table 2).
Microplastics can be found in the atmosphere, rivers, soil, and sediment in a variety of colors (Habibi et al., 2022; Fahrenfeld et al., 2019). Nguyen et al. (2022) detected fourteen colors (such as white, blue, red, orange, black, sand, aqua, yellow, green, pink, and transparent) while investigating the occurrence of microplastics in the Mekong Delta, Vietnam. Depending on where the microplastic level was being checked, the color dispersion changed. The color distributions with the highest frequency for the entire samples were blue (n = 102, 25.4%), white (n = 103, 25.6%), and aqua (n = 107, 26.6%), followed by green (n = 51, 12.7%). Transparent microplastics make up just 1.5% of all microplastics, but they are frequently made of single-use plastics that are meant to be used only once, like disposable plastic bottles, bags, and cups (Prata et al., 2019). The least frequent hues found in peatland sediments were forest green, pink, red, brown, and buttercup.
According to Hu et al. (2022) the most typical forms of microplastics found in soil, rivers, and sediments include pellets, fibers, films, foams, and pieces. Unlike a number of studies which have reported fibrous microplastics as dominant shape of detected microplastics, Nguyen et al. (2022) showed that fragments, fibers, films, and foams are among the most common morphological elements of microplastics in the selected tropical peatland in Vietnam with fragments being the most common morphology. They utilized FT–IR and microscope in order to recognize the aforementioned morphologies. In the collected peat samples, fragments (67.0%) and films (24.6%) are the two most prevalent microplastic shapes, followed by fibers (7.6%) and foams (0.9%). The low number of fibrous particles may be attributed to the resuspension of fibrous microplastics due to wind/water erosion. Nguyen made assumptions about the origins of the detected microplastics. The primary source of the film may be plastic bags and/or packing supplies but the presence of fragment microplastics can be attributed to the collapse, strain, exposure to UV or sunlight and other factors, which affect larger plastic waste. In comparison with other environments, the results indicated higher levels of fragments. For instance, Chia et al. (2021) stated that the two most common microplastic forms found in groundwater and soil are fibers and pellets.
On the contrary, Allen et al. (2019) showed that fiber particles were dominant in selected the ombrotrophic peatland in the central Pyrenees. The higher relative peat surface fiber counts were thought to be explained by the possibility that fibers would travel more slowly or get caught in the catchment vegetation and soils, as well as by the effective vegetation collection of fibers (and particles) by peat. Moreover, since the field study has been located in a remote catchment, the ubiquity of the fiber particles can imply their long-range transport.
The microplastics particle size was determined by Nguyen et al. (2022) to be between 300 and 5000 μm. The ranges 500–1,000 μm (33.9%) and 300–500 μm (30.1%) had the highest percentage of sample sizes, followed by 1,000–3,000 μm (28.3%) and 3,000–5000 μm (7.7%). They considered the presence of larger particles (3,000–5000 μm) as a sign of human activities (cosmetics, shampoo, personal care products, etc.) and agricultural practices (compost fertilizers, agricultural films, etc).
In the other study, Allen et al. (2021) indicated that over 50% of the peat microplastic particles were smaller than 30 μm. Allen et al. (2019) conducted a previous study on the atmospheric mobility and deposition of microplastics in a remote mountain watershed in the French Pyrenees. The results were consistent with the microplastic size distribution for atmospheric deposition obtained from direct atmospheric sampling for the nearby Pyrenees catchment (>50% microplastic particles <20 μm). The majority of atmospheric deposition is composed of smaller microplastic particles, as indicated by the overall preponderance of ≤30 μm microplastics. Since 1980, the percentage of microplastic less than 20 μm has been trending slightly higher. This might be the result of an augment of human activities, producing smaller microplastic (such as emissions from laundry machines and dryers, inefficient incineration, and agricultural practices) that are released directly into the atmosphere or/and an increase in the amount of macroplastic waste that is lost to the environment and degrades over time (either in situ or during transportation), leading to an increase in microplastic and an increase in atmospheric transport. It should be noted that during the project, lake sediment samples were collected as well. The particle size distribution of the lake core microplastic samples is comparable to that of peat. There was minimal fluctuation in the percentage of microplastics smaller than 20 μm before 1995, a rise in particles smaller than 20 μm after 1995, but a decline in the top sample between 2010 and 2015. There could be a pre- and post-burial difference in this water-sediment interface difference.
Nguyen et al. (2022) illustrated that microplastic concentrations ranged from 10 to 770 items kg−1 in three peatland sites. Veerasingam et al. (2016) and Zhang (2017) have already indicated that the abundance of microplastics displayed significant spatial variability due to both natural and anthropogenic factors influencing the microplastics accumulation and transport. The microplastic spatial variation was also examined in the three peatland areas. The sampling sites in Duc Hue had the lowest concentration of microplastics, ranging from 10 to 30 items kg−1. Tan Thanh had the highest concentration, and these locations are off-limits to home wastewater discharges, construction sites, and agricultural activity areas.
The results of the other investigation by Allen et al. (2021) showed that the top half of the single peat core had the highest microplastic content, with a deposition rate of 178 (±79) MP/m2/day. More importantly, the amount of microplastic diminished with depth; samples dated between 1940 and 1960 had minuscule levels of microplastic particles (1–2 MP/sample, ≤1 MP/m2/day). The sample periods with the greatest changes in the rate of microplastic deposition were 1980–2000 and 2015–2020. The highest percentage of microplastic fibers (>30% of all microplastics) was found in the top peat sample, and it decreased to ≤20% in the samples from 1960 to 1980. The cause of this depletion may be attributed to atmospheric movement and deposition of the plastic particles or the degradation (chemical, UV, mechanical) of microplastics in the peat. Therefore, it can be deduced that the proportion of fiber microplastics declines with the increase in depth of the collected samples. It should be noticed that during the project, lake sediment samples collected as well. A comparable decline with depth was observed in the microplastic numbers in the lake samples taken from the single lake core. However, there were a number of differences between the peat and the lake samples. Microplastic counts may also be related to changes in the plant cover (1990–2020), which resulted in an increase in shrub and heathland and an increase in the filtration function of vegetation. Only a portion of the catchment microplastic may be deposited in the lake’s sediment by microplastics that float on the lake’s surface and discharge downstream. In general, Nguyen et al. (2022) found a substantial correlation between the categories of microplastics and activities connected to humans.
