- 1Natural Resources Survey Institute of Heilongjiang Province, Heilongjiang Provincial Bureau of Geology and Mineral Resources, Harbin, China
- 2MNR Key Laboratory of Metallogeny and Mineral Assessment, Institute of Mineral Resources, Chines Academy of Geological Sciences, Beijing, China
- 3Harbin Center for Integrated Natural Resources Survey, Chines Academy of Geological Sciences, Observation and Research Station of Earth Critical Zone in Black Soil, Ministry of Natural Resources, Harbin, China
- 4The Fifth Geological Exploration Institute of Heilongjiang Province, Heilongjiang Provincial Bureau of Geology and Mineral Resources, Harbin, China
- 5Department of Earth Sciences, Karakoram International University, Gilgit, Pakistan
The occurrence of intrusive rocks within the Xinlin area, northeastern China, provides insights into the Neoproterozoic–Mesozoic geodynamic setting of the Erguna block. In this study, we present petrographic, geochemical, and geochronological data on intrusive rocks from the Xinlin area. Zircon U–Pb and muscovite 40Ar/39Ar geochronology reveal that magmatism occurred during the Neoproterozoic (ca. 864.98 Ma), Early Ordovician (ca. 470.0 Ma), Late Carboniferous (ca. 306.9 Ma), Early Permian (ca. 296.9 Ma), and Early Cretaceous (ca. 117.8 Ma) periods. The Neoproterozoic and Early Ordovician intermediate–mafic intrusive rocks have low Rb/Sr contents, high Mg#, and weakly negative Eu anomalies. These results suggest that the magma sources of these rocks varied: intermediate–acidic magmas were derived from the lower crust, and intermediate–mafic magmas originated from the mantle and were subsequently contaminated by crustal material. In contrast, the Late Carboniferous, Early Permian, Late Triassic–Early Jurassic, and Early Cretaceous intermediate–acidic intrusive rocks display high Rb/Sr contents, low Mg#, and strongly negative Eu anomalies, indicating derivation from the partial melting of the lower crust. Our findings, along with previous studies, suggest that Neoproterozoic intrusive rocks were formed during the breakup of the Rodinia supercontinent. The Paleozoic intrusive rocks are associated with the collision and amalgamation of the Erguna and Xing’an blocks, as well as the Songnen and Xing’an blocks. Early Mesozoic intrusive rocks were developed during the subduction of the Mongol-Okhotsk oceanic intracontinental system. Finally, the late Mesozoic intrusive rocks were formed in a non-orogenic extensional setting, potentially linked to the final closure of the Mongol-Okhotsk Ocean or the rollback of the Paleo-Pacific Plate.
1 Introduction
The Great Xing’an Range is located within the suture zone between the Siberian and North China cratons (Figure 1A; Liu et al., 2017). This area records evidence of several tectonic events, including the convergence and breakup of the Rodinia supercontinent during the Proterozoic (Zhao, 2017), the closure of the Paleo-Asian Ocean during the late Paleozoic, the closure of the Mongol-Okhotsk Ocean (MOO) in the late Mesozoic, and the subduction of Paleo-Pacific oceanic plate since the Jurassic (Xu et al., 2013). The tectonic setting and geochemical characteristics of the magmatic rocks in this area are notably complex, featuring diverse rock types. Consequently, the region has garnered significant interest from geologists both domestically and internationally (Jia et al., 2011; Liu et al., 2011; Ge et al., 2015; Ouyang et al., 2015; Zheng., 2015).
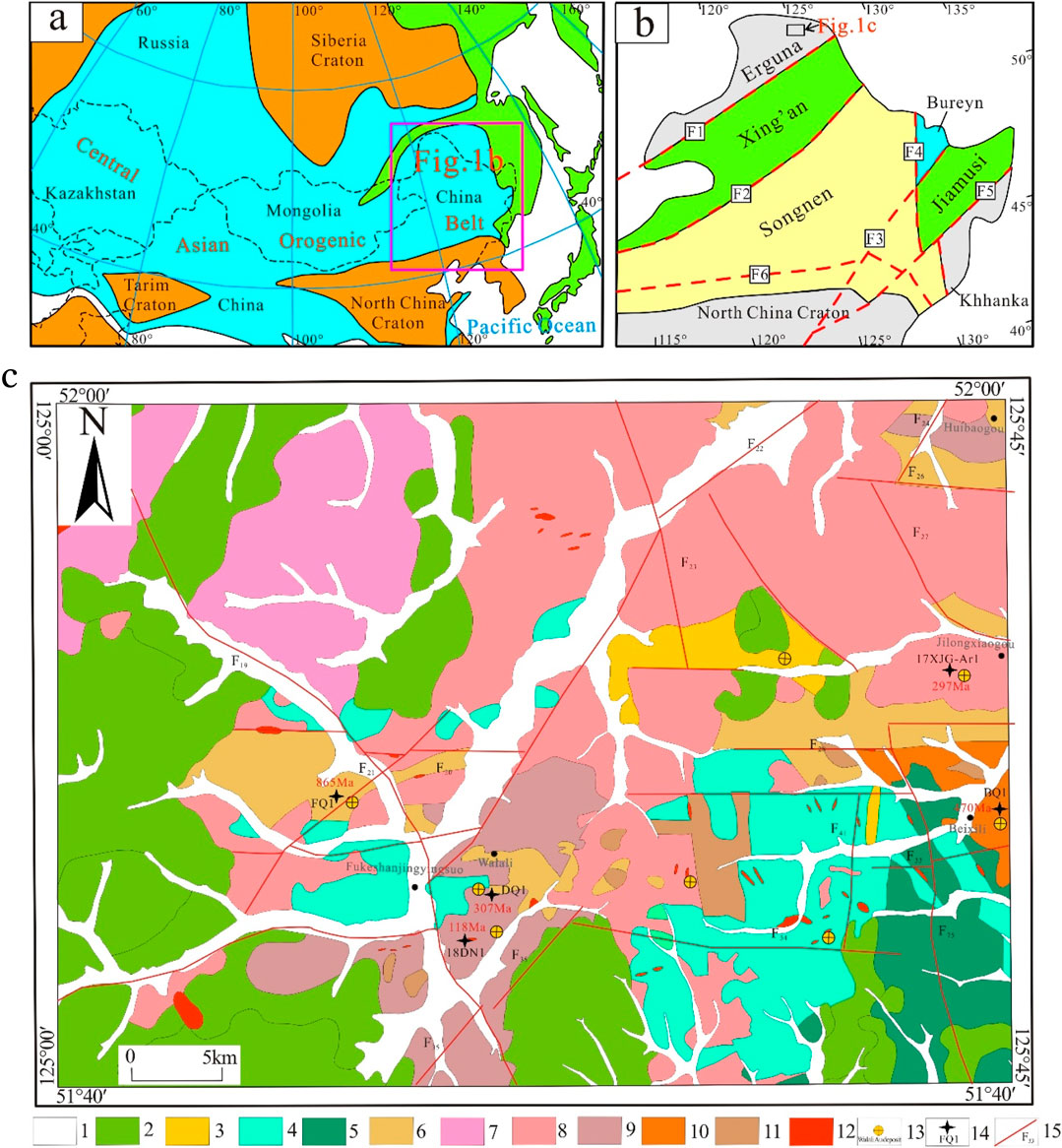
Figure 1. (A) Location of the study area in the Central Asian Orogenic Belt (modified after Jahn, 2004). (B) Tectonic map of the Great Xing’an Range, showing its structures and tectonic belts (modified after Liu et al., 2017). (C) Geological map of the study area showing sampling locations. 1, Quaternary; 2, Early Cretaceous volcanic rocks; 3, Silurian–Devonian strata; 4, Ordovician strata; 5, Cambrian strata; 6, Neoproterozoic Xinghuadukou rock group; 7, Late Triassic–Early Jurassic intrusive rocks; 8, Early Permian intrusive rocks; 9, Late Carboniferous intrusive rocks; 10, Early Ordovician intrusive rocks; 11, Neoproterozoic intrusive rocks; 12, vein rock; 13, Au deposit location and number; 14, sample location and number; 15, fault, including fault number.
The Xinlin area, located in the Erguna block, is an important gold-mining district (Figure 1B; Liu et al., 2017). The area hosts well-developed intrusive rocks, primarily comprising Neoproterozoic, Paleozoic, and Mesozoic formations (Figure 1C). Extensive research has been conducted on the chronology, geochemistry, and tectonic framework of the magmatic rocks in this area (Wu et al., 2009; Wang et al., 2012; Shi et al., 2013; Feng, 2015; Liu et al., 2016; Tang, 2016; Zhao, 2017; Qian et al., 2018; Liu et al., 2021). However, debates persist regarding the tectonic setting and origin of the Neoproterozoic–Mesozoic intrusive rocks in the Erguna block.
In this study, we present new petrographic, geochemical, and geochronological data on the different types of intrusive rocks within the Xinlin area. We discuss their petrogenesis and reconstruct the tectonic evolution of the region from the Neoproterozoic to the Mesozoic periods.
