- 1 Heilongjiang Province Key Laboratory of Geographical Environment Monitoring and Spatial Information Service in Cold Regions, Harbin Normal University, Harbin, China
- 2 School of Geographical Sciences, Nanjing University of Information Science and Technology, Nanjing, China
- 3 Cryosphere Research Station on the Qinghai–Tibet Plateau, Key Laboratory of Cryospheric Science and Frozen Soil Engineering, Northwest Institute of Eco-Environment and Resources, Chinese Academy of Sciences, Lanzhou, China
Permafrost degradation may have significant impacts on regional water cycles, while little is known about the recharge sources of runoff in permafrost regions, hindering our capability to predict river discharges. Here, a small permafrost basin in Northeast China was selected as the study area. We analyzed isotopic tracers of 186 precipitation, river water, and supra-permafrost water samples collected from May to October 2021. We further calculated the contribution of supra-permafrost water and precipitation to river discharges. The δ18O and δD of precipitation exhibited a significant correlation with air temperature (p<0.05). Similar values and trends were observed in the stable isotope changes of river water and supra-permafrost water, indicating a close hydraulic relationship between the two water sources. Hydrograph separation revealed that supra-permafrost water and precipitation are the first and second end-member of river water in the basin, with a contribution of 85% and 15%, respectively. Overall, our results suggest that supra-permafrost water is the main recharge source of runoff, highlighting the importance of permafrost in the regulation of runoff in small catchments.
1 Introduction
Over the past few years, permafrost degradation has become a common phenomenon in the permafrost regions due to the rapid global warming. Permafrost degradation caused by climate warming, including deepening of the active layer and melting of ground ice, can lead to changes in the surface thermal regime (Wu and Zhang, 2008; Wu and Zhang, 2010; Piao et al., 2019). Permafrost regions experience unique hydrological processes (Chai et al., 2023) because permafrost is a weak permeable layer that can prevent downward infiltration of water. In addition, melted ground ice can replenish surface water, and thus change in the form and amount of surface water recharge (Cheng and Jin, 2013). The groundwater systems in permafrost regions are composed of supra-permafrost, sub-permafrost and intra-permafrost water, which occur above the permafrost, below the permafrost, and within taliks, respectively (Kane et al., 2013). Supra-permafrost water in the active layer generally exhibits seasonality as the layer freezes and melts (Chang et al., 2018). Precipitation infiltrates the active layer and mixes with supra-permafrost water before flowing into rivers (Li Z. X. et al., 2020). The permafrost region of northern Greater Khingan Mountains is located near the southern limit of the Eurasian permafrost, where climate warming and permafrost degradation will inevitably have a significant impact on the hydrological processes in the region. However, due to the challenging field conditions and limited observations, the quantification of runoff sources of small permafrost basins in northern Greater Khingan Mountains is still absent and the understanding of local hydrological processes is still limited.
Environmental isotopes are important for studying the relationship among precipitation, groundwater, and surface water within the water cycle (Chen and Wang, 2009; Bolduc et al., 2018). δ18O and δD are key isotopes of natural water. Evaporation and condensation in the water cycle process can lead to isotope fractionation. The water from different sources exhibits distinct stable isotopic composition characteristics of hydrogen and oxygen. As natural tracers, δ18O and δD can provide valuable information on the water source, the processes controlling stream water dynamics, and its flow paths. Deuterium excess (D-excess) can provide valuable information about atmospheric humidity, surface temperature, and water evaporation (Gat et al., 2003; Vodila et al., 2011).