Additionally, machine learning techniques were proposed by Tran et al. (2023) to forecast microplastic pollution in peatland areas. They investigated the possibility of forecasting, using readily measurable parameters in the samples, the amounts of microplastics in samples of peatland sediments. In order to predict the composite quantity of the plastic particles, three machine learning models (LS-SVM, RF, and LSTM) were created and evaluated using the sediment features and physicochemical parameters that were found in the samples as predictors. In addition, associations between the amounts of microplastics in the samples and the physicochemical characteristics were evaluated through the use of correlation and Bayesian network analysis (Tran et al., 2023). Table 3 summarizes the behavior of microplastics in the studies areas.
FT-IR analysis was utilized by Nguyen et al. (2022) to ascertain the microplastics polymeric composition in peat samples. There were fifteen distinct types of polymers. According to the FT–IR results, PVC (n = 265, 46.2%) and PE (n = 120, 20.9%) account for the largest proportion of microplastics. These are followed by PP (n = 53, 9.2%), PVCA (n = 38, 6.6%), EPDM (n = 28, 4.9%), DINCH (n = 26, 4.5%), and others. As demonstrated by the results, the location had an impact on the chemical composition of the microplastics that were found. For instance, PVC (n = 228, 49.5%) was the sample type with the highest proportion among those analyzed in the Tan Thanh area. It is followed by PE (n = 103, 22.3%) and PP (n = 52, 11.3%), whereas VC (n = 44, 77.2%) was the predominant polymer for microplastics in Thanh Hoa, followed by PVCA (n = 6, 10.5%). Furthermore, HNBR and PVC (a total of 85.7%) were the most prevalent in Duc Hue. Andrady and Neal (2009) expressed that PVC is considerably utilized in industrial processes and that its polymers are detected in a wide range of everyday items and technical products. PE, PP, and PS, on the other hand, are frequently utilized in food packaging, including jars, bottles, films, and reusable bags. These plastic materials are also utilized in quickly-used disposable items (Liu et al., 2019).
In addition, the most prevalent compositions found in the peat core samples by Allen et al. (2021) were polypropylene (PP), polyethylene terephthalate (PET), polyethylene (PE), polystyrene (PS), and polyvinyl chloride (PVC). The study revealed a general downward trend for PE, PET, PP, and PVC with depth. This suggests that the atmospheric deposition of these polymers has been steadily increasing over time, which is in line with the expanding production and utilization of plastic. The polymer types that make up the sample microplastics are complicated mixtures. The post-1980 samples contain plastics such as acrylonitrile butadiene styrene (ABS), polycarbonate (PC), ethylene vinyl acetate (EVA), and others. This is consistent with the development and marketing of high-performance plastics after the 1980s as well as the marketing of affordable plastic homecare and personal electronics. Similar to the peat archive, the lake samples showed a falling trend in the prevalence of particular polymers and sample complexity with depth. PE and PET exhibited a steadily declining trend with depth, but PS and PP showed a small increase in incidence between 1990 and 1995. In general, the peat and lake archives demonstrate an increase in the quantity of all plastic polymers throughout time, along with a noted complexity in their composition.
Moreover, determing the composition of microplastics can be beneficial when it comes to assessing the residence time of microplastics. IT must be mentioned that the lower the density of plastic particles is the higher the residence time of them in the atmosphere since compared to less dense microplastics it takes denser particles more time to deposit. A number of studies have examined the residence time of microplastics in different environments. For instance, Trainic et al. (2020) evaluated the residence time of airborne microplastics which had been detected in a specific trajectory in order to scrutinize the open ocean as a source of airborne microplastics. They compared the chemical components of the airborne microplastics with microplastic particles extracted from seawater obtained at a similar approximate location to the AMP samples in order to further investigate the role of the open ocean as a source of Airborne microplastics. About 20% of their air samples contained atmospheric microplastics. Since they were unable to test airborne microplastic particles smaller than 5 µm due to present technical constraints, they hypothesize that these results understate the real airborne microplastic content in the marine atmosphere. Moreover, methodological constraints in nanoplastic gathering and detection have resulted in the relatively recent identification of nanoplastic particles (<1 µm) at the single particle level in the environment. The type pf plastic particles were determined by micro-Raman spectroscopy and their densities, diameters and terminal settling velocities were also examined. Different types of microplastics on different filters showed difeerent lifetimes. The lowest residence time belonged to the detected Polystyrerne on one of the filters (2) with only 5 h. On the contrary, Polypropylene had the highest lifetime (2 days). By comparing the two plastic type, it can be understood that Polypropylene had the lowest terminal velocity and biggest size of major and minor axis. As mentioned before, Polypropylene was the highest proportion of microplastics which was detected by Allen et al. (2021). As mentioned before Allen et al. (2021) indicated that polypropylene was the dominant type of plastic particles in central Pyrenees. The density of polypropylene is estimated within the range of 0.86–0.95 g cm-3, which increases the capability of PP particles of being transported from distant sources, which also implies higher residence time.
To our best knowledge, only one study has investigated the consequences of the presence of microplastics in peatland areas and the details have been provided by Nguyen et al. (2023). Nguyen et al. (2023) indicated that polyvinyl chloride, polyethylene, and polypropylene are the main sources of microplastics in the peatlands of Long An province, Mekong Delta, which have been produced by human activities. The entire peatland region was categorized as being at extreme risk. Notably, the findings showed a strong positive association (r = 0.49, 0.69, and 0.43, respectively) between the degree of human activity and the ecological risk indices HI, PLI, and RI. Appropriate strategies have been put forth to address current issues and encourage long-term peatland area protection. Using alternative materials like bioplastics, cutting back on single-use plastic products (SUPs), and adhering to the 4Rs principle are important additional strategies. The SWOT framework has been used to identify obstacles in the way of removing microplastic pollution and putting specific priority actions into action.