2 Geological background
The strata exposed in the study area include the Neoproterozoic Xinghuadukou Group; the Lower Cambrian Hongshenggou, Sanyigou, and Jiaobuleshihe formations; the Lower Ordovician Kunasenhe, Huangbanji, and Daxiyikanghe formations; the Lower–Middle Ordovician Tongshan and Duobaoshan formations; the Upper Ordovician Anniangniangqiao and Aihui formations; the Lower Silurian Huanghuagou Formation; the Upper Silurian to Middle Devonian Niqiuhe Formation; and the Lower Cretaceous Longjiang, Guanghua, and Ganhe formations (Figure 1C; HBGMR, 1997).
Intrusive rocks are well-developed in the study area, and they primarily include Neoproterozoic, Paleozoic, and Mesozoic intrusive rocks (Figure 1C). The Neoproterozoic magmatic rocks are mainly distributed in the northern part of Walali, with lithologies predominantly comprising gneiss and schist. The Paleozoic intrusive rocks exposed in the study area are mainly Hercynian and Indosinian in origin. Mesozoic intrusive rocks can be divided into early Yanshanian and late Yanshanian phases. The early Yanshanian intrusive rocks are mainly distributed in the northwest of the study area, with lithologies mainly consisting of monzonitic granite and syenite granite, which are dated to the late Triassic–early Jurassic. The late Yanshanian magmatic rocks are less exposed and show scattered distribution characteristics across the study area, with lithologies primarily comprising granite veins and diorite veins. The majority of the faults within the study area are normal or strike-slip faults, predominantly trending NW–SE and NE–SW, with some trending N–S and E–W. These faults are interpreted to have formed in an extensional setting (Shao and Mu, 1999; Figure 1C).
2.1 Petrology
2.1.1 Neoproterozoic intrusive rocks
The Neoproterozoic intrusive rocks outcrop in the northern part of Walali within the study area. These rocks have undergone regional metamorphism. The dominant lithology is hornblende gneiss (Figure 1C). The hornblende gneisses are gray–black, exhibit a sheet-like, columnar, granular, crystalloblastic structure, and are primarily composed of plagioclase (50 vol.%), quartz (25 vol.%), biotite (20 vol.%), and amphibole (5 vol.%) (Figures 2A, B).
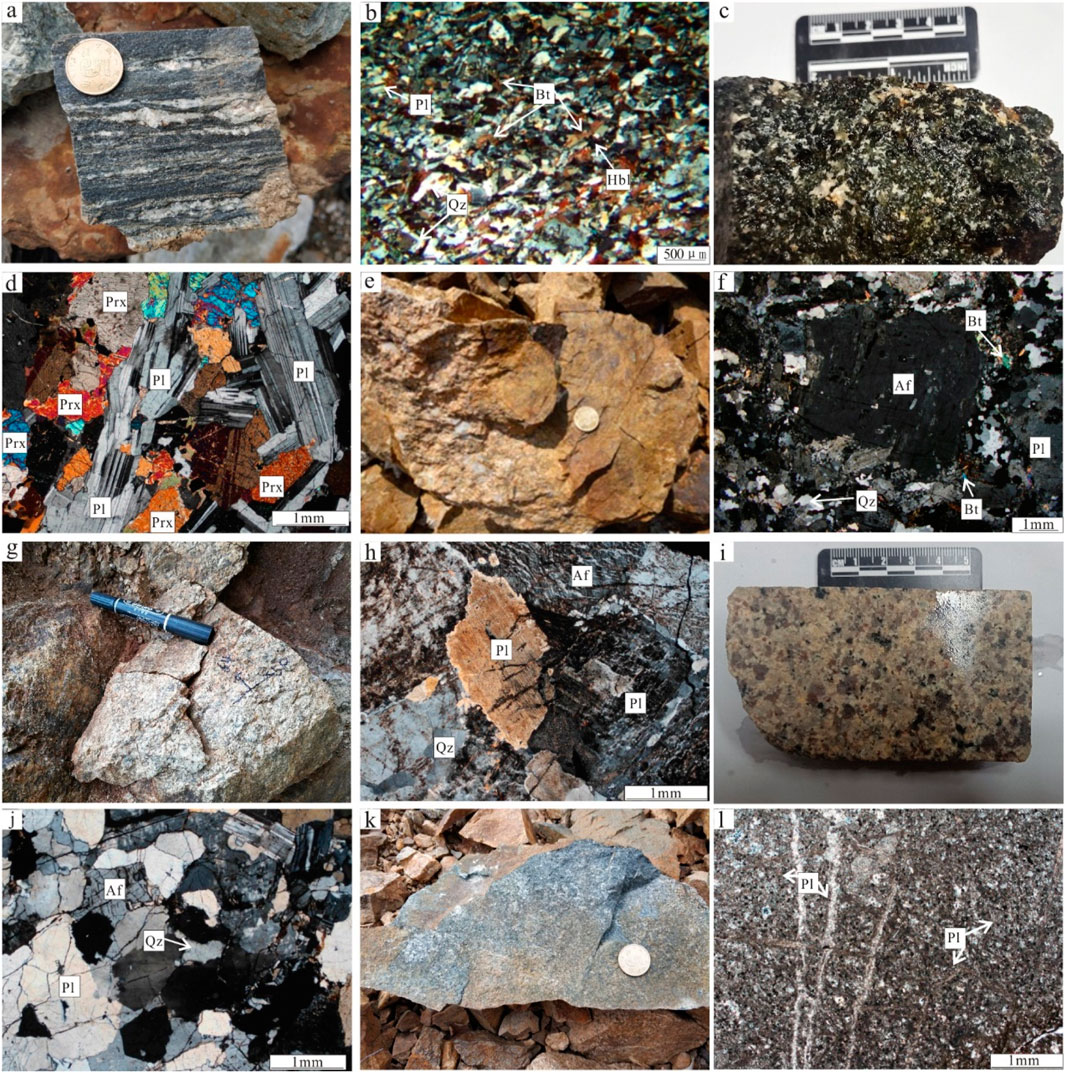
Figure 2. Photomicrographs and field photographs of intrusive rocks in the study area. (A) Hornblende gneisses; (B) hornblende gneisses (+); (C) gabbro; (D) gabbro (+); (E) monzonitic granite; (F) monzonitic granite (+); (G) potash feldspar granite; (H) potash feldspar granite (+); (I) monzonitic granite; (J) monzonitic granite (+); (K) diorite; (L) diorite (+). Af, alkali feldspar; Pl, plagioclase; Qz, quartz; Hb, hornblende; Bt, biotite; Prx,pyroxene.
2.1.2 Paleozoic intrusive rocks
Paleozoic intrusive rocks primarily include Early Ordovician, Late Carboniferous, and Early Permian intrusive rocks in the study area. The Early Ordovician intrusive rocks mainly outcrop in the Beixili region of the study area. The predominant lithology is gabbro (Figure 1C). The gabbros are gray–black, display a cataclastic structure, and are mainly composed of plagioclase (70 vol.%), pyroxene (15 vol.%), biotite (10 vol.%), and hornblende (5 vol.%) (Figures 2C,D). The Late Carboniferous intrusive rocks outcrop mainly near the Walali gold deposit. The dominant lithologies are monzonitic granite (Figures 2E,F) and potash feldspar granite (Figures 2G,H). The Early Permian intrusive rocks extensively outcrop in the northeastern part of the study area. The lithologies are mainly monzonitic granite and syenogranite.
2.1.3 Early Cretaceous intrusive rocks
Mesozoic intrusive rocks primarily include Late Triassic–Early Jurassic and Early Cretaceous rocks in the study area. The Late Triassic–Early Jurassic intrusive rocks are widely exposed in the northwestern part of the study area. The predominant lithologies are monzonitic granite (Figures 2I,J) and syenogranite. The Early Cretaceous intrusive rocks occur as scattered small exposures resembling “rock trees” across the study area. The dominant lithologies are granite and diorite veins (Figures 2K,L).
3 Sample collection and analytical methods
3.1 Sample collection
Four rock samples were collected for zircon U–Pb geochronology, with rock types including hornblende gneiss (FQ1), gabbro (BQ1), monzonitic granite (DQ1), and diorite (18DN1). One sample was collected for muscovite Ar–Ar geochronology (sample 17XJG-Ar1). Twenty-seven samples were collected for whole-rock geochemical analyses, with rock types including six hornblende gneisses (samples FQ1, FQ2, FQ4, FQ5, FQ7, and FQ8), five gabbros (samples BQ1, BQ2, BQ3, BQ4, and BQ5), eight monzonitic granites (samples DQ1, DQ2, DQ3, P60GS3, GS1179, GS4075, P54GS1, and P52GS30), three potash feldspar granites (samples WQ15, WQ16, and WQ17), three syenogranites (samples P52GS3, GS4071, and GS 2065), one alkali feldspar granite (sample P50GS9), and one diorite (sample P63GS6).
3.2 Analytical methods
The zircon grains were separated from the crushed samples by using conventional heavy liquid and magnetic techniques, and cathodoluminescence (CL) imaging and zircon U–Pb dating were conducted at the Beijing Createch Testing Technology, Beijing, China. The ICPMSDataCal (version 9.9; Liu et al., 2010) and Isoplot (version 3.0; Ludwig, 2003) programs were used for data reduction. Common Pb was corrected following the method outlined by Andersen (2002). Analytical uncertainties are reported at the 95% (2σ) confidence level.
