- Department of Geography, School of Environment, Education and Development, University of Manchester, Manchester, United Kingdom
Introduction: Vegetation fires lead to the formation of charred materials, often referred to as pyrogenic carbon (PyC), which are recalcitrant and have a high carbon densitymeaning they have the potential to act as a long-term carbon store. In the United Kingdom, peatlands are periodically subject to fire, both management burns and wildfires, which generate PyC. However, in the United Kingdom context, the characterisation of physical and chemical properties of PyC is limited.
Methods: In this study, samples of peatland vegetation (Calluna vulgaris, Polytrichum juniperinum, Vaccinium myrtillus and Eriophorum vaginatum) were burnt in laboratory conditions across typical ranges of characteristics from United Kingdom peatland vegetation fires (250°C–800°C and 2–10 min burn duration). Four broad severity groupings were established (low, moderate, high, very high) corresponding to 60, 70, 80% and 90% mass loss respectively. The PyC samples were then analysed using Brunauer-Emmett-Teller (BET) surface area analysis, CHNO elemental analysis, and Fouriertransform infrared spectroscopy (FTIR) to gain a greater understanding of their physiochemical characteristics.
Results: While there was a good degree of similarity between samples within each severity group, there were significant differences between severity groups. Low to high severity samples had relatively low surface areas compared to the very high severity samples, which exhibited the greatest surface areas and a high degree of variability. O/C and H/C ratios decreased with increasing severity. FTIR showed that distinct spectra were produced between severity groups, reflecting increased sample aromaticity with burn severity.
Discussion: The findings of this study suggest that burn severity is a good predictor of PyC physiochemical characteristics.
1 Introduction
Pyrogenic carbon (PyC) is a refractory form of carbon produced by the incomplete combustion of organic matter, with estimates suggesting 50–270 Tg of PyC is produced globally per year from biomass burning (Kuhlbusch and Crutzen, 1995). PyC has the potential to remain relatively unaltered in soils and marine reservoirs for millennia (e.g., de Lafontaine and Asselin, 2011), though the residence times of PyC can vary markedly between different soil and hydrological conditions as well as differing starting fuel (Santín et al., 2016; Titirici et al., 2007). PyC is an important component of the global carbon cycle and has the potential to significantly contribute to terrestrial and aquatic carbon stocks (Bird et al., 2015). As such gaining greater understanding of PyC characteristics and degradation trends is of great importance to achieve a more complete understanding of wildfire impacts.
Globally peatland ecosystems are affected by fire, including wildfires and prescribed burns (e.g., Bargmann et al., 2016; Turetsky et al., 2015). Peatland fires are of international concern as they have the potential to emit substantial quantities of greenhouse gases during smouldering combustion of peat sequences. For example, 0.81–2.57 GtC were released during 1997/98 fires in Indonesia that burned 0.73 Mha of peatland (Page et al., 2002). Meanwhile, the equivalent of an estimated 0.16 GtCO2 is emitted from Canadian fires that burn ∼20,000–30,000 ha of boreal forest per year (Amiro et al., 2009). These emissions can be largely attributed to smouldering of peat layers, where substantial quantities of organic matter can be combusted under low intensity (i.e., low energy release or heat during combustion) burn conditions (e.g., Davies et al., 2013; Huang and Rein, 2014). Peatland carbon (C) cycling can also be affected by above-ground vegetation fires (i.e., fires without a smouldering peat soil component), including prescribed burns and non-drought fires (Davies et al., 2016). For example, through alterations to the composition of dissolved organic carbon (DOC) in surface runoff (e.g., Clay et al., 2012) or altered CO2 emissions correlating to reduced microbial abundance following fires due to mortality from exposure to elevated (i.e., ∼100°C) temperatures and altered soil physical (e.g., increased hydrophobicity) and chemical (e.g., reduced nutrient and C content) properties (Dooley and Treseder, 2012).
In the UK deep peat soils are typically defined as at least 40 cm depth (England and Wales) or at least 50 cm (Scotland and Northern Ireland) with at least 20%–40% organic matter, (JNCC, 2011), though these depth-based definitioins do not cover all possible peat soils (see IUCN UK Peatland Programme 2023). Many UK upland peatlands are periodically subjected to both management burns, typically for grouse-moor management on a small scale (<2 ha) in rotating patch schemes (Defra, 2007), and episodic wildfires that predominantly result from anthropogenic activities (Gazzard et al., 2016; McMorrow, 2011). Peatlands cover approximately 12% of the UK’s land area (Office for National Statistics, 2019) and are typically made up of varying mixes of woody shrubs (e.g., Calluna vulgaris, Vaccinium spp.), grasses and rushes (e.g., Eriophorum spp. and Juncus effuses), and mosses (e.g., Sphagnum spp., Polytrichum spp.). Douglas et al. (2015) state that 20%–60% of heather dominated moorlands are managed for grouse shooting but suggest that not all of this land will be managed by rotational burns. An estimated 18% of United Kingdom peatlands are subjected to prescribed burns, though these are unevenly distributed and primarily occur in upland areas (Worrall et al., 2010). Between 2009/10 and 2020/21, in England ∼360,000 wildfires were attended by the Fire and Rescue Services with just over 79,000 ha being burnt, 20,000 of which were in upland areas (Forestry Commission England, 2023). Of these 20,000 ha–77% were in mountain, heath or bog settings, ∼22% were in semi-natural grassland and <1% were in woodland (Forestry Commission England, 2023).
Previous research on UK peatland vegetation fires has focussed on C. vulgaris (hereafter Calluna) dominated ecosystems. Fires in these systems exhibit burn temperatures between 200°C–800°C (Nilsen et al., 2005; Santana and Marrs, 2014; Whittaker, 1961; Worrall et al., 2013) though this does vary within fire events. Peak burn temperatures in Calluna stands are typically ∼800°C but may be as low as ∼400°C in early growth vegetation (Hobbs and Gimingham, 1984). This range in temperature can be attributed to various factors including type, quantity and continuity of fuels present at a site, as well as fuel moisture content and the growth stage of the vegetation (Hobbs and Gimingham, 1987; Davies and Legg, 2011; Shetler et al., 2008). Thermocouple analysis has found the burn duration at any given point in Calluna systems to be relatively short (≤10 min; Hobbs and Gimingham, 1984). The degree of burn severity (i.e., the amount of biomass combusted per study plot) (sensu Keeley, 2009), can also vary within a single fire event (Worrall et al., 2013). For example, after a large 2008 wildfire in the Peak District, UK, around 86% of overall biomass was lost, though in some quadrats up to 50% of the initial biomass remained (Clay and Worrall, 2011). Variation in burn severity can occur within a single fire event with the potential to exert a range of effects on the peatland vegetation assemblages, water quality and carbon cycling (Harper et al., 2018). For example, areas of low severity fire can increase Sphagnum abundance by reducing shading canopy vegetation (e.g., Grau-Andrés et al., 2017). Meanwhile, areas experiencing higher severity burns can undergo various deleterious changes, such as altered peat hydrology (e.g., Holden et al., 2014), as well as potential for near complete Sphagnum dieback (Noble et al., 2019).
Burn conditions can have significant effects on the characteristics of resultant PyC, with fires producing a continuum of residues equating to increasing degrees of combustion completeness (Masiello, 2004). The range of PyC characteristics resulting from different burn conditions are indicative of physical and molecular changes that take place during the thermal transformation of plant matter (McBeath et al., 2014). Notable changes include losses of elemental hydrogen and oxygen and aliphatic compounds (Knicker, 2009; Knicker et al., 1996; Wiedemeier et al., 2015). High temperature burns will result in high degrees of aromatic condensation and the formation of ash under near complete combustion, whilst a greater proportion of uncharred material and charcoal with a low degree of aromatic condensation may be produced under lower temperature burns (Belcher et al., 2018; McParland et al., 2009). Burn duration can similarly affect PyC characteristics, with near complete combustion generally occurring during longer burns. Therefore, burn temperature and burn duration, and their interaction, are important factors for the resultant PyC produced (e.g., Hudspith and Belcher, 2017). As such, a key issue that requires investigation is whether ‘short-hot’ burns and ‘long-cool’ burns in UK peatland vegetation have the potential to create comparable PyC materials. The fuel of a fire also substantially affects the resultant PyC characteristics due to the range in plant physiology, moisture content and flammability. Highly combustible fine fuels such as grasses will have a tendency to form ash, whereas woody fuels may have a higher proportion of charcoal following a burn (Hudspith et al., 2018; Keiluweit et al., 2010). This is a key consideration as UK peatland fires interact with a range of fuel types, including woody shrubs (e.g., Calluna, Erica spp., Vaccinium myrtillus), grasses and sedges (e.g., Eriophorum vaginatum, J. effuses, Molinia caerulea) and mosses (e.g., Polytrichum spp., Sphagnum spp.).
There is a growing body of research in the UK relating to the impact of fire (both wildfires and managed burning) on peatland environments, including hydrology (e.g., Clay et al., 2009; Holden et al., 2015; Ramchunder et al., 2009), ecology (e.g., Ellis, 2008; Milligan et al., 2018; Noble et al., 2018), carbon cycling (e.g., Davies et al., 2013; Heinemeyer et al., 2018; Worrall et al., 2011) and pollutant mobilisation (e.g., Kettridge et al., 2019; Rothwell et al., 2007). However, there is still relatively limited data on PyC properties produced in UK peatland settings. Some recent PyC characterisation work has been undertaken outside of the UK (e.g., Gao et al., 2022). Note, this research is not evaluating the effects of fires on the characteristics of the peat soils or looking at the geochemistry of smouldering burns, rather it is evaluating the compositional changes of organic matter from above-ground vegetation fires.
This study therefore aims to characterise the range of PyC properties produced under laboratory conditions (1) from different UK peatland vegetation types and (2) under different burn severities. We hypothesise that: (1) PyC produced from woody fuels will exhibit greater aromaticity, aromatic condensation and carbon content than PyC produced from non-woody fuels; (2) PyC aromaticity, aromatic condensation and carbon content will increase with burn severity.
2 Methods
2.1 Sample production
Samples of UK peatland vegetation species were collected from the Kinder Scout region of the Peak District National Park, UK (53°23′04″N, 1°52′20″W). The species were C. vulgaris, E. vaginatum, Polytrichum juniperinum and V. myrtillus (hereafter Calluna, Eriophorum, Polytrichum and Vaccinium respectively). These samples were selected to be representative of ‘woody’ shrubs (Calluna and Vaccinium), and ‘non-woody’ grass (Eriophorum) and moss (Polytrichum) communities. A modest quantity (∼250 g) of vegetation was collected per species studied, with cuttings being taken from a 1 m2 area in an effort to reduce PyC feedstock variability. Vegetation cuttings were taken with secateurs, placed in sealed plastic bags in the field then stored at 4°C in a laboratory fridge to minimise moisture losses prior to PyC production. Initial moisture contents for Calluna, Eriophorum, Polytrichum and Vaccinium were 44 ± 1, 67 ± 5, 58% ± 2% and 41% ± 2%, respectively. Whilst moisture content is an important factor with regards to mass loss in early phases of combustion (Keiluweit et al., 2010), it was considered too difficult to manipulate as an experimental factor and as such it was recorded but otherwise left unaltered.