Overall, despite some geographical limitations, ombrotrophic peatlands can be considered as efficient atmospheric microplastic deposition collectors. However, the effect of vegetation type and general characteristics of the peat on the behavior of plastic particles is still ambiguous. These parameters (vegetation type and other characteristics of the studied peatland) can be considered as limiting factors when it comes to evaluating peatlands as natural archives of airborne microplastics. Optimum sampling methods and pretreatment processes were proposed. However, the lack of an integrated sampling and treatment process may be an obstacle in preparing peat samples. Therefore, the existence of a consistent process is essential while examining peatland microplastics. The dating techniques and their importance were also discussed and the proposed technique was introduced.
Despite the fact that the availability of field studies makes it difficult to draw a comprehensive conclusion, the demonstrated efficacy of peatlands as natural archives for other pollutants, coupled with the limited number of studies on the ability of peatlands to retain microplastic particles, suggests that peatlands may serve as an ideal choice for documenting accumulation and deposition rates of microplastic particles in different time intervals. Furthermore, the global distribution and abundance of peatlands provide additional justification for their utilization as natural archives for microplastics. It is essential to consider that the regulation of inflow to peatlands significantly influences their effectiveness as natural archives. Accordingly, among the various types of peatlands, ombrotrophic peatlands appear to be a more logical option, as they are exclusively fed by atmospheric precipitation. The climatic conditions of the study area appear to significantly influence the progression of the research process. In regions characterized by lower peat depths, the application of the Wardenaar peat corer for sampling surface layers warrants consideration and further investigation. A variety of methodologies may be employed for dating. Investigations into the presence of microplastics in peatlands have utilized 210Pb dating. Determining the optimal technique for 210Pb dating requires further research.
It is imperative to undertake additional field studies to elucidate the distribution and dispersion mechanisms of microplastic particles within peatland ecosystems. Additionally, the role of vegetation and the diverse attributes of peatlands in influencing the adsorption and distribution of microplastic particles warrants thorough examination. The majority of existing research has predominantly focused on ombrotrophic peatlands. Investigating non-atmospheric inflow of microplastic particles in peatlands necessitates further research focusing on their presence in minerotrophic peatlands, a critical area requiring detailed examination by researchers. Addressing the aforementioned aspects necessitates conducting studies across diverse climatic conditions. Ultimately, determining the chronological framework of microplastic particles represents a pivotal component of such studies. At present, employing 210Pb dating appears to be a logical and reliable method for establishing the temporal presence of microplastic particles within peatlands. However, an important question arises: is it feasible to utilize the microplastic particles themselves as a tool for dating peat samples? What are the most effective methodologies for dating within this area of investigation?
AR: Conceptualization, Data curation, Investigation, Methodology, Software, Visualization, Writing–original draft, Writing–review and editing. SA: Conceptualization, Data curation, Funding acquisition, Investigation, Methodology, Project administration, Resources, Software, Supervision, Validation, Visualization, Writing–review and editing. ND: Investigation, Validation, Visualization, Writing–review and editing. RD: Investigation, Validation, Visualization, Writing–review and editing.
The author(s) declare that financial support was received for the research, authorship, and/or publication of this article. This project has received funding from the Research Council of Lithuania (LMTLT), agreement No Nr. S-MIP-23-139.
The authors declare that the research was conducted in the absence of any commercial or financial relationships that could be construed as a potential conflict of interest.
The authors declare that no Generative AI was used in the creation of this manuscript.
All claims expressed in this article are solely those of the authors and do not necessarily represent those of their affiliated organizations, or those of the publisher, the editors and the reviewers. Any product that may be evaluated in this article, or claim that may be made by its manufacturer, is not guaranteed or endorsed by the publisher.
Abbasi, S., Turner, A., Hoseini, M., and Amiri, H. (2021). Microplastics in the lut and kavir deserts, Iran. Environ. Sci. and Technol. 55 (9), 5993–6000. doi:10.1021/acs.est.1c00615
Allen, D., Allen, S., Abbasi, S., Baker, A., Bergmann, M., Brahney, J., et al. (2022). Microplastics and nanoplastics in the marine-atmosphere environment. Nat. Rev. Earth and Environ. 3 (6), 393–405. doi:10.1038/s43017-022-00292-x
Allen, D., Allen, S., Le Roux, G., Simonneau, A., Galop, D., and Phoenix, V. R. (2021). Temporal archive of atmospheric microplastic deposition presented in ombrotrophic peat. Environ. Sci. and Technol. Lett. 8 (11), 954–960. doi:10.1021/acs.estlett.1c00697
Allen, S., Dušan, M., Deonie, A., Anna, M., Rupert, H., Gael, L. R., et al. (2022). An early comparison of nano to microplastic mass in a remote catchment's atmospheric deposition. J. Hazard. Mater. Adv. 7, 100104. doi:10.1016/j.hazadv.2022.100104
Allen, S., Phoenix, V. R., Roux, G. L., Pilar, D. J., Anaëlle, S., Binet, S., et al. (2019). Atmospheric transport and deposition of microplastics in a remote mountain catchment. Nat. Geosci. 12 (5), 339–344. doi:10.1038/s41561-019-0335-5
Andrady, A. L., and Neal., M. A. (2009). Applications and societal benefits of plastics. Philosophical Trans. R. Soc. B Biol. Sci. 364, 1977–1984.
Appleby, P. G., Nolan, P. J., Oldfield, F., Richardson, N., and Higgitt, S. R. (1988). 210Pb dating of lake sediments and ombrotrophic peats by gamma essay. Sci. total Environ. 69, 157–177. doi:10.1016/0048-9697(88)90341-5
Arthur, C., Baker, J., and Bamford, H. (2009). Proceedings of the international research workshop on the occurrence, effects and fate of microplastic marine debris. NOAA Technical Memorandum NOS-OR&R-30, 49.