The muscovite samples were collected from granite closely associated with mineralization. The correction factors used for interfering argon isotopes derived from Ca and K were (39 Ar/ 37 Ar)Ca = 8.06 × 10−4, (36 Ar/37 Ar)Ca = 2.389 × 10−4, and (40 Ar/39 Ar) K = 5.872 × 10−3. 37Ar was corrected for radioactive decay, and the 40K decay constant is λ = 5.543 × 10−10a−1 (Steiger and Jager, 1977). The plateau age and the positive and negative isochrones (Ludwig, v2.49) were calculated using the Isoplot program, with the plateau age errors reported at 2 σ. The analytical procedures followed those outlined by Zhang et al. (2006) and Chen et al. (2006).
Major and trace-element analyses were conducted at the Institute of Regional Geology and Mineral Resources, Hebei Province, China. The major elements were analyzed using X-ray fluorescence (XRF), with accuracy better than 5%, and the trace elements were analyzed by inductively coupled plasma–mass spectrometry (ICP–MS), with accuracy better than 10%.
4 Analytical results
4.1 Zircon U–Pb geochronology
Zircons from hornblende gneiss (sample FQ1) are predominantly rounded, with some displaying long columnar shapes (Figure 3A). The U and Th contents of hornblende gneiss (sample FQ1) range from 142.86 to 452.90 ppm and 302.48 to 650.35 ppm, respectively, with Th/U ratios of 0.39–0.72 (n = 17; Table 1), which is consistent with a magmatic origin. Analyses yield 206Pb/238U ages of 839–883 Ma with a weighted-mean age of 864.9 ± 5.4 Ma (MSWD = 1.02) (Figure 3B).
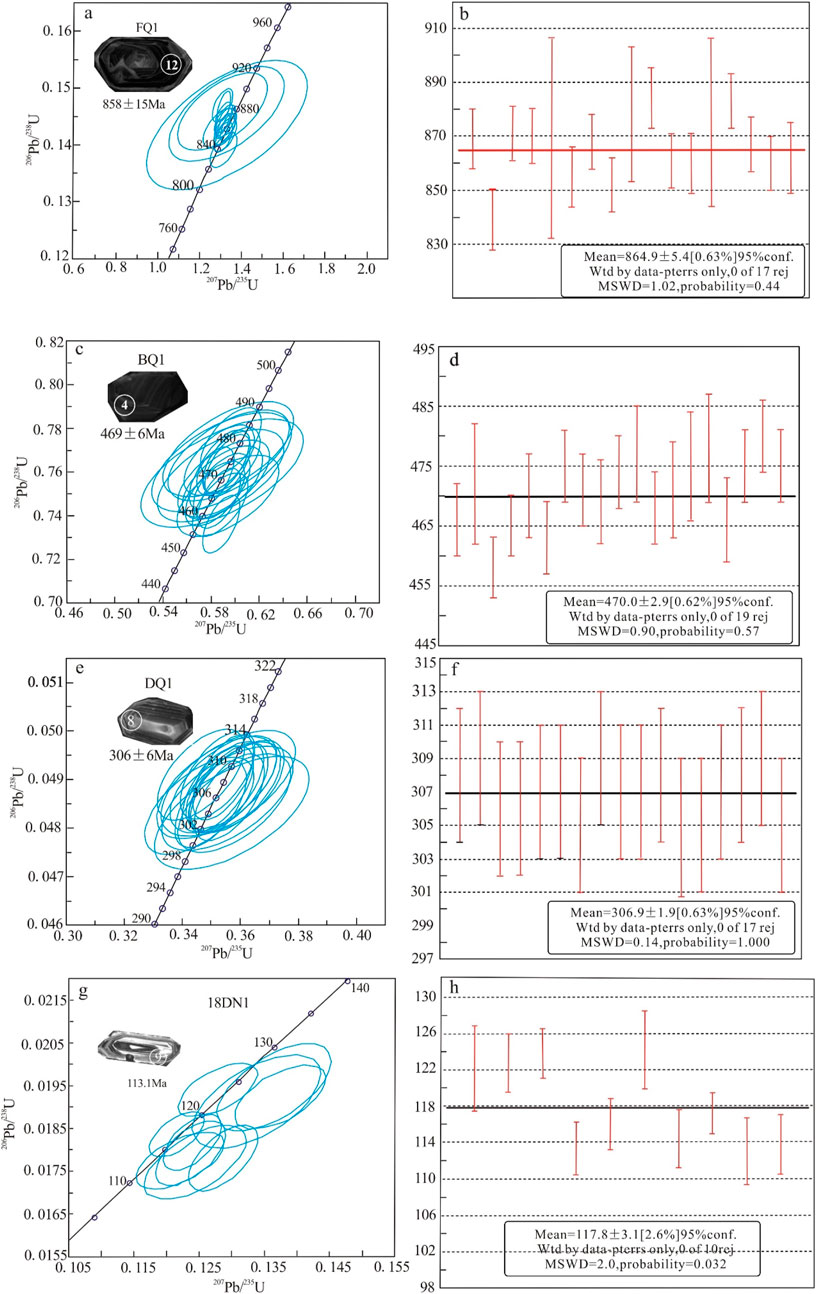
Figure 3. Zircon harmonic age map (A, C, E, and G) and weighted average map (B, D, F, and H) of intrusive rocks from the study area.
Zircons from gabbro (sample BQ1) are primarily rounded, with some exhibiting columnar shapes (Figure 3C). The U and Th contents of gabbro (sample BQ1) range from 1,020.27 to 10,980.61 ppm and 6,553.42 to 42,051.31 ppm, respectively, with Th/U ratios of 0.13–0.29 (n = 19; Table 1), which is consistent with a magmatic origin. Analyses yield 206Pb/238U ages of 458–480 Ma with a weighted-mean age of 470.0 ± 2.9 Ma (MSWD = 0.90) (Figure 3D).
Zircons from monzonitic granite (sample DQ1) exhibit well-developed crystals, bright cathodoluminescence, complete growth rings, and pronounced rhythmic zoning (Figure 3E). The U and Th contents range from 42.21 to 572.40 ppm and 92.40 to 521.29 ppm, respectively, with Th/U ratios of 0.86–2.19 (n = 17; Table 1), suggesting a magmatic origin. Analyses yield 206Pb/238U ages of 304–309 Ma with a weighted-mean age of 306.9 ± 1.9 Ma (MSWD = 0.14) (Figure 3F).
Zircons of diorite (sample 18DN1) are well-crystallized with flattened shapes (Figure 3G). The U and Th contents range from 205.55 to 885.81 ppm and 257.12 to 788.38 ppm, respectively, with Th/U ratios of 0.74–1.28 (n = 10; Table 1), indicative of a magmatic origin. Analyses yield 206Pb/238U ages of 113–124 Ma with a weighted-mean age of 117.8 ± 3.1 Ma (MSWD = 2.0) (Figure 3H).
4.2 Muscovite 40Ar/39Ar dating
The Ar–Ar dating results of muscovite are presented in Table 2. The corresponding plateau age, isochron age (Figure 4A), and inverse isochron age are illustrated in Figure 4B. The muscovite sample yielded an 40Ar/39Ar plateau age of 296.9 ± 1.7 Ma (nine-step age-heating spectrum from 850°C to 1,300°C, and 87.9% of 39Ar released), a corresponding isochron age of 300.3 ± 1.7 Ma (950°C–1,200°C, N = 8, MSWD = 3.2), and an inverse isochron age of 299.9 ± 1.7 Ma (950°C–1,200°C, N = 8, MSWD = 3.7). These data indicate that the plateau age (296.9 ± 1.7 Ma) represents the reliable crystallization age of the muscovite.
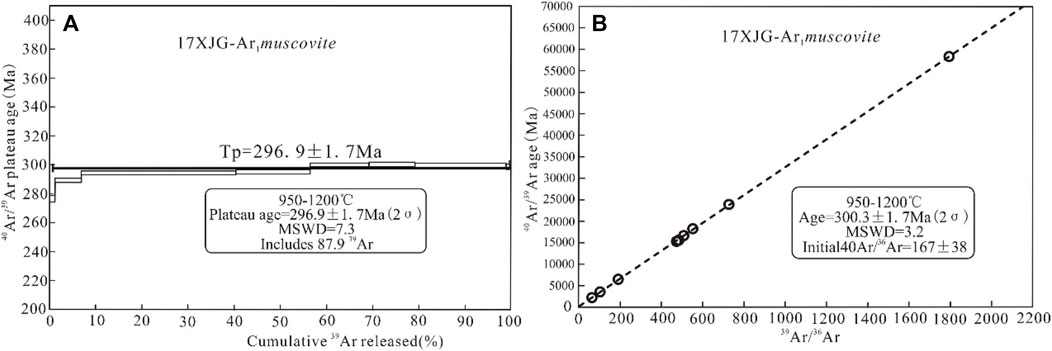
Figure 4. 40Ar/39Ar plateau age (Ma) (A) and isochron of 40Ar/39Ar age (Ma) (B) of muscovite from the study area.
