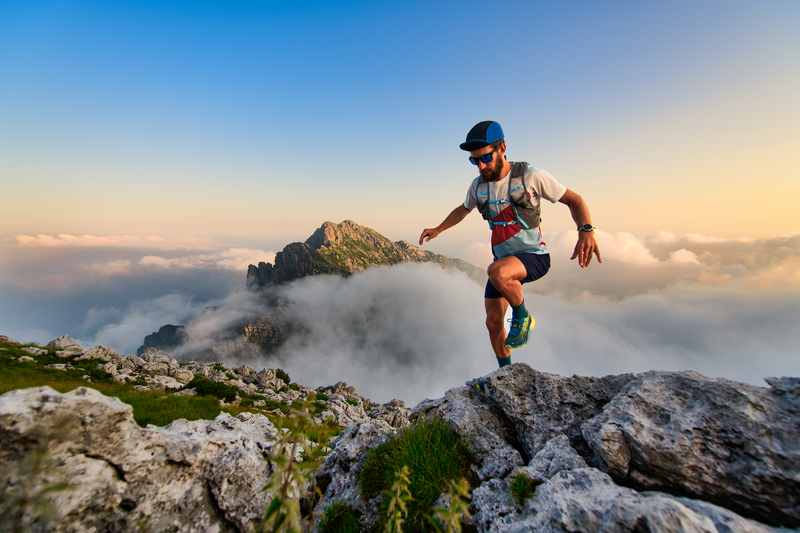
95% of researchers rate our articles as excellent or good
Learn more about the work of our research integrity team to safeguard the quality of each article we publish.
Find out more
ORIGINAL RESEARCH article
Front. Earth Sci. , 17 March 2025
Sec. Marine Geoscience
Volume 13 - 2025 | https://doi.org/10.3389/feart.2025.1400289
Submarine canyon systems are gateways for sediment transport from the shelf to the deep sea. They are the primary conduit for gravity flows that move substantial amounts of carbon, nutrients, and pollutants from the continental shelves to the deep sea. Despite their importance, it often remains unclear how these canyons initiate, and which factors determine their evolution from initiation to mature stage. The Agadir Canyon, located at the Northwest African Continental Margin, represents one of the largest canyon systems worldwide and a conduit for some of the largest turbidity currents on Earth. However, our understanding currently rests on legacy DSDP drilling and poorly resolved seismic imaging of turbidites beyond the canyon mouth. Here, we present multichannel seismic data from proximal and distal reaches of the Agadir Canyon combined with age constraints from the DSDP borehole. This allows us to detail its morphological and temporal evolution from inception to present-day. The Agadir Canyon initiated during the Middle Miocene on top of a preceding wide and shallow channel-levee-system. The Middle to Late Miocene saw the canyon continue eroding and narrowing into its present morphology. The evolution of the canyon was primarily driven by climate perturbations, sea level fluctuations and regional tectonic uplift of the Moroccan hinterland. These factors increased sandy sediment supply to the shelf edge, which promoted powerful erosive turbidity currents. However, most of the canyon fill is slope-derived mud-rich mass transport deposits, which healed the canyon morphology over geologic timescales. Regional salt diapirism, the development of the Canary Islands and the onset of bottom currents during the Miocene actively re-routed sediments and actively shaped its morphology. These competing processes produced the distinct giant canyon morphology seen today: deeply incised (up to 1.2 km) with a flat-bottomed and wide floor (up to 30 km across) that extends for ∼400 km down slope.
Submarine canyon systems are gateways from the coast or continental shelf to the deep abyssal plains. Gravity flows (turbidity currents and debris flows) are funneled through these systems and transport vast quantities of sediment into the deep ocean (Talling et al., 2012). Submarine canyon heads can be “attached” to a terrestrial river source (Anka et al., 2009; Curray et al., 2002; Pirmez and Flood, 1995) or “detached” and fed by longshore transport of sediments across the shelf (Arzola et al., 2008; Covault, 2011; Covault et al., 2007). In general, the incision of submarine canyons is thought to be primarily related to the supply of coarse sediments into the canyon, which feeds powerful and erosive gravity flows (Smith et al., 2018). The controls on sediment supply are principally: 1) hinterland dynamics, including factors such as drainage-basin area, relief, climate, bedrock lithology, which determine the amount and type of sediment being delivered to the shelf (Syvitski et al., 2003), and; 2) sediment routing on the shelf influencing how much and what type of sediment ultimately reaches the canyon (Smith et al., 2018). The interplay of these factors allows canyon systems to be active during sea-level low-stand or high-stand conditions (Burgess and Hovius, 1998; Canals et al., 2006; Covault et al., 2007; Heijnen et al., 2022b; Paull et al., 2010; Pratson et al., 2007). Over long timescales it is challenging to interpret how canyons initiate and evolve due to their size and high degree of stratigraphic incompleteness. Without clear constraints on the age of strata and its relationship to canyon morphology, it is challenging to link canyon evolution with external controls.
The Agadir Canyon forms the most proximal part of the Moroccan Turbidite System, which comprises three interconnected sub-basins: the Agadir Basin, the Seine Abyssal Plain and the Madeira Abyssal Plain (Figure 1). It cuts back into the Moroccan continental shelf, offshore Agadir, where the head region spans 100 km is located between the Tafelny Plateau and Tarfaya Basin along the Moroccan continental margin (Hinz et al., 1982). From there, the canyon extends ∼450 km west, connecting with the Agadir Basin and, in part, the Seine and Madeira Abyssal Plains. The Moroccan Turbidite System has been intensively studied over the past 30 years with extensive acoustic data and >200 short sediment cores that have mapped and investigated turbidites over the past 200 kyr (Frenz et al., 2009; Hunt, 2017; Hunt et al., 2013; Stevenson et al., 2014; Talling et al., 2007; Wynn et al., 2002). Turbidites mapped across the system fall into three categories: 1. Siliciclastic turbidites sourced from the Moroccan Margin; 2. Volcaniclastic turbidites sourced from flank collapses in and around the Canary Islands; and 3. Carbonate-rich turbidites sourced from local failures of hemipelagic sediments such as seamount flank collapses or localized slope failure on the basin margins (Wynn et al., 2003). The system hosts some of the largest turbidite events ever mapped with run-out distances exceeding 2000 km and sediment volumes up to 200 km3 (Stevenson et al., 2014; Wynn et al., 2003).
Figure 1. Combined bathymetric and topographic map of the NW African continental margin and hinterland, including the Agadir Canyon, offshore Morocco. (A) DSDP site locations are marked with a red dot. (B) The Jebel Siroua volcanic region is shown with ages in Ma (Berrahma and Delaloye, 1989). Ages for the individual seamounts of the Canary Island Seamount Province (CISP) are taken from van den Bogaard (2013) and given in million years (Ma). TC = Tributary Channel. Stream orders are calculated with ArcGIS. Colors of streams darken with stream order (increase in order number at confluences of two same order streams) and thus indicate major river systems and catchment areas.
The Agadir Canyon exists and has been stable at least since the Neogene (Ercilla et al., 1998). However, our understanding is either skin deep with shallow penetrating acoustic data and sediment cores from the distal mouth of the canyon (Ercilla et al., 1998; Wynn et al., 2003) or solely based on borehole information from the central part of the canyon, which lacks further spatial information (Seibold, 1982; Uchupi et al., 1976). How the Agadir Canyon developed and contributed to the extensive turbidite system remains poorly understood. Further open questions remain regarding the present-day activity as a function of ongoing seismicity in the Anti-Atlas and High Atlas Mountain ranges, and the interplay between the rivers and contour currents supplying and redistributing sediments from the hinterland on the shelf (Ercilla et al., 1998). Hence, deeper penetrating data constrained by borehole information across the length of the canyon are needed to interpret the canyon’s inception and subsequent evolution.
Here, we use a suite of bathymetric data, multichannel seismic data, and stratigraphic information from a nearby DSDP borehole to map and date the evolving geomorphology of the Agadir Canyon from inception to present day. Distinct packages of sedimentation occur within the canyon over time which characterize distinct steps in its evolution. We link this stepped evolution to changes in environmental conditions and interpret important controlling factors.
The Northwest African passive continental margin offshore Morocco formed 180–170 Ma ago (Late Jurassic) during the opening of the North Atlantic (Davison, 2005; Sahabi et al., 2004). The evolution of the sedimentary record of the Moroccan continental margin has been intensely investigated over the past 5 decades with the seminal DSDP drilling campaign covering the slope and deeper water basins offshore DSDP leg 50 site 415, Figure 1; (Lancelot and Winterer, 1980). During the Paleogene, the Moroccan margin was affected by erosional processes, which drove numerous slope failures, mass-movements, and high-energy sediment gravity flows (Hinz et al., 1982; Ulrich Von Rad and Wissmann, 1982). During the Neogene, the Moroccan hinterland is believed to have become more arid resulting in reduced sediment supply to the shelf, with little terrigenous material transported into the deep-water basins (Sarnthein et al., 1982). During the Quaternary, sedimentation was and is still controlled by glacio-eustatic sea level changes that dictate accommodation along the far-reaching shelf, ocean bottom currents that flow along the continental rise, and sediment gravity flows guided by the abundant canyons and gullies down slope (Ercilla et al., 1998; Sarnthein et al., 1982; Wynn et al., 2000).
The evolution of the Atlas and Anti-Atlas Mountains control hinterland geology and sediment supply into the Agadir Canyon. The Anti-Atlas was repeatedly uplifted due to the convergence of the African and Iberian plates during the Late Triassic to the Middle Jurassic (ca. 160–150 Ma), Late Jurassic, and most of the Early Cretaceous (Gouiza et al., 2017; Uchupi et al., 1976). The High Atlas was mainly formed by two main phases of uplift during the Cenozoic, which also affected the Anti-Atlas Mountain range: First, uplift due to the Oligocene compressive phase, which was followed by a relative tectonic relaxation during the Miocene (Frizon de Lamotte et al., 2009). Second, uplift caused by Alpine collisional tectonics at the Pliocene-Pleistocene boundary and potential mantle upwelling because the tectonic shortening during the Cenozoic is insufficient to explain the high topography of the High Atlas. As much as 1,000 m of topography are likely supported by underlying mantle processes potentially related to hot, thinned, or delaminated lithosphere (Babault et al., 2008; Benabdellouahed et al., 2017; Teixell et al., 2010; Teixell et al., 2005). Both Cenozoic uplift phases were accompanied by increased volcanic activity in the Atlas region (Benabdellouahed et al., 2017), likely fired by underlying mantle upwelling (Teixell et al., 2003; Teixell et al., 2005).
The present-day continental margin shows a narrow continental shelf with ∼35 km width with the shelf break located in 110–150 m water depth (Seibold, 1982). The continental slope extends 200 km from the shelf break towards the deep-water basins and reaches down to a water depth of 1,500–4,000 m with slopes between 1° and 6° [Figure 1 (Wynn et al., 2000)]. On the slope there are abundant sedimentary waves and contourite deposits, which were attributed to bottom current reworking of sediments (Sarnthein et al., 1982). The Agadir Canyon has multiple tributaries that span over 100 km between the Tarfaya Basin and the Tafelny Plateau and cut deep into deep Moroccan continental shelf. The Tafelney Plateau is an offshore extension of the High Atlas system forming a basement high located offshore of Essaouira (Benabdellouahed et al., 2017; Hafid et al., 2000). Tarfaya Basin is a Late Permian to Triassic sedimentary basin controlled by the post-Variscan break up spanning between onshore to offshore southern Morocco and east of Spanish Fuerteventura and Lanzarote islands (Davison, 2005; Sachse et al., 2016; Wenke, 2014). At present, the canyon head is located ∼50 km north of the Souss river mouth. The Souss river lies in a synclinal valley, which is bounded to the North by the High Atlas Mountain range and towards the South by the Anti-Atlas Mountain range (Figure 1).