Based on biomass loss estimates from Worrall et al. (2013), and references therein), typical biomass losses from fires in UK peatland vegetation range between 60% and 100%. We used this mass loss range to define four different severity groups: ‘low severity’, ‘moderate severity’, ‘high severity’ and ‘very high severity’ (hereafter LS, MS, HS and VHS respectively) corresponding to 60%, 70%, 80% and 90% mass loss respectively. This range allowed us to simulate a PyC continuum ranging from materials that had only undergone minimal charring to those that have undergone near complete combustion, whilst also comparing the effects of ‘short-hot’ and ‘long-cool’ burn conditions. To achieve the desired mass losses, samples were burnt for durations of 2, 5 and 10 min, and exposed to constant temperatures which ranged from 250°C–800°C (see Table 1 for further details). These temperatures and durations are representative of field conditions (Hobbs and Gimingham, 1984; Hudspith et al., 2014) and have been used in other experimental studies (e.g., Santana and Marrs, 2014; Worrall et al., 2013).
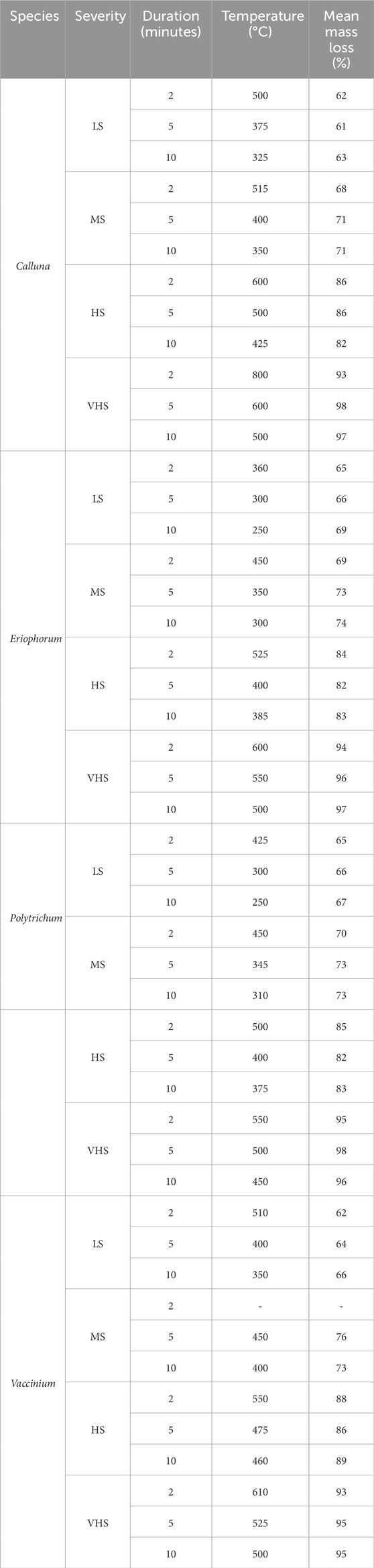
Table 1. An overview of the burn conditions used per species to achieve the desired mass loss percentages (produced from triplicate crucible burns) for the sample severity groups.
Vegetation samples, ∼1–2 cm in length, were weighed into 15 mL ceramic crucibles and placed in a Carbolite ELF 11/14 muffle furnace. Samples were not pre-dried and oxygen availability was not restricted (e.g., by wrapping fuels in foil) with samples burnt on the order of minutes rather than hours. Samples were placed inside the furnace as quickly as possible once they had reached the intended target temperature. Repeated triplicate burns were carried out until sufficient PyC material was available for analysis. Any individual sample that deviated more than 5% from the desired mass loss was discarded. Whilst muffle furnaces do not recreate the temperature curves and airflow conditions experienced in real-world wildfires, it is an approach that has been successfully implemented in previous UK peatland PyC studies (e.g., Worrall et al., 2013) and other related research (Bodí et al., 2011; Santos et al., 2016; Saputro et al., 2018). The chosen production method also allowed for observations against carefully controlled burn conditions, where open air burns provide greater realism at the cost of greater difficulty in studying specific fire scenarios (e.g., ‘short-hot’ and ‘long-cool’ burn conditions). Future research, without the aim of studying specific formation conditions, may wish to focus open-air PyC production methods (e.g., modified combustion efficiency) in order to maximise the real-world relevance of PyC formation conditions. Whilst much of the research looking at peatland wildfire phenomena assess smouldering fire conditions (i.e., limited oxygen combustion), the research presented here is part of a series of experiments evaluating above-ground fire scenarios, where vegetation is subjected to varying degrees of combustion. This research makes no attempt to study the effects of fire on peat or burning in low oxygen conditions.
2.2 Sample analysis
The PyC samples were subject to Brunauer-Emmett-Teller (BET) analysis in bulk to determine surface area. The PyC samples were then crushed and homogenized in an agate pestle and mortar and oven dried at 105°C for 24 h for subsequent Fourier-transform infrared spectroscopy (FTIR) and CHNO elemental analysis. Triplicate analyses were carried for each combination of factors (e.g., 3x 2 min LS Calluna, 3x 2 min MS Calluna, etc.).
2.2.1 Brunauer–Emmett–Teller (BET) surface area analysis
BET nitrogen adsorption surface area measurements were taken to track changes in the physical characteristics of PyC samples across the combustion continuum, where we would expect PyC to experience increases in surface area under high degrees of combustion completeness (e.g., Brewer et al., 2014; Sigmund et al., 2017). These analyses were performed using a Micromeritics Gemini V 2365. The samples were purged using a Micromeritics Flowprep 060 prior to analysis. Sample vials were purged under a flow of chemically pure grade nitrogen gas whilst being heated to 100°C for 2 h. The vials were subsequently air cooled and weighed before ∼0.2 g of sample was added and again purged for approximately 18 h. Once the vials had again cooled, they were weighed and the empty weight was subtracted to calculate the mass of the purged sample within. Measurements were performed at 77 K, a standard approach to this analysis (e.g., Brewer et al., 2014).
2.2.2 Elemental (CHNO) analysis
Elemental analyses were performed to track changes in key elements (i.e., carbon, hydrogen, nitrogen and oxygen) across the combustion continuum, where we would expect PyC to exhibit high C and low H and N concentrations under high degrees of combustion completeness (e.g., Keiluweit et al., 2010). Sub-samples of ∼1–2 mg of PyC were analysed on a Thermo Scientific Flash 2000 CHNS-O analyser. CHN was analysed via flash combustion in oxygen, and gases were then reduced to convert nitrous oxides to N using a copper reaction tube. O analysis used a pyrolysis reaction with nickel coated carbon to form carbon monoxide. A gas chromatograph then separated gases which were then measured via a thermal conductivity detector. A six-point calibration curve (R2 >0.999), produced from a methionine standard, was used to calculate the concentration of each element by calculating the area under peaks. Each sample was analysed in triplicate, i.e., three times on the CHN setup and a further three times on O set up, and a mean % calculated for C, H, N, and O.
2.2.3 Fourier transform infrared spectroscopy (FTIR)
FTIR analysis was performed to track changes in PyC molecular composition across the combustion continuum, where previous studies have shown increasing aromaticity in PyC with progressive combustion (Wiedemeier et al., 2015). Analysis of samples was completed using a Perkin Elmer Frontier benchtop FTIR unit, with µATR attachment for coupling to samples. The instrument was set to the mid infrared function, covering 4000–650 cm-1, with 16 repeats performed per spectrum at a resolution of 4 cm-1. The results were collected using Perkin Elmer Spectrum software. The data was normalised by subtracting the minimum value and dividing by the mean of the spectra to account for differences in amplitude and baseline value (Artz et al., 2008). Following this initial normalization procedure, spectra underwent baseline correction using the baseline package (Liland et al., 2010) in R version 4.0.3 in order to remove the effects of spectral drift (R Core Team, 2020).
Following normalization and baseline correction of the data, individual wavenumbers were selected for analysis of spectral peaks (e.g., Rytwo et al., 2015), with the wavenumber corresponding to the maximum absorbance value per peak selected for ratio calculations (Table 2). Spectral ratios were calculated to track overall change in PyC quality and losses of H and O containing molecules with progressive combustion. These ratios have previously been used as proxy measures of OM dehydration (Sharma et al., 2004), losses of labile molecules (Guo and Bustin, 1998; Vítek et al., 2017), increasing degrees of carbon concentration in aromatic molecules (Pyle et al., 2015) and the degree to which aromatic molecules are arranged in organised lattices (Guo and Bustin, 1998).
Whilst alternative forms of FTIR (e.g., DRIFT) have the to successfully analyse heterogeneous samples via beam contact with multiple points on a sample (e.g.,.Hobley et al., 2014; Uhelski et al., 2022), µATR was the preferred method owing to the ease of analysis, the large sample set analysed here and the relatively fast sample preparation. Additionally, the inclusion of sample replication captured the spectra variability that might otherwise be captured by DRIFT analysis. However, future research may wish to adopt the DRIFT method, particularly when analysing PyC residues that may be composed of mixed materials (e.g., high degrees of surface charring and low degrees of interior charring).
2.3 Statistical analysis
Statistical analyses were performed using IBM SPSS version 28 (IBM Corp. Released, 2021). The data were non-normally distributed necessitating the use of non-parametric Kruskal–Wallis one-way ANOVA. The study can be considered a two-factor experiment: species and severity. The species factor had four levels (Calluna, Eriophorum, Polytrichum, Vaccinium) and severity also had four levels (LS, MS, HS, VHS). All relationships were assessed at the 95% confidence level (p < 0.05). Pairwise comparisons were performed using a Kruskal–Wallis 1-way ANOVA with Bonferroni corrections. Eta-squared values (η2) were calculated to determine each factor’s effect size (Meyer et al., 2018).
Principal component analyses (PCA) were performed using Past 4 (a freeware software statistical package originally designed for palaeoenvironmental data; Hammer et al., 2001) to assess the clustering of samples in relation to different production conditions. Individual PCA were performed on each vegetation species, using the CHNO data and FTIR ratios. BET results were excluded from the PCA due to missing values in this dataset. To remove the effects of differences in scale between variables, data were normalised by subtracting the average value and then dividing by the standard deviation of that variable for all values in each variable. Components with eigenvalues >1.0 were retained (Wold et al., 1987).
3 Results
3.1 Characteristics of UK peatland vegetation PyC
3.1.1 Brunauer–Emmett–Teller (BET) surface area analysis
The results of the BET analysis are presented in Figure 1 and Table 3. Surface areas were generally low for LS to HS samples, with markedly higher surface areas for VHS samples. The exception to this was Calluna which underwent significant surface area increases under HS conditions. For all four species, the mean surface area of LS and MS samples was <5 m2/g. For HS samples, most samples also remained below 5 m2/g, except for the HS Calluna samples, which all had surface areas that exceeded 10 m2/g. The maximum surface areas were all found in VHS samples, and in most cases, VHS samples exhibited mean surface areas >10 m2/g. Calluna, Vaccinium and Eriophorum each had individual VHS samples with surface areas >100 m2/g, whilst the highest individual Polytrichum surface area was 52.4 m2/g. Variation in surface area values existed within each severity group and this variability increased with increasing severity. Observed surface area differed strongly for the VHS samples, with values differing by orders of magnitude for all species other than Polytrichum. The VHS samples showed increasing surface area with longer duration burns for Eriophorum and Polytrichum. The opposite is true for Calluna and Vaccinium, which both gave higher surface area readings for the 2 min burns than the 5 and 10 min burns (see Supplementary data).