4.3 Geochemistry
The Neoproterozoic hornblende gneisses belong to the calc-alkaline series and exhibit high total alkali contents (Table 3). The samples mainly plot within the monzonite field on the TAS diagram (Figure 5A). In the K2O–SiO2 diagram, they fall within the high-K calc-alkaline series and are peraluminous (Figure 5C). The Neoproterozoic hornblende gneisses yielded Eu/Eu* values of 0.62–0.82 and (La/Yb)N values ranging from 5.30 to 30.62. Rare earth element (REE) fractionation is minimal. The chondrite-normalized REE diagram (Figure 6A) indicates a slight enrichment of light rare earth elements (LREEs) with a subtle positive trend. The primitive-mantle-normalized trace-element spider diagram (Figure 6B) shows enrichment in large-ion lithophile elements (LILEs; e.g., Rb, Ba, Th, U, La, Nd, and Ce) and depletion in high-field-strength elements (HFSEs; e.g., Ta, P, and Nb; Table 3).
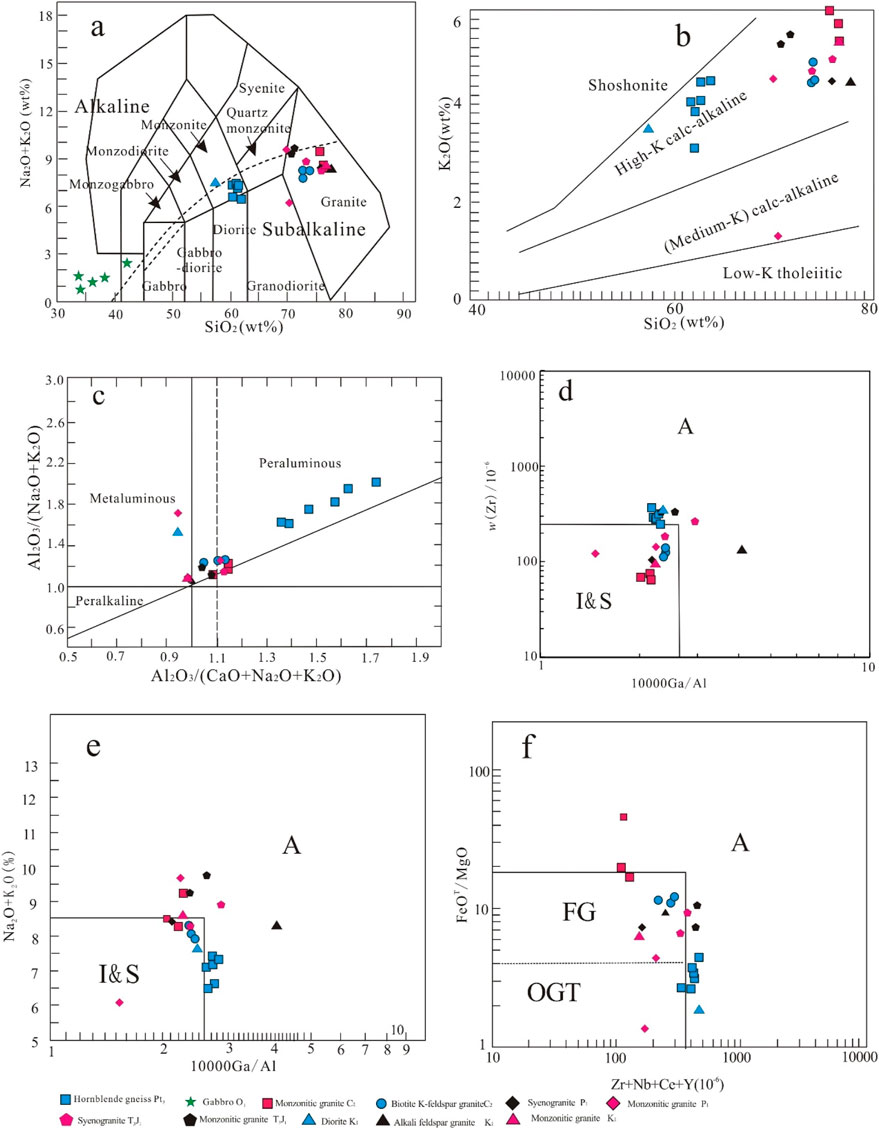
Figure 5. (A) TAS diagram, (B) K2O versus SiO2 diagram, (C) A/CNK versus A/NK diagram, and (D–F) discrimination diagrams for A-type granite for intrusive rocks of the study area are from Irvine and Baragar (1971), Peccerillo and Taylor (1976), Maniar and Piccoli (1989), Whalen et al. (1987), and Frost et al. (2001), respectively.
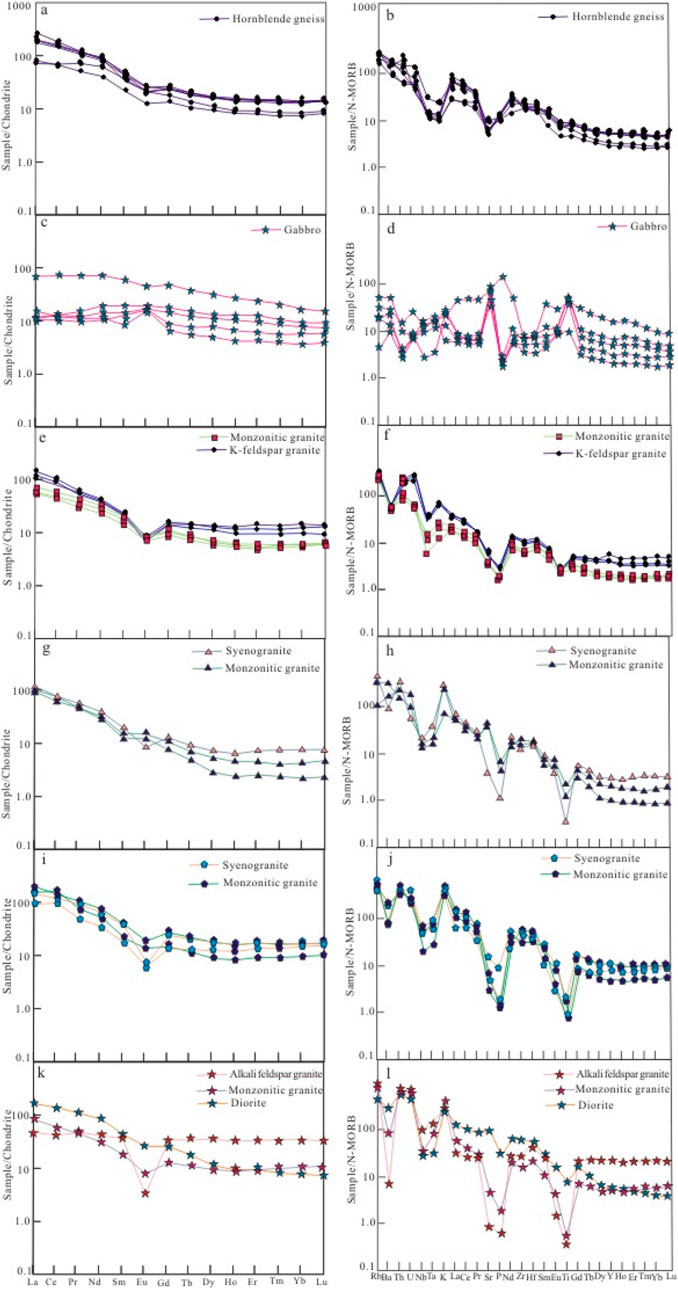
Figure 6. REE patterns (A,C,E,G,I, and K) and primary mantle trace-element patterns (B,D,F,H,J, and L) for intrusive rocks in the study area. Chondrite and N-MORB normalizing values are from Boynton (1984) and Sun and McDonough (1989), respectively.
The Early Ordovician gabbro rocks are characterized by low Si and high Mg contents (Table 3). These samples primarily plot near the gabbro field on the TAS diagram (Figure 5A). They exhibit Eu/Eu* values ranging from 0.85 to 2.16 and (La/Yb)N values of 1.10–3.94, with significant REE fractionation. The chondrite-normalized REE diagram (Figure 6C) shows enrichment in LREEs, exhibiting a positive trend. The primitive-mantle-normalized trace-element spider diagram (Figure 6D) indicates enrichment in LILEs (e.g., Rb, Sr, and Ba) and depletion in HFSEs (e.g., Zr, Hf, and P; Table 3).
The Late Carboniferous, Early Permian, Late Triassic–Early Jurassic, and Early Cretaceous intrusive rocks belong to the calc-alkaline series, with high total alkali contents (Table 3). These samples fall in the field of high-K calc-alkaline series on the K2O–SiO2 diagram (Figure 5B) and are peraluminous (Figure 5C). The Eu/Eu* values range from 0.36 to 1.30, and (La/Yb)N values are between 1.3 and 41.54, with significant REE fractionation. The chondrite-normalized REE diagram (Figure 6E) shows enrichment in LREEs, exhibiting a positive trend. The primitive-mantle-normalized trace-element spider diagram (Figure 6F) reveals enrichment in LILEs (e.g., Rb, Th, U, K, and Nd) and depletion in HFSEs (e.g., Ta, P, Eu, Ti, and Nb; Table 3).