The water column structure of the Moroccan continental margin is defined by the Canary Current System, which consists of four main water masses now described from top to bottom (Knoll et al., 2002). Surface Water (SW), influenced by local atmospheric conditions, extends to the seasonal thermocline at about 150 m depth. Below this, the North Atlantic Central Water (NACW) reaches down to 600 m (Knoll et al., 2002). Antarctic Intermediate Water (AAIW) extends from 600 m to about 1,600 m and is flowing northward through the Lanzarote Passage at ∼900 m water depth (Hernández-Guerra et al., 2003). The AAIW is influence by the Mediterranean Water (MW), which is flowing southward through Lanzarote passage at 900–1,300 m (Hernández-Guerra et al., 2003). From 1,600 m to 3,800 m is the eastern North Atlantic Deep Water (NADW), which flows towards the south. Below 3,800 m flows the Antarctic Bottom Water (AABW) in the abyssal plains towards the North (Ambar et al., 1999; Sarnthein et al., 1982). The Agadir Canyon head sits within part of the high-productive trade-wind driven continental margin upwelling off Morocco (Freudenthal et al., 2002; Kuhlmann et al., 2004).
Data were collected during two consecutive cruises aboard the RV Maria S. Merian. The first cruise MSM32 was conducted between 25/09/2013 and 30/10/2013 and second cruise MSM113 between 09/12/2022 and 12/01/2023. The bathymetric data were acquired using the hull mounted EM120 (MSM32) respectively the newer EM122 (MSM113). Both systems were operating at a nominal frequency of 12 kHz at a maximal swath width of 130° and are used for bathymetric data. Processing included the application of sound velocity profiles, the application of manual and automatic methods to remove outliers. Data were gridded to a resolution of 30 m using a Gaussian weighted mean filter. Processing was done using the open-source software mbsystem (Caress and Chayes, 1995) and commercial QPS software Qimera, Fledermaus, and FMGT.
2D high-resolution seismic data were recorded during both MSM32 and MSM113 using a Geometrics GeoEel streamer (MSM32: 88 channels, MSM113: 56 channels) with a standard GI-gun (1.7 L) as source. Processing of both data sets was done with Vista Seismic Data Processing Software and included trace binning, NMO-correction, bandpass and fk-filtering, noise removal (THOR), stacking, and post-stack finite-difference migration. The data has a vertical resolution of ∼5 m (80 Hz, 1,500 m/s) and a high effective horizontal resolution (common midpoint spacing: 2 m). The IHS Kingdom software was used to visualize and interpret seismic data. All thicknesses of seismic units are calculated with a constant velocity of 1,500 m/s.
Seismic data were integrated with borehole information data of DSDP leg 50 site 415, from here on summarized as DSDP 415 [Figure 2 (Lancelot and Winterer, 1980)]. The site comprises three individual boreholes (415, 415A, 415B) to a maximum downhole depth of 1,079.5 m. The borehole information enables a seismo-stratigraphic reconstruction of the Agadir Canyon for most of the Cenozoic (Figure 2). However, Oligocene sediments are not present in the borehole, representing an erosional hiatus exists between the Late Eocene and Early Miocene (Lancelot and Winterer, 1980). Depth conversion of single stratigraphic horizons to time domain was achieved with interval velocities (Figure 2) derived by the shipboard scientific crew and taken from the DSDP data repository (Lancelot and Winterer, 1980).
Figure 2. Summary of drilling results of DSDP 415 (Lancelot and Winterer, 1980) down to the base of seismic imaging at approx. 600 m downhole depth. The target depth of the borehole is 1,079.5 m in two stages 415 and 415A. Lithology is correlated with age and distinct depths are given for individual ages. These depth/age correlations could be converted to seconds two-way travel time (TWTT) via given interval velocities from the DSDP data repository (see details in (Lancelot and Winterer, 1980)).
The present-day Agadir Canyon system starts at the Moroccan Shelf break and extends ∼450 km to the west across the Atlantic seafloor. Here, we describe the canyon morphology in 3 regions: the Canyon Head, the Upper Canyon and the Lower Canyon (Figure 3). The canyon head consists of two deeply incised tributaries (Northern and Southern) and the less pronounced central tributary between them (Figure 3D). These tributaries cut back into the shelf edge and merge approximately 80 km downslope into a single large conduit: the main Agadir Canyon (Figure 3). This main canyon has an exceptional cross-sectional area with a flat canyon floor ∼25–30 km that is bordered by steep canyon margins ∼1.2 km high. This morphology is broadly maintained through the Upper and Lower Canyon regions downslope. The Upper Canyon region starts about 20 km downslope from the canyon head confluence and extends ∼180 km along the main canyon to where it has a right-hand bend towards the northwest adjacent to Conception Bank (Figure 3A). Approximately 50 km downslope of the canyon head confluence there are two conduits that merge with the Upper Canyon: a deeply incised tributary herein called the Massa Canyon and part of the open slope called the Agadir Fairway (Figures 3A, C). The head of the Massa Canyon comprises several tributaries that are cut into the shelf edge, which then merge downslope into a single deeply incised (up to 400 m) sinuous channel (Figure 3A). The Massa Canyon is initially oriented east-west before bending progressively northwards and merging with the Upper Canyon (Figure 3C). The channel extends into the main Agadir Canyon as a weakly incised (∼20 m deep) and small (∼300 m wide) surficial channel. This surficial channel bounces back and forth between the canyon margins for another 180 km downslope before its morphology disappears. The Lower Canyon borders several seamounts along the remaining ∼190 km of the canyon: starting from ∼180 km from the Canyon Head confluence (after the right-hand bend) and terminating at the canyon mouth (Figure 3A). The Lower Canyon includes a relatively sharp (∼90°) left-hand bend most likely developed in response to the Essaouira Seamount. Here, the main canyon narrows considerably to ∼8 km wide and develops an outer bend overspill channel feature, which is elevated ∼300 m above the canyon floor (Figure 3B).
Figure 3. (A) Bathymetric map of Agadir Canyon from its head to mouth. The canyons’ main segments are the canyon head, the Agadir Fairway, upper and lower canyon. (B) Lower canyon with its mouth and the prominent overspill channel close to Essaouira seamount. The overspill channel shows a prominent sidewall failure at the location of our seismic profile. (C) Upper canyon 20 km downslope of the confluence, where the Agadir Fairway and the Tributary Channel enter into the main canyon. A small-scale surficial channel forms at the flat canyon floor. (D) Canyon head region that spans 100 km and comprises the three main tributaries: Northern (NT), Central (CT) and Southern Tributary (ST).
The high-resolution seismic data penetrates up to 1.5 s TWT (1,125 m at 1,500 m/s) into the subsurface, which allows us to resolve and correlate stratigraphic horizons from the DSDP core (Figure 2): Paleocene (dark red), Eocene (red), Early Miocene (orange), Middle Miocene (yellow), Late Miocene (purple), Pliocene (dark blue), and Pleistocene (turquois). A major unconformity in the borehole between the Eocene and Miocene has removed the entire Oligocene sedimentary record (Figure 2). The image of the sedimentary system is limited by the base tertiary unconformity (BTU) and interpretations of seismic reflections below the BTU are omitted (Figure 2). DSDP 415 penetrates the BTU at 491 m downhole depth. Here, 60 m of presumably Coniacian mudstones were encountered, which were deposited in palaeo-water-depths of 3,000–4,000 m (Lancelot and Winterer, 1980).
For the sedimentary succession of Agadir Canyon, we distinguish three main sedimentary packages which are demarcated by distinct erosional surfaces (Figures 4A, B, 5). The first erosional surface occurs around the Middle Miocene and is wider (∼18–30 km) than the present-day Agadir Canyon (∼10–15 km). Subsequent incision and deposition (Unit A) are maintained over this template (Figures 4E, 5). The second erosional surface occurs later in the Middle Miocene and is much narrower and more pronounced with the development of two distinct conduits in the upper segments of the Agadir Canyon (Figures 4B, D). This is overlain by Unit B. The third erosional hiatus is characterized by a series of relatively shallow incision surfaces that mark the onset of the Late Miocene within the main canyon (Unit C, Figure 5). This sedimentary package is bound by the present-day seafloor (Figures 4C, 5).
Figure 4. ∼36 km-long high-resolution seismic profile following the buried fairway in its main direction and crossing main canyon perpendicular to its thalweg (see Figure 1). The profile is shown as (A) seismological record and (B) interpreted color-coded seismo-sedimentary units, which are demarcated by major regional unconformities: (C) Late Miocene depth demarcating Uni C, (D) Middle Miocene incision surface demarcating Unit B and (E) Earlier Middle Miocene incision demarcating Unit A. All shown sections shows distance along profile in meter [m] on the x-axis, two-way travel time (TWT) in [s] along the y-axis and are ∼14 times vertically exaggerated.
Figure 5. Chronostratigraphic framework and the characteristic sedimentary units that are demarcated by the erosional surfaces.
We use a standard set of seismic facies that characterize the seismic sections. The corresponding nomenclature is summarized in Figure 6: (I) Finely-laminated layering of parallel reflections with no disturbance that are continuous over most of the continental slope and along the margins of the Agadir Canyon. These are interpreted as background sedimentation of hemipelagic muds (Li et al., 2018). (II) Facies II is mainly confined to the Canyon Head and is characterized by a strong flat-lying top reflection below or at the seafloor spanning several hundred meters (up to 1,200 m) with angular unconformities and complex onlapping geometries against it. We interpret these sediments to be affected by uplift and subsequent deformation above the abundant salt domes of the salt province (Tari and Jabour, 2013). (III) In all canyon regions, we see high-amplitude reflector (HAR) packages. In the canyon head, we see them tapering towards the outer bend bound to the canyon thalweg with interspersed thin (∼15–30 m) chaotic to transparent units with no internal reflections, in particular close the abundant salt domes and to steep canyon sidewalls. We interpret the HARs to be sandy turbidite deposits from powerful turbidity currents from the shelf (Böttner et al., 2024) interspersed with mass transport deposits from localized failures at salt domes or canyon margins (Carter et al., 2016; Davison, 2005). (IV) In all seismic images, we see chaotic to transparent units with no internal reflections punctuated by thin negative polarity HARs. We interpret these as stacks of mass transport deposit with mud-rich composition punctuated by thin turbidite beds (Böttner et al., 2024; Krastel et al., 2016; Li et al., 2018). The flat top is likely related to erosion of the MTD tops by of sandy turbidites flushing the canyon (Canals et al., 2006; Hunt, 2017; Hunt et al., 2013). The negative polarity can be related to compaction of clay-rich sediments at the base of the landslide during deposition (Sawyer et al., 2009). (V) Inside the canyon’s head and mouth, we observe thick (up to 100 ms) HAR packages with complex internal reflections and erosional surfaces. We interpret these as thick (up to 80 m) sandy turbidite deposits with phases of erosion and deposition (Böttner et al., 2024; Janocko et al., 2013). (VI) At the canyon’s mouth, the seismic image shows 100–300 m wide sub-vertical features with chaotic and largely transparent acoustic character associated with local uplift of adjacent and overlying sediments. We interpret thee to be syn-sedimentary volcanic intrusions or dykes from the CISP (Neumaier et al., 2016; van den Bogaard, 2013). (VII) A characteristic facies of the Agadir Fairway – and tied to Massa Canyon – are stacked high-amplitude reflectors (HAR) with rugged top surfaces (up to 25 ms) and V-shaped incisions (diffraction hyperbolas). We interpret these as small-scale channels that form by (overspilling) turbidity current erosion as a part of a secondary drainage system (with respect to the Massa Canyon) on the channel levees (de Leeuw et al., 2018; Miramontes et al., 2020; Normandeau et al., 2019). (VIII) In the Canyon Head, Agadir Fairway, and around Essaouira and Conception Bank seamounts our seismic image shows stepped finely-laminated reflectors with low vertical offsets (8 ms or 6 m) in between individual packages, partially centered on top of strong-flat lying reflectors. We interpret these as sets of closely spaced normal faults (“piano key faults”) with low offsets in response to localized uplift from salt domes or seamount formation (Alves et al., 2009; Benabdellouahed et al., 2017; Gamboa et al., 2010; León et al., 2022; Tari and Jabour, 2013). (IX) At the base of Agadir Canyon and more pronounced in the Massa Canyon sidewalls we see stacked high-amplitude reflectors (HAR) with rugged top surfaces that gently taper towards each side (from 25 ms to ∼0 ms). These features are interpreted as turbidite channel-levee systems with repeated overspilling of turbidity currents developing tapered levees (Janocko et al., 2013; Schwenk et al., 2005). (X) Bound to the northern side of the Agadir Canyon and buried within the sidewall, our seismic data shows undulating finely-laminated packages with continuous reflections and no vertical offsets. These features are interpreted as sediment waves developing on the northward side of the canyon in response to bottom currents flowing from the North to the South (Fuhrmann et al., 2020; Jacobi et al., 1975).