Bandekar, M., Abdolahpur Monikh, F., Kekäläinen, J., Tahvanainen, T., Kortet, R., Zhang, P., et al. (2022). Submicron plastic adsorption by peat, accumulation in sphagnum mosses and influence on bacterial communities in peatland ecosystems. Environ. Sci. and Technol. 56 (22), 15661–15671. doi:10.1021/acs.est.2c04892
Biswas, T., and Pal, S. C. (2024). Emerging threats of microplastics on marine environment: a critical review of toxicity measurement, policy practice gap and future research direction. J. Clean. Prod. 434, 139941. doi:10.1016/j.jclepro.2023.139941
Black, W. A. P., Cornhill, W. J., and Woodward, E. F. (1955). A preliminary investigation on the chemical composition of Sphagnum moss and peat. J. Appl. Chem. 5 (9), 484–492. doi:10.1002/jctb.5010050907
Boots, B., Russell, C. W., and Senga Green, D. (2019). Effects of microplastics in soil ecosystems: above and below ground. Environ. Sci. and Technol. 53 (19), 11496–11506. doi:10.1021/acs.est.9b03304
Brahney, J., Hallerud, M., Heim, E., Hahnenberger, M., and Sukumaran, S. (2020). Plastic rain in protected areas of the United States. Science 368 (6496), 1257–1260. doi:10.1126/science.aaz5819
Burton, R. G. O., and Hodgson, J. M. (1987). Lowland peat in england and wales. Lawes Agricultural Trust (Soil Survey of England and Wales.
Catarino, A. I., Thompson, R., Sanderson, W., and Henry, T. B. (2017). Development and optimization of a standard method for extraction of microplastics in mussels by enzyme digestion of soft tissues. Environ. Toxicol. Chem. 36 (4), 947–951. doi:10.1002/etc.3608
Chen, B., Krachler, M., and Shotyk, W. (2003). Determination of antimony in plant and peat samples by hydride generation-atomic fluorescence spectrometry (HG-AFS). J. Anal. Atomic Spectrom. 18 (10), 1256–1262. doi:10.1039/b306597a
Chen, B., Zhang, Z., Wang, T., Hu, H., Qin, G., Lu, T., et al. (2023). Global distribution of marine microplastics and potential for biodegradation. J. Hazard. Mater. 451, 131198. doi:10.1016/j.jhazmat.2023.131198
Chen, H., Zou, X., Ding, Y., Wang, Y., Fu, G., and Yuan, F. (2022). Are microplastics the ‘technofossils’ of the Anthropocene? Anthr. Coasts 5 (1), 8. doi:10.1007/s44218-022-00007-1
Chia, R. W., Lee, J. Y., Kim, H., and Jang, J. (2021). Microplastic pollution in soil and groundwater: a review. Environ. Chem. Lett. 19 (6), 4211–4224. doi:10.1007/s10311-021-01297-6
Coggins, A. M., Jennings, S. G., and Ebinghaus, R. (2006). Accumulation rates of the heavy metals lead, mercury and cadmium in ombrotrophic peatlands in the west of Ireland. Atmos. Environ. 40 (2), 260–278. doi:10.1016/j.atmosenv.2005.09.049
Cole, M., Lindeque, P., Halsband, C., and Galloway, T. S. (2011). Microplastics as contaminants in the marine environment: a review. Mar. Pollut. Bull. 62 (12), 2588–2597. doi:10.1016/j.marpolbul.2011.09.025
Courtene-Jones, W., Quinn, B., Murphy, F., Gary, S. F., and Narayanaswamy, B. E. (2017). Optimisation of enzymatic digestion and validation of specimen preservation methods for the analysis of ingested microplastics. Anal. Methods 9 (9), 1437–1445. doi:10.1039/c6ay02343f
Cuttle, S. P., and Malcolm, D. C. (1979). A corer for taking undisturbed peat samples. Plant soil 51, 297–300. doi:10.1007/bf02232893
Dehaut, A., Cassone, A. L., Frère, L., Hermabessiere, L., Himber, C., Rinnert, E., et al. (2016). Microplastics in seafood: benchmark protocol for their extraction and characterization. Environ. Pollut. 215, 223–233. doi:10.1016/j.envpol.2016.05.018
Dutta, A. (2017). Fourier transform infrared spectroscopy. Spectrosc. methods Nanomater. Charact., 73–93. doi:10.1016/b978-0-323-46140-5.00004-2
Eerkes-Medrano, D., Thompson, R. C., and Aldridge, D. C. (2015). Microplastics in freshwater systems: a review of the emerging threats, identification of knowledge gaps and prioritisation of research needs. Water Res. 75, 63–82. doi:10.1016/j.watres.2015.02.012
Esiukova, E., Khatmullina, L., Lobchuk, O., Grave, A., Kileso, A., Haseler, M., et al. (2020). From macro to micro: dataset on plastic contamination along and across a sandy tide-less coast (the Curonian Spit, the Baltic Sea). Data brief 30, 105635. doi:10.1016/j.dib.2020.105635
Fahrenfeld, N. L., Arbuckle-Keil, G., Beni, N. N., and Bartelt-Hunt, S. L. (2019). Source tracking microplastics in the freshwater environment. TrAC Trends Anal. Chem. 112, 248–254. doi:10.1016/j.trac.2018.11.030
Fracasso, I., Zaccone, C., Oskolkov, N., Da Ros, L., Dinella, A., Marchesini, L. B., et al. (2024). Exploring different methodological approaches to unlock paleobiodiversity in peat profiles using ancient DNA. Sci. Total Environ. 908, 168159. doi:10.1016/j.scitotenv.2023.168159
François, D. V., Chambers, F. M., and Swindles, G. T. (2010). Coring and sub-sampling of peatlands for palaeoenvironmental research. Mires peat 7, 10.