5 Discussion
5.1 Neoproterozoic–Mesozoic magmatism in the Xinlin area
Previous geochronological studies reported a wide range of ages for the intrusive rocks of the Erguna block (Table 4). At least ∼929 Ma, ∼887 Ma, ∼850 Ma, ∼819 Ma, ∼792 Ma, ∼764 Ma, and ∼738 Ma magmatic events occurred in the Neoproterozoic Era on the Erguna block (Guo et al., 2016; Zhao et al., 2016; Yang et al., 2017). The Paleozoic magmatic activity in the Erguna block can be divided into the Early and Late Paleozoic activities. The Early Paleozoic magmatic activity can be mainly divided into four stages: ∼500 Ma (Middle-Late Cambrian: 504–500 Ma), ∼480 Ma (Early Ordovician: 485–475 Ma), ∼460 Ma (Middle-Late Ordovician: 465–454 Ma), and ∼440 Ma (Early Silurian: 439–434 Ma) (Zhao, 2017; Wu et al., 2005); the Late Paleozoic magmatic activity occurred from 330 to 241 Ma (Ju et al., 2005; Wang et al., 2012; Feng et al., 2014). The early Late Paleozoic granitic magmatism occurred between 405 and 325 Ma, which can be further refined into three stages: Early Middle Devonian (Stage I, 405 to 380 Ma), Late Devonian–Early Carboniferous (Stage II, 365 to 350 Ma), and Late Early Carboniferous (Stage III, 335 to 325 Ma) (Qian et al., 2018). The Mesozoic magmatism in the Erguna block can be divided into seven stages: ∼246 Ma, ∼225 Ma, ∼205 Ma, ∼185 Ma, ∼155 Ma, ∼137 Ma, and ∼125 Ma (Wang et al., 2012; Tang, 2016).
To obtain a more comprehensive understanding of granitic magmatism in the northern Great Xing’an Range, we selected 66 zircon U–Pb results and combined them with the five new ages of this study. Based on this combined geochronological dataset, three stages of granitic magmatism can be identified, namely, Neoproterozoic, Paleozoic, and Mesozoic granites (Table 4). Neoproterozoic granitoids, which are rarely developed in the Erguna block, comprise hornblende gneisses and mica quartz schists, with isotopic ages ranging from 915 to 791 Ma (Table 4). Paleozoic intrusive rocks are widely distributed in the Erguna block, with ages ranging from 504 to 241 Ma. Mesozoic granitoids are widely exposed in the northern Great Xing’an Range, primarily near the Xinlin–Xiguitu suture, with ages spanning 240 to 116 Ma.
5.2 Petrogenesis
The origin and source of the magmas that formed the intrusive rocks in the Erguna block are varied and have been the focus of numerous studies (Gao et al., 2013; Hu et al., 2014; Liu et al., 2016; Wang et al., 2016; Zhao, 2017; Yang et al., 2017; Lu et al., 2020). However, debates persist regarding the petrogenesis of the Neoproterozoic–Mesozoic intrusive rocks in the Erguna block.
5.2.1 Petrogenesis of Neoproterozoic intrusive rocks
The information regarding the genesis of Neoproterozoic intrusive rocks in the Erguna block is relatively consistent, which is related to the convergence and breakup of the Rodinia supercontinent (Zhao, 2017; Lu, 2019). The approximately 887 Ma granites are similar to post-collisional granites (Liu et al., 2011; Zhao et al., 2016), whereas the 850–737 Ma granitoids are generally similar to A-type granites, and the magmas could have originated not only from the partial melting of a depleted lower crust that accreted during the Meso-Neoproterozoic, with a contribution of ancient crustal materials in their petrogenesis, but also from the partial melting of the residual ancient mafic crustal material (Tang et al., 2013; Zhao et al., 2016).
The Neoproterozoic Xinghuadukou rock group hornblende gneisses in the study area exhibit high Mg# values (average 50.25), which rules out the possibility that they were derived from partial melting of the lower crust. The hornblende gneisses show Nb contents ranging from 8.20 to 21.70 ppm, higher than those of N-MORB (typically 2–3 ppm; Sun and McDonough, 1989). The TiO2 contents of hornblende gneisses range from 0.57% to 0.62%, lower than those of OIB (≈2.68%; Ewart et al., 1998). The hornblende gneisses are also characterized by relatively high V, Sr, Ni, Co, and Cr contents and low Si content, suggesting a mantle-derived origin.
5.2.2 Petrogenesis of Early Paleozoic intrusive rocks
The Early Paleozoic intermediate–acidic rocks in the Erguna block mainly originated from the partial melting of the newly accreted lower crust, with the participation of some residual ancient crustal materials; the primitive magma of the basic rocks mainly originated from the partial melting of the depleted lithospheric mantle that was metasomatized by subducting fluids (Zhao, 2017). The collision granite magma on the northern edge of the Erguna block originated from the mantle during the Early Paleozoic and was involved in the crustal material (Wu et al., 2005; Lu et al., 2022).
The Early Paleozoic intrusive rocks in the Xinlin area are predominantly the Early Ordovician gabbros, exhibiting high Mg# values (average 59.75), which rules out the partial melting of the lower crust as their source. The gabbros have Nb contents of 1.40 to 6.90 ppm (average 4.64 ppm), which is higher than those of N-MORB (generally 2–3 ppm; Sun and McDonough, 1989). The TiO2 contents of the gabbros are between 1.33% and 6.77% (average 4.64%), which is higher than those of OIB (≈2.68%; Ewart et al., 1998). Additionally, these gabbros yielded an average Rb/Sr value of 0.015, which is close to the primitive mantle value (0.03; Sun and McDonough, 1989). They also exhibit low Si and high Sr (334–835 ppm), Ni (26–101 ppm), Co (39.2–77.2 ppm), and Cr (20–130 ppm) contents, further supporting their origin from the mantle.
5.2.3 Petrogenesis of the intermediate–acidic intrusive rocks
In the Triassic to Early Jurassic period, the primitive magma of basic intrusive rocks originated from the partial melting of the depleted lithospheric mantle, and intermediate–acidic intrusive rocks originated from the partial melting of the newly accreted lower crust and were mixed with a small amount of ancient continental crust material (Tang et al., 2014; Tang, 2016). The Early Jurassic granitic magmas formed from the partial melting of the felsic crust (Wang et al., 2016; Tang, 2016) or crust–mantle mixing (Dai et al., 2013); the Late Jurassic granitic magmas formed as a result of the dismantling/melting of the thickened lower crust in a collisional orogenic belt (Wu et al., 2008) or from crust–mantle interaction (Dai et al., 2013; Hu et al., 2014; Tang, 2016); and the Early Cretaceous granitic magmas were derived from the partial melting of crustal material (Wu et al., 2008; Gao et al., 2013; Shi et al., 2013; Niu et al., 2016), where the lithospheric mantle was metasomatized by subduction-related fluids, forming the parent magmas to the diorites. This process may have involved the partial melting of the subducted plate and metasomatism of the lithospheric mantle by sediment-derived fluids (Liu et al., 2016; Chai et al., 2018).
The Late Carboniferous, Early Permian, Late Triassic–Early Jurassic, and Early Cretaceous intrusive rocks in the study area are predominantly intermediate–acidic, peraluminous, and a part of the high-K calc-alkaline series; the major elements show high SiO2 content and low Fe2O3T and Mg# values (average 24.12), and the trace elements are enriched in LREEs, such as Rb, Th, U, K, Nd, Zr, and Hf, and relatively depleted in HREEs, such as Ba, Nb, Sr, P, Eu, and Ti. The negative Eu anomalies of the intermediate–acidic intrusive rocks indicate that the plagioclases likely originate from the residual phases (Lightfoot et al., 1987). The intermediate–acidic intrusive rocks yield an average Rb/Sr value of 2.62, which is close to the crustal ratio (0.15) but higher than that of the primitive mantle (0.03), E-MORB (0.033), and OIB (0.047) (Sun and McDonough, 1989), and these geochemical features suggest that they were derived from the partial melting of the lower crust.
5.3 Tectonic setting
5.3.1 Neoproterozoic tectonic setting
The Neoproterozoic magmatism of the Erguna block provides key insights into the convergence and breakup of the Rodinia supercontinent, a significant global geological event, and several magmatic events related to the Rodinia supercontinent breakup have been reported in the Erguna block (Wu et al., 2011; Sun et al., 2012; Tang et al., 2013; Feng, 2015). Magmatisms between 927 Ma and 880 Ma were the result of collision-orogeny during the stage of assembly of the Rodinia supercontinent, whereas the 850–737 Ma magmatisms record the breakup of the Rodinia supercontinent (Zhao, 2017). The study area is located near the Xinlin–Xiguitu suture, and the Neoproterozoic hornblende gneisses of the study area belong to A-type granite, suggesting emplacement in an extensional setting (Figure 5). These gneisses also exhibit characteristics of volcanic-arc granites (Figure 7), indicating their association with the breakup of the Rodinia supercontinent.