Figure 6. Seismic Facies in the Agadir Canyon, their facies description, interpretation, and distribution across the survey area.
Here, we use high-resolution seismic data with age constraints from DSDP 415 and bathymetric data to characterize the canyon strata and map its distribution through the canyon profile.
The canyon head region spans 100 km and comprises three large tributaries (Northern, Central and Southern) with a network of smaller drainage channels (Figure 3). The canyon head tributaries are ∼10 km west of Cape Ghir (Figure 7). The structural high that forms the Cap Ghir peninsula extends offshore across the shelf (∼50 km) as a prominent 40-m high mound on the seafloor, which is mostly buried by a thick accumulation of finely laminated sediments overlying an unconformity. The sedimentary body that builds above the mound is significantly thicker on the northern side of Cape Ghir.
Figure 7. Parasound profile at the head of Agadir Canyon showing a sediment drift body on top of an erosional unconformity. (B) Bathytmetric map of the head of Agadir Canyon indicating the location of the parasound profile (A), offshore Cape Ghir. The slate blue arrows indicate possible bottom current directions. White dashed lines indicate the thalwegs of the three main Agadir Canyon and the tributary channel. NT, Northern Tributary, CT, Central Tributary, ST, Southern Tributary [cf. Böttner et al. (2024).
The Northern Tributary is a flat-bottomed, steep-sided conduit ∼3 km wide and up to 400 m deep. Along the margins of both tributaries are finely-laminated reflectors, which represent background hemipelagic sediments (Facies I). Salt diapirs from depth (below maximum penetration) confine the canyon sediments, which are characterized by HAR interspersed with chaotic to transparent units (Figure 8). The Southern Tributary records a similar character with multiple salt diapirs (Facies II) confining sedimentary packages of HAR (Facies III) and chaotic to transparent units (Facies IV). In both tributaries, the sedimentary packages show numerous incisional surfaces that are variably cut into and occasionally cut-out underlying packages (Facies V).
Figure 8. Two seismic sections of high-resolution seismic profile P22 crossing Agadir Canyon Head. (A) 15-km long section across the northern tributary showing salt diapirs, small scale MTDs and high-amplitude reflections (HAR) within the thalweg. (B) 22 km-long section across the southern tributary thalweg and towards the southeast a salt diapir. (C) Zoom in of (B). The sedimentary succession comprises mainly packages of high-amplitude reflections (HAR) and few small MTDs. The profiles show distance along profile in meter [m] on the x-axis, two-way travel time (TWTT) in [s] along the y-axis. The vertical exaggeration is indicated in each section.
The canyon head tributaries amalgamate into a single large conduit (∼30 km wide and ∼1.2 km deep) at about 100 km from the coast at ∼2,500 m water depth (Figures 3, 9). The present-day morphology is a relatively flat-bottomed canyon around 8 km wide and ∼400 m deep. Several salt diapirs are present just beneath the surface (Facies II), which confine the canyon sedimentation into three smaller conduits 1–2 km wide. Similar to the upper tributaries, the main canyon sediments are characterized by weakly incisional HAR (Facies III) and chaotic units (Facies IV). In the canyon head confluence, the canyon margin sediments are correlated with the DSPD stratigraphy via overlapping seismic profiles across the slope. This provides age constraints down to Late Miocene to Pliocene strata. However, none of these horizons can be correlated into the canyon stratigraphy. Hence, the age of the canyon fill remains unknown in this area.
Figure 9. 20 km-long high-resolution seismic profile crossing Agadir Canyon at the confluence of all head tributaries. The image shows four salt diapirs that emerge from below toward the seabed. In between the salt diapirs form mini basins with high-amplitude package infill. Only the central basin infill shows chaotic to transparent facies of mass transport deposits (MTDs). The seismic image is dominated by packages of high-amplitude reflections (HARs). The profile shows distance along profile in meter [m] on the x-axis, two-way travel time (TWTT) in [s] along the y-axis and is ∼6.6 times vertically exaggerated.
The Upper Canyon stratigraphic packages are bound by the established DSDP chronological boundaries (Figure 4). The lowest penetration captures the Paleocene and Eocene showing mid-range amplitude parallel reflectors separated by chaotic units, which taper to the south and gently thicken into the central part of the profile. DSPD 415 records a major unconformity between Eocene and Miocene sediments (Figure 2), which has been interpreted as an erosional hiatus with missing Oligocene sediments (Lancelot and Winterer, 1980). In contrast to DSDP 415, our seismic data shows no obvious erosional hiatus between the Late Eocene and Early Miocene (Figure 10). Instead, our data show three distinct erosion surfaces developed from the Middle Miocene onwards (Figure 4B). First, an initial incision surface occurs just after the Middle Miocene, which is overlain by thick (up to 80 m) low-amplitude chaotic units separated by thinner high-amplitude reflection (HAR) packages (Unit A; Figures 4E, 10). These HAR packages (Facies III) in Unit A show concave upward geometries tapering away from a central crest, which form wedge-like geometries with onlapping relationships across the canyon. The second incision occurs during the Middle to Late Miocene and shows a much narrower and pronounced erosional surface (which is overlain by thick, up to 70 m) chaotic to transparent units (Facies IV) separated by very thin HARs (Unit B; Figures 4D, 10). Unit B completely heals the previous incision. The third incision surface occurs in the Late Miocene and is characterized by shallow erosion within the main canyon and along its southern margins. It is overlain by thick chaotic units (Facies IV) separated by thin HARs (Facies IV), which are thicker along the northern side of the canyon floor and taper away from the margin (Figures 4C, 10).
Figure 10. ∼25 km-long high-resolution seismic profile crossing DSDP 415 (see Figure 3) shown as A pure high-resolution seismic imaging and B interpreted color-coded units A–C, three major unconformities (red lines) and minor erosional surfaces (red dashed lines) with age constraints. The profile shows distance along profile in meter [m] on the x-axis, two-way travel time (TWTT) in [s] along the y-axis and is ∼9.2 times vertically exaggerated.
Unfortunately, our stratigraphic interpretations for the distal part of the canyon lack age control, whereby we can only qualitatively compare this stratigraphy with the better constrained sections upstream. However, the general stratigraphy appears similar with three distinct units (A, B, and C) demarcated by three canyon-wide erosion surfaces (Figure 11). The first incision truncates poorly resolved basal reflectors, which record opposing dip angles from south to north (Figure 11). Overlying this surface is Unit A, comprising thick (up to 70 m) packages of transparent and chaotic facies punctuated by thinner HARs. The Lower Canyon Unit A lacks the shallow, localized, erosional surfaces and tapering geometries found in the Upper Canyon Unit A stratigraphy. Instead, the unit records a progressive pinch out to the south and has been subject to gentle folding. A second erosion surface cuts into Unit A and is, in turn, overlain by Unit B. Unit B immediately mantles the erosion surface with thin HARs, which are locally thicker in the central part of the profile (Figure 11). The remainder of Unit B is dominated by stacked transparent and chaotic packages that heal the underlying incision (Facies IV). Finally, a third erosion surface incises across the canyon profile and is overlain by Unit C (Figure 11). The erosion surface is relatively shallow and rugose with sporadic truncation of HARs along the surface. Overlying this surface, Unit C comprises stacked transparent facies (Facies IV) punctuated by weakly developed, flat-lying, higher-amplitude reflectors (Facies III). This Lower Canyon profile records syn-sedimentary deformation with concertina folds and southerly pinch out of Unit A, followed by a gentle northerly tilt on Unit B and an almost flat-lying Unit C.
Figure 11. ∼14 km-long high-resolution seismic profile crossing main canyon perpendicular to its thalweg (see Figure 1). This profile has no stratigraphic control from the DSDP drill hole. Seismic units are identified based on their distinct facies. The profile is shown as (A) seismological record and (B) interpreted color-coded units (A–C) and distinct erosional surfaces (red lines). The profile shows distance along profile in meter [m] on the x-axis, two-way travel time (TWTT) in [s] along the y-axis and is ∼8.5 times vertically exaggerated.
A profile across the sharp ∼90° turn at the mouth of the canyon shows a more complex picture compared to upstream profiles (Figure 12). Here, there is an interplay between regional and locally developing geological structures, and syn-sedimentary deposition within the canyon (Figure 12). The southern basal part of the profile records laterally offset stacked channels ∼300–500 m wide that cut down ∼50–100 m and are subsequently filled by 30–50 m thick HARs (Figure 12B). Above these channels is a thick package (∼100 m) of HARs (Facies III) that extend ∼15 km to the north beyond the present-day canyon floor. Numerous shallow and localized erosion surfaces are present within this package (Facies V). Across the main canyon floor this package records repeated folds and has a gentle south-facing tilt with concomitant thinning towards the north (Figure 12). The HAR package onlaps with fanning stratigraphy against a local structural high (Figure 12D). The structure is characterized by a sub-vertical pipe of transparent facies associated with truncation and deformation of adjacent and overlying reflectors (Facies VI, Figure 12D). Smaller faulting recorded in the profile has similar character but associated with less deformation of overlying reflectors. The top of Unit A has been eroded with sporadic shallow incisions truncating the HARs (Figure 12F). Within the canyon to the south Unit A is incised and overlain by sub-horizontal chaotic and transparent facies (Facies IV, ∼30–40 m thick), occasionally punctuated by thin HARs (Unit B; Figures 12A, C). Laterally adjacent facies outside the incision surface to the north comprise finely laminated low- and mid-range amplitude reflectors. In turn, a relatively shallow erosion surface incises into the main canyon-floor sediments, which is overlain by a thinner package of HARs and chaotic facies including a local thickness of HARs (Facies III) against the inner bend (southern margin) of the canyon (Figure 12C). This shallow erosion surface correlates northward into a much deeper incision across the “overspill channel” (Figures 12D, E). This is immediately mantled by HARs (∼30–40 m thick) clustered against the northern and southern margins, followed by a mixture of lower-amplitude reflectors and very thin HARs (∼7.5–15 m thick). The incision appears healed by 5.5 s TWT and is subsequently overlain by sub-horizontal mid-amplitude reflectors with occasional thin MTDs (Facies IV, ∼10–20 m thick; Figure 12F). This motif continues upwards with another two unconformities and a change in dip towards the south (Figure 12E).