Gabov, D., Yakovleva, E., and Vasilevich, R. (2020). Vertical distribution of PAHs during the evolution of permafrost peatlands of the European arctic zone. Appl. Geochem. 123, 104790. doi:10.1016/j.apgeochem.2020.104790
Gabrielli, P., and Vallelonga, P. (2015). “Contaminant records in ice cores,” in Environmental contaminants. Developments in paleoenvironmental research. Editors J. Blais, M. Rosen, and J. Smol (Dordrecht: Spriger), 18, 393–430. doi:10.1007/978-94-017-9541-8_14
Gasperi, J., Wright, S. L., Dris, R., Collard, F., Mandin, C., Guerrouache, M., et al. (2018). Microplastics in air: are we breathing it in? Curr. Opin. Environ. Sci. and Health 1, 1–5. doi:10.1016/j.coesh.2017.10.002
Gilbert, D., and Mitchell, E. A. (2006). Microbial diversity in Sphagnum peatlands. Dev. earth Surf. Process. 9, 287–318.
Givelet, N., Le Roux, G., Cheburkin, A., Chen, B., Frank, J., Goodsite, M. E., et al. (2004b). Suggested protocol for collecting, handling and preparing peat cores and peat samples for physical, chemical, mineralogical and isotopic analyses. J. Environ. Monit. 6 (5), 481–492. doi:10.1039/b401601g
Givelet, N., Roos-Barraclough, F., Goodsite, M. E., Cheburkin, A. K., and Shotyk, W. (2004a). Atmospheric mercury accumulation rates between 5900 and 800 calibrated years BP in the High Arctic of Canada recorded by peat hummocks. Environ. Sci. and Technol. 38 (19), 4964–4972. doi:10.1021/es035293l
Habibi, N., Uddin, S., Fowler, S. W., and Behbehani, M. (2022). Microplastics in the atmosphere: a review. J. Environ. Expo. Assess. 1 (6), 10–20517. doi:10.20517/jeea.2021.07
Hagelskjaer, O., Le Roux, G., and Sonke, J. E. (2023a). “Using ombrotrophic peat archives to determine the evolution of atmospheric microplastic deposition patterns,” in Goldschmidt 2023 conference (GOLDSCHMIDT).
Hagelskjær, O., Le Roux, G., Liu, R., Dubreuil, B., Behra, P., and Sonke, J. E. (2023b). The recovery of aerosol-sized microplastics in highly refractory vegetal matrices for identification by automated Raman microspectroscopy. Chemosphere 328, 138487. doi:10.1016/j.chemosphere.2023.138487
Hajdas, I., Ascough, P., Garnett, M. H., Fallon, S. J., Pearson, C. L., Quarta, G., et al. (2021). Radiocarbon dating. Nat. Rev. Methods Prim. 1 (1), 62. doi:10.1038/s43586-021-00058-7
Hansson, S. V., Bindler, R., and De Vleeschouwer, F. (2015). Using peat records as natural archives of past atmospheric metal deposition. in Environmental Contaminants, 323–354. doi:10.1007/978-94-017-9541-8_12
Harmon, S. M., Chen, Q., Ma, C., Ji, M., Yan, X., Ji, R., et al. (2024). “The effects of microplastic pollution on aquatic organisms,” in Microplastic contamination in aquatic environments (Elsevier), 355–379.
Hoang, V. H., Nguyen, M. K., Hoang, T. D., Ha, M. C., Huyen, N. T. T., Bui, V. K. H., et al. (2024). Sources, environmental fate, and impacts of microplastic contamination in agricultural soils: a comprehensive review. Sci. Total Environ. 950, 175276. doi:10.1016/j.scitotenv.2024.175276
Hooijer, A., Silvius, M., Wösten, H., and Page, S. (2006). “PEAT-CO2. Assessment of CO2 emissions from drained peatlands in SE Asia,” in IPCC: climate change, 2007: the physical science basis, Contribution of working group I to the fourth assessment report of the intergovernmental panel on climate change. Editors S. Solomon, D. Qin, M. Manning, Z. Chen, M. Marquis, K. B. Averytet al. (Cambridge, UK, New York, USA: Cambridge University Press), 996.
Hu, K., Yang, Y., Zuo, J., Tian, W., Wang, Y., Duan, X., et al. (2022). Emerging microplastics in the environment: properties, distributions, and impacts. Chemosphere 297, 134118. doi:10.1016/j.chemosphere.2022.134118
Hughes, P. D., Mallon, G., Brown, A., Essex, H. J., Stanford, J. D., and Hotes, S. (2013). The impact of high tephra loading on late-Holocene carbon accumulation and vegetation succession in peatland communities. Quat. Sci. Rev. 67, 160–175. doi:10.1016/j.quascirev.2013.01.015
Joosten, H., and Clarke, D. (2002). Wise use of mires and peatlands. in Int. mire conservation group Int. peat Soc. 304.
Joshi, K. B., Goswami, V., Banerji, U. S., and Shankar, R. (2021). Recent developments in instrumentation and its application in absolute Dating: historical perspective and overview. J. Asian Earth Sci. 211, 104690. doi:10.1016/j.jseaes.2021.104690
Karami, A., Golieskardi, A., Choo, C. K., Romano, N., Ho, Y. B., and Salamatinia, B. (2017). A high-performance protocol for extraction of microplastics in fish. Sci. total Environ. 578, 485–494. doi:10.1016/j.scitotenv.2016.10.213
Kierdorf, U., Ludolphy, C., and Kierdorf, H. (2022). Antlers as bioindicators of environmental pollution: principles, achievements, and future research directions. Animal Prod. Sci. 63, 1594–1606. doi:10.1071/an22145
Kromer, B., and Münnich, K. O. (1992). CO2 gas proportional counting in radiocarbon dating—review and perspective. Radiocarbon after four decades: An interdisciplinary perspective, 184–197.