In recent decades, stable isotopes of precipitation (Yao et al., 2013; Ren et al., 2021), river water (Ala-aho et al., 2018), groundwater (Adomako et al., 2010), lake water (Pang et al., 2007) and snowmelt water (Jin et al., 2012; Miller et al., 2021) have been extensively studied. Hydrogen and oxygen stable isotope tracers have been widely employed to study the recharge sources of different water bodies in the water cycle of various regions (Sugimoto et al., 2003; Carey et al., 2013), as well as to explore the mutual transformation relationship between surface water and groundwater (Hren et al., 2009; Boucher and Carey, 2010; Turner et al., 2014). Sun et al. (2016) pointed out that precipitation serves as the primary source of runoff in inland river basins. Li Z. X. et al. (2019) utilized stable isotope tracing to reveal that the surface water and groundwater in the Qilian Mountains undergo distinct transformation patterns during different periods of the year, shedding light on their dynamic hydrological processes. Langman et al. (2022) found that winter meltwater (an early signal of depletion) may be a primary contributor to creeks and groundwater along the Palouse Mountains. Furthermore, stable isotope tracing has also made notable advancements in the study of biogeochemical cycles (Mul et al., 2008; Wu et al., 2009) and the determination of stream water residence times (Wang et al., 2022). In addition, numerous studies have demonstrated that permafrost degradation results in decreased or vanished soil impermeability, consequently intensifying the dynamic connectivity between surface water and groundwater under warming climatic conditions (Liu et al., 2003; Ye et al., 2003). These studies also confirmed the importance of supra-permafrost for surface river water in the permafrost regions (Li Z. X. et al., 2020; Gui et al., 2024). However, quantifying the contribution of supra-permafrost water to surface runoff in permafrost basins is largely unknown. Mountainous permafrost regions are more sensitive to climate change than other regions (Dedieu et al., 2014), and runoff changes in mountain areas can lead to floods or droughts in the downstream regions. Therefore, it is necessary to understand the relevant hydrological processes in order to provide references for the scientific use and effective prediction of water resources.
The permafrost region of the northern Greater Khingan Mountains is located in the southern margin of the Eurasian permafrost region. The permafrost in this region is sensitive to climate change. In this study, we explore the temporal variation of isotopes in various water sources, conduct hydrograph separation analysis of runoff, and identify their contributions to the river. The specific aims are to: (a) document the temporal characteristics of δ18O and δD in various water bodies; (b) reveal the hydrological processes influenced by the stable isotopes; and (c) determine the contribution of each water source to runoff. The results can reveal the replenishment mechanism of runoff in cold regions and provide parameters for simulating and predicting runoff changes.
2 Data and methodology
2.1 Study area
The permafrost region in the north of the Greater Khingan Mountains is situated on the southern margin of the Eurasian permafrost region (Ma et al., 2021). It is predominantly characterized by the presence of discontinuous permafrost, sporadic permafrost, and isolated permafrost. This region features a complex network of water systems and numerous small river basins. The Laoyeling basin, as a small permafrost basin within China, covers an area of 22.22 km2. It is located in Mohe City, the highest latitude city in China and in the northern Greater Khingan Mountains (Figure 1). The main stream melts completely in late April to early May and freezes in late October to early November, while the tributaries appear intermittently. The water level of the main stream varies between 19.96 cm and 57.58 cm from May to October 2021, with the highest value appearing in June. Influenced by the continental monsoon climate of the cold temperate zone, winters are cold and prolonged, summers are cool and brief, and autumn arrives swiftly. The average annual temperature in the region ranges from −2.5°C–5.0°C, with 80–110 frost-free days per year (Zheng, 1980). Annual precipitation ranges from 350 to 500 mm, primarily concentrated between July and August. There is snowpack in the basin from November to April of the following year, with the deepest snowpack reaching about 40 cm in mid-March. The snowpack melting starts in mid-March and ends in mid-to-late April, lasting about 1 month. During melting periods, precipitation and supra-permafrost water provide the water sources. The active layer thickness in this region varies from 1.5 to 4.5 m (Duan et al., 2017). The soil is rich in gravels and exhibits high permeability. Larix gmelinii, Betula platyphylla, and Pinus sylvestris var. mongolica are the key forest species (Ma et al., 2021), while arbor and herbaceous vegetation in marshes line the river, along with a small area of meadow vegetation.