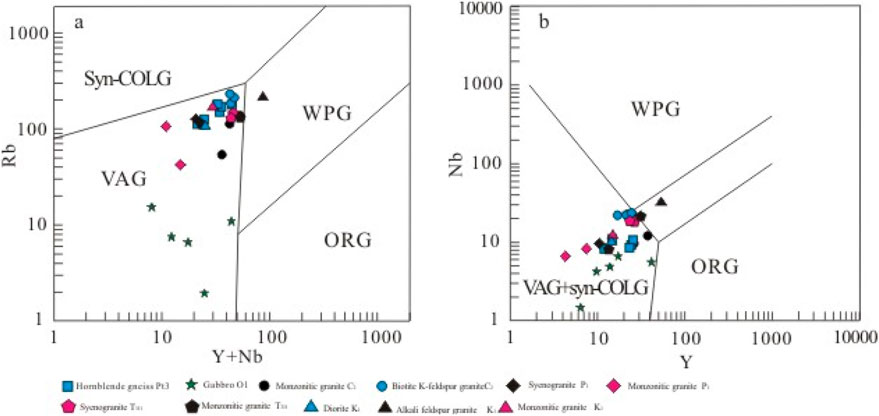
Figure 7. (A) (Y + Nb) vs. Rb diagram and (B) Nb vs. Y diagram for intrusive rocks in the study area are from Pearce et al. (1984).
5.3.2 Paleozoic tectonic setting
For the Paleozoic era, various tectonic settings have been proposed for the Erguna block. Early Paleozoic igneous rocks in the Erguna block were formed in a post-collision extensional environment, likely linked to the collision and amalgamation of the Erguna and the Xing’an blocks (Feng, 2015; Zhao, 2017). Late Paleozoic granitic magmatism is associated with the collision and assembly of the Erguna–Xing’an and Songnen blocks, transitioning from an orogenic to an extensional environment (Wu et al., 2005; Qian et al., 2018). The collision and assembly between the Erguna block and the Xing’an block were completed in the Early Paleozoic era (Ge et al., 2005), forming collisional accretionary terranes that were accreted during the Late Pan-African global event (Zhou et al., 2011).
The Early Ordovician gabbros show characteristics similar to those of volcanic-arc granites (Figure 7), supporting their connection to the collision and merging of the Erguna and Xing’an blocks. Late Carboniferous–Early Permian intrusive rocks include both A-type and I-type granites, suggesting their emplacement during a transition from orogenic to extensional tectonic settings (Figure 5). These rocks display the characteristics of volcanic-arc and collisional granites (Figure 7), suggesting their emplacement in an orogenic setting. Tectonic activity in the study area during this period was primarily controlled by the collision and amalgamation of the Erguna and Xing’an blocks, followed by the Songnen and Xing’an blocks, which resulted in the generation of a large amount of intermediate–acidic magmas.
5.3.3 Mesozoic tectonic setting
The Late Permian–Early Jurassic intrusive rocks on the Erguna block were formed in the subduction environment of the MOO plate; the Late Permian–Early Middle Triassic intrusive rocks were formed in the active continental margin environment; the Late Middle Triassic–Early Late Triassic intrusive rocks were formed in a local extensional setting; the Late Triassic–Early Jurassic intrusive rocks were formed in the active continental margin environment; and the Early Jurassic igneous rocks were formed in the active continental margin environment; the Late Jurassic magmatic activity took place in a lithospheric extensional environment caused by the collapse and subsidence of the thickened continental crust after the closure of the Mongolian Okhotsk Ocean (Tang, 2016). The magmatic activity in the Middle Triassic may be related to the extensional environment after the closure of the ancient Asian Ocean; the Early Middle Jurassic magmatic events may be related to the subduction of the MOO (Wang et al., 2012). The Late Triassic intrusive rocks are related to the subduction of the MOO (Liu et al., 2021). The MOO underwent subduction, collision, and post-collision processes during the Early Jurassic to Early Cretaceous, with its closure in the northern part of the Great Xing’an Range likely occurring between the Late Jurassic and Early Cretaceous (Liu et al., 2021). The Early Cretaceous granites were formed in an extensional tectonic setting (Wu et al. ,2009; Shi et al., 2013; Liu et al., 2016) and were related to the final closure of the MOO (Shi et al., 2013; Liu et al., 2016) or the rollback of the Paleo-Pacific Plate (Gao et al., 2013). The geochronological data and tectonic interpretations suggest that the Early Cretaceous intrusive rocks within the study area were formed during non-orogenic extension and were related to the final closure of the MOO or the rollback of the Paleo-Pacific Plate. These intrusive rocks within the study area are distributed in an NE–SW orientation, parallel to the continental margin, and their age decreases from west to east. The intrusive rocks within the Xinlin area yielded a mean age of 125 Ma, which is consistent with the timing of the final closure of the MOO or of the rollback of the Paleo-Pacific Plate. In the discrimination diagram for A-type granites in Figures 5D–F, all the Late Triassic–Early Cretaceous intrusive samples plot within the A-type granite field, suggesting that they formed in an extensional setting.
6 Conclusion
Our study of the tectonic–magmatic activity in the Xinlin area of the Erguna block from the Neoproterozoic to the Mesozoic period leads to the following conclusions:
(1) LA-ICP-MS zircon U–Pb and Muscovite 40Ar/ 39Ar dating suggest that magmatism in the study area occurred during the Neoproterozoic (ca. 864.98 Ma), Early Ordovician (ca. 470.0 Ma), Late Carboniferous (ca. 306.9 Ma), Early Permian (ca. 296.9 Ma), and Early Cretaceous (ca. 117.8 Ma) periods.
(2) The Neoproterozoic and Early Ordovician intermediate–mafic intrusive rocks exhibit low Rb/Sr contents, high Mg#, and weakly negative Eu anomalies. In contrast, the Late Carboniferous, Early Permian, Late Triassic–Early Jurassic, and Early Cretaceous intermediate–acidic intrusive rocks display high Rb/Sr contents, low Mg#, and strongly negative Eu anomalies. These characteristics suggest distinct magma sources: intermediate–acidic magmas were derived from the lower crust, while intermediate–mafic magmas were derived from the mantle and subsequently contaminated by crustal material.
(3) Neoproterozoic intrusive rocks in the Xinlin area formed during the breakup of the Rodinia supercontinent. Paleozoic intrusive rocks were formed during the collision and amalgamation of the Erguna, Xing’an, and Songnen blocks. Early Mesozoic intrusive rocks were associated with the subduction of the Mongol-Okhotsk oceanic intracontinental system, while Late Mesozoic intrusive rocks developed in a non-orogenic extensional tectonic setting, linked to either the final closure of the MOO or the rollback of the Paleo-Pacific Plate.
Data availability statement
The original contributions presented in the study are included in the article/supplementary material; further inquiries can be directed to the corresponding author.
Author contributions
SL: data curation, formal analysis, software, and writing–original draft. CL: data curation and writing–review and editing. MA: methodology and writing–review and editing. ZS: software and writing–review and editing. XZ: formal analysis and writing–review and editing. AF: methodology and writing–review and editing. WY: methodology and writing–review and editing.
Funding
The author(s) declare that financial support was received for the research, authorship, and/or publication of this article. This work was financially supported by the Key Research and Development Project of Heilongjiang Province (GA21A204 and HKY202401).
Acknowledgments
The authors are grateful to professor Yang Liu for his kind help during the field work and professor Keyong Wang for his guidance in preparing this manuscript.
Conflict of interest
The authors declare that the research was conducted in the absence of any commercial or financial relationships that could be construed as a potential conflict of interest.
Generative AI statement
The author(s) declare that no generative AI was used in the creation of this manuscript.
Publisher’s note
All claims expressed in this article are solely those of the authors and do not necessarily represent those of their affiliated organizations, or those of the publisher, the editors and the reviewers. Any product that may be evaluated in this article, or claim that may be made by its manufacturer, is not guaranteed or endorsed by the publisher.
Supplementary material
The Supplementary Material for this article can be found online at: https://www.frontiersin.org/articles/10.3389/feart.2025.1514658/full#supplementary-material
References
Andersen, T. (2002). Correction of common lead in U–Pb analyses that do not report 204Pb. Chem. Geol. 192, 59–79. doi:10.1016/s0009-2541(02)00195-x
Boynton, W. V. (1984). “Geochemistry of the rare earth elements: meteor-ite studies,” in Rare earth element geochemistry. Editor P. Henderson (New York: Elservier), 63–114.
Chai, M. C., Wang, Q., Zhao, G. Y., Gao, S., and Cao, K. (2018). Zircon U-Pb ages and geochemical characteristics of Late Mesozoic volcanic rocks from Shibazhan-Hanjiayanzi area of Da Hinggan Mountains and their tectonic significance. Geol. Bull. China 37 (10), 1866–1881. (in Chinese with English abstract). doi:10.16509/j.georeview.2018.03.004
Chen, W., Zhang, W., Zhang, Y. Q., Jin, G. S., and &Wang, Q. L. (2006). Late Cenozoic episodic uplifting in southeastern part of the Tibetan plateau-evidence from Ar-Ar thermochronology. Acta Petrol. Sin. 22 (4), 867–872. (in Chinese with English abstract).
Dai, H. M., Yang, Z. F., Ma, Z. D., and Gong, C. D. (2013). The petrogeochemical characteristics and tectonic setting of Mesozoic intrusive rocks in Chabaqi area of the Da Hinggan Mountains. Geol. China 40 (1), 232–247. (in Chinese with English abstract).
Ewart, A., Milner, S. C., Armstrong, R. A., and Dungan, A. R. (1998). Etendeka volcanism of the goboboseb mountains and messum igneous complex, Namibia. Part I: geochemical evidence of early cretaceous tristan plume melts and the role of crustal contamination in the parana-etendeka CFB. J. Petrology 39 (2), 191–225. doi:10.1093/petroj/39.2.191
Feng, Z. Q. (2015). The Palezoic tectono-magmatic evolution of the northern Great Xing’an range. M.Phil. Diss. Jilin Univ. (in Chinese with English abstract).