Krachler, M., Burow, M., and Emons, H. (1999). Development and evaluation of an analytical procedure for the determination of antimony in plant materials by hydride generation atomic absorption spectrometry. Analyst 124 (5), 777–782. doi:10.1039/a900437h
Kutschera, W. (2016). Accelerator mass spectrometry: state of the art and perspectives. Adv. Phys. X 1 (4), 570–595. doi:10.1080/23746149.2016.1224603
Kylander, M. E., Weiss, D. J., Varela, E. P., Rodriguez, T. T., and Cortizas, A. M. (2006). Archiving natural and anthropogenic lead deposition in peatlands. Dev. earth Surf. Process. 9, 479–497.
Lamentowicz, M., Tobolski, K., and Mitchell, E. A. (2007). Palaeoecological evidence for anthropogenic acidification of a kettle-hole peatland in northern Poland. Holocene 17 (8), 1185–1196. doi:10.1177/0959683607085123
Lavoie, C. (2013). Biological collections in an ever changing world: herbaria as tools for biogeographical and environmental studies. Perspect. Plant Ecol. Evol. Syst. 15 (1), 68–76. doi:10.1016/j.ppees.2012.10.002
Lennartz, B., and Liu, H. (2019). Hydraulic functions of peat soils and ecosystem service. Front. Environ. Sci. 7, 92. doi:10.3389/fenvs.2019.00092
Limpens, J., Berendse, F., Blodau, C., Canadell, J. G., Freeman, C., Holden, J., et al. (2008). Peatlands and the carbon cycle: from local processes to global implications–a synthesis. Biogeosciences 5 (5), 1475–1491. doi:10.5194/bg-5-1475-2008
Litherland, A. E., Zhao, X. L., and Kieser, W. E. (2011). Mass spectrometry with accelerators. Mass Spectrom. Rev. 30 (6), 1037–1072. doi:10.1002/mas.20311
Liu, F., Olesen, K. B., Borregaard, A. R., and Vollertsen, J. (2019). Microplastics in urban and highway stormwater retention ponds. Sci. Total Environ. 671, 992–1000. doi:10.1016/j.scitotenv.2019.03.416
Luo, N., Yu, R., Wen, B., Li, X., Liu, X., and Li, X. (2023). Identifying anthropogenic sources of heavy metals in alpine peatlands over the past 150 Years: examples from typical peatlands in altay mountains, northwest China. Int. J. Environ. Res. Public Health 20 (6), 5013. doi:10.3390/ijerph20065013
Malawska, M., and Ekonomiuk, A. (2008). The use of wetlands for the monitoring of non-point source air pollution. Pol. J. Environ. Stud. 17 (1).
Marcisz, K., Kołaczek, P., Gałka, M., Diaconu, A. C., and Lamentowicz, M. (2020). Exceptional hydrological stability of a Sphagnum-dominated peatland over the late Holocene. Quat. Sci. Rev. 231, 106180. doi:10.1016/j.quascirev.2020.106180
Mbachu, O., Jenkins, G., Pratt, C., and Kaparaju, P. (2021). Enzymatic purification of microplastics in soil. MethodsX 8, 101254. doi:10.1016/j.mex.2021.101254
Michéli, E., Schad, P., Spaargaren, O., Dent, D., and Nachtergale, F. (2006). World reference base for soil resources 2006. World soil Resour. Rep. 103, 1–128.
Minayeva, T., Sirin, A., Kershaw, P., and Bragg, O. (2018). Arctic peatlands. The Wetland Book II: Distribution, Description, and Conservation.
Mishra, A. K., Singh, J., and Mishra, P. P. (2021). Microplastics in polar regions: an early warning to the world's pristine ecosystem. Sci. Total Environ. 784, 147149. doi:10.1016/j.scitotenv.2021.147149
Miszczak, E., Stefaniak, S., Michczyński, A., Steinnes, E., and Twardowska, I. (2020). A novel approach to peatlands as archives of total cumulative spatial pollution loads from atmospheric deposition of airborne elements complementary to EMEP data: priority pollutants (Pb, Cd, Hg). Sci. Total Environ. 705, 135776. doi:10.1016/j.scitotenv.2019.135776
Montanari, S. (2018). Cracking the egg: the use of modern and fossil eggs for ecological, environmental and biological interpretation. R. Soc. Open Sci. 5 (6), 180006. doi:10.1098/rsos.180006
Munno, K., Helm, P. A., Jackson, D. A., Rochman, C., and Sims, A. (2018). Impacts of temperature and selected chemical digestion methods on microplastic particles. Environ. Toxicol. Chem. 37 (1), 91–98. doi:10.1002/etc.3935
Naidoo, T., Goordiyal, K., and Glassom, D. (2017). Are nitric acid (HNO3) digestions efficient in isolating microplastics from juvenile fish? Water, Air, and Soil Pollut. 228, 470–511. doi:10.1007/s11270-017-3654-4
Nguyen, M. K., Lin, C., Hung, N. T. Q., Vo, D. V. N., Nguyen, K. N., Thuy, B. T. P., et al. (2022). Occurrence and distribution of microplastics in peatland areas: a case study in Long an province of the Mekong Delta, Vietnam. Sci. Total Environ. 844, 157066. doi:10.1016/j.scitotenv.2022.157066
Nguyen, M. K., Lin, C., Hung, N. T. Q., Hoang, H. G., Vo, D. V. N., and Tran, H. T. (2023). Investigation of ecological risk of microplastics in peatland areas: a case study in Vietnam. Environ. Res. 220, 115190. doi:10.1016/j.envres.2022.115190
Nguyen, M. K., Rakib, M. R. J., Lin, C., Hwangbo, M., and Kim, J. (2024). Is micro (nano) plastic contamination in wet atmospheric deposition a prominent issue requiring heightened attention? J. Hazard. Mater. 476, 135027. doi:10.1016/j.jhazmat.2024.135027
Nørnberg, T., Goodsite, M. E., and Shotyk, W. (2004). An improved motorized corer and sample processing system for frozen peat. Arctic 57, 242–246. doi:10.14430/arctic501
Nunes, B. Z., Huang, Y., Ribeiro, V. V., Wu, S., Holbech, H., Moreira, L. B., et al. (2023). Microplastic contamination in seawater across global marine protected areas boundaries. Environ. Pollut. 316, 120692. doi:10.1016/j.envpol.2022.120692
O'Brien, S., Rauert, C., Ribeiro, F., Okoffo, E. D., Burrows, S. D., O’Brien, J. W., et al. (2023). There's something in the air: a review of sources, prevalence and behaviour of microplastics in the atmosphere. Sci. Total Environ. 874, 162193. doi:10.1016/j.scitotenv.2023.162193
Okubo, R., Yamamoto, A., Kurima, A., Sakabe, T., Ide, Y., and Isobe, A. (2023). Estimation of the age of polyethylene microplastics collected from oceans: application to the western North Pacific Ocean. Mar. Pollut. Bull. 192, 114951. doi:10.1016/j.marpolbul.2023.114951
Oldfield, F., Richardson, N., and Appleby, P. G. (1995). Radiometric dating (210Pb, 137Cs, 241Am) of recent ombrotrophic peat accumulation and evidence for changes in mass balance. Holocene 5 (2), 141–148. doi:10.1177/095968369500500202
Oldfield, F., Richardson, N., Clymo, R. S., Appleby, P. G., Pearson, G. W., and Ratnesar, P. (1990). The record of atmospheric deposition on a rainwater-dependent peatland. Philosophical Trans. R. Soc. Lond. B, Biol. Sci. 327 (1240), 331–338.