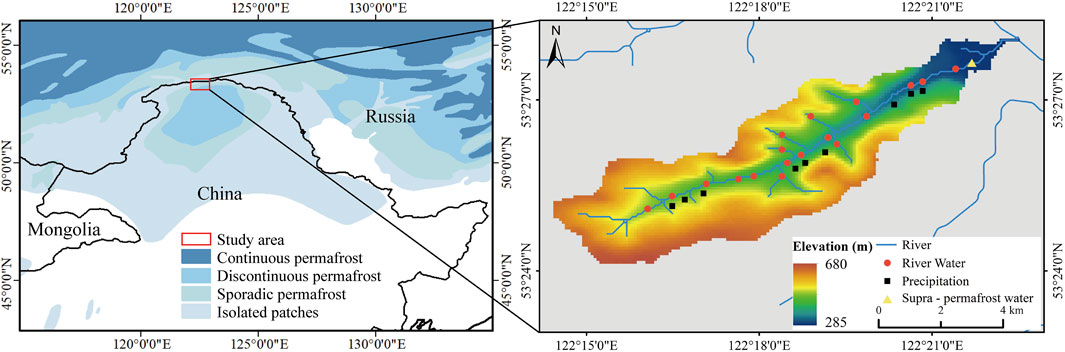
Figure 1. The location of the Laoyeling river basin in the northern Greater Khingan Mountains and the sampling sites.
2.2 Sampling and laboratory analysis
Precipitation samples were collected at the Laoyeling Hydrological Station (Figure 1) during precipitation events, resulting in a total of 49 samples from May to October 2021 (Table 1). Sample collectors were installed on the roof approximately 8 m above the ground, with an additional 1 m above the roof for protection against surface soil and other potential contaminants. Following each precipitation event, samples were carefully collected into pre-cleaned polyethylene bottles and sealed with Parafilm to prevent evaporation. Collection devices such as funnels, flecks, and polyethylene bottles were cleaned before each sampling event. All samples were stored in a refrigerator at 4°C. Meteorological data were obtained from the Mohe Forest Ecological Research Station, situated approximately 300 m above sea level and 200 m away from the study area (Figure 1).
A total of 83 water samples were collected along the Laoyeling River from May 2021 to October 2021 for the river water (Table 1). Monthly sampling was conducted at 12 sampling points in the mainstream, and six sampling sites in intermittently existing tributaries, which disappeared after July due to precipitation and snow meltwater effects (Figure 1).
Supra-permafrost water is widely distributed as a type of groundwater in the northern permafrost area of the Greater Khingan Mountains. It is mainly stored in the active layer above the permafrost. Affected by the melting of permafrost and the freezing-thawing cycles of seasonal frozen soil, the supra-permafrost water has complex and variable hydrological characteristics (Li Z. X. et al., 2020). The aquifer primarily consists of quaternary loose sediments and weathered fractures of bedrock in the study area. Permafrost water not only stores substantial solid water resources, but also plays an important role in maintaining the water levels of surface water and groundwater (Cheng and Jin, 2013). To investigate the characteristics of water on the permafrost, a total of 54 samples were collected monthly from May to October 2021. In order to have a more scientific and comprehensive understanding of the characteristics of supra-permafrost water in the basin, three sampling points were established in the upstream, midstream, and downstream respectively (Figure 1; Table 1). A 1.5-m deep profile of the permafrost active layer was excavated at each sampling site, and water samples were then collected after being filtered through a 0.45-µm Millipore filtration membrane at the bottom of the profile.
The analysis work was conducted at the Heilongjiang Provincial Key Laboratory of Geographical Environment Monitoring and Spatial Information Services in Cold Regions, Harbin Normal University. Prior to testing, all water samples were treated with a disposable membrane filter of 0.22 μm. Stable isotopes of hydrogen and oxygen were analyzed using a stable isotope ratio mass spectrometer (MAT253, United States). The results are reported in standard δ-notation (‰) relative to the Vienna Standard Mean Ocean Water, with precisions of ±2‰ for δD and ±0.1‰ for δ18O.