Figure 12. ∼30 km-long high-resolution seismic profile located at the mouth of Agadir Canyon. The profile is shown as (A) seismological record with zoom ins on the (B) deep buried channels, (C) present-day Agadir Canyon, (D) uplift in between the Overspill and Agadir Canyon as well as (E) the sidewall failure and (F) interpreted color-coded units (A–C) and major erosional surfaces (red lines). On the left is the Agadir Canyon while on the right is the overspill canyon. Both canyons are crossed perpendicular to their thalweg directions proximal to the 105° bend at the outflow of Agadir Canyon (see Figure 1). The profile shows distance along profile in meter [m] on the x-axis, two-way travel time (TWTT) in [s] along the y-axis and is ∼11 times vertically exaggerated.
The Middle Miocene documents the earliest indications for downslope sediment transport through the Agadir Fairway (Figure 13). The succession comprises three packages, Units A, B and C, which are demarcated by three erosion surfaces and the development of the Massa Canyon. The first erosion surface is wide and shallow and overlain by relatively thick (up to 70 m) units with weakly developed mixtures of low- and high-amplitude reflectors (Unit A, Figure 13). Individual facies show gentle tapering geometries in across slope orientations (north-south). The second erosion surface occurs in the Middle to Late Miocene and incises ∼130 m into Unit A forming a distinct V-shaped channel in the central part of the profile. This erosional surface is overlain by multiple stacked low-amplitude chaotic units (Facies IV) punctuated by occasional thin HARs (Unit B; Figure 13). Outside the incision surface across the open slope are finely laminated low-amplitude reflectors (Facies I). The Unit B channel form is almost completely healed by the Late Miocene as shown by continuous reflectors that cross through the channel and onto the open slope, with only small-scale incision appearing at the central thalweg (Facies VII). A third laterally restricted erosion surface occurs in the Late Miocene that weakly cuts into Unit B across the former V-shaped channel form (Figure 13). This is subsequently overlain by Unit C, which comprises an inter-fingered succession of open slope (Facies I) and channel margin facies (Facies VII). Initially, Unit C comprises a mixture of laterally restricted chaotic and transparent packages, punctuated by thin HARs (Facies IV). These facies are laterally restricted with abrupt pinch outs forming a progressively widening V-shaped cross-section. The adjacent slope records finely laminated low-amplitude reflectors (Facies I) that host abundant vertical faults to the west (Facies VIII; Figure 13). In the Early Pliocene, the Massa Canyon initiates with the development of tapering wedge-shaped HARs with rugged top surfaces in the northern part of the fairway (Facies IX; Figure 13). In the Late Pliocene, these units become thicker (∼20 m) and extend farther across the buried fairway (up to ∼20 km) with more pronounced erosion surfaces along their tops (Facies VII). These facies interfinger with transparent and chaotic units situated in the central parts of the profile (Facies IV). From the Early Pleistocene the Massa Canyon changes character with a switch to finely laminated low-amplitude reflectors on its margins with only occasional thin higher-amplitude intervals. This coincides with a deep incision over 100s of meters through Unit C into the Middle-to Late-Miocene. An erosion surface is preserved on the eastern margin of the Massa Canyon, which is draped by finely laminated low-amplitude reflectors (Facies I). This constrains the onset of major canyon incision to around the Early Pleistocene. Coincident across the open slope are low to mid-range reflectors punctuated by thick (∼40 m) and laterally extensive (∼15 km) chaotic packages (Facies IV; Figure 13). The uppermost chaotic unit imaged at the surface is the “Agadir Slide,” which has been mapped downslope into the Agadir Canyon (Li et al., 2018).
Figure 13. ∼45 km-long high-resolution seismic profile crossing the Agadir Fairway and tributary channel (see Figure 1) shown as (A) pure seismological record and (B) interpreted color-coded units A–C and erosional surfaces (red lines) with age constraints. The profile shows distance along profile in meter [m] on the x-axis, two-way travel time (TWT) in [s] along the y-axis and is ∼18 times vertically exaggerated.
The chronostratigraphic framework comprises three major sedimentary units which are separated by three major regional unconformities tied to distinct geologic periods (Figure 5). Here, we discuss the onshore and offshore geodynamic, tectonic, climatic, and oceanographic controls in the onset and evolution of the Agadir Canyon.
The Eocene/Oligocene boundary shows a large drop in eustatic sea-level (∼100 m) coupled with a period of regional Alpine development in the High Atlas which resulted in a flexural uplift of the shelf area of the Tarfaya Basin (Frizon de Lamotte et al., 2009; Miller et al., 2020). The combination of both during this boundary increased sediment supply from the Moroccan hinterland towards the shelf edge.
In the Miocene, the tectonic uplift played no significant role as tectonic activity was in a phase of relaxation after the Oligocene compressive phase (Frizon de Lamotte et al., 2009). During this tectonic quiescence, sea level was likely the dominant influence on sediment supply to the shelf edge as a relative sea level high stand during the Miocene climatic optimum (Early to Middle Miocene) was followed by a sea level drop during the Middle Miocene climatic transition (Miller et al., 2020).
At the end of the Pliocene, eustatic sea-level fell by ∼40 m (Miller et al., 2020), which would have promoted shoreline advancement and increased sediment delivery to the shelf edge. In the Quaternary, the uplift of the Atlas caused higher onshore erosion rates (Charton et al., 2021) and increased the overall sediment input into Agadir Canyon. The sustained fall in eustatic sea-level during the Pleistocene may have allowed terrestrial river systems such as the Souss, to directly connect with the canyon heads (Figures 1, 3). Onshore, the Draa, Massa and Souss River systems show a long-lived evolution with repeated erosional hiatuses and terracing (Tesón et al., 2010), which links closely with the development of the offshore shelf feeding the Agadir Canyon System (Benabdellouahed et al., 2017).
Salt diapirism can function as a driver for submarine channel evolution (Carter et al., 2016; Pandolpho et al., 2024). Salt in the area is sourced from Late Triassic/Early Jurassic strata and started moving during the Early Cretaceous (Hafid et al., 2000; Hinz et al., 1982; Tari and Jabour, 2013). This makes it likely that salt diapirism has influenced canyon development across its entire lifespan.
Indeed, the sedimentary packages in the tributaries show persistent angular unconformities throughout and complex onlapping geometries against the salt (Figures 8, 9). This indicates a chronic syn-sedimentary relationship with salt growth as far back as we can see. The complex and amalgamated nature of the canyon head that spans over 100 km along the shelf break with multiple tributaries and secondary channels documents that the abundant salt diapirs in the head region of Agadir Canyon have actively reshaped the canyons morphology (Figures 3, 4, 9). The constant upward movement of the salt has changed the sediment routing by changing the morphology and thereby dictating the style and type of sediments that are funneled into the main canyon throughout the canyon’s evolution. The earliest phase of influence was likely during the Middle Miocene, where the salt has likely redirected sandy gravity flows from the Moroccan shelf and helped formed the complex and intertwined unconfined channel system (Figures 4, 8, 9). A phase of strong salt influence was the later Middle Miocene, where the Upper Agadir Canyon shows the contemporary development of two different conduits (Figure 4D), which we also attribute to sediment re-routing caused by the abundant salt diapirs in the canyon head area.
The Canary Island Seamount Province (CISP) forms a scattered hotspot track on the Atlantic Ocean floor that forms perpendicular to lithospheric fractures and parallel to the northwest African continental margin. The CISP comprises more than one hundred seamounts that form isolated volcanic structures on the seafloor. The seamounts of the CISP range from small (<1,000 m height), to mid-sized (>1,000 m), to ocean islands such as Lanzarote, which have been active since the Late Jurassic (van den Bogaard, 2013). Along the Agadir Canyon are several seamounts that influence its formation and deflect its thalweg: Essaouira (68 Ma), Rybin (55 Ma), Conception Bank (18 Ma) and potentially Lanzarote (15 Ma) (van den Bogaard, 2013).
The main canyon morphology shows directional changes of the thalweg in response to the seamounts related to the CISP (Figures 1, 3). Especially the oldest seamounts Essaouira and Rybin that are present throughout the whole lifespan of the Agadir Canyon and have caused sharp directional changes of thalweg (Uchupi et al., 1976). For example, the sharp bend before the Canyon Mouth occurs in proximity to the Essaouira Seamount, which initiated ∼68 Ma (van den Bogaard, 2013). Syn-sedimentary relationships found within the Canyon Mouth stratigraphy indicate continued seamount growth with regional uplift tilting sediments to the south and vertical dykes locally influencing deposition (Figure 12). Indeed, tectonic activity from the seamount is most likely driving the development of the overspill channel with vertical intrusions locally diverting flow pathways and general seafloor uplift elevating the overspill channel progressively higher above the main canyon floor.
The development of Conception Bank (18 Ma) and Lanzarote (15 Ma) during the Early to Middle Miocene has likely had a strong influence on sediment routing and supply (León et al., 2019; van den Bogaard, 2013). The development of Conception Bank caused regional uplift as evidenced by folding and tilting of stratigraphy within the Main Canyon (Figure 11). The formation of the seamounts in the Middle to Late Miocene might also have resulted in local slope failures (León et al., 2019; Palomino et al., 2016), which may have produced MTD deposits within the middle to lower parts of the canyon. However, DSDP 415 documents only very minor contributions of volcanic glass from the Middle Miocene onwards and no mass transport deposits of volcanic origin (Lancelot and Winterer, 1980). From the Late Miocene to Pliocene (11-2 Ma), the minor volcanic admixtures found in DSDP415 could also relate to high volcanic activity in the onshore Sirwa (Jebel Siroua) volcanic region (Berrahma and Delaloye, 1989; Liégeois et al., 2005), which is located in the catchment of the Souss river (Figure 1).
However, Conception Bank (and Lanzarote farther South) also formed a topographic barrier parallel with the Moroccan Margin, which produced another pathway into the canyon: the Agadir Fairway. The Agadir Fairway records similar timings to the Main Canyon and Conception Bank growth with initiation around Early to Middle Miocene (Figure 12). Continuous along-canyon seismic profiles show that the thick MTDs found within the main canyon predominantly come from the Agadir Fairway (Moroccan Margin), rather than the slopes of Conception Bank (Li et al., 2018). Indeed, up-slope of the Agadir Fairway-Main Canyon confluence, MTDs become thinner and less numerous within the stratigraphy (Figure 8). This analysis suggests the Agadir Fairway is the primary source for MTDs recorded in the Agadir Canyon stratigraphy. The coincident timing of Conception Bank growth and slope failures through the Agadir Fairway suggest a significant relationship. The growing seamount chain not only developed the Fairway itself but likely influenced the Moroccan Margin via progressive steepening of the slope and periodic earthquakes. These factors would promote slope destabilization and MTD emplacement into the Main Canyon, which ultimately dominate its stratigraphy.
Climate perturbations constantly cause changes in the intensity of the trade winds, surface water circulation and coastal upwelling (Sarnthein et al., 1982). Neogene paleoenvironments along the northwest African continental margin have likely evolved synchronous with global climatic changes (Miller et al., 2020). Significant climatic disruptions during the Oligocene and Early Miocene recovered to more steady environmental conditions, nearly reaching stable Eocene (Zachos et al., 2001). During the Neogene, the North African vegetation underwent significant changes from the tropical forests of the Early Miocene towards the evolution of the Sahara Desert in the Miocene/Pliocene (Jacobs, 2004; Le Houérou, 1997; Micheels et al., 2009; Wolfe, 1985). Over the last 13–18 million years distinct major episodes of climatic deterioration have influenced the evolution of Agadir Canyon: First, major changes have consistently correlated with intensified oceanic circulation, glacial-style meridional wind systems, and arid climates in northern Africa. Second, intervening phases of climatic improvement and stability were associated with dominant zonal wind circulation and increased continental wetness (Demenocal, 1995; Sarnthein et al., 1981; Sarnthein et al., 1982). Overall, both alternating climate types persisted for approximately equal durations. The enhanced fertility driven by coastal upwelling and/or significant river discharge will have driven sediment into the Agadir Canyon. These climatic conditions were primarily limited to two periods: the Early and Middle Miocene until 14 million years ago, and the last 3 million years. Increased productivity due to equatorial upwelling ended around 17.5 million years ago and only moderately returned during the Middle and Late Pleistocene (Freudenthal et al., 2002; Kuhlmann et al., 2004; Sarnthein et al., 1982). Coastal upwelling and increased productivity will have promoted the characteristic drainage system of the Agadir Canyon by which giant turbidity currents are fueled by finer grained (muddy) material (MTDs) to exceptionally grow, erode and reshape the lower Agadir Canyon (Böttner et al., 2024).