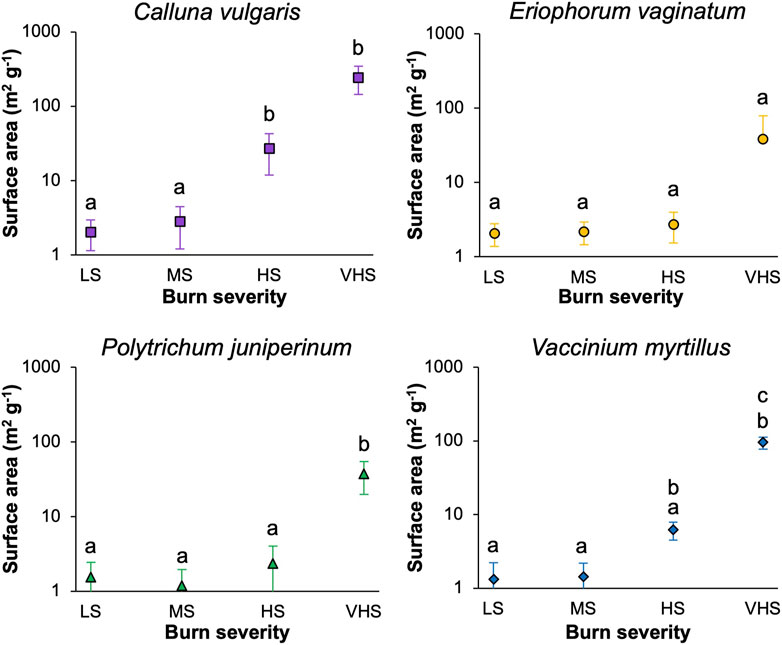
Figure 1. Mean BET surface area results plotted against burn severity for the four species, note the logarithmic Y-axis. Standard deviation error bars are included for all points.
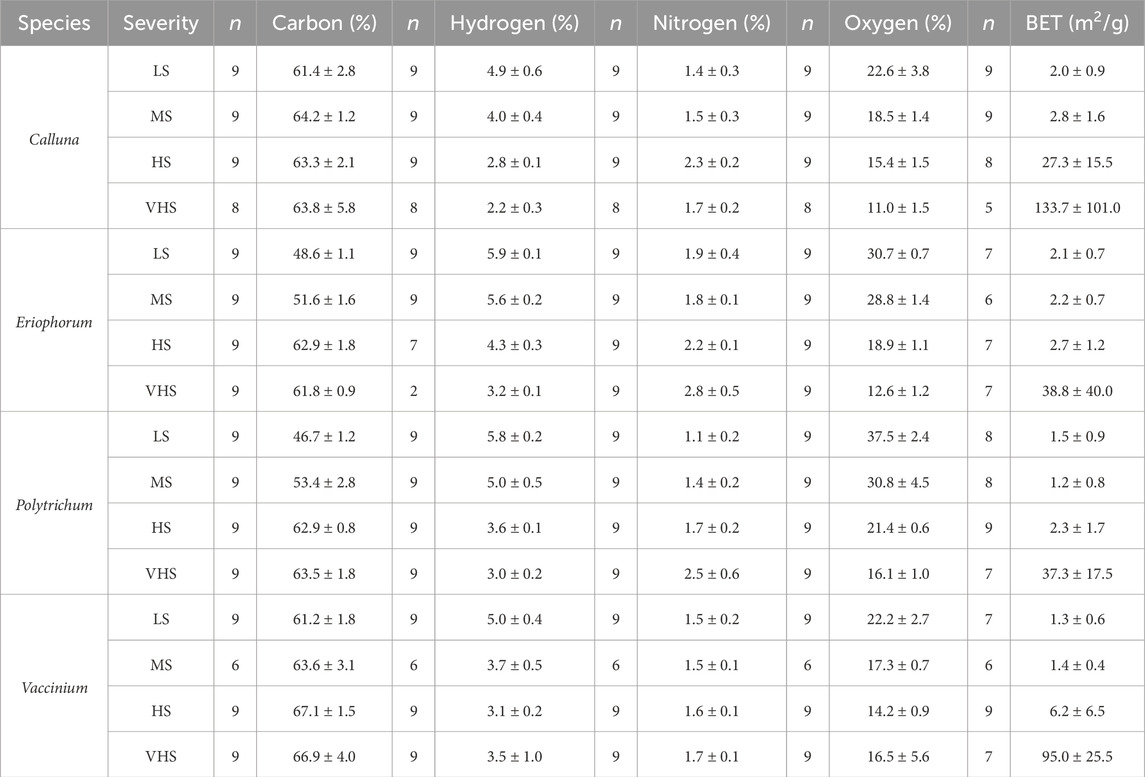
Table 3. Elemental (CHNO; %) and surface area (BET; m2/g) analyses. LS = low severity, MS = moderate severity, HS = high severity, VHS = very high severity. n = number of analyses.
The results of Kruskal–Wallis ANOVA can be found in Table 4. There was no significant difference in surface area between the different species (p = 0.063), but severity was a significant factor (p < 0.001; Table 4). Here severity explained 53.7% of observed variance. Post-hoc tests of the entire dataset show that the VHS and HS samples were significantly different to each other and to all other burn severity groups, but there was no significant difference between the LS and MS samples (Table 5).

Table 4. Kruskal–Wallis test results for ‘severity’ and ‘species’ factors for each PyC parameter: elemental ratios; surface area via BET (g/m2); FTIR ratios. Effect sizes are given as η2 values.
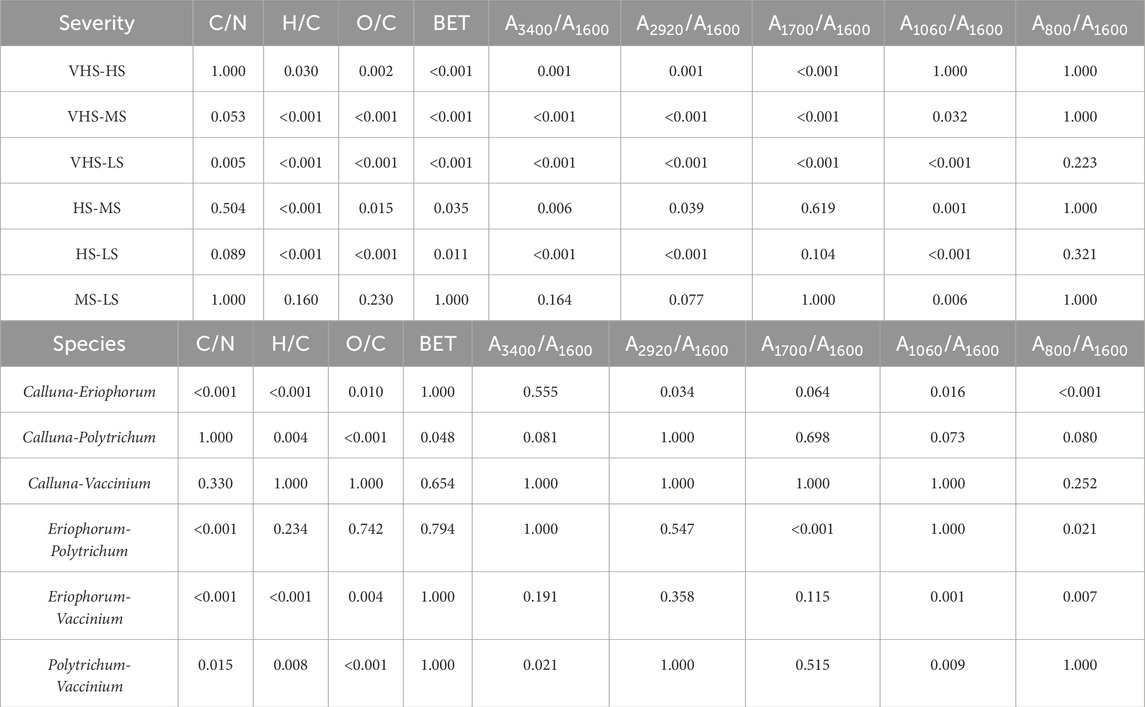
Table 5. Pairwise comparisons of the measured dependant variables (elemental ratios; surface area via BET (g/m2); FTIR ratios) against ‘severity’ and ‘species’ as determined via Kruskal–Wallis tests. Significance values have been adjusted by the Bonferroni correction. LS = low severity, MS = moderate severity, HS = high severity, VHS = very high severity.
3.1.2 Elemental (CHNO) analysis
Elemental analyses found consistent patterns of change across all the vegetation types (Table 3). The C content of the woody shrubs (Calluna and Vaccinium) showed limited change across the burn severities (61%–67%) but varied more markedly for the non-woody fuels (Eriophorum and Polytrichum: 47%–62%). N content remained low across the samples (1%–3%). Both H (3%–6%) and O (11%–30%) consistently decreased with increasing severity.
Van Krevelen biplots, where H/C ratios are plotted against O/C ratios, are an effective way of discussing this type of elemental data (e.g., Hammes et al., 2008; Nocentini et al., 2010). This provides a simplified means by which to track reductions in functional groups present in a sample (Preston and Schmidt, 2006). These can be found in Figure 2. In all cases H/C and O/C ratios decrease with increasing burn severity, with increasing sample C density towards the origin on both axes reflecting losses of H and O respectively.
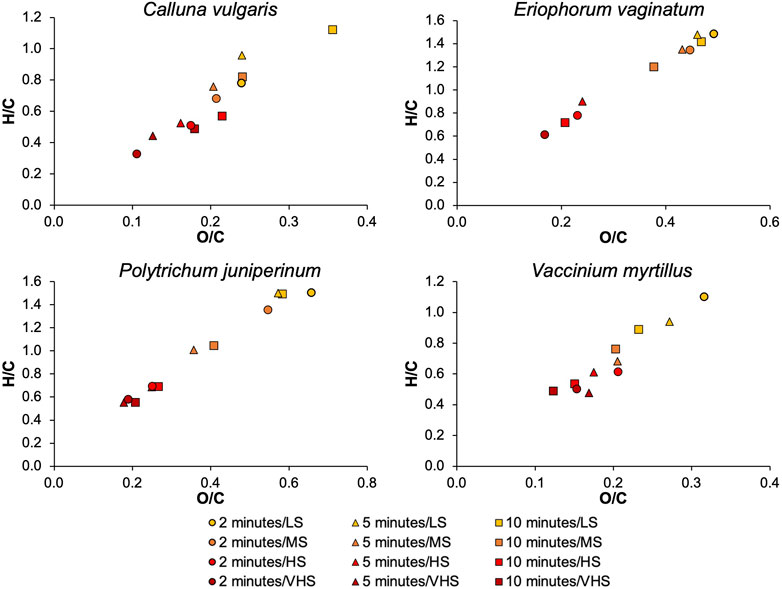
Figure 2. Elemental ratio biplots showing compositional change with increasing burn severity. Note the differences in axis value ranges.
Whilst there is no significant difference between severity groups for Vaccinium (Figure 3), Calluna remains largely unchanged between LS and MS, then undergoes reductions in C/N values by HS. Calluna C/N then returns to a similar level to LS and MS by VHS. C/N values show no significant change between MS and HS samples for the non-woody fuels. Polytrichum C/N values for LS and VHS are significantly different, whilst VHS samples are significantly different to MS and HS samples for Eriophorum.