2.3 Hydrograph separation
End-member mixing analysis (EMMA) is commonly employed to analyze potential recharge sources of river water (Hooper et al., 1990; Gibson et al., 2015). It quantifies the contribution of different water sources to runoff based on the mass balance principle (Genereux, 1998; Uhlenbrook and Hoeg, 2003). The approach assumes that the chemical properties of stable isotopic tracers (δ18O, δD, etc.) and geochemical tracers (TDS, EC, Cl-, etc.) are constant, and any changes observed in the tracers are mainly caused by water transformation and mixing processes. In this study, δ18O was used as a tracer to separate water sources. The two-component method of isotopic hydrograph separation (IHS) described using Equations 1, 2 as follows:
where
where
3 Results
3.1 Stable isotopes composition
The δ18O value of precipitation ranged from −24.32‰ to −5.20‰, with an average of −11.63‰. The corresponding δD value ranged from −152.47‰ to −40.53‰, with an average of −92.37‰. The stable isotopes of precipitation in the Laoyeling basin exhibited clear time variation, initially increasing and subsequently decreasing with time (Figure 2). Stable isotopic composition of summer precipitation (June to August) ranged from −16.70‰ to −5.20‰ for δ18O and from −119.65‰ to −40.53‰ for δD, with mean values of −10.82‰ and −82.29‰ for δ18O and δD, which were greater than those obtained for the entire sampling period. The δ18O and δD values of precipitation in May, September, and October ranged from −24.32‰ to −9.28‰ and −188.01‰ to −78.07‰, with average values of −16.20‰ and −124.49‰, respectively. These values were lower than those obtained for the entire sampling period.
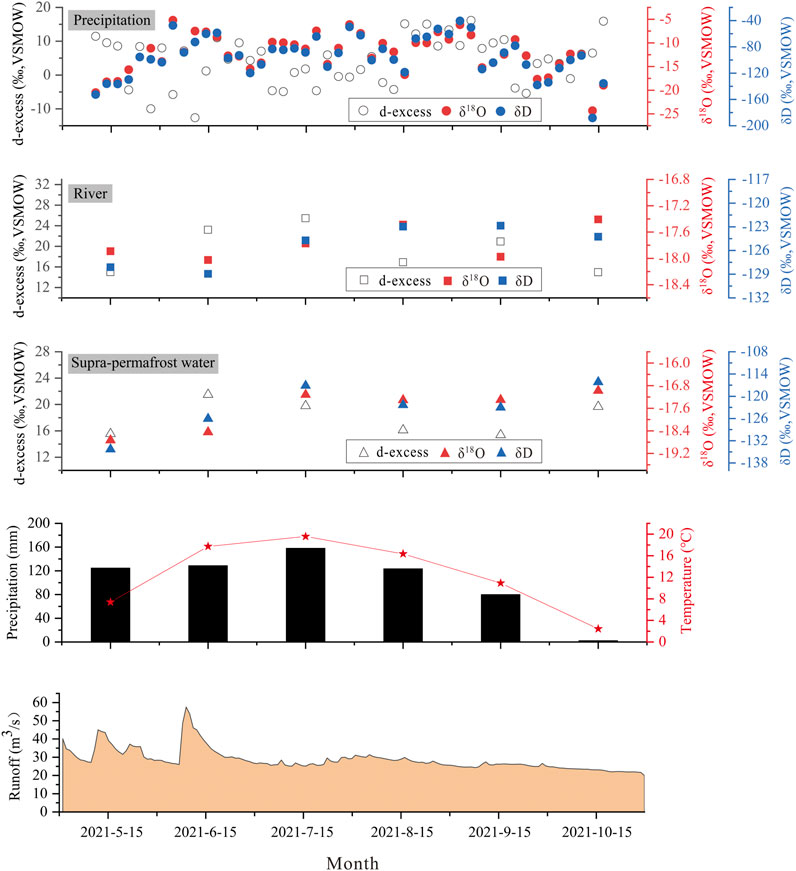
Figure 2. Stable isotopic composition of precipitation, river water, supra-permafrost water, air temperature, precipitation amount, and runoff depth in the Laoyeling basin.