Feng, Z. Q., Liu, Y. J., Wen, Q. B., Han, G. Q., Li, W. M., and Zhang, L. (2014). Petrogenesis of ∼330 Ma meta-gabbro-granite from the Tayuan area in the northern segment of the Da Xing'an Mts and its tectonic implication. Acta Petrol. Sin. 30 (7), 1982–1994. (in Chinese with English abstract).
Frost, B. R., Barnes, C. G., Collins, W. J., Arculus, R. J., Ellis, D. J., and Frost, C. D. (2001). A geochemical classification for granitic rocks. J. Petrol 42, 2033–2048. doi:10.1093/petrology/42.11.2033
Gao, Y., Zheng, C. Q., Yao, W. G., Wang, H., Li, J., Shi, L., et al. (2013). Geochemistry and Ziron U-Pb geochronlogy of the Luotuobozi pluton in the Haduohe area in the northern DaXing’anling. Acta Geol. Sin. 87 (9), 1293–1310. (in Chinese with English abstract).
Ge, W. C., Chen, J. S., Yang, H., Zhao, G. C., Zhang, Y. L., and Tian, D. X. (2015). Tectonic implications of new zircon U–Pb ages for the Xinghuadukou complex, Erguna massif, northern Great Xing’an range, NE China. J. Asian Earth Sci. 106, 169–185. doi:10.1016/j.jseaes.2015.03.011
Ge, W. C., Wu, F. Y., Zhou, C. Y., and Abdel Rahman, &A. A. (2005). The age of Tahe granite in the northern Daxing'an mountains and its constraints on the structural attribution of the Erguna block. Sci. Bull. 50 (12), 1239–1247. (in Chinese with English abstract).
Guo, Y. F., Yang, Y. C., Han, S. J., Tan, Y., and &Bo, J. W. (2016). Geochemistry and zircon U Pb dating of the tonalite from fenghuangshan area in daxing'anling. J. Univ. ( Earth Sci. Edtion) 46 (5), 1406–1417. (in Chinese with English abstract). doi:10.13278/j.cnki.jjuese.201605111
HBGMR (Heilongjiang Bureau of Geology and Mineral Resources) (1997). Rock stratigraphic units. Wuhan: China university of Geoscience Press. (in Chinese with English abstract).
Hu, X. L., Yao, S. Z., He, M. C., Ding, Z. J., Liu, M., Cui, Y. B., et al. (2014). Sulfur and lead isotopic characteristics of Chalukou and Daheishan porphyry Mo deposits in northern segment of Da Hinggan Mountains. Mineral. Deposits 33 (4), 776–784. (in Chinese with English abstract). doi:10.16111/j.0258-7106.2014.04.027
Irvine, T. H., and Baragar, W. R. A. (1971). A guide to the chemical classifica-tion of the common volcanic rocks. Can. J. Earth Sci. 8 (5), 523–548. doi:10.1139/e71-055
Jahn, B. M. (2004). The central asian orogenic belt and growth of the continental crust in the phanerozoic. Geological Society, London, Special Publications, 226 (1), 73–100.
Jia, P. P., Wei, J. H., Gong, Q. W., and Zhao, W. L. (2011). Analysis of geolog-ical background and ore-searching prospect for copper-molybdenum deposits in the Da Hingan Ling area. Geol. Explor. 47 (2), 0151–0162. (in Chinese with English abstract).
Ju, W. X., Su, S. J., Bai, C. X., and Shao, J. (2005). Charateristics of the late carboniferous banlashan series of intrusive rock in orqohan area, dahingganling. Geol. Resour. 14 (3), 161–165. (in Chinese with English abstract).
Lightfoot, P. C., Hawkesworth, C. J., and Sethna, S. F. (1987). Petrogenesis of rhyolites and trachytes from the Deccan Trap: Sr, Nd and Pb isotope and trace element evidence. Contributions Mineralogy Petrology 95 (1), 44–54. doi:10.1007/bf00518029
Liu, B. B., Wang, J. G., Bai, C. D., Zhang, X. Q., Li, D., Zhang, Z. X., et al. (2021). Zircon U-Pb geochronology, geochemistry and geological significance of late triassic and early cretaceous granites in aduta area, northern daxing’an mountains. Hebeidizhidaxuexuebao 44 (2), 8–18. (in Chinese with Englishabstract). doi:10.13937/j.cnki.hbdzdxxb.2021.02.002
Liu, J., Liu, Z. H., Li, S. C., Zhao, C., Wang, C. J., Peng, Y. B., et al. (2016). Geochronology and geochemistry of Triassic intrusive rocks in Kaiyuan area of the eastern section of the northern margin of North China. Acta Petrol. Sin. 32 (9), 2739–2756. (in Chinese with English abstract).
Liu, J. L., Bai, X. D., Zhao, S. J., Tran, M. D., Zhang, Z. C., Zhao, Z. D., et al. (2011). Geology of the Sandaowanzi telluride gold deposit of the northern GreatXing'an Range, NE China: geochronology and tectonic controls. J. Asian Earth Sci. 41, 107–118. doi:10.1016/j.jseaes.2010.12.011
Liu, Y. F., Jiang, S. H., and &Zhang, Y. (2010). The SHRIMP zircon U-Pb dating and geological features of Bairendaba diorite in the Xilin-haote area, Inner Mongolia, China. Geol. Bull. China 29 (5), 688–696. (in Chinese with English abstract).
Liu, Y. J., Li, W. M., Feng, Z. Q., Wen, Q. B., Franz, N., and &Liang, C. Y. (2017). A review of the paleozoic tectonics in the eastern part of central asian orogenic belt. Gondwana Res. 43, 123–148. doi:10.1016/j.gr.2016.03.013
Lu, S. (2019). Metallogenic regularities and metallogenic prognosis of gold deposits in Xinlin- han jia Yuan area, Great Xing’an range, China M.Phil. Jilin University. (in Chinese with English abstract).
Lu, S., Deng, C. Z., Wang, K. Y., Feng, Y. Z., Li, C. L., Chen, J. Y., et al. (2022). Crustal contribution for the formation of the Walali Au deposit and implications on the early cretaceous Au mineralization in the northern Great xing’an range. Ore Geol. Rev. 147 (2022), 105000. doi:10.1016/j.oregeorev.2022.105000
Lu, S., Wang, K. Y., Liu, Y., Deng, C. Z., and Jing, B. Y. (2020). Geochronological and geochemical data for Late Palaeozoic–Mesozoic intrusive rocks in the Wenkutu area, northeastern China: new constraints on the tectonic evolution of the Great Xing'an Range. Geol. J. 55, 1357–1379. doi:10.1002/gj.3494
Ludwig, K. R. (2003). Isoplot 3.00: a geochronological toolkit for microsoft excel. California, Berkeley: Berkeley Geochronology Center, 39.
Maniar, P. D., and Piccoli, P. M. (1989). Tectonic discrimination of granitoids. Geol. Soc. Am. Bull. 101, 635–643. doi:10.1130/0016-7606(1989)101<0635:tdog>2.3.co;2
Miao, L. C., Fan, W. M., Zhang, F. Q., Liu, D. Y., Jian, P., Shi, G. H., et al. (2004). Zircon SHRIMP geochronology of the Xinkailing-Kele complex in the northwestern Lesser Xing’an Range, and its geological implications. Chin. Sci. Bull. 49, 201–209. doi:10.1360/03wd0316
Niu, Y. H., Liu, Y., Zhou, Z. G., Niu, W. Z., Liu, C. F., Zhao, X. Q., et al. (2016). Chronology,geochemistry and geological significance of the Early Cretaceous intrusive rocks from the Tahe region,northeastern China. Sediment. Geol. Tethyan Geol. 36 (4), 95–105. (in Chinese withEnglish abstract).
Ouyang, H. G., Mao, J. W., Zhou, Z. H., and Su, H. M. (2015). Late mesozoic metallogeny and intracontinental magmatism, southern Great Xing'an range, northeastern China. Gondwana Res. 27, 1153–1172. doi:10.1016/j.gr.2014.08.010
Peasrce, J. A., Harris, N. B. W., and &Tindle, A. G. (1984). Trace element discrimination diagrams for the tectonic interpretation of granitic rocks. J. Petrology 25 (4), 956–983. doi:10.1093/petrology/25.4.956
Peccerillo, A., and Taylor, A. R. (1976). Geochemistry of Eocene calc-alkaline volcanic rocks from the Kastamonu area, Northern Turkey. Contribu-tions Mineralogy Petrology 58, 63–81. doi:10.1007/bf00384745
Qian, C., Lu, L., Qin, T., Li, L. C., Chen, H. J., Cui, T. R., et al. (2018). The early late-Paleozoic granitic magmatism in the Zalantun region, northern Great Xing’an range, NE China: constraintson the timing of amalgamation of erguna-Xing’an and Songen blocks. Acta Geol. Sin. 92 (11), 2190–2214. (in Chinese with Englishabstract).
Shao, J. A., and Mu, B. L. (1999). Magmatism in the mesozoic extending orogenic process of da Hinggan MTS. Earth Sci. Front. 6 (4), 339–346. (in Chinese withEnglish abstract).