Ortiz, J. E., Sánchez-Palencia, Y., Gallego, J. L., Borrego, Á. G., Baragaño, D., and Torres, T. (2023). Deposition of atmospheric polycyclic aromatic hydrocarbons in rural areas: current data and historical record from an ombrotrophic peatland. Int. J. Coal Geol. 268, 104199. doi:10.1016/j.coal.2023.104199
Osman, A. I., Hosny, M., Eltaweil, A. S., Omar, S., Elgarahy, A. M., Farghali, M., et al. (2023). Microplastic sources, formation, toxicity and remediation: a review. Environ. Chem. Lett. 21 (4), 2129–2169. doi:10.1007/s10311-023-01593-3
Outridge, P. M., Goodsite, M. E., Bennike, O., Rausch, N., and Shotyk, W. (2016). Seabird transfer of nutrients and trace elements from the north water polynya to land during the mid-holocene warm period, Carey Islands, northwest Greenland + supplementary appendix figure S1 (see article tools). Arctic 69, 253–265. doi:10.14430/arctic4577
Parish, F., Sirin, A. A., Charman, D., Joosten, H., Minaeva, T. Y., and Silvius, M. (2008). Assessment on peatlands, biodiversity and climate change.
Parolini, M., Stucchi, M., Ambrosini, R., and Romano, A. (2023). A global perspective on microplastic bioaccumulation in marine organisms. Ecol. Indic. 149, 110179. doi:10.1016/j.ecolind.2023.110179
Prata, J. C., da Costa, J. P., Girão, A. V., Lopes, I., Duarte, A. C., and Rocha-Santos, T. (2019). Identifying a quick and efficient method of removing organic matter without damaging microplastic samples. Sci. total Environ. 686, 131–139. doi:10.1016/j.scitotenv.2019.05.456
Radford, F., Zapata-Restrepo, L. M., Horton, A. A., Hudson, M. D., Shaw, P. J., and Williams, I. D. (2021). Developing a systematic method for extraction of microplastics in soils. Anal. Methods 13 (14), 1695–1705. doi:10.1039/d0ay02086a
Reimer, P. J., Brown, T. A., and Reimer, R. W. (2004). Discussion: reporting and calibration of post-bomb 14C data. Radiocarbon 46 (3), 1299–1304.
Roos-Barraclough, F., Givelet, N., Martinez-Cortizas, A., Goodsite, M. E., Biester, H., and Shotyk, W. (2002). An analytical protocol for the determination of total mercury concentrations in solid peat samples. Sci. total Environ. 292 (1-2), 129–139. doi:10.1016/s0048-9697(02)00035-9
Schmitt, C. J., Cook, J. A., Zamudio, K. R., and Edwards, S. V. (2019). Museum specimens of terrestrial vertebrates are sensitive indicators of environmental change in the Anthropocene. Philosophical Trans. R. Soc. B 374 (1763), 20170387. doi:10.1098/rstb.2017.0387
Shotyk, W., Goodsite, M. E., Roos-Barraclough, F., Frei, R., Heinemeier, J., Asmund, G., et al. (2003). Anthropogenic contributions to atmospheric Hg, Pb and as accumulation recorded by peat cores from southern Greenland and Denmark dated using the 14C “bomb pulse curve”. Geochimica Cosmochimica Acta 67 (21), 3991–4011. doi:10.1016/s0016-7037(03)00409-5
Smith, P., Poch, R. M., Lobb, D. A., Bhattacharyya, R., Alloush, G., Eudoxie, G. D., et al. (2024). Status of the world's soils. Annu. Rev. Environ. Resour. 49, 73–104. doi:10.1146/annurev-environ-030323-075629
Souter, L., and Watmough, S. A. (2016). The impact of drought and air pollution on metal profiles in peat cores. Sci. Total Environ. 541, 1031–1040. doi:10.1016/j.scitotenv.2015.09.137
Sridharan, S., Kumar, M., Singh, L., Bolan, N. S., and Saha, M. (2021). Microplastics as an emerging source of particulate air pollution: a critical review. J. Hazard. Mater. 418, 126245. doi:10.1016/j.jhazmat.2021.126245
Synal, H. A., Jacob, S., and Suter, M. (2000). The PSI/ETH small radiocarbon dating system. Nucl. Instrum. Methods Phys. Res. Sect. B Beam Interact. Mater. Atoms 172 (1-4), 1–7. doi:10.1016/s0168-583x(00)00376-1
Synal, H. A., Schulze-König, T., Seiler, M., Suter, M., and Wacker, L. (2013). Mass spectrometric detection of radiocarbon for dating applications. Nucl. Instrum. Methods Phys. Res. Sect. B Beam Interact. Mater. Atoms 294, 349–352. doi:10.1016/j.nimb.2012.01.026
Talbot, J., Moore, T. R., Wang, M., Dallaire, C. O., and Riley, J. L. (2017). Distribution of lead and mercury in Ontario peatlands. Environ. Pollut. 231, 890–898. doi:10.1016/j.envpol.2017.08.095
Terrazas-López, R., Guadarrama-Guzman, P., Sujitha, S. B., Arreola-Mendoza, L., and Ponniah, J. M. (2024). The occurrence of microplastics in the marine food web in Latin America: insights on the current state of knowledge and future perspectives. Sustainability 16 (14), 5905. doi:10.3390/su16145905