The δ18O of river ranged from −19.01‰ to −15.59‰, with a mean value of −17.08‰. The δD ranged from −128.75‰ to −109.39‰, with a mean value of −118.00‰. The mean value of d-excess was 19.86‰, ranging from 6.64‰ to 29.43‰. Despite the large seasonal variation in precipitation isotopes, the stable isotopes of river water showed slight fluctuations during the study period, with more positive values in summer (June−August) and more negative values in May, September, and October. For the summer months, the stable isotopic values of river water ranged from −19.96‰ to −17.15‰ for δ18O and from −131.00‰ to −116.01‰ for δD, with mean values of −18.43 and −125.54‰, respectively. The depleted isotopic values were attributed to increased supra-permafrost water recharge to rivers. In May, September, and October, the δ18O and δD values ranged from −20.04‰ to −16.71‰ and from −139.19‰ to −113.71‰, with average values of −17.76‰ and −125.07‰, respectively. Although the average flow of river water is large in precipitation months, the change in stable isotopes is not obvious (Figure 2).
The δ18O of supra-permafrost water ranged from −20.64‰ to −16.96‰, with an average value of −18.53‰. The δD ranged from −146.66‰ to −114.43‰, with an average value of −21.44‰. The d-excess ranged from 8.76‰ to 29.16‰, with an average value of 21.44‰. The δ18O, δD, and d-excess values of supra-permafrost water exhibited slight temporal variation during the observation period, while greater fluctuations were observed for the corresponding values of precipitation and river water (Figure 2).
The deuterium excess (d-excess) is an important parameter for precipitation because it contains key information related to water vapor sources. The d-excess range of precipitation were determined as −12.62‰ to 16.15‰, with an average of 3.70‰. The temporal variation of the d-excess showed an inverse trend compared to that of δ18O, with low values in June to August and high values in May, September, and October (Figure 2). The d-excess values of precipitation varied widely in summer (June–August), ranging from −12.62‰ to 16.15‰, with an average value of 4.24‰. A narrow range was observed for the d-excess values of precipitation in May, September, and October, ranging from −5.39‰ to 15.92‰, with an average of 5.09‰. The d-excess values of both river water and supra-permafrost water showed slight fluctuations, similar to the patterns of their δ18O and δD values.
3.2 Meteorological effects
From May to October, a significant positive correlation was observed between the isotopic composition of precipitation and temperature in the study area (Figure 3A). When the temperature increased by 1°C, the isotope value of precipitation δ18O increased by 0.66‰ and δD by 4.96‰, indicating a significant temperature effect. This shows that sub-cloud evaporation significantly affects precipitation during the fall of raindrops. An increase in precipitation was accompanied by a significant rise in temperature and noticeable secondary evaporation occurring below cloud. As a result, the amount effect was not significant (Figure 3B). In addition, this may also be due to the mixing of raindrops with a certain amount of locally recirculated water vapor during their descent. Further analysis of the relationship between isotopic composition and precipitation in summer (June–August) showed that the relationship between δ18O and precipitation remained non-significant. These findings indicated that precipitation alone is not the key factor affecting its isotopic composition in this region.
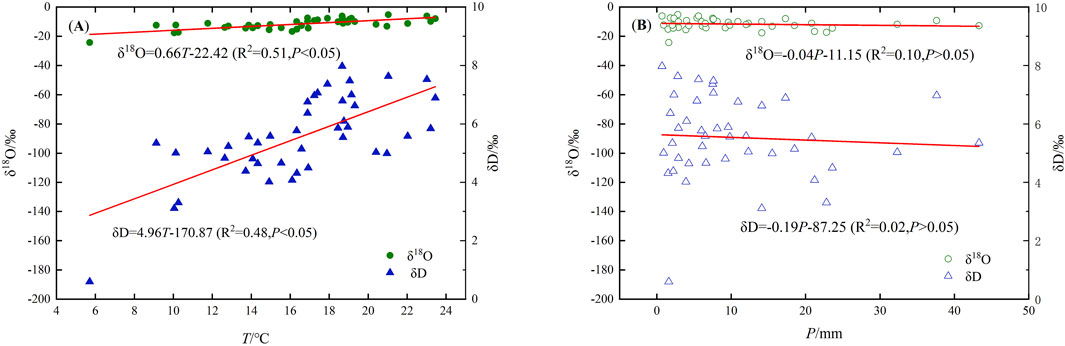
Figure 3. Relationship between the stable isotopes of precipitation and temperature (A), amount (B) in the Laoyeling basin.