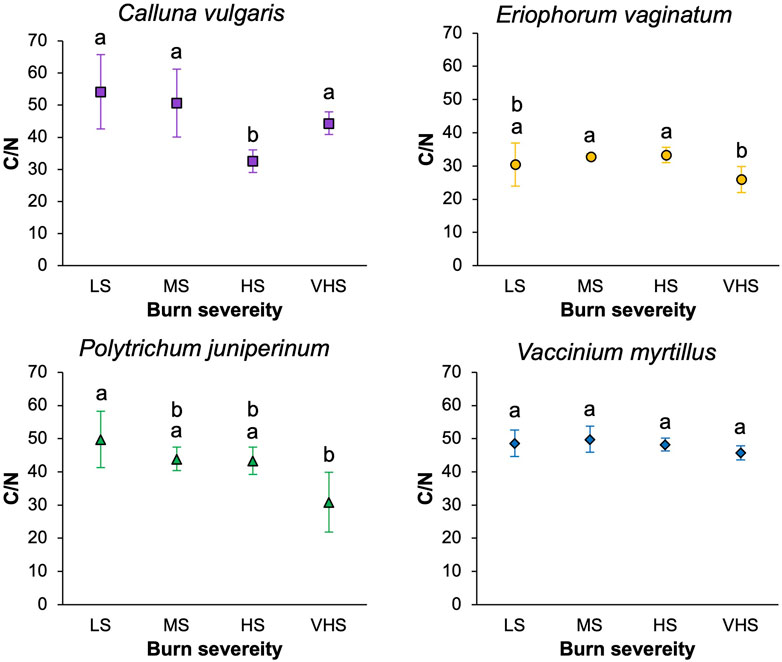
Figure 3. Mean (± standard deviation) for C/N ratios for each severity group for each species. Significant differences between samples are denoted by different letters.
The results of the Kruskal–Wallis ANOVA can be found in Table 4. Both ‘species’ and ‘severity’ were significant factors with respect to C/N, H/C and O/C ratios. ‘Species’ explained 58, 32% and 29%, and ‘severity’ explained 8, 92% and 81% of the variability in C/N, H/C and O/C ratios respectively. Pairwise comparisons showed that there were no significant differences between any of the measured elemental variables for the woody fuels (Calluna and Vaccinium; Table 5). The only significant difference in non-woody fuels (Eriophorum and Polytrichum) was with the C/N ratios (Table 5).
3.1.3 Fourier transform infrared spectroscopy (FTIR)
The mean FTIR spectra for each species and severity combination are presented in Figure 4. Broad shifts can be observed across the severity spectra for each species, with some strong similarities within the woody and non-woody fuels. The primary changes to sample spectra occur at 3400–3300 cm-1, 3000–2800 cm-1, 1700 cm-1, 1060–1030 cm-1 and 900–700 cm-1 (bands 1, 2, 3, 5 and six on Figure 4). 1610–1560 cm-1 (band 4 on Figure 4) is largely unchanged across the burn severities. There are distinct differences in FTIR spectra between the severity end-members (i.e., LS and VHS) across all species, with a general loss of oxygen and hydrogen containing aliphatic features, indicating increasing aromaticity with increasing burn severity.
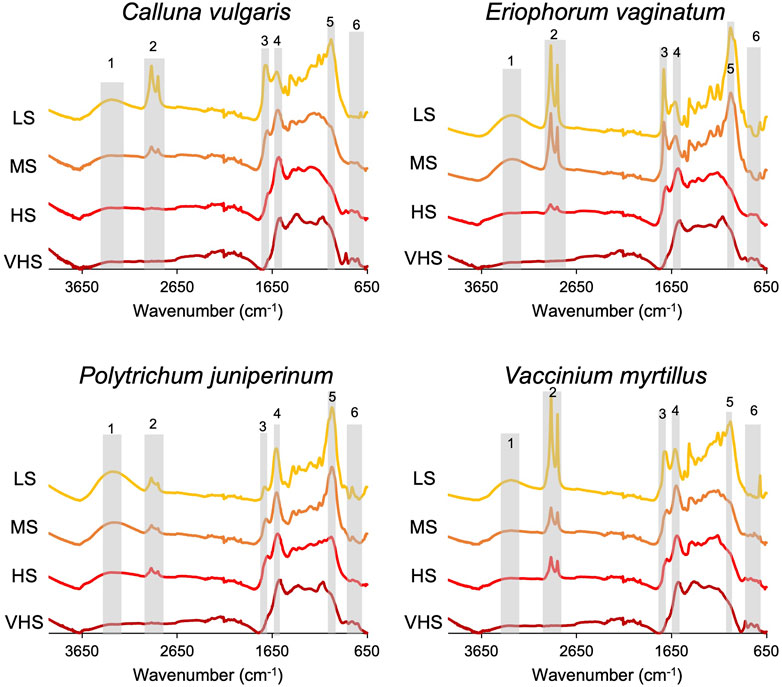
Figure 4. Mean FTIR spectra for each burn severity for each species. LS ‘low severity’, MS ‘moderate severity’, HS ‘high severity’, VHS ‘very high severity’. Key bands of interest: 1. 3400–3300 cm-1 (-OH), 2. 3000–2800 cm-1 (CH), 3. 1700 cm-1 (C=O), 4. 1610–1560 cm-1 (C=C), 5. 1060–1030 cm-1 (C–O), 6. 900–700 cm-1 CH). See Table 2 for discussion of key band ratios.
Peak ratios, in particular A1700/A1600 and A2920/A1600, show marked changes with increasing burn severity (Figure 5). 1610–1560 cm-1 (band 4 in Figure 4) remains relatively abundant across the burn severity groups, whilst decreases occur at 1700 and 2920 cm-1 as severity increases reflecting the loss of O and H containing functional groups (Figures 2, 4). Peaks at 1060–1030 cm-1 undergo clear changes between LS and MS samples for Calluna and Vaccinium, though remain unchanged in Eriophorum and Polytrichum until HS burn conditions. Distinct spectral features at 1060–1030 cm-1 are no longer present for the VHS samples. The 1700 cm-1 peaks undergo rapid reduction in absorbance by the MS samples, with the exception of Eriophorum where clear reductions in absorbance occur by HS, with no evident features in this region by VHS. Features in the range of 3000–2800 cm-1 show a clear response to increasing burn severity, though this varies by species, with no observable peaks by VHS. Broad features at 3400–3000 cm-1 (band 1 in Figure 4) are generally no longer distinguishable by HS. Across all sampled species and the burn severities used, aromatic C=C features at 1610–1560 cm-1 remain relatively abundant. Whilst spectral variation is evident, there are no clear and consistent trends of burn duration on overall spectral composition.
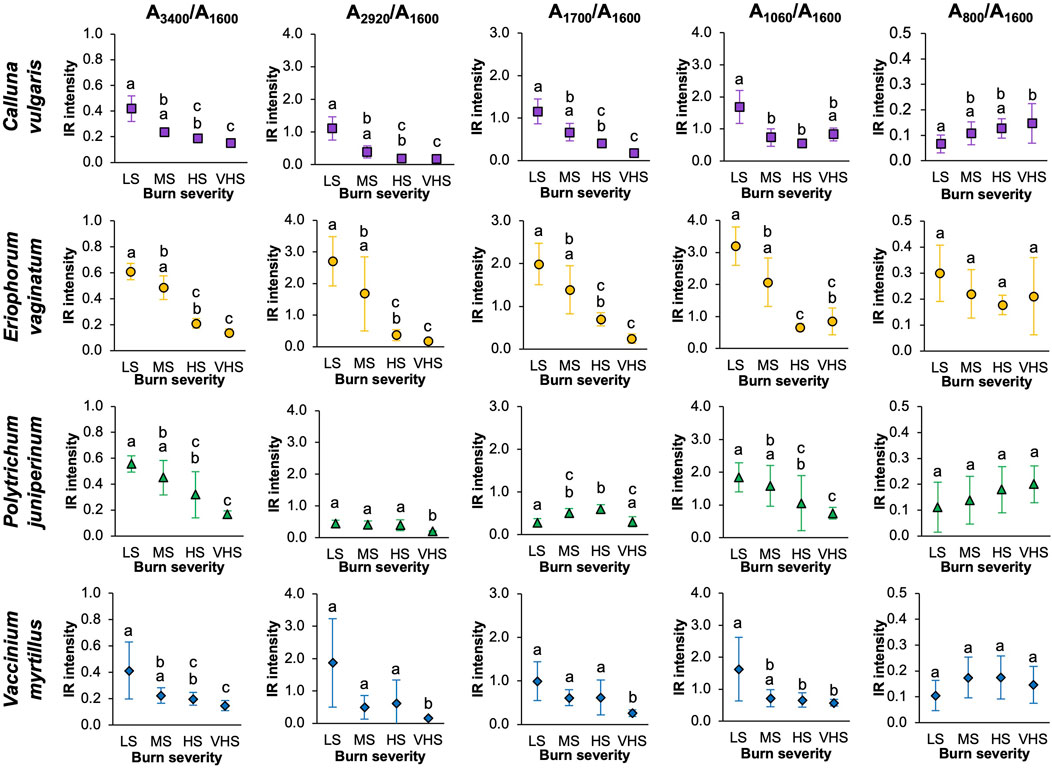
Figure 5. Mean (± standard deviation) spectral ratios for the four species and by severity groupings. Mean values for each severity group with standard deviation error bars. The different letters represent significant differences between groups.
‘Severity’ was a significant factor for all FTIR ratios (except for A800/A1600) where ∼60–90% of variance was explained (Table 4). ‘Species’ was also significant for all FTIR ratios though explained a lower proportion of variance (∼2–20%; Table 4). There were several significant pairwise comparisons (Table 5), typically between VHS samples and other severity levels, whilst LS and MS samples were generally not significantly different to each other. These findings indicate a clear effect of progressive burn severity, though the exact patterns of change differ by FTIR ratio. Whilst there were also some pairwise comparisons between species, these were less consistent (Table 5), though Calluna and Vaccinium consistently showed no significant difference to each other across all FTIR ratios.
3.2 Principal component analysis of PyC residues
The results of the PCA are shown in Figure 6. For Calluna and Eriophorum PC1 explained ∼70% variance, whilst >55% variance was explained in the case of Polytrichum and Vaccinium. PC2 explained <20% of variance for all species (Table 6).