Shi, L., Zheng, C. Q., Yao, W. G., Li, J., Xu, J. L., Gao, Y., et al. (2013). Geochronology, petro-geochemistry and tectonic setting of the hamagou forest farm A-type granites in the wuchagou region, central Great xinggan range. Acta Geol. Sin. 87 (9), 1264–1276. (in Chinese with English abstract).
Steiger, R. H., and Jager, E. (1977). Subcommission on geochronology:convention on the use of decay constants in geo- and cosmochronology. Earth Planet. Sci. Lett. 36, 359–362. doi:10.1016/0012-821x(77)90060-7
Sui, Z. M., Ge, W. C., Wu, F. Y., Zhang, J. H., Xu, X. C., and Cheng, R. Y. (2007). U-Pb ages,geochemistry and its petrogenesis of Jurassic granites in northeastern part of the Da Hinggan Mts. Acta Petrol. Sin. 23 (2), 461–480. (in Chinese withEnglish abstract).
Sun, L. X., Ren, B. F., Zhao, F. Q., and Peng, L. N. (2012). Zircon U-Pb ages and Hf isotope characterstics of Taipingchuan large porphyritic granite pluton of Erguna Massif in the Great Xing’an Range. Earth Sci. Front. 19 (5), 114–122. (in Chinese with English abstract).
Sun, S. S., and McDonough, W. F. (1989). “Chemical and isotopic systematics of oceanic basalts: implications for mantle composition and processes,”. Magmatism in ocean basins. Editors A. D. Saunders, and M. J. Norry (London: Geological Society Special Publication), 42, 313–345. doi:10.1144/gsl.sp.1989.042.01.19
Tang, J. (2016). Geochronology and geochemistry of the Mesozoic igneous rocks in the Erguna Massif, NE China: constraints on the tectonic evolution of the Mongol-Okhotsk suture zone. M.Phil. Diss. Jilin Univ. (in Chinese with English abstract).
Tang, J., Xu, W. L., Wang, F., Wang, W., Xu, M. J., and Zhang, Y. H. (2014). Geochronology and geochemistry of early–middle triassic magmatism in the Erguna massif, NE China: constraints on the tectonic evolution of the mongol–okhotsk ocean. Lithos 184-187, 1–16. doi:10.1016/j.lithos.2013.10.024
Tang, J., Xu, W. L., Wang, F., Xu, M. J., and Zhang, Y. H. (2013). Geochronology and geochemistry of neoproterozoic magmatismin the Erguna massif,NE China: petrogenesis and implications for the breakup of the Rodinia supercontinent. Precambrian Res. 224, 597–611. doi:10.1016/j.precamres.2012.10.019
Wang, F. L., Wang, H. P., Lu, H. F., Yu, H. F., Chen, Y., Yang, H., et al. (2016). Geochronology, petrogeochemical characteristics and tectonic setting of Mesozoic granite in Shangqi area of the Da Hinggan Mountains. Geol. Sci. Technol. Inf. 35 (4), 18–28. (in Chinese with English abstract).
Wang, W., Xu, W. L., Wang, F., and &Meng, E. (2012). Zircon U-Pb chronology and assemblages of mesozoic granitoids in the manzhouli-erguna area, NE China: constraints on the regional tectonic evolution. Geol. J. China Univ. 18 (1), 88–105. (in Chinese with Englishabstract). doi:10.16108/j.issn1006-7493.2012.01.010
Whalen, J. B., Currie, K. L., and Chappell, B. W. (1987). A-type granites: geochemical characteristics, discrimination and petrogenesis. Contrib. Mineral. petr 95, 407–419. doi:10.1007/bf00402202
Wu, F. Y., Sun, D. Y., Ge, W. C., Zhang, Y. B., Grant, M. L., Wilde, S. A., et al. (2011). Geochronology of the Phanerozoic granitoids in northeastern China. J. Asian Earth Sci. 41, 1–30. doi:10.1016/j.jseaes.2010.11.014
Wu, G. (2006). Metallogenic setting and metallogenesis of nonferrous -precious metals in northern da Hinggan moutain. M.Phil. Diss. Jilin Univ. (in Chinese with English abstract).
Wu, G., Chen, Y. J., Sun, F. Y., Li, J. C., Li, Z. T., and Wang, X. J. (2008). Geochemistry of the Late Jurassic granitoids in the northern end area of Da Hinggan Mountains and their geological and prospecting implications. Acta Petrol. Sin. 24 (4), 899–910. (in Chinese with English abstract).
Wu, G., Chen, Y. J., Zhao, Z. H., Zhao, T. P., Li, Z. T., and Zhang, Z. (2009). Geochemistry, zircon SHRIMP U-Pb age and petrogenesis of the east luoguhe granites at the northern end of the Great hinggan range. Acta Petrol. Sin. 25 (2), 233–247.
Wu, G., Sun, F. Y., Zhao, C. S., Li, Z. T., Zhao, A. L., Pang, Q. B., et al. (2005). Discovery and geological significance of early paleozoic post collisional granites on the northern edge of the Erguna block. Sci. Bull. 50 (20), 2278–2288. (in Chinese with English abstract).
Xu, W. L., Pei, F. P., Wang, F., Meng, E., Ji, W. Q., Yang, D. B., et al. (2013). Spatial-temporal relationships of Mesozoic volcanic rocks in NE China: constraints on tectonic overprinting and transformations between multiple tectonic regimes. J. Asian Earth Sci. 74, 167–193. doi:10.1016/j.jseaes.2013.04.003
Yang, H. B., Liu, Y., Zheng, J. L., Liang, Z. K., Wang, X. Y., Tang, X. F., et al. (2017). Petrogenesis and geological significance of Neopro⁃terozoic amphibolite and granite in Bowuleshan area, Erguna massif, Northeast China. Geol. Bull. China 36 (2/3), 342–356. (in Chinese with English abstract).
Zhang, Y., Chen, W., Chen, K. L., and &Liu, X. Y. (2006). Study on the Ar-Ar age spectrum of diagenetic I/S and the mechanism of 39Ar recoil loss-examples from the clay minerals of P-T boundary in changxing, Zhejiang Province. Geol. Rev. 52 (4), 556–561. (in Chinese with English abstract).
Zhang, Y. L., Ge, W. C., Liu, X. M., and Zhang, J. H. (2008). Isotopic characteristics and their significance of the Xinlinzhen pluton, Great Hinggan mountains. J. Jilin Univ. Earth Sci. 38, 177–186. (in Chinese with English abstract). doi:10.1109/tmag.2018.2845903
Zhao, S., (2017). Neoproterozoic-Early Paleozoic tectonic evolution and attribute of the Erguna Massif: constraints from detrital zircon U-Pb geochronology and igneous rock associations. M.Phil. Dissertation of Jilin University (in Chinese with English abstract).
Zhao, S., Xu, W. L., Wang, F., Wang, W., Tang, J., and &Zhang, Y. H. (2016). Neoproterozoic magmatisms in the Erguna massif, NE China: evidence from zircon U-Pb geochronology. Geotect. Metallogenia 40 (3), 559–573. (in Chinese with English abstract). doi:10.16539/j.ddgzyckx.2016.03.012
Zheng, J. L., Zhang, W. Q., and Xu, L. M. (2015). Geochemistry characteristics and metallogenic prognosis of the volcanic rocks from Manitu Forma-tion of Bowule mountain area in Da Hinggan mountain. GoldScience Technol. 23 (1), 46–52. (in Chinese with English abstract). doi:10.11872/j.issn.1005-2518.2015.01.046
Zhou, J. B., Wilde, S. A., Zhang, X. Z., Ren, S. M., and Zheng, C. Q. (2011). Early Paleozoic metamorphic rocks of the Erguna block in the Great Xing'an Range, NE China: evidence for the timing of magmatic and metamorphic events and their tectonic implications. Tectonophysics 499, 105–117. doi:10.1016/j.tecto.2010.12.009
Keywords: LA-ICP-MS zircon U–Pb dating, intrusive rock, Xinlin area, muscovite 40Ar/39Ar dating, geochemistry
Citation: Lu S, Li C, Alam M, Song Z, Zhu X, Fu A and Yang W (2025) Geochronology and geochemistry of the Neoproterozoic–Mesozoic intrusive rocks in the Xinlin area, northeastern China: new constraints on the tectonic evolution of the Erguna block. Front. Earth Sci. 12:1514658. doi: 10.3389/feart.2024.1514658
Received: 21 October 2024; Accepted: 12 December 2024;
Published: 22 January 2025.
Edited by:
Hossein Azizi, University of Kurdistan, IranReviewed by:
Liang Qiu, China University of Geosciences, ChinaAbdolnaser Fazlnia, Urmia University, Iran
Copyright © 2025 Lu, Li, Alam, Song, Zhu, Fu and Yang. This is an open-access article distributed under the terms of the Creative Commons Attribution License (CC BY). The use, distribution or reproduction in other forums is permitted, provided the original author(s) and the copyright owner(s) are credited and that the original publication in this journal is cited, in accordance with accepted academic practice. No use, distribution or reproduction is permitted which does not comply with these terms.
*Correspondence: Chenglu Li, TENMMjMwODgxQDE2My5jb20=