Bottom currents are actively shaping the canyon through time. For deeper segments of the canyon, seismic profiles crossing the show aggradation of the southern margin and prograding of deposits on the northward side since the Middle Miocene (Figures 4, 5). This type of pattern is usually seen in hybrid turbidite-drift channel complexes, that are created by the interaction of steady bottom currents with episodic sediment gravity flows through canyons or channels (Fuhrmann et al., 2020; He et al., 2013). Based on the southward progradation on the northern canyon margin, the bottom currents are flowing southward. Indeed, at present the Lanzarote Passage shows strong bottom currents that are bound by the Canarian volcanic domain and the Northwest African slope apron. MW is flowing southward in the deep part of the passage (900–1,300 m water depth) with a mean transport of −0.05 ± 0.17 Sv (Hernández-Guerra et al., 2003). However, through geologic time, the MW is highly variant and largely dependent on the closure and opening of the Atlantic-Mediterranean gateway (Capella et al., 2019; Hernández-Molina et al., 2014). NADW (1,500–3,800 m water depth) circulation likely started in the Oligocene but might have started in the Early Cretaceous (Davies et al., 2001; Mourlot et al., 2018; Via and Thomas, 2006). Intensification of the NADW circulation during the Miocene because of the final closure of the western Tethyan equatorial pathways for deepwater circulation and the opening of an outlet for cold Norwegian Sea Overflow Water across the Island-Faeroe Ridge initiated a phase of extensive bottom current erosion at the northwest African continental rise (Herold et al., 2012; Sarnthein et al., 1982). It is feasible that the resulting bottom currents are actively reshaping the canyon at least from the Miocene until present.
On the shelf, the structural high that forms the Cap Ghir peninsula extends offshore across the shelf (∼50 km) as a prominent 40-m high mound on the seafloor is buried by a thick accumulation of sediments overlying an unconformity (Figure 7). Because the sediment body is significantly thicker on the northern side of the Ghir peninsula and its seismic character, we interpret it as a contourite that has been emplaced by bottom currents. This suggests that ocean currents are actively redistributing sediments along the shelf and depositing them against the upstream side of the topographic obstacles. Thereby, bottom currents are at present actively reshaping the shelf and influence the sediment supply into the Agadir Canyon head.
The inception of Agadir Canyon has long been associated with the Eocene/Oligocene boundary, when a major sea-level fall (Miller et al., 2020) would have led to strong shoreline progradation and more direct delivery of sediments to the shelf edge, and ultimately the deep ocean. The Late Eocene uplift of the High Atlas promoted increased erosion across the hinterland (Charton et al., 2021) and would have significantly increased sediment supply to the Moroccan shelf. Together the major sea-level fall and rise of the High Atlas across the Eocene/Oligocene boundary most likely increased sediment supply to the shelf edge. This period is thought to be the onset of many submarine canyon systems along the NW African coast (Hinz et al., 1982; Seibold, 1982; Uchupi et al., 1976; Wynn et al., 2000). These factors also produced an erosive hiatus in DSDP 415 through the entire Oligocene and promoted the development of proximal submarine fan systems within the salt province, fed by sandy turbidites from the shelf region (Neumaier et al., 2016; Sachse et al., 2016; Wenke, 2014) and distal unconfined mass wasting from the open slope (Heyman, 1989; Lee et al., 2004) or adjacent seamounts (Gamboa et al., 2021; León et al., 2019; van den Bogaard, 2013). However, while DSDP 415 shows the corresponding hiatus in the sedimentary record missing the entire Oligocene (Lancelot and Winterer, 1980), all other DSDP boreholes in the region (DSDP 416, 397, 369) show hundreds of meters of Oligocene sedimentation [Lancelot and Seibold, 1978; Lancelot and Winterer, 1980; von Rad and Ryan, 1979]. This suggests that no regional unconformity exists, but rather localized erosion caused the hiatus in DSDP 415. This matches our seismic data crossing the DSDP 415 (Figure 10), where no major hiatus is visible in between the Eocene and Miocene sediments. Indeed, the first stratigraphic unit recorded in the Agadir Canyon (Unit A) is only emplaced during the Middle Miocene (Unit A). This onset of sediment delivery shows a consistent age through the Upper and Lower Canyon, and on the Upper slope across the Agadir Fairway, which suggests a rapid (geologically instant) canyon inception from proximal to distal rather than progressive progradation over time. It is possible that older (Oligocene) sediments may be recorded in the Canyon Head region, where we lack age control. In this scenario, the Agadir Canyon must have developed as a slope apron with sediments restricted to the Moroccan shelf-break. Over time the canyon became more confined and able to deliver sediments more effectively to deeper water, which is represented by Unit A in the Middle Miocene. On balance this second model seems unlikely for two reasons: 1) Unit A in the Agadir Fairway, which is located on the upper slope comparable in proximity to the canyon head region, is dated the same as in the Main Canyon (Middle Miocene), and; 2) It is unlikely that a major input of sediment during the Eocene/Oligocene boundary would be restricted to the proximal upper slope, particularly as elsewhere along the margin Oligocene sediments are found far beyond the slope in deep-water basins (DSDP 416, 397, 369).
Our seismic data show the first erosional surface inside Agadir Canyon and the Agadir Fairway occurs in the early Middle Miocene and subsequent deposition of Unit A is maintained over this template until the late Middle Miocene (Figures 4E, 5, 10, 13).
In proximal parts, Unit A has packages showing wedge-like geometry with tapering and onlapping relationships across the profile, which are interpreted as weakly developed channel-levees (Janocko et al., 2013; Schwenk et al., 2005). The channel-levees comprise most of this sedimentary package and suggest that sediment transport was dominated by sandy turbidity currents that formed many localized channel-levee systems as part of the Tarfaya Basin Sedimentary System (Lee et al., 2004; Sachse et al., 2016; Wenke, 2014). During the tectonic quiescence of the Miocene, sea level was likely the dominant influence where a relative sea level high stand during the Miocene climatic optimum (Early to Middle Miocene) was followed by a sea level drop during the Middle Miocene climatic transition (Frizon de Lamotte et al., 2009; Miller et al., 2020). In addition, the Middle Miocene climate was characterized by enhanced coastal upwelling and significant river discharge, which in combination will have driven sediment input into this early sedimentary system (Sarnthein et al., 1982). Thus, the combination of sea level and climate perturbations likely controlled the formation of this early distributary channel system (Unit A) close to the Moroccan shelf edge, preceding the development of the Agadir Canyon (Figure 14). The development of Conception Bank (18 Ma) and Lanzarote (15 Ma) during the Early to Middle Miocene (León et al., 2019; van den Bogaard, 2013) started forming the Agadir Fairway with concomitant initiation of mass wasting from the Moroccan Margin (Figure 12). MTDs sourced through the Agadir Fairway dominate canyon stratigraphy, particularly in the Lower Canyon where Unit A is characterized by thicker MTDs and less HARs than Upper Canyon profiles (Figure 9). Unit A within the Lower Canyon is gently folded (Figures 9, 11), most likely in response to crustal shortening generated by the uplift of Rybin seamount and Conception Bank (Gamboa et al., 2021). We note that overlying units are not folded like Unit A, which suggests that seamount growth reduced significantly by the time Unit B was emplaced (later Middle Miocene).
Figure 14. Stratigraphic chart with reconstruction of eustatic sea level during the Late Cenozoic [after Miller et al. (2020)]. Annotations highlight key phases of tectonic and volcanic activity in the hinterland, major climatic events, and timing of influence from the salt province and bottom currents. The three main stages of canyon evolution are shown: (1) Early sedimentary system with proximal shallow incision and turbidite channel-levees, (2) Repeated periods of major canyon incision with filling by MTDs, and (3) Canyon incision with filling by MTDs.
The second erosional surface occurs later in the Middle Miocene and is much narrower and pronounced (Figures 4, 5 10). The consequent incision has a pronounced V-shape which acts as a template over which the subsequent erosion and deposition is maintained until present day (Unit B, Figure 14). During this phase, our seismic data show the development of two distinct conduits in the upper segments of the Agadir Canyon (Figure 4). While earlier studies suggest the Oligocene sea-level drop (∼100 m) as the onset of canyon development along the Northwest African Margin (Ercilla et al., 1998; Lancelot and Winterer, 1980), our data show that Agadir Canyon inception was not until the Middle Miocene, eroding and overprinting Unit A the first sedimentary system (Figures 4, 5, 10, 13).
Prior to deposition of Unit B, the main Agadir Canyon was subject to 100s of meters of incision both in proximal locations and more distally through the main canyon (Figures 4, 10, 13). The presence of deep incision during the Middle Miocene across both the Agadir Canyon and Fairway indicate powerful and highly erosive gravity flows, which most likely came from the shelf edge (Böttner et al., 2024). Sediment input into the Agadir Canyon in this phase of tectonic quiescence was mainly driven by sea level fluctuations. The high sea level during the Miocene climatic optimum (Early to Middle Miocene) was followed by a sea level drop during the Middle Miocene climatic transition (Miller et al., 2020). In the Middle Miocene, significant river discharge driven by increased continental wetness (Demenocal, 1995; Sarnthein et al., 1981; Sarnthein et al., 1982) will have promoted the alternating system of muddy landslide deposition into the canyon and flushing by powerful sandy turbidites from the shelf (Böttner et al., 2024; Canals et al., 2006; Heijnen et al., 2022a).
On the northern flanks of the Agadir Canyon, Unit B shows that hemipelagic sediments are truncated by a stepped sequence of concave-up erosion surfaces that show stepped migration to the south (Facies X, Figures 4D, 5). These surfaces are all onlapped by HARs and relatively thin chaotic units that pinch out to the north and downlap against surface to the south (Figure 4D). This style of channel stacking is commonly found at the base of channel-complexes before the system develops stronger confinement (McHargue et al., 2011; Peakall et al., 2000) or in hybrid turbidite-drift channel complexes, that are created by the interaction of steady bottom currents with episodic sediment gravity flows through canyons or channels (Fuhrmann et al., 2020; He et al., 2013).
The development of Conception Bank (18 Ma) and Lanzarote (15 Ma) during the Early to Middle Miocene (van den Bogaard, 2013) would have been a key influence on Agadir Canyon development as their construction maintained the Agadir Fairway, which allowed MTDs from localized seamount growth (Figure 9) and the Moroccan Margin (Figure 13) to be emplaced within the Main Canyon.
The morphology of the canyon was heavily overprinted from its inception onwards in both proximal and distal parts (Figure 3). In proximal parts, salt diapirs of the salt province along the Moroccan slope and shelf have reshaped the canyons thalweg and forced the development of multiple tributaries (Figure 4). The evolution of two different canyon branches during that period (Figure 4D) is likely related to sediment re-routing caused by the salt diapirs in the canyon head area (Carter et al., 2016; Lee et al., 2004; Tari and Jabour, 2013). In distal parts, seamounts of the CISP (Essaouira, Rybin, Conception Bank) have reshaped the canyon thalweg and deflected its path down slope (Figures 3, 12). At least since the Middle Miocene, southward flowing (strong) bottom currents have also actively reshaped the canyon and forced aggradation of sediments on the southern margin and prograding sediment waves on the northward side (Facies X, Figures 4, 5).