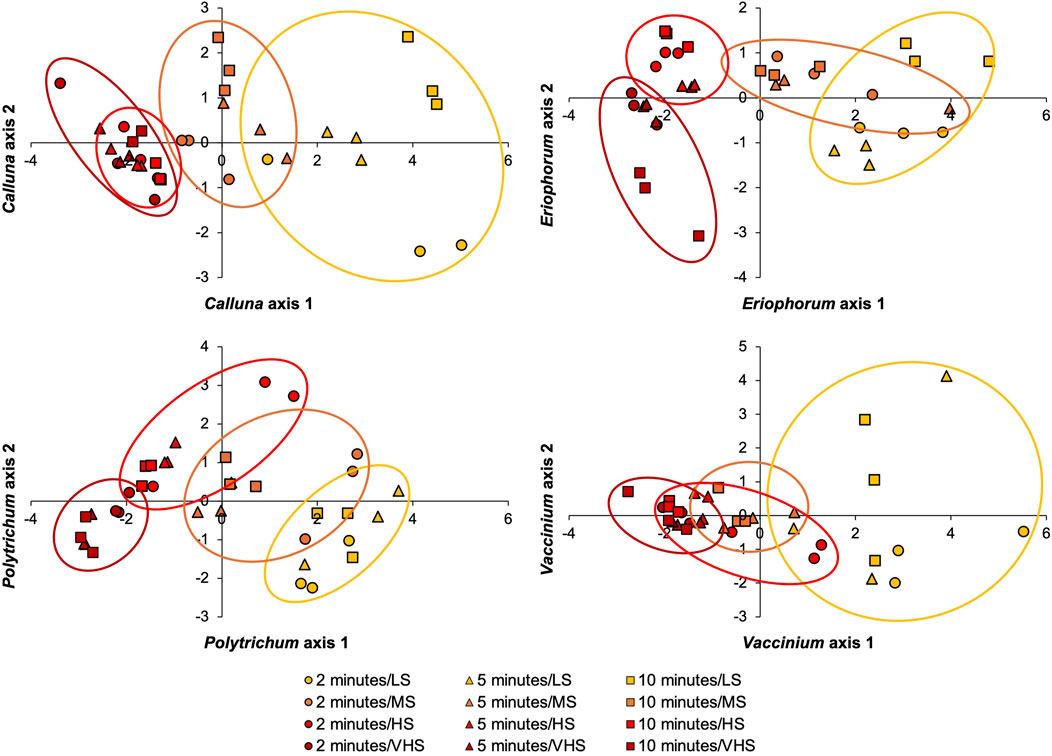
Figure 6. Principal component analysis of elemental data for four vegetation species under different burn severities. Burn severity varies by symbol/ellipsoid colour and duration of burn varies by symbol shape.
For all species, PC1 values increase with H/C, O/C, C/N, A3340/A1600, A2920/A1600 and A1060/A1600 values, whilst A800/A1600 values decline for all species except Eriophorum. Additionally, A1700/A1600 undergoes very slight declines for Polytrichum as PC1 increases. Therefore, PC1 essentially tracks moisture evolution and thermal degradation of aliphatic components, with lower values indicating a greater degree of aromatic condensation in most species. The patterns of PC2 are less consistent across the different species. Woody species see increases in A800/A1600 and decreases in A2920/A1600 and A1700/A1600 as PC2 values increase, indicating combustion of aliphatic components and increases in the degree of aromatic condensation. Non-woody species undergo increases in A3340/A1600, A1700/A1600 and C/N values as PC2 increases.
With the exception of Vaccinium, the different burn severity groups produce distinct clusters on the PCA axes demonstrating that they represent comparable materials in spite of differing formation conditions (i.e., burn durations).
4 Discussion
4.1 Characteristics of UK peatland vegetation PyC
Clear physical and chemical changes were evident with increasing burn severity for peatland PyC, though trends differed by fuel type. These changes to PyC characteristics included increasing carbon concentration and aromaticity, and consistent shifts in elemental and spectral composition with greater burn severity. The observations discussed here allowed for a partial acceptance of both hypotheses owing to mixed results from fuel types and the analytical methods employed.
The methods used to produce the samples meant that LS samples had small quantities of uncharred material remaining in some cases. Whilst some small components of the samples remained uncharred with LS samples, reflecting very early phases of combustion that could be seen to relate to peripheral fire effects, this was no longer present by MS burn conditions. Early changes in PyC elemental composition likely reflect dehydration reactions between LS and MS, whilst shifts in H content at higher burn severities likely relate to dehydrogenation and demethylation, and reductions in O abundance through decarboxylation reactions (Hammes et al., 2006). These changes in elemental composition mirrored progressive reductions in peaks at 3400–3300 cm-1, 3000–2800 cm-1 and 1060–1030 cm-1, reflecting the losses of–OH, aliphatic CH, and C-O or alcohol–OH compounds respectively (Figures 4, 5), which have been shown to lose absorption intensity with relatively low combustion temperatures (∼300°C) (Guo and Bustin, 1998; Marchessault, 1962). NMR studies of similar low severity PyC have found very low levels of carbohydrate and methoxyl C, diminishing O-aryl and alkyl C, and the emergence of aromatic aryl spectral features that are prominent in higher severity PyC (350°C–700°C) (McBeath et al., 2011). Whilst–OH and CH were largely absent in the HS samples, this pattern differed across species. For example, Calluna and Eriophorum showed marked reductions in CH molecules from LS to HS samples, whilst Polytrichum and Vaccinium showed no significant difference across this range. These compounds can be affected by low burn temperatures, but dehydroxylation has been observed to occur at relatively high temperatures (440°C–620°C) (Lammers et al., 2009). Thus, the LS, MS and HS samples presented here may still have been undergoing dehydroxylation. These molecular changes, alongside peaks at 1600 cm-1 persisting across species and burn severities (Figures 4, 5), reflect increases in PyC aromaticity with burn severity and thus support hypothesis 2.
A800/A1600 values observed in the samples presented here (Figure 5) varied between fuels but generally showed increases with burn severity. In the case of Eriophorum, the lowest degree of sample condensation is at HS, with declining signals to this point likely reflecting losses of aliphatic CH (e.g., see Figure 5 in Guo and Bustin, 1998). However, ‘severity’ was not found to have a significant effect on this condensation proxy, which was not anticipated and thus deviates from the expectations of hypothesis 2.
Indicators of the degree of aromatic condensation were greatest for Eriophorum suggesting greater losses of amorphous biomass structures with more formation of graphitic sheet structures in this fuel than the other fuels investigated, potentially pointing to Eriophorum being more susceptible to molecular changes under the burn conditions used here relative to the other species investigated. This finding in conjunction with the significant effect of species on A800/A1600 (Table 4) directly contradicts hypothesis 1. The non-significant effect of burn severity on A800/A1600 potentially relates to the relatively short duration/low temperature conditions used here (2–10 min, 250°C–800°C), whereas increases in condensation have been noted in the range of 400°C–1000°C in previous studies (Schneider et al., 2010; Wiedemeier et al., 2015). The burn conditions used in this study may therefore have resulted in dehydration occurring up to the HS samples presented here, meaning that apparent early declines in condensation likely reflect losses of H in the range of ∼800 cm-1 and increases in A800/A1600 for HS and VHS samples are indicative of the formation of aromatic complexes. In addition to differences in response to specific burn conditions between fuels, the significant effect of ‘species’ on A800/A1600 values may be the result of differing lignin content present in the ‘non-woody’ and ‘woody’ species. In previous research this has been found to result in a greater presence of aromatic complexes in ‘woody’ fuels than ‘non-woody’ fuels under higher severity burn scenarios (Wurster et al., 2013). However, this trend is not reflected in the data presented here (Figure 5), suggesting that further analysis (e.g., BCPA) may be necessary to more comprehensively understand the molecular changes occurring in United Kingdom peatland PyC under more complete combustion (Schneider et al., 2010).
For some samples there was relatively little change between MS and HS for certain measures. For example, features at 2920 cm-1 and 1700 cm-1, and A2920/A1600 and A1700/A1600 values, for Polytrichum and Vaccinium (Figures 4, 5) showed <0.2 change in ratio values. However, for some species (e.g., Eriophorum) this phase of the sample continuum shows much more marked changes. This reflects a transitionary phase between largely unaltered plant material, where original plant structures remain, to more amorphous char materials. Here continued dehydration and depolymerization of cellulose and lignin likely led to the formation of volatile compounds and randomly arranged aromatic structures, with pore space beginning to increase (Keiluweit et al., 2010). Spectral features at 2920 cm-1 and 1700 cm-1 were amongst those identified both in this study and by Uhelski et al. (2022), with 1700 cm-1 being strongly associated with peat-bound PyC. Uhelski et al. (2022) suggest that spectral features in the range of 1700 cm-1 in PyC may arise from field aging of PyC, indicating that it has potential in PyC field degradation studies (e.g., Kennedy-Blundell et al., 2023). However, the variable response of certain spectral features across the species studied here suggests that further investigation of PyC produced from a range of United Kingdom peatland fuels is required to more fully understand the relationship between initial biomass and resultant PyC characteristics.
The spectra of the HS samples, particularly in the woody fuels, showed clear losses of C-O rich molecules (1060–1030 cm-1) (Figure 4), characteristic of cellulose and hemicellulose (Yang et al., 2007). These spectral changes suggest a relative increase in lignin abundance, which persisted across a wider temperature range than the less thermally resistant cellulose (Mackay and Roberts, 1982). However, Calluna and Eriophorum showed reduced absorbance at 1700 cm-1 showing losses of C=O bonds, whilst C=C bonds remained relatively abundant (Figures 4, 5). This changing ratio of C=C to C=O molecules likely reflects emissions of CO and CO2, with gas phase FTIR studies showing these to be the primary products of lignin combustion and aromatization (Cao et al., 2013). These changes, alongside O/C and H/C shifts towards 0,0 in the Van Krevelen plots (Figure 2), indicate more complete combustion of samples, with increasing shifts away from the properties of the initial biomass towards ‘composite chars’ as discussed by Keiluweit et al. (2010). These composite chars will be partially composed of aliphatic and O containing compounds, which are preserved alongside aromatic components, with these labile components being largely absent at higher burn severities PyC (Keiluweit et al., 2010). These shifts in elemental composition (i.e., declining O/C and H/C values) alongside molecular changes support hypothesis 2.
C/N values differed across the vegetation species and fuel types (i.e., woody vs. non-woody). Additionally, C/N values at different burn severities varied by fuel type but generally decreased by HS and VHS (Figure 3), potentially reflecting thermal degradation of amino acids and amide groups under higher severity combustion. These biomass components were found to remain relatively stable in simulated fires at 350°C for up to 90 s (Knicker et al., 1996), though these conditions do not equate to those observed for United Kingdom peatland settings or the conditions under which PyC is typically formed. Pyle et al. (2015) found that %N responded to charring intensity thresholds (rather than changing linearly), with the greater losses for non-woody than woody fuels. They proposed that N is transformed into heterocyclic and aromatic compounds before being decomposed at higher severities. The chemical transformations noted by Pyle et al. (2015) were similar to the trends observed for non-woody C/N values in this study (Figure 3); however, the trends varied by fuel suggesting that charring thresholds may have differed by initial fuel composition.
Changes in PyC physical characteristics were limited in low to high severity samples, particularly with non-woody fuels, where surface area values were generally <10 m2/g. Higher burn severities consistently produced samples with the greatest surface area (Figure 1) suggesting that physical changes, such as the generation of fine ash particles and soot microstructures (e.g., Hammes et al., 2008), are strongly linked to burn severity. Furthermore, as Keiluweit et al. (2010) state, the surface area of low burn severity chars may have been limited by lack of atomic pore space and blocking of pores by recondensation of volatile matter. Additionally, high burn severity (particularly VHS samples) PyC samples may have experienced an increased lateral growth in graphene-like sheets and development of nanopores (Keiluweit et al., 2010), potentially explaining the surface area and A800/A1600 increases between the HS and VHS Calluna and Eriophorum samples presented in this study.