Thompson, R. C., Olsen, Y., Mitchell, R. P., Davis, A., Rowland, S. J., John, A. W., et al. (2004). Lost at sea: where is all the plastic? Science 304 (5672), 838. doi:10.1126/science.1094559
Trainic, M., Flores, J. M., Pinkas, I., Pedrotti, M. L., Lombard, F., Bourdin, G., et al. (2020). Airborne microplastic particles detected in the remote marine atmosphere. Commun. Earth and Environ. 1 (1), 64. doi:10.1038/s43247-020-00061-y
Tran, H. T., Hadi, M., Nguyen, T. T. H., Hoang, H. G., Nguyen, M. K., Nguyen, K. N., et al. (2023). Machine learning approaches for predicting microplastic pollution in peatland areas. Mar. Pollut. Bull. 194, 115417. doi:10.1016/j.marpolbul.2023.115417
Turner, S. D., Horton, A., Rose, N. L., and Hall, C. J. (2019). A temporal sediment record of microplastics in an urban lake, London, UK. J. Paleolimnol. 61, 449–462. doi:10.1007/s10933-019-00071-7
Vallelonga, P., Maffezzoli, N., Saiz-Lopez, A., Scoto, F., Kjær, H. A., and Spolaor, A. (2021). Sea-ice reconstructions from bromine and iodine in ice cores. Quat. Sci. Rev. 269, 107133. doi:10.1016/j.quascirev.2021.107133
Veerasingam, S., Saha, M., Suneel, V., Vethamony, P., Rodrigues, A. C., Bhattacharyya, S., et al. (2016). Characteristics, seasonal distribution and surface degradation features of microplastic pellets along the Goa coast, India. Chemosphere 159, 496–505. doi:10.1016/j.chemosphere.2016.06.056
Walling, C. (1975). Fenton's reagent revisited. Accounts Chem. Res. 8 (4), 125–131. doi:10.1021/ar50088a003
Wang, W., Ge, J., Yu, X., and Li, H. (2020). Environmental fate and impacts of microplastics in soil ecosystems: progress and perspective. Sci. total Environ. 708, 134841. doi:10.1016/j.scitotenv.2019.134841
Wardenaar, E. C. P. (1987). A new hand tool for cutting peat profiles. Can. J. Bot. 65 (8), 1772–1773. doi:10.1139/b87-243
Wheeler, B. D., and Proctor, M. C. F. (2000). Ecological gradients, subdivisions and terminology of north-west European mires. J. Ecol. Essay Rev. 88 (2), 187–203. doi:10.1046/j.1365-2745.2000.00455.x
Williams, B. (2020). Proteinaceous corals as proxy archives of paleo-environmental change. Earth-Science Rev. 209, 103326. doi:10.1016/j.earscirev.2020.103326
Xu, J., Morris, P. J., Liu, J., and Holden, J. (2018). PEATMAP: refining estimates of global peatland distribution based on a meta-analysis. Catena 160, 134–140. doi:10.1016/j.catena.2017.09.010
Yang, L., Zhang, Y., Kang, S., Wang, Z., and Wu, C. (2021). Microplastics in soil: a review on methods, occurrence, sources, and potential risk. Sci. Total Environ. 780, 146546. doi:10.1016/j.scitotenv.2021.146546
Zhang, H. (2017). Transport of microplastics in coastal seas. Estuar. Coast. Shelf Sci. 199, 74–86. doi:10.1016/j.ecss.2017.09.032
Keywords: airborne, archive, transportation, remote, microplastic, nanoplastic
Citation: Rahimpouri A, Abbasi S, Dzingelevičius N and Dzingelevičienė R (2025) Peatlands as geo-archives for atmospheric micro- and nano-plastics: a perspective review. Front. Earth Sci. 13:1514255. doi: 10.3389/feart.2025.1514255
Received: 21 October 2024; Accepted: 31 January 2025;
Published: 06 March 2025.
Edited by:
Patrick G. Hatcher, Old Dominion University, United StatesReviewed by:
Minh Ky Nguyen, Ho Chi Minh City University of Agriculture and Forestry, VietnamCopyright © 2025 Rahimpouri, Abbasi, Dzingelevičius and Dzingelevičienė. This is an open-access article distributed under the terms of the Creative Commons Attribution License (CC BY). The use, distribution or reproduction in other forums is permitted, provided the original author(s) and the copyright owner(s) are credited and that the original publication in this journal is cited, in accordance with accepted academic practice. No use, distribution or reproduction is permitted which does not comply with these terms.
*Correspondence: Sajjad Abbasi, c2FqamFkLmFiYmFzaUBzaGlyYXp1LmFjLmly, c2FqamFkLmFiYmFzaS5oQGdtYWlsLmNvbQ==
†ORCID: Sajjad Abbasi, orcid.org/0000-0002-5194-9334
Disclaimer: All claims expressed in this article are solely those of the authors and do not necessarily represent those of their affiliated organizations, or those of the publisher, the editors and the reviewers. Any product that may be evaluated in this article or claim that may be made by its manufacturer is not guaranteed or endorsed by the publisher.
Research integrity at Frontiers
Learn more about the work of our research integrity team to safeguard the quality of each article we publish.