The third erosional hiatus is characterized by a series of relatively shallow incision surfaces that mark the onset of Late Miocene within the main canyon (Unit C, Figure 14). These cuts are confined within the present-day canyon morphology and follow the template of incision laid down during the Middle Miocene (Figure 4B). The sedimentary package of Unit C is bound by the present-day seafloor (Figure 4C) and comprises tiers of thick chaotic to transparent units which are intercalated by occasional HARs (Figures 4, 5, 10, 13). By linking the seismic facies (Facies II and IV) to seafloor observations (Böttner et al., 2024; Ercilla et al., 1998; Hunt, 2017; Hunt et al., 2013; Stevenson et al., 2014; Talling et al., 2007), these likely represent alternating deposition of laterally restricted thick MTDs and occasional sandy turbidites.
The MTDs (Facies IV) are composed of remobilized mud sourced from hemipelagic sediments across the continental slope (Li et al., 2018). MTDs enter the Agadir Canyon from the Agadir Fairway but also occur as part of the Upper Canyon and Canyon Head stratigraphy (Figures 8, 9, 13), which indicates slope failures are frequent across a wide area of the Moroccan continental slope. Inside the canyon, the MTDs are stacked in multiple tiers and heal the previously deeply incised topography (Figures 4, 6). This process is contradictory as MTDs are usually considered as erosional processes that transport material away from the slopes (Talling et al., 2012). Most buried MTDs have sharp, flat tops with negative polarity reflectors (Figures 4, 10–13). The negative polarity is likely related to compaction of clay-rich sediments at the base of the landslide during deposition (Sawyer et al., 2009). The flat top is likely related to erosion of the MTD tops by of sandy turbidites flushing the canyon (Canals et al., 2006). The late Quaternary canyon activity has been well documented, showing sandy turbidites are sourced from shelf sediments that accumulate in the canyon heads (Hunt, 2017; Hunt et al., 2013; Stevenson et al., 2014; Wynn et al., 2002). These sandy turbidity currents have been infrequent over the past 250 kyr (1 every 5–10 kyr; Hunt et al. (2013)), however, individual flows can be giant a highly destructive. One example from ∼60 ka eroded along the entire length of the canyon to an average depth of 30 m over an area about 4,500 km2 (Böttner et al., 2024). This explains why the main Agadir Canyon has such a flat canyon floor and why most buried MTDs have sharp, flat tops (Figures 4, 10–13).
This binary system of sandy turbidites from the shelf that flush the canyon and muddy landslides that fill and heal the resulting topography persists throughout the whole lifespan of Agadir Canyon. However, Unit C is distinct from Unit B and separated by a clear erosional hiatus. This series of incisions was likely influenced by a ∼60 m sea level drop from the Middle to Late Miocene (Miller et al., 2020) in combination with hinterland becoming more arid and concurrent change of the North African vegetation from tropical forests to the Sahara Desert (Jacobs, 2004; Le Houérou, 1997; Wolfe, 1985). From the Late Miocene, high volcanic activity in the catchment area of the Souss river will have further promoted sediment input into the Agadir Canyon (Berrahma and Delaloye, 1989; Liégeois et al., 2005).
In the Pliocene, the Massa Canyon initially developed as an aggrading sand-rich channel-levee system before switching to a highly erosive system during the Pleistocene (Figures 5, 13). The Massa canyon development was favored by eustatic sea level drop of ∼40 m (Miller et al., 2020) that would have allowed terrestrial river systems to extend across the shelf and drive increased sediment supply into the canyon heads. The switch to highly erosive system was likely caused by the Alpine collision at the Pliocene-Pleistocene boundary and potential mantle upwelling driving uplift of the onshore Atlas region, which increased onshore erosion rates (Benabdellouahed et al., 2017; Charton et al., 2021; Frizon de Lamotte et al., 2009).
In the Quaternary, climatic improvement and stability were marked by prevailing zonal wind circulation and increased continental wetness. Enhanced fertility, driven by coastal upwelling and substantial river discharge, contributed to increased sediment input into the Agadir Canyon (Freudenthal et al., 2002; Kuhlmann et al., 2004; Sarnthein et al., 1981; Sarnthein et al., 1982). In the proximal canyon head area, the re-routing of these sediments by the abundant salt domes caused further development of the pronounced tributaries (Figures 3, 5, 8, 9). From the Pliocene onwards, all tributaries connect into one conduit that forms the main Agadir Canyon (Figures 3, 9). During high sea level of the Quaternary interglacial phases, bottom currents actively redistribute sediments along the shelf and transport them northward from the Souss and Massa rivers into the Agadir Canyon head area (Figure 7).
The Canary Island seamounts likely play a minor role in adding sediments to the system in the Quaternary as our data show no landslides or localized failure from the CISP into the canyon (Figures 3, 13). But the seamounts continue to shape the canyon’s morphology and thalweg through time (Figure 3). For example, the prominent 90° turn at the canyon’s mouth is forced by the Essaouira seamount (Figure 3), which also caused the canyon to form an overspill channel that likely accommodates the top parts of the powerful flows that flush the canyon (Figure 12).
From a suite of seismic profiles tied to DSPD Site 415, we document the evolution of the Agadir Canyon from inception to present-day morphology. Canyon stratigraphy is dominated by thick MTDs that are separated by sporadic thin sandy turbidite deposits. Canyon initiation occurred in the Middle Miocene with a wide, shallow incision followed by several shallow incision and fill cycles in the Later Middle Miocene and Late Miocene. Temporal relationships are established between canyon stratigraphy and a suite of potential controls on canyon development including eustatic sea level, salt movement, seamount growth and the developing Atlas Mountains in the hinterland. Canyon inception coincides with Middle Miocene sea-level fall and the growth of Conception Bank, which likely drove increased sediment supply from the Moroccan Margin into the Agadir Canyon. However, there is a striking disparity between the depth of intra-canyon incision surfaces (up to ∼100 m) and the height of the present-day canyon walls (up to ∼1.2 km). This suggests that the canyon may have incised deeply at the surface reflector (geologically recent), or that the canyon was not primarily developed by erosion, rather, constructive processes have elevated the canyon margins high above the thalweg.
The data that support the findings of this study are available from the corresponding author upon reasonable request. Parts of the data are freely available from Pangaea Data Repository (https://www.pangaea.de/).
CB: Conceptualization, Formal Analysis, Investigation, Methodology, Visualization, Writing–original draft, Writing–review and editing. CS: Conceptualization, Formal Analysis, Investigation, Methodology, Supervision, Writing–original draft, Writing–review and editing. JG: Formal Analysis, Methodology, Writing–review and editing. SK: Conceptualization, Funding acquisition, Investigation, Project administration, Resources, Supervision, Writing–review and editing.
The author(s) declare that financial support was received for the research, authorship, and/or publication of this article. This work has been funded through the German Research Foundation (DFG). Co-funded by the European Union under grant agreement No 101060851. We acknowledge financial support by Land Schleswig-Holstein within the funding program Open Access Publikationsfonds.
We thank the captains, crews, and shipboard scientific parties of R/V Maria S. Merian cruises 32 and 113. We are thankful to the academic licensing programs of IHS for providing the Kingdom Seismic Interpretation Software, ESRI for providing the ArcGIS/Pro Software, Schlumberger for providing VISTA Seismic Processing Software and QPS for providing Qimera Software.
The authors declare that the research was conducted in the absence of any commercial or financial relationships that could be construed as a potential conflict of interest.
All claims expressed in this article are solely those of the authors and do not necessarily represent those of their affiliated organizations, or those of the publisher, the editors and the reviewers. Any product that may be evaluated in this article, or claim that may be made by its manufacturer, is not guaranteed or endorsed by the publisher.
Alves, T. M., Cartwright, J., and Davies, R. J. (2009). Faulting of salt-withdrawal basins during early halokinesis: effects on the Paleogene rio doce canyon system (espirito santo basin, Brazil). Aapg Bull. 93 (5), 617–652. doi:10.1306/02030908105
Ambar, I., Armi, L., Bower, A., and Ferreira, T. (1999). Some aspects of time variability of the Mediterranean Water off south Portugal. Deep-Sea Res. Pt I 46 (7), 1109–1136. doi:10.1016/s0967-0637(99)00006-0
Anka, Z., Séranne, M., Lopez, M., Scheck-Wenderoth, M., and Savoye, B. (2009). The long-term evolution of the Congo deep-sea fan: a basin-wide view of the interaction between a giant submarine fan and a mature passive margin (ZaiAngo project). Tectonophysics 470 (1-2), 42–56. doi:10.1016/j.tecto.2008.04.009
Arzola, R. G., Wynn, R. B., Lastras, G., Masson, D. G., and Weaver, P. P. E. (2008). Sedimentary features and processes in the Nazare and Setubal submarine canyons, west Iberian margin. Mar. Geol. 250 (1-2), 64–88. doi:10.1016/j.margeo.2007.12.006
Babault, J., Teixell, A., Arboleya, M. L., and Charroud, M. (2008). A late cenozoic age for long-wavelength surface uplift of the atlas mountains of Morocco. Terra nova. 20 (2), 102–107. doi:10.1111/j.1365-3121.2008.00794.x
Benabdellouahed, M., Klingelhoefer, F., Gutscher, M. A., Rabineau, M., Biari, Y., Hafid, M., et al. (2017). Recent uplift of the Atlantic Atlas (offshore West Morocco): tectonic arch and submarine terraces. Tectonophysics 706, 46–58. doi:10.1016/j.tecto.2017.03.024
Berrahma, M., and Delaloye, M. (1989). Données géochronologiques nouvelles sur le massif volcanique du Siroua (Anti-Atlas, Maroc). J. Afr. Earth Sci. (and the Middle East) 9 (3-4), 651–656. doi:10.1016/0899-5362(89)90049-3
Böttner, C., Stevenson, C. J., Englert, R., Schönke, M., Pandolpho, B. T., Geersen, J., et al. (2024). Extreme erosion and bulking in a giant submarine gravity flow. Sci Adv 10 (34), eadp2584. doi:10.1126/sciadv.adp2584
Burgess, P. M., and Hovius, N. (1998). Rates of delta progradation during highstands: consequences for timing of deposition in deep-marine systems. J Geol Soc London 155, 217–222. doi:10.1144/gsjgs.155.2.0217
Canals, M., Puig, P., de Madron, X. D., Heussner, S., Palanques, A., and Fabres, J. (2006). Flushing submarine canyons. Nature 444 (7117), 354–357. doi:10.1038/nature05271
Capella, W., Flecker, R., Hernández-Molina, F. J., Simon, D., Meijer, P. T., Rogerson, M., et al. (2019). Mediterranean isolation preconditioning the Earth System for late Miocene climate cooling. Sci Rep-Uk 9, 3795. doi:10.1038/s41598-019-40208-2
Caress, D. W., and Chayes, D. N. (1995). New software for processing sidescan data from sidescan-capable multibeam sonars. Oceans '95 Mts/Ieee - Challenges of Our Changing Global Environment, Conference Proceedings, Vols 1-3, 997–1000. doi:10.1109/OCEANS.1995.528558
Carter, R. C., Gani, M. R., Roesler, T., and Sarwar, A. K. M. (2016). Submarine channel evolution linked to rising salt domes, Gulf of Mexico, USA. Sediment Geol 342, 237–253. doi:10.1016/j.sedgeo.2016.06.021
Charton, R., Bertotti, G., Arnould, A. D., Redfern, J., and Storms, J. E. A. (2021). Low-temperature thermochronology as a control on vertical movements for semi-quantitative source-to-sink analysis: a case study for the Permian to Neogene of Morocco and surroundings. Basin Research 33 (2), 1337–1383. doi:10.1111/bre.12517
Covault, J. A. (2011). Submarine fans and canyon-channel systems: a review of processes, products, and models. Nature Education Knowledge 3 (10), 4.