Whilst surface area underwent marked increases between HS and VHS, large standard deviations relating to differing burn characteristics were observed at HS and VHS, particularly for Calluna. Brewer et al. (2014) highlighted the importance of pyrolysis conditions on PyC physical characteristics, which can be seen in our results: Calluna and Vaccinium show the greatest surface area with high temperature 2 min burns, whilst Eriophorum and Polytrichum surface area values were greatest with lower temperature 10 min burns (see Supplementary data). Increasing surface area values have been observed with greater burn temperatures (e.g., Keiluweit et al., 2010; Sigmund et al., 2017) and in some cases burn duration (e.g., Sun and Zhou, 2008). However, the finding from this study, that PyC produced from differing fuel types (i.e., woody vs. non-woody fuels) may exhibit divergent physical characteristics from comparable burn conditions, is a novel observation and may warrant further investigation. In spite of this interesting trend, there were no significant differences in BET values produced by the four different species used in this study. As such, despite woody shrubs returning higher overall BET values and responding differently to burn temperature/duration conditions, surface area values only diverged from those of the non-woody fuels under the highest severity burn conditions (Figure 1). Therefore, shifts in PyC physical characteristics respond to complex interactions between burn conditions and initial fuel.
Throughout the analyses presented here the woody shrubs (Calluna and Vaccinium) showed no significant difference to each other (Table 5). The non-woody species produced a wider range of CHNO values than the woody species (Table 3), with higher H/C and O/C values (Figure 2), suggesting a lower degree of aromatisation and dehydration, particularly in the lower severity non-woody samples (Knicker, 2009). This range in CHNO values between PyC fuels likely related to differing characteristics of the unburnt fuels, where the greater lignin content of the woody fuels will have resulted in thermal decomposed over a higher temperature range then the non-woody fuels (Yang et al., 2007). Consequently, the woody shrubs generally required higher treatment temperatures (325°C–800°C) than the non-woody species (250°C–700°C), in combination with the set burn durations, to achieve the desired mass loss percentages. This highlights the importance of considering starting fuel characteristics, burn temperature and burn duration when discussing lab produced PyC samples and their characteristics (e.g., Gibson et al., 2018; Pyle et al., 2015; Santín et al., 2016). Finally, our results show that varying combinations of burn temperature and duration can result in broadly comparable PyC residues for each severity class, but combined physical, elemental and molecular techniques may provide a strong basis for identifying specific PyC formation conditions.
4.2 Wider significance
Whilst post-fire burn severity estimates allow for insights into fire behaviour and estimates of biomass loss, they provide limited insight into the key details of burn characteristics. The coupling of PyC mass loss estimates with tools like the composite burn index (CBI) (e.g., Davies et al., 2016), may allow for greater understanding of the burn severity experienced in a given study area after a fire (Key and Benson, 2006). For samples with unknown formation conditions, it may be possible to infer PyC characteristics (e.g., degree of aromaticity) from simple mass loss estimates. These inferences may be strengthened by comparisons to PyC produced under known conditions (i.e., burn temperature and burn duration). It is important to note though that, whilst the general observations made here are comparable to other studies (e.g., Guo and Bustin, 1998; Hammes et al., 2006; McBeath et al., 2011; Uhelski et al., 2022), clear differences can occur between lab and field burn conditions, meaning that caution should be taken when comparing between PyC properties generated in these two settings.
By coupling fire severity estimates with an improved understanding of how PyC changes over time, it may be possible to predict the longevity of PyC and understand the longer-term impacts of fire on C cycling in United Kingdom peatlands. Kennedy-Blundell et al. (2023) provide one of the few studies that has looked at in-field PyC degradation in United Kingdom peatlands, but this was limited to a short term (≤1 year) field campaign. Long term studies (i.e., multi-year) are needed to gain a greater understanding of PyC degradation prior to incorporation into peat sequences or their mobilisation to other parts of the landscape. Such research is key as the landscape the PyC was produced in, including nearby aquatic systems, constitutes the main depositional settings for PyC over short to moderate timescales (e.g., Abney et al., 2019; Smith and Dragovich, 2008). Studies that have analysed PyC-soil interactions (e.g., DeCiucies et al., 2018; Liang et al., 2010) have observed decreases and increases in native soil carbon due to the presence of PyC (e.g., Maestrini et al., 2015; Zimmerman et al., 2011), with PyC also being noted as an important contributor to aquatic DOC (Wagner et al., 2018).
The role of PyC in carbon cycling in United Kingdom peatlands has been relatively understudied (with some notable exceptions, e.g., Clay and Worrall, 2011; Heinemeyer et al., 2018; Worrall et al., 2013), meaning that fundamental C cycling mechanisms may have been overlooked in these settings (e.g., early PyC derived C fluxes, PyC incorporation into peat, transport to the aquatic systems). Therefore, this study has added valuable new insights in this research lacuna.
5 Conclusion
This study has characterised PyC from common peatland vegetation species produced under lab conditions and grouped by severity. The PyC production methods applied here created a continuum of PyC, ranging from slightly charred biomass (with some small unaltered fuel components remaining) through to heavily charred biomass reflecting more complete combustion. We demonstrate that the physical and chemical properties of PyC varied significantly with increasing burn severity. Additionally, the experimental design enabled an analysis of the effects of combinations of differing burn conditions (i.e., temperature, duration) – an issue that has received limited investigation in previous PyC research. The findings indicate that, within the typical ranges of burn characteristics present in United Kingdom peatland vegetation, varying combined burn characteristics can result in the production of broadly comparable PyC materials.
There are a range of possible means by which to identify the physicochemical composition of PyC samples found in the field, with the methods used here complementing other analytical techniques. Such PyC field identification has the potential to provide greater insight into any subsequent PyC degradation as well as the effects of formation conditions (e.g., burn duration) on PyC composition.
Data availability statement
The raw data supporting the conclusions of this article will be made available by the authors, without undue reservation.
Author contributions
OK-B: Conceptualization, Data curation, Formal Analysis, Investigation, Methodology, Writing–original draft, Writing–review and editing, Visualization. ES: Supervision, Writing–review and editing, Conceptualization, Methodology. JR: Supervision, Writing–review and editing, Conceptualization, Methodology. GC: Supervision, Writing–review and editing, Conceptualization, Methodology.
Funding
The author(s) declare that financial support was received for the research, authorship, and/or publication of this article. This research was funded by a PhD scholarship awarded to O. Kennedy-Blundell by the School of Environment, Education and Development, University of Manchester.
Acknowledgments
We would like to thank the following people for help during fieldwork: Annie Kennedy-Blundell, Dael Sassoon, Henk Cornelissen, Adam Johnston, James Allard and Martin Kay. We thank the following people for help in the laboratory: John Waters for analysis of BET samples, Jon Yarwood for analysis of CHNO and TOC samples as well as general advice, Heath Bagshaw for advice on FTIR analyses, Tom Bishop and John Moore for general advice and help.
For the purpose of open access, the author has applied a Creative Commons Attribution (CC BY) licence to any Author Accepted Manuscript version arising from this submission.
Conflict of interest
The authors declare that the research was conducted in the absence of any commercial or financial relationships that could be construed as a potential conflict of interest.
Publisher’s note
All claims expressed in this article are solely those of the authors and do not necessarily represent those of their affiliated organizations, or those of the publisher, the editors and the reviewers. Any product that may be evaluated in this article, or claim that may be made by its manufacturer, is not guaranteed or endorsed by the publisher.
Supplementary material
The Supplementary Material for this article can be found online at: https://www.frontiersin.org/articles/10.3389/feart.2024.1492624/full#supplementary-material
References
Abney, R. B., Kuhn, T. J., Chow, A., Hockaday, W., Fogel, M. L., and Berhe, A. A. (2019). Pyrogenic carbon erosion after the Rim Fire, Yosemite National Park: the role of burn severity and slope. J. Geophys. Res-Biogeo. 124, 432–449. doi:10.1029/2018jg004787
Amiro, B. D., Cantin, A., Flannigan, M. D., and De Groot, W. J. (2009). Future emissions from Canadian boreal forest fires. Can. J. For. Res. 39, 383–395. doi:10.1139/x08-154
Artz, R. R., Chapman, S. J., Robertson, A. J., Potts, J. M., Laggoun-Défarge, F., Gogo, S., et al. (2008). FTIR spectroscopy can be used as a screening tool for organic matter quality in regenerating cutover peatlands. Soil. Biol. biochem. 40, 515–527. doi:10.1016/j.soilbio.2007.09.019
Bargmann, T., Heegaard, E., Hatteland, B. A., Chipperfield, J. D., and Grytnes, J. A. (2016). Species trait selection along a prescribed fire chronosequence. Insect. Conserv. diver. 9, 446–455. doi:10.1111/icad.12182
Belcher, C. M., New, S. L., Santín, C., Doerr, S. H., Dewhirst, R. A., Grosvenor, M. J., et al. (2018). What can charcoal reflectance tell us about energy release in wildfires and the properties of pyrogenic carbon? Front. Earth. Sci. 6, 169. doi:10.3389/feart.2018.00169
Bird, M. I., Wynn, J. G., Saiz, G., Wurster, C. M., and McBeath, A. (2015). The pyrogenic carbon cycle. Annu. Rev. Earth Planet. Sci. 43, 273–298. doi:10.1146/annurev-earth-060614-105038
Bodí, M. B., Mataix-Solera, J., Doerr, S. H., and Cerdà, A. (2011). The wettability of ash from burned vegetation and its relationship to Mediterranean plant species type, burn severity and total organic carbon content. Geoderma 160 (3-4), 599–607. doi:10.1016/j.geoderma.2010.11.009
Brewer, C. E., Chuang, V. J., Masiello, C. A., Gonnermann, H., Gao, X., Dugan, B., et al. (2014). New approaches to measuring biochar density and porosity. Biomass. Bioenerg. 66, 176–185. doi:10.1016/j.biombioe.2014.03.059
Cao, J., Xiao, G., Xu, X., Shen, D., and Jin, B. (2013). Study on carbonization of lignin by TG-FTIR and high-temperature carbonization reactor. Fuel. Process. Technol. 106, 41–47. doi:10.1016/j.fuproc.2012.06.016
Clay, G. D., and Worrall, F. (2011). Charcoal production in a UK moorland wildfire - how important is it? J. Environ. Manage. 92, 676–682. doi:10.1016/j.jenvman.2010.10.006
Clay, G. D., Worrall, F., and Aebischer, N. J. (2012). Does prescribed burning on peat soils influence DOC concentrations in soil and runoff waters? Results from a 10 year chronosequence. J. Hydrol. 448, 139–148. doi:10.1016/j.jhydrol.2012.04.048
Clay, G. D., Worrall, F., Clark, E., and Fraser, E. D. (2009). Hydrological responses to managed burning and grazing in an upland blanket bog. J. Hydrol. 376, 486–495. doi:10.1016/j.jhydrol.2009.07.055
Davies, G. M., Domènech, R., Gray, A., and Johnson, P. C. D. (2016). Vegetation structure and fire weather influence variation in burn severity and fuel consumption during peatland wildfires. Biogeosciences 13, 389–398. doi:10.5194/bg-13-389-2016
Davies, G. M., Gray, A., Rein, G., and Legg, C. J. (2013). Peat consumption and carbon loss due to smouldering wildfire in a temperate peatland. For. Ecol. Manag. 308, 169–177. doi:10.1016/j.foreco.2013.07.051
Davies, G. M., and Legg, C. J. (2011). Fuel moisture thresholds in the flammability of Calluna vulgaris. Fire. Technol. 47, 421–436. doi:10.1007/s10694-010-0162-0
DeCiucies, S., Whitman, T., Woolf, D., Enders, A., and Lehmann, J. (2018). Priming mechanisms with additions of pyrogenic organic matter to soil. Geochim. Cosmochim. Ac. 238, 329–342. doi:10.1016/j.gca.2018.07.004
de Lafontaine, G., and Asselin, H. (2011). Soil charcoal stability over the Holocene across boreal northeastern North America. Quat. Res. 76, 196–200. doi:10.1016/j.yqres.2011.06.006
Dooley, S. R., and Treseder, K. K. (2012). The effect of fire on microbial biomass: a meta-analysis of field studies. Biogeochemistry 109 (1-3), 49–61. doi:10.1007/s10533-011-9633-8
Douglas, D. J., Buchanan, G. M., Thompson, P., Amar, A., Fielding, D. A., Redpath, S. M., et al. (2015). Vegetation burning for game management in the UK uplands is increasing and overlaps spatially with soil carbon and protected areas. Biol. Conserv. 191, 243–250. doi:10.1016/j.biocon.2015.06.014
Ellis, C. J. (2008). Interactions between hydrology, burning and contrasting plant groups during the millennial-scale development of sub-montane wet heath. J. Veg. Sci. 19, 693–704. doi:10.3170/2008-8-18439
Forestry Commission England (2023). Wildfire Statistics for England: report to 2020-21. Bristol: Forestry Commission England, 96.
Gao, C., Cong, J., Sun, Y., Han, D., and Wang, G. (2022). Variability in pyrogenic carbon properties generated by different burning temperatures and peatland plant litters: implication for identifying fire intensity and fuel types. Int. J. Wildland. Fire. 31 (4), 395–408. doi:10.1071/wf21053
Gazzard, R., McMorrow, J., and Aylen, J. (2016). Wildfire policy and management in England: an evolving response from Fire and Rescue Services, forestry and cross-sector groups. Phil. Trans. R. Soc. B 371, 20150341. doi:10.1098/rstb.2015.0341
Gibson, C., Hatton, P. J., Bird, J. A., Nadelhoffer, K., Le Moine, J., and Filley, T. (2018). Tree taxa and pyrolysis temperature interact to control pyrogenic organic matter induced native soil organic carbon priming. Soil. Biol. biochem. 119, 174–183. doi:10.1016/j.soilbio.2018.01.022
Grau-Andrés, R., Gray, A., and Davies, G. M. (2017). Sphagnum abundance and photosynthetic capacity show rapid short-term recovery following managed burning. Plant. Ecol. divers. 10, 353–359. doi:10.1080/17550874.2017.1394394
Guo, Y., and Bustin, R. M. (1998). FTIR spectroscopy and reflectance of modern charcoals and fungal decayed woods: implications for studies of inertinite in coals. Int. J. Coal. Geol. 37, 29–53. doi:10.1016/s0166-5162(98)00019-6
Hammer, Ø., Harper, D. A., and Ryan, P. D. (2001). PAST: paleontological statistics software package for education and data analysis. Palaeontol. Electron. 4, 9.
Hammes, K., Smernik, R. J., Skjemstad, J. O., Herzog, A., Vogt, U. F., and Schmidt, M. W. (2006). Synthesis and characterisation of laboratory-charred grass straw (Oryza sativa) and chestnut wood (Castanea sativa) as reference materials for black carbon quantification. Org. Geochem. 37, 1629–1633. doi:10.1016/j.orggeochem.2006.07.003
Hammes, K., Smernik, R. J., Skjemstad, J. O., and Schmidt, M. W. (2008). Characterisation and evaluation of reference materials for black carbon analysis using elemental composition, colour, BET surface area and 13C NMR spectroscopy. Appl. Geochem. 23, 2113–2122. doi:10.1016/j.apgeochem.2008.04.023
Harper, A. R., Doerr, S. H., Santín, C., Froyd, C. A., and Sinnadurai, P. (2018). Prescribed fire and its impacts on ecosystem services in the UK. Sci. Total. Environ. 624, 691–703. doi:10.1016/j.scitotenv.2017.12.161
Heinemeyer, A., Asena, Q., Burn, W. L., and Jones, A. L. (2018). Peatland carbon stocks and burn history: blanket bog peat core evidence highlights charcoal impacts on peat physical properties and long-term carbon storage. Geo. Geog. Environ. 5, e00063. doi:10.1002/geo2.63
Hobbs, R. J., and Gimingham, C. H. (1984). Studies on fire in scottish heathland communities: I. Fire characteristics. J. Ecol. 83, 713–726. doi:10.2307/2260015
Hobbs, R. J., and Gimingham, C. H. (1987). Vegetation, fire and herbivire interactions in heathland. Adv. Ecol. Res. 16, 87–173. doi:10.1016/S0065-2504(08)60088-4
Hobley, E., Willgoose, G. R., Frisia, S., and Jacobsen, G. (2014). Vertical distribution of charcoal in a sandy soil: evidence from DRIFT spectra and field emission scanning electron microscopy. Eur. J. Soil Sci. 65 (5), 751–762. doi:10.1111/ejss.12171
Holden, J., Palmer, S. M., Johnston, K., Wearing, C., Irvine, B., and Brown, L. E. (2015). Impact of prescribed burning on blanket peat hydrology. Water. Resour. Res. 51, 6472–6484. doi:10.1002/2014wr016782
Holden, J., Wearing, C., Palmer, S., Jackson, B., Johnston, K., and Brown, L. E. (2014). Fire decreases near-surface hydraulic conductivity and macropore flow in blanket peat. Hydrol. Process. 28, 2868–2876. doi:10.1002/hyp.9875
Huang, X., and Rein, G. (2014). Smouldering combustion of peat in wildfires: inverse modelling of the drying and the thermal and oxidative decomposition kinetics. Combust. Flame. 161 (6), 1633–1644. doi:10.1016/j.combustflame.2013.12.013
Hudspith, V. A., and Belcher, C. M. (2017). Observations of the structural changes that occur during charcoalification: implications for identifying charcoal in the fossil record. Palaeontology 60, 503–510. doi:10.1111/pala.12304
Hudspith, V. A., Belcher, C. M., and Yearsley, J. M. (2014). Charring temperatures are driven by the fuel types burned in a peatland wildfire. Front. Plant. Sci. 5, 714. doi:10.3389/fpls.2014.00714
Hudspith, V. A., Hadden, R. M., Bartlett, A. I., and Belcher, C. M. (2018). Does fuel type influence the amount of charcoal produced in wildfires? Implications for the fossil record. Palaeontology 61, 159–171. doi:10.1111/pala.12341
Joint Nature Conservation Committee (2011). Towards an assessment of the state of UK Peatlands. JNCC Rep. 445, 1–82.
Keeley, J. E. (2009). Fire intensity, fire severity and burn severity: a brief review and suggested usage. Int. J. Wildland. Fire. 18, 116–126. doi:10.1071/wf07049
Keiluweit, M., Nico, P. S., Johnson, M. G., and Kleber, M. (2010). Dynamic molecular structure of plant biomass-derived black carbon (biochar). Environ. Sci. Technol. 44, 1247–1253. doi:10.1021/es9031419
Kennedy-Blundell, O. J., Shuttleworth, E. L., Rothwell, J. J., and Clay, G. D. (2023). Vegetation-derived pyrogenic carbon degradation and stabilisation in UK peatlands. Int. J. Wildland. Fire. 32 (8), 1187–1199. doi:10.1071/wf22166
Kettridge, N., Shuttleworth, E., Neris, J., Doerr, S., Satin, C., Belcher, C., et al. (2019). The impact of wildfire on contaminated moorland catchment water quality. Geophys. Res. Abstr. 21, EGU2019–7772.
Key, C. H., and Benson, N. C. (2006). “Landscape assessment (LA),” in FIREMON: fire effects monitoring and inventory system. Gen. Tech. Rep. RMRS-GTR-164-CD. Editors D. C. Lutes, R. E. Keane, J. F. Caratti, C. H. Key, N. C. Benson, S. Sutherlandet al. (Fort Collins, CO: US Department of Agriculture, Forest Service, Rocky Mountain Research Station). Available at: https://www.fs.usda.gov/treesearch/pubs/24066 (accessed on August 06, 2024).
Knicker, H. (2009). “Black carbon and thermally altered (pyrogenic) organic matter: chemical characteristics and the role in the environment,” in Biophysico-chemical processes involving natural nonliving organic matter in environmental systems. Editors N. Senesi, B. Xing, and P. M. Huang (New York: John Wiley and Sons Inc), 273–303.
Knicker, H., Almendros, G., González-Vila, F. J., Martín, F., and Lüdemann, H. D. (1996). 13C-and 15N-NMR spectroscopic examination of the transformation of organic nitrogen in plant biomass during thermal treatment. Soil. Biol. biochem. 28, 1053–1060. doi:10.1016/0038-0717(96)00078-8
Kuhlbusch, T. A. J., and Crutzen, P. J. (1995). Toward a global estimate of black carbon in residues of vegetation fires representing a sink of atmospheric CO2 and a source of O2. Glob. Biogeochem. Cy. 9 (4), 491–501. doi:10.1029/95gb02742
Lammers, K., Arbuckle-Keil, G., and Dighton, J. (2009). FT-IR study of the changes in carbohydrate chemistry of three New Jersey pine barrens leaf litters during simulated control burning. Soil. Biol. biochem. 41, 340–347. doi:10.1016/j.soilbio.2008.11.005
Liang, B., Lehmann, J., Sohi, S. P., Thies, J. E., O’Neill, B., Trujillo, L., et al. (2010). Black carbon affects the cycling of non-black carbon in soil. Org. Geochem. 41, 206–213. doi:10.1016/j.orggeochem.2009.09.007
Liland, K. H., Almøy, T., and Mevik, B. H. (2010). Optimal choice of baseline correction for multivariate calibration of spectra. Appl. Spectrosc. 64 (9), 1007–1016. doi:10.1366/000370210792434350
Mackay, D. M., and Roberts, P. V. (1982). The influence of pyrolysis conditions on the subsequent gasification of lignocellulosic chars. Carbon 20, 105–111. doi:10.1016/0008-6223(82)90414-6
Maestrini, B., Nannipieri, P., and Abiven, S. (2015). A meta-analysis on pyrogenic organic matter induced priming effect. Gcb. Bioenergy. 7, 577–590. doi:10.1111/gcbb.12194
Marchessault, R. H. (1962). Application of infra-red spectroscopy to cellulose and wood polysaccharides. Pure. Appl. Chem. 5, 107–130. doi:10.1351/pac196205010107
Masiello, C. A. (2004). New directions in black carbon organic geochemistry. Mar. Chem. 92, 201–213. doi:10.1016/j.marchem.2004.06.043
McBeath, A. V., Smernik, R. J., Krull, E. S., and Lehmann, J. (2014). The influence of feedstock and production temperature on biochar carbon chemistry: a solid-state 13C NMR study. Biomass. Bioenerg. 60, 121–129. doi:10.1016/j.biombioe.2013.11.002
McBeath, A. V., Smernik, R. J., Schneider, M. P., Schmidt, M. W., and Plant, E. L. (2011). Determination of the aromaticity and the degree of aromatic condensation of a thermosequence of wood charcoal using NMR. Org. Geochem. 42, 1194–1202. doi:10.1016/j.orggeochem.2011.08.008
McMorrow, J. (2011). “Wildfire in the United Kingdom: status and key issues,” in Proceedings of the second conference on the human dimensions of wildland fire. Gen. Tech. Rep. NRS-P-84. Editors S. M. McCaffrey, and C. L. Fisher (Newtown Square, PA: U.S. Department of Agriculture, Forest Service), 44–56. Available at: https://research.manchester.ac.uk/en/publications/wildfire-in-the-united-kingdom-status-and-key-issues (Accessed on August 06, 2024).