Covault, J. A., Normark, W. R., Romans, B. W., and Graham, S. A. (2007). Highstand fans in the California borderland: the overlooked deep-water depositional systems. Geology 35 (9), 783–786. doi:10.1130/g23800a.1
Curray, J. R., Emmel, F. J., and Moore, D. G. (2002). The Bengal Fan: morphology, geometry, stratigraphy, history and processes. Mar Petrol Geol 19 (10), 1191–1223. doi:10.1016/s0264-8172(03)00035-7
Davies, R., Cartwright, J., Pike, J., and Line, C. (2001). Early Oligocene initiation of North Atlantic deep water formation. Nature 410 (6831), 917–920. doi:10.1038/35073551
Davison, I. (2005). Central atlantic margin basins of north west Africa: geology and hydrocarbon potential (Morocco to Guinea). J Afr Earth Sci 43 (1-3), 254–274. doi:10.1016/j.jafrearsci.2005.07.018
de Leeuw, J., Eggenhuisen, J. T., and Cartigny, M. J. B. (2018). Linking submarine channel-levee facies and architecture to flow structure of turbidity currents: insights from flume tank experiments. Sedimentology 65 (3), 931–951. doi:10.1111/sed.12411
Demenocal, P. B. (1995). Plio-pleistocene african climate. Science 270 (5233), 53–59. doi:10.1126/science.270.5233.53
Ercilla, G., Alonso, B., Perez-Belzuz, F., Estrada, F., Baraza, J., Farran, M., et al. (1998). Origin, sedimentary processes and depositional evolution of the Agadir turbidite system, central eastern Atlantic. J Geol Soc London 155, 929–939. doi:10.1144/gsjgs.155.6.0929
Frenz, M., Wynn, R. B., Georgiopoulou, A., Bender, V. B., Hough, G., Masson, D. G., et al. (2009). Provenance and pathways of late Quaternary turbidites in the deep-water Agadir Basin, northwest African margin. Int J Earth Sci 98 (4), 721–733. doi:10.1007/s00531-008-0313-4
Freudenthal, T., Meggers, H., Henderiks, J., Kuhlmann, H., Moreno, A., and Wefer, G. (2002). Upwelling intensity and filament activity off Morocco during the last 250,000 years. Deep Sea Research Part II Topical Studies in Oceanography 49 (17), 3655–3674. doi:10.1016/s0967-0645(02)00101-7
Frizon de Lamotte, D., Leturmy, P., Missenard, Y., Khomsi, S., Ruiz, G., Saddiqi, O., et al. (2009). Mesozoic and Cenozoic vertical movements in the Atlas system (Algeria, Morocco, Tunisia): an overview. Tectonophysics 475 (1), 9–28. doi:10.1016/j.tecto.2008.10.024
Fuhrmann, A., Kane, I. A., Clare, M. A., Ferguson, R. A., Schomacker, E., Bonamini, E., et al. (2020). Hybrid turbidite-drift channel complexes: an integrated multiscale model. Geology 48 (6), 562–568. doi:10.1130/g47179.1
Gamboa, D., Alves, T., Cartwright, J., and Terrinha, P. (2010). MTD distribution on a 'passive' continental margin: the Espirito Santo Basin (SE Brazil) during the Palaeogene. Mar Petrol Geol 27 (7), 1311–1324. doi:10.1016/j.marpetgeo.2010.05.008
Gamboa, D., Omira, R., Piedade, A., Terrinha, P., Roque, C., and Zitellini, N. (2021). Destructive episodes and morphological rejuvenation during the lifecycles of tectonically active seamounts: insights from the Gorringe Bank in the NE Atlantic. Earth and Planetary Science Letters 559, 116772. doi:10.1016/j.epsl.2021.116772
Gouiza, M., Charton, R., Bertotti, G., Andriessen, P., and Storms, J. E. A. (2017). Post-Variscan evolution of the Anti-Atlas belt of Morocco constrained from low-temperature geochronology. Int J Earth Sci 106 (2), 593–616. doi:10.1007/s00531-016-1325-0
Hafid, M., Salem, A. A., and Bally, A. W. (2000). The western termination of the jebilet-high atlas system (offshore Essaouira basin, Morocco). Mar Petrol Geol 17 (3), 431–443. doi:10.1016/s0264-8172(98)00082-8
He, Y. L., Xie, X. N., Kneller, B. C., Wang, Z. F., and Li, X. S. (2013). Architecture and controlling factors of canyon fills on the shelf margin in the Qiongdongnan Basin, northern South China Sea. Mar Petrol Geol 41, 264–276. doi:10.1016/j.marpetgeo.2012.03.002
Heijnen, M. S., Clare, M. A., Cartigny, M. J., Talling, P. J., Hage, S., Pope, E. L., et al. (2022a). Fill, flush or shuffle: how is sediment carried through submarine channels to build lobes? Earth and Planetary Science Letters 584, 117481. doi:10.1016/j.epsl.2022.117481
Heijnen, M. S., Mienis, F., Gates, A. R., Bett, B. J., Hall, R. A., Hunt, J., et al. (2022b). Challenging the highstand-dormant paradigm for land-detached submarine canyons. Nat Commun 13 (1), 3448. doi:10.1038/s41467-022-31114-9
Hernández-Guerra, A., Fraile-Nuez, E., Borges, R., López-Laatzen, F., Vélez-Belchí, P., Parrilla, G., et al. (2003). Transport variability in the Lanzarote passage (eastern boundary current of the North Atlantic subtropical Gyre). Deep-Sea Res Pt I 50 (2), 189–200. doi:10.1016/s0967-0637(02)00163-2
Hernández-Molina, F. J., Stow, D. A. V., Alvarez-Zarikian, C. A., Acton, G., Bahr, A., Balestra, B., et al. (2014). Onset of mediterranean outflow into the North atlantic. Science 344 (6189), 1244–1250. doi:10.1126/science.1251306
Herold, N., Huber, M., Müller, R. D., and Seton, M. (2012). Modeling the Miocene climatic optimum: ocean circulation. Paleoceanography 27. doi:10.1029/2010pa002041
Heyman, M. (1989). Tectonic and depositional history of the Moroccan continental margin: Chapter 21: European-African Margins.
Hinz, K., Dostmann, H., and Fritsch, J. (1982). “The continental margin of Morocco: seismic sequences, structural elements and geological development,” in Paper presented at Geology of the Northwest African continental margin. Springer.
Hunt, J. E. (2017). Identifying and quantifying erosion beneath the deposits of long-runout turbidity currents along their pathway. Mar Geol 389, 32–51. doi:10.1016/j.margeo.2017.04.005
Hunt, J. E., Wynn, R. B., Talling, P. J., and Masson, D. G. (2013). Frequency and timing of landslide-triggered turbidity currents within the Agadir Basin, offshore NW Africa: are there associations with climate change, sea level change and slope sedimentation rates? Mar Geol 346, 274–291. doi:10.1016/j.margeo.2013.09.004
Jacobi, R. D., Rabinowitz, P. D., and Embley, R. W. (1975). Sediment waves on the Moroccan continental rise. Mar Geol 19 (5), M61–M67. doi:10.1016/0025-3227(75)90079-1
Jacobs, B. F. (2004). Palaeobotanical studies from tropical Africa: relevance to the evolution of forest, woodland and savannah biomes. Philosophical Transactions of the Royal Society of London. Series B Biological Sciences 359 (1450), 1573–1583. doi:10.1098/rstb.2004.1533
Janocko, M., Nemec, W., Henriksen, S., and Warchol, M. (2013). The diversity of deep-water sinuous channel belts and slope valley-fill complexes. Mar Petrol Geol 41, 7–34. doi:10.1016/j.marpetgeo.2012.06.012
Knoll, M., Hernández-Guerra, A., Lenz, B., Laatzen, F. L., Machín, F., Müller, T. J., et al. (2002). The eastern boundary current system between the canary islands and the african coast. Deep-Sea Res Pt Ii 49 (17), 3427–3440. doi:10.1016/s0967-0645(02)00105-4
Krastel, S., Wynn, R. B., Feldens, P., Schürer, A., Böttner, C., Stevenson, C., et al. (2016). Flow behaviour of a giant landslide and debris flow entering Agadir canyon, NW Africa. Adv Nat Tech Haz Res 41, 145–154. doi:10.1007/978-3-319-20979-1_14
Kuhlmann, H., Meggers, H., Freudenthal, T., and Wefer, G. (2004). The transition of the monsoonal and the N Atlantic climate system off NW Africa during the Holocene. Geophys Res Lett 31 (22). doi:10.1029/2004gl021267
Lancelot, Y., and Seibold, E. (1978). Initial reports of the deep sea drilling project. Washington: U.S. Government Printing Office. 41
Lancelot, Y., and Winterer, E. L. (1980). Initial reports of the deep sea drilling project, 50. (Washington: U.S. Government Printing Office), 353–428.
Lee, C., Nott, J. A., Keller, F. B., and Parrish, A. R. (2004). Seismic expression of the Cenozoic mass transport complexes, deepwater Tarfaya-Agadir Basin. offshore Morocco, paper presented at Offshore Technology Conference, OTC.
Le Houérou, H. N. (1997). Climate, flora and fauna changes in the Sahara over the past 500 million years. Journal of Arid Environments 37 (4), 619–647. doi:10.1006/jare.1997.0315
León, R., Palomino, D., Sánchez-Guillamón, O., Fernández-Salas, L. M., and Vázquez, J. T. (2022). Tectonic control on sedimentary dynamics in intraplate oceanic settings: a geomorphological image of the eastern Canary Basin and insights on its middle-upper Miocene to quaternary volcano-tectonic-sedimentary evolution. Mar Geol 445, 106737. doi:10.1016/j.margeo.2022.106737
León, R., Palomino, D., Vázquez, J. T., Medialdea, T., and Somoza, L. (2019). A new scenario for the mass transport deposits west Canary volcanic province. Earth and Planetary Science Letters 509, 27–37. doi:10.1016/j.epsl.2018.12.020
Li, W., Krastel, S., Alves, T. M., Urlaub, M., Mehringer, L., Schürer, A., et al. (2018). The Agadir Slide offshore NW Africa: morphology, emplacement dynamics, and potential contribution to the Moroccan turbidite system. Earth and Planetary Science Letters 498, 436–449. doi:10.1016/j.epsl.2018.07.005
Liégeois, J., Benhallou, A., Azzouni-Sekkal, A., Yahiaoui, R., and Bonin, B. (2005). The Hoggar swell and volcanism: reactivation of the Precambrian Tuareg shield during Alpine convergence and West African Cenozoic volcanism, in Plates, Plumes and Paradigms. Editors G. R. Foulger, J. H. Natland, D. C. Presnall, and D. L. Anderson (United States: Geological Society of America). doi:10.1130/0-8137-2388-4.379
McHargue, T., Pyrcz, M. J., Sullivan, M. D., Clark, J. D., Fildani, A., Romans, B. W., et al. (2011). Architecture of turbidite channel systems on the continental slope: patterns and predictions. Mar Petrol Geol 28 (3), 728–743. doi:10.1016/j.marpetgeo.2010.07.008
Micheels, A., Eronen, J., and Mosbrugger, V. (2009). The Late Miocene climate response to a modern Sahara desert. Global Planet Change 67 (3-4), 193–204. doi:10.1016/j.gloplacha.2009.02.005
Miller, K. G., Browning, J. V., Schmelz, W. J., Kopp, R. E., Mountain, G. S., and Wright, J. D. (2020). Cenozoic sea-level and cryospheric evolution from deep-sea geochemical and continental margin records. Sci Adv 6 (20), eaaz1346. doi:10.1126/sciadv.aaz1346
Miramontes, E., Eggenhuisen, J. T., Jacinto, R. S., Poneti, G., Pohl, F., Normandeau, A., et al. (2020). Channel-levee evolution in combined contour current-turbidity current flows from flume-tank experiments. Geology 48 (4), 353–357. doi:10.1130/g47111.1
Mourlot, Y., Calvès, G., Clift, P. D., Baby, G., Chaboureau, A. C., and Raisson, F. (2018). Seismic stratigraphy of cretaceous eastern central Atlantic Ocean: basin evolution and palaeoceanographic implications. Earth and Planetary Science Letters 499, 107–121. doi:10.1016/j.epsl.2018.07.023
Neumaier, M., Back, S., Littke, R., Kukla, P. A., Schnabel, M., and Reichert, C. (2016). Late cretaceous to cenozoic geodynamic evolution of the atlantic margin offshore Essaouira (Morocco). Basin Research 28 (5), 712–730. doi:10.1111/bre.12127
Normandeau, A., Campbell, D. C., and Cartigny, M. J. B. (2019). The influence of turbidity currents and contour currents on the distribution of deep-water sediment waves offshore eastern Canada. Sedimentology 66 (5), 1746–1767. doi:10.1111/sed.12557
Palomino, D., Vázquez, J. T., Somoza, L., León, R., López-González, N., Medialdea, T., et al. (2016). Geomorphological features in the southern Canary Island. Volcanic Province: the importance of volcanic processes and massive slope instabilities associated with seamounts. Geomorphology 255, 125–139. doi:10.1016/j.geomorph.2015.12.016
Pandolpho, B. T., Urlaub, M., Berndt, C., and Bialas, J. (2024). Identification and differentiation of vertical movement through morphological changes and stratigraphic imprint: two distinct uplifting mechanisms in the upper Calabrian accretionary wedge, western Ionian Sea. Basin Research 36 (1). doi:10.1111/bre.12819
Paull, C. K., Schlining, B., Ussler, W., Lundsten, E., Barry, J. P., Caress, D. W., et al. (2010). “Submarine mass transport within monterey canyon: benthic disturbance controls on the distribution of chemosynthetic biological communities,” in Submarine Mass Movements and Their Consequences. Advances in Natural and Technological Hazards Research. Editor D. C. Mosheret al. (Dordrecht: Springer), 28. doi:10.1007/978-90-481-3071-9_19
Peakall, J., McCaffrey, B., and Kneller, B. (2000). A process model for the evolution, morphology, and architecture of sinuous submarine channels. J Sediment Res 70 (3), 434–448. doi:10.1306/2dc4091c-0e47-11d7-8643000102c1865d
Pirmez, C., and Flood, R. (1995). “Morphology and structure of amazon channel,” in Proceedings of the ocean drilling program. Part A, initial report. paper presented at.