McParland, L. C., Collinson, M. E., Scott, A. C., and Campbell, G. (2009). The use of reflectance values for the interpretation of natural and anthropogenic charcoal assemblages. Archaeol. Anthr. Sci. 1, 249–261. doi:10.1007/s12520-009-0018-z
Meyer, N., Welp, G., and Amelung, W. (2018). The temperature sensitivity (Q10) of soil respiration: controlling factors and spatial prediction at regional scale based on environmental soil classes. Glob. Biogeochem. Cy. 32, 306–323. doi:10.1002/2017gb005644
Milligan, G., Rose, R. J., O'Reilly, J., and Marrs, R. H. (2018). Effects of rotational prescribed burning and sheep grazing on moorland plant communities: results from a 60-year intervention experiment. Land. Degrad. Dev. 29, 1397–1412. doi:10.1002/ldr.2953
Nilsen, L. S., Johansen, L., and Velle, L. G. (2005). Early stages of Calluna vulgaris regeneration after burning of coastal heath in central Norway. Appl. Veg. Sci. 8, 57–64. doi:10.1658/1402-2001(2005)008[0057:esocvr]2.0.co;2
Noble, A., Crowle, A., Glaves, D. J., Palmer, S. M., and Holden, J. (2019). Fire temperatures and Sphagnum damage during prescribed burning on peatlands. Ecol. Indic. 103, 471–478. doi:10.1016/j.ecolind.2019.04.044
Noble, A., Palmer, S. M., Glaves, D. J., Crowle, A., Brown, L. E., and Holden, J. (2018). Prescribed burning, atmospheric pollution and grazing effects on peatland vegetation composition. J. Appl. Ecol. 55, 559–569. doi:10.1111/1365-2664.12994
Nocentini, C., Certini, G., Knicker, H., Francioso, O., and Rumpel, C. (2010). Nature and reactivity of charcoal produced and added to soil during wildfire are particle-size dependent. Org. Geochem. 41, 682–689. doi:10.1016/j.orggeochem.2010.03.010
Office for National Statistics (2019). UK natural capital: peatlands. London: Office for National Statistics.
Page, S. E., Siegert, F., Rieley, J. O., Boehm, H. D. V., Jaya, A., and Limin, S. (2002). The amount of carbon released from peat and forest fires in Indonesia during 1997. Nature 420, 61–65. doi:10.1038/nature01131
Preston, C. M., and Schmidt, M. W. (2006). Black (pyrogenic) carbon: a synthesis of current knowledge and uncertainties with special consideration of boreal regions. Biogeosciences 3, 397–420. doi:10.5194/bg-3-397-2006
Pyle, L. A., Hockaday, W. C., Boutton, T., Zygourakis, K., Kinney, T. J., and Masiello, C. A. (2015). Chemical and isotopic thresholds in charring: implications for the interpretation of charcoal mass and isotopic data. Environ. Sci. Technol. 49, 14057–14064. doi:10.1021/acs.est.5b03087
Ramchunder, S. J., Brown, L. E., and Holden, J. (2009). Environmental effects of drainage, drain-blocking and prescribed vegetation burning in UK upland peatlands. Prog. Phys. Geog. 33, 49–79. doi:10.1177/0309133309105245
R Core Team (2020). R: a language and environment for statistical computing. Vienna, Austria: R Foundation for Statistical Computing. Available at: https://www.R-project.org/.
Rothwell, J. J., Evans, M. G., Liddaman, L. C., and Allott, T. E. H. (2007). The role of wildfire and gully erosion in particulate Pb export from contaminated peatland catchments in the southern Pennines, UK. Geomorphology 88 (3-4), 276–284. doi:10.1016/j.geomorph.2006.11.011
Rytwo, G., Zakai, R., and Wicklein, B. (2015). The use of ATR-FTIR spectroscopy for quantification of adsorbed compounds. J. Spectrosc. 2015, 1–8. doi:10.1155/2015/727595
Santana, V. M., and Marrs, R. H. (2014). Flammability properties of British heathland and moorland vegetation: models for predicting fire ignition. J. Environ. Manage. 139, 88–96. doi:10.1016/j.jenvman.2014.02.027
Santín, C., Doerr, S. H., Kane, E. S., Masiello, C. A., Ohlson, M., de la Rosa, J. M., et al. (2016). Towards a global assessment of pyrogenic carbon from vegetation fires. Glob. Change. Biol. 22, 76–91. doi:10.1111/gcb.12985
Santos, F., Russell, D., and Berhe, A. A. (2016). Thermal alteration of water extractable organic matter in climosequence soils from the Sierra Nevada, California. J. Geophys. Res. Biogeosciences 121 (11), 2877–2885. doi:10.1002/2016jg003597
Saputro, S., Masykuri, M., Mahardiani, L., and Kurniastuti, D. (2018). The synthesis of corncobs (zea mays) active charcoal and water hyacinth (eichornia crassipes) adsorbent to adsorb Pb (II) with it’s analysis using solid-phase spectrophotometry (sps). IOP Conf. Ser. Mater. Sci. Eng. 333 (1), 012054. doi:10.1088/1757-899x/333/1/012054
Schneider, M. P., Hilf, M., Vogt, U. F., and Schmidt, M. W. (2010). The benzene polycarboxylic acid (BPCA) pattern of wood pyrolyzed between 200°C and 1000°C. Org. Geochem. 41, 1082–1088. doi:10.1016/j.orggeochem.2010.07.001
Sharma, R. K., Wooten, J. B., Baliga, V. L., Lin, X., Chan, W. G., and Hajaligol, M. R. (2004). Characterization of chars from pyrolysis of lignin. Fuel 83 (11-12), 1469–1482. doi:10.1016/j.fuel.2003.11.015
Shetler, G., Turetsky, M. R., Kane, E., and Kasischke, E. (2008). Sphagnum mosses limit total carbon consumption during fire in Alaskan black spruce forests. Can. J. For. Res. 38, 2328–2336. doi:10.1139/x08-057
Sigmund, G., Hüffer, T., Hofmann, T., and Kah, M. (2017). Biochar total surface area and total pore volume determined by N2 and CO2 physisorption are strongly influenced by degassing temperature. Sci. Total. Environ. 580, 770–775. doi:10.1016/j.scitotenv.2016.12.023
Smith, H. G., and Dragovich, D. (2008). Post-fire hillslope erosion response in a sub-alpine environment, south-eastern Australia. Catena 73, 274–285. doi:10.1016/j.catena.2007.11.003
Sun, H., and Zhou, Z. (2008). Impacts of charcoal characteristics on sorption of polycyclic aromatic hydrocarbons. Chemosphere 71 (11), 2113–2120. doi:10.1016/j.chemosphere.2008.01.016
Titirici, M. M., Thomas, A., and Antonietti, M. (2007). Back in the black: hydrothermal carbonization of plant material as an efficient chemical process to treat the CO2 problem? New. J. Chem. 31, 787–789. doi:10.1039/b616045j
Turetsky, M. R., Benscoter, B., Page, S., Rein, G., Van Der Werf, G. R., and Watts, A. (2015). Global vulnerability of peatlands to fire and carbon loss. Nat. Geosci. 8, 11–14. doi:10.1038/ngeo2325
Uhelski, D. M., Kane, E. S., Chimner, R. A., Heckman, K. A., Miesel, J., and Xie, L. (2022). Pyrogenic carbon content of Sphagnum peat soils estimated using diffuse reflectance FTIR spectrometry. Mires and Peat 28. doi:10.19189/MaP.2022.OMB.StA.2420
Vítek, P., Klem, K., and Urban, O. (2017). Application of Raman spectroscopy to analyse lignin/cellulose ratio in Norway spruce tree rings. Beskydy 10 (1-2), 41–48. doi:10.11118/beskyd201710010041
Wagner, S., Jaffé, R., and Stubbins, A. (2018). Dissolved black carbon in aquatic ecosystems. Limnol. Oceanogr. Lett. 3 (3), 168–185. doi:10.1002/lol2.10076
Wiedemeier, D. B., Abiven, S., Hockaday, W. C., Keiluweit, M., Kleber, M., Masiello, C. A., et al. (2015). Aromaticity and degree of aromatic condensation of char. Org. Geochem. 78, 135–143. doi:10.1016/j.orggeochem.2014.10.002
Wold, S., Esbensen, K., and Geladi, P. (1987). Principal component analysis. Chemom. Intell. Lab. 2, 37–52. doi:10.1016/0169-7439(87)80084-9
Worrall, F., Clay, G., Marrs, R., and Reed, M. S. (2010). Impacts of burning management on peatlands – Scientific review. UK Peatland Programme: IUCN.
Worrall, F., Clay, G. D., and May, R. (2013). Controls upon biomass losses and char production from prescribed burning on UK moorland. J. Environ. Manage. 120, 27–36. doi:10.1016/j.jenvman.2013.01.030
Worrall, F., Rowson, J. G., Evans, M. G., Pawson, R., Daniels, S., and Bonn, A. (2011). Carbon fluxes from eroding peatlands–the carbon benefit of revegetation following wildfire. Earth Surface Processes and Landforms (11) 36, 1487–1498.
Wurster, C. M., Saiz, G., Schneider, M. P., Schmidt, M. W., and Bird, M. I. (2013). Quantifying pyrogenic carbon from thermosequences of wood and grass using hydrogen pyrolysis. Org. Geochem. 62, 28–32. doi:10.1016/j.orggeochem.2013.06.009
Yang, H., Yan, R., Chen, H., Lee, D. H., and Zheng, C. (2007). Characteristics of hemicellulose, cellulose and lignin pyrolysis. Fuel 86 (12-13), 1781–1788. doi:10.1016/j.fuel.2006.12.013
Keywords: pyrogenic carbon, woody fuels, non-woody fuels, burn severity, aromaticity, surface area
Citation: Kennedy-Blundell OJ, Shuttleworth EL, Rothwell JJ and Clay GD (2025) Physical and chemical characteristics of pyrogenic carbon from peatland vegetation fires differ across burn severities. Front. Earth Sci. 12:1492624. doi: 10.3389/feart.2024.1492624
Received: 07 September 2024; Accepted: 03 December 2024;
Published: 06 January 2025.
Edited by:
Dong Liu, Chinese Academy of Sciences (CAS), ChinaReviewed by:
Kajar Köster, University of Eastern Finland, FinlandEvan S. Kane, Michigan Technological University, United States
Copyright © 2025 Kennedy-Blundell, Shuttleworth, Rothwell and Clay. This is an open-access article distributed under the terms of the Creative Commons Attribution License (CC BY). The use, distribution or reproduction in other forums is permitted, provided the original author(s) and the copyright owner(s) are credited and that the original publication in this journal is cited, in accordance with accepted academic practice. No use, distribution or reproduction is permitted which does not comply with these terms.
*Correspondence: Oscar J. Kennedy-Blundell, by5qLmtlbm5lZHktYmx1bmRlbGxAZXhldGVyLmFjLnVr
†ORCID: Oscar J. Kennedy-Blundell, orcid.org/0000-0002-1661-028X; Emma L. Shuttleworth, orcid.org/0000-0003-0661-1366; James J. Rothwell, orcid.org/0000-0003-2667-5818; Gareth D. Clay, orcid.org/0000-0002-8477-2774
‡Present address: Oscar J. Kennedy-Blundell, Geography, University of Exeter, Exeter, United Kingdom