Pratson, L. F., Nittrouer, C. A., Wiberg, P. L., Steckler, M. S., Swenson, J. B., Cacchione, D. A., et al. (2007). Seascape evolution on clastic continental shelves and slopes. Continental margin sedimentation From sediment transport to sequence stratigraphy, 339–380. doi:10.1002/9781444304398.ch7
Sachse, V. F., Wenke, A., Littke, R., Jabour, H., Kluth, O., and Zühlke, R. (2016). 2D petroleum system analysis of the Tarfaya Basin, on-offshore Morocco, north Africa. Mar Petrol Geol 77, 1108–1124. doi:10.1016/j.marpetgeo.2016.08.006
Sahabi, M., Aslanian, D., and Olivet, J.-L. (2004). Un nouveau point de départ pour l'histoire de l'Atlantique central. Comptes Rendus Geoscience 336 (12), 1041–1052. doi:10.1016/j.crte.2004.03.017
Sarnthein, M., Tetzlaff, G., Koopmann, B., Wolter, K., and Pflaumann, U. (1981). Glacial and interglacial wind regimes over the eastern subtropical Atlantic and North-West Africa. Nature 293 (5829), 193–196. doi:10.1038/293193a0
Sarnthein, M., Thiede, J., Pflaumann, U., Erlenkeuser, H., Fütterer, D., Koopmann, B., et al. (1982). “Atmospheric and oceanic circulation patterns off Northwest Africa during the past 25 million years,” in Paper presented at Geology of the Northwest African continental margin. Springer.
Sawyer, D. E., Flemings, P. B., Dugan, B., and Germaine, J. T. (2009). Retrogressive failures recorded in mass transport deposits in the Ursa Basin, Northern Gulf of Mexico. J Geophys Res-Sol Ea 114. doi:10.1029/2008jb006159
Schwenk, T., Spiess, V., Breitzke, M., and Hübscher, C. (2005). The architecture and evolution of the Middle Bengal Fan in vicinity of the active channel-levee system imaged by high-resolution seismic data. Mar Petrol Geol 22 (5), 637–656. doi:10.1016/j.marpetgeo.2005.01.007
Seibold, E. (1982). The northwest African continental margin—an introduction, in Geology of the Northwest African continental margin. Springer. 3–20.
Smith, M. E., Werner, S. H., Buscombe, D., Finnegan, N. J., Sumner, E. J., and Mueller, E. R. (2018). Seeking the shore: evidence for active submarine canyon head incision due to coarse sediment supply and focusing of wave energy. Geophys Res Lett 45 (22), 12403–12413. doi:10.1029/2018gl080396
Stevenson, C. J., Talling, P. J., Masson, D. G., Sumner, E. J., Frenz, M., and Wynn, R. B. (2014). The spatial and temporal distribution of grain-size breaks in turbidites. Sedimentology 61 (4), 1120–1156. doi:10.1111/sed.12091
Syvitski, J. P. M., Peckham, S. D., Hilberman, R., and Mulder, T. (2003). Predicting the terrestrial flux of sediment to the global ocean: a planetary perspective. Sediment Geol 162 (1-2), 5–24. doi:10.1016/s0037-0738(03)00232-x
Talling, P. J., Masson, D. G., Sumner, E. J., and Malgesini, G. (2012). Subaqueous sediment density flows: depositional processes and deposit types. Sedimentology 59 (7), 1937–2003. doi:10.1111/j.1365-3091.2012.01353.x
Talling, P. J., Wynn, R. B., Masson, D. G., Frenz, M., Cronin, B. T., Schiebel, R., et al. (2007). Onset of submarine debris flow deposition far from original giant landslide. Nature 450 (7169), 541–544. doi:10.1038/nature06313
Tari, G., and Jabour, H. (2013). Salt tectonics along the Atlantic margin of Morocco. Geological Society, London, Special Publications 369 (1), 337–353. doi:10.1144/sp369.23
Teixell, A., Arboleya, M. L., Julivert, M., and Charroud, M. (2003). Tectonic shortening and topography in the central High Atlas (Morocco). Tectonics 22 (5). doi:10.1029/2002tc001460
Teixell, A., Ayarza, P., Zeyen, H., Fernàndez, M., and Arboleya, M. L. (2005). Effects of mantle upwelling in a compressional setting:: the Atlas Mountains of Morocco. Terra Nova 17 (5), 456–461. doi:10.1111/j.1365-3121.2005.00633.x
Teixell, A., Bertotti, G., Frizon de Lamotte, D., and Charroud, M. (2010). Erratum to “The geology of vertical movements of the lithosphere: an overview” [Tectonophysics 475 (2009), 1–8]. Tectonophysics 485 (1-4), 332. doi:10.1016/j.tecto.2009.11.019
Tesón, E., Pueyo, E. L., Teixell, A., Barnolas, A., Agustí, J., and Furió, M. (2010). Magnetostratigraphy of the ouarzazate basin: implications for the timing of deformation and mountain building in the high atlas mountains of Morocco. Geodin Acta 23 (4), 151–165. doi:10.3166/ga.23.151-165
Uchupi, E., Emery, K. O., Bowin, C. O., and Phillips, J. D. (1976). Continental margin off western Africa: Senegal to Portugal. AAPG Bulletin 60 (5), 809–878. doi:10.1306/c1ea35be-16c9-11d7-8645000102c1865d
van den Bogaard, P. (2013). The origin of the canary island seamount province - new ages of old seamounts. Sci Rep-Uk 3, 2107. doi:10.1038/srep02107
Via, R. K., and Thomas, D. J. (2006). Evolution of atlantic thermohaline circulation: early Oligocene onset of deep-water production in the North atlantic. Geology 34 (6), 441–444. doi:10.1130/g22545.1
von Rad, U., Fagerberg, E. M., and Laughter, F. H. (1979). Initial Reports of the Deep Sea Drilling Project, 47, Part 1: Covering Leg 47, Part 1 of the Cruises of the Drilling Vessel “Glomar Challenger”, Las Palmas, Canary Islands to Vigo, Spain, March-April 1976. US Government Printing Office, Washington.
Von Rad, U., and Wissmann, G. (1982). Initial reports of the deep sea drilling project, Vol. 47, Part 1: covering leg 47, Part 1 of the cruises of the drilling vessel “Glomar Challenger”, Las Palmas, Canary Islands to Vigo, Spain, March–April, 1976. Washington: US Government Printing Office.
Wenke, A. A. O. (2014). Sequence stratigraphy and basin analysis of the meso-to cenozoic tarfaya-laâyoune basins, on-and offshore Morocco.
Wolfe, J. A. (1985). Distribution of major vegetational types during the Tertiary. The carbon cycle and atmospheric CO2 natural variations Archean to present 32, 357–375. doi:10.1029/gm032p0357
Wynn, R. B., Masson, D. G., Stow, D. A. V., and Weaver, P. P. E. (2000). The Northwest African slope apron: a modern analogue for deep-water systems with complex seafloor topography. Mar Petrol Geol 17 (2), 253–265. doi:10.1016/s0264-8172(99)00014-8
Wynn, R. B., Weaver, P. P. E., and Masson, D. G. (2003). “Canyon switching in the Moroccan turbidite system,” in Northwest african margin, European margin sediment dynamics: side-scan Sonar and seismic images, 289–291.
Wynn, R. B., Weaver, P. P. E., Masson, D. G., and Stow, D. A. V. (2002). Turbidite depositional architecture across three interconnected deep-water basins on the north-west African margin. Sedimentology 49 (4), 669–695. doi:10.1046/j.1365-3091.2002.00471.x
Keywords: submarine canyon, canyon evolution, gravity flow deposits, NW Africa, DSDP, seismic analyses, sediment transport
Citation: Böttner C, Stevenson CJ, Geersen J and Krastel S (2025) The spatiotemporal evolution of a giant submarine canyon system – the Agadir Canyon. Front. Earth Sci. 13:1400289. doi: 10.3389/feart.2025.1400289
Received: 13 March 2024; Accepted: 21 February 2025;
Published: 17 March 2025.
Edited by:
Dimitris Sakellariou, Hellenic Centre for Marine Research (HCMR), GreeceReviewed by:
Luis Somoza, Instituto Geológico y Minero de España (IGME), SpainCopyright © 2025 Böttner, Stevenson, Geersen and Krastel. This is an open-access article distributed under the terms of the Creative Commons Attribution License (CC BY). The use, distribution or reproduction in other forums is permitted, provided the original author(s) and the copyright owner(s) are credited and that the original publication in this journal is cited, in accordance with accepted academic practice. No use, distribution or reproduction is permitted which does not comply with these terms.
*Correspondence: Christoph Böttner, Y2hyaXN0b3BoLmJvZXR0bmVyQGdlby5hdS5kaw==
†ORCID: Christoph Böttner, orcid.org/0000-0001-7321-0699
Disclaimer: All claims expressed in this article are solely those of the authors and do not necessarily represent those of their affiliated organizations, or those of the publisher, the editors and the reviewers. Any product that may be evaluated in this article or claim that may be made by its manufacturer is not guaranteed or endorsed by the publisher.
Research integrity at Frontiers
Learn more about the work of our research integrity team to safeguard the quality of each article we publish.