The stable isotopes exhibited a gradual decrease during continuous precipitation. For example, during a sustained precipitation event from June 14 to June 15, δ18O and δD decreased from −7.66‰ and −60.07‰ to −8.72‰ and −64.74‰, respectively. Similarly, during a sustained precipitation event from July 22 to 23, δ18O and δD decreased from −6.11‰ and −48.28‰ to −7.97‰ and −62.14‰, respectively (Table 2). The results reveal that the amount effect was more significant during the continuous precipitation period. During the early stage of continuous precipitation, water vapor, enriched in the heavier isotopes δ18O and δD, preferentially condenses into raindrops, resulting in a gradual depletion of heavy isotopes in subsequent precipitation. Therefore, δ18O and δD in water vapor gradually deplete with the duration of precipitation events, leading to a decline in stable isotope concentration over time.
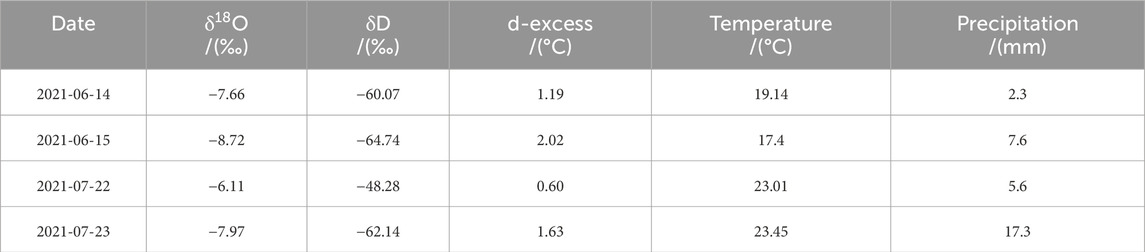
Table 2. Variation of isotopic composition and meteorological factors of continuous precipitation events.
3.3 Recharge sources of river water in the laoyeling basin
The distribution of river water and supra-permafrost water was observed to closely follow a similar distribution pattern, with river water falling between supra-permafrost water and precipitation (Figure 4). The isotopic composition of both river water and supra-permafrost water in the basin coincided with the local meteoric water line (LMWL), and the clustering of isotopic data from river water, precipitation, and supra-permafrost water demonstrated a close hydraulic connection in terms of recharge and discharge (Figure 5). A local evaporation line (LEL) for river water was established as δD = 3.12δ18O – 68.93 (R2 = 0.63). The slope of the LEL (3.12) was notably lower than that of the LMWL (7.37), indicating a stronger evaporation effect.
The stable isotope composition of precipitation in the Laoyeling basin exhibited noticeable temporal variation, characterized by an initial increase followed by a decrease. In contrast, the stable isotope composition of river water showed only slight temporal variation, suggesting that the direct influence of precipitation on river water was not pronounced during the study period. Correlation analysis indicated a positive but not significant correlation between precipitation and runoff depth during the sampling period (Table 3). From April to June and from August to October, a significant positive correlation was observed, indicating that precipitation was an important water source of runoff (Table 3). Notably, the correlation between precipitation and runoff depth from June to August was not significant (Table 3). This may be attributed to that vegetation intercepted and utilized a significant portion of precipitation, which then infiltrated underground to contribute to other water sources. Moreover, the maximum monthly mean value of runoff depth occurred from April to June, while the maximum monthly mean value of precipitation appeared from June to August. All the above indicated that precipitation was not the sole source of the runoff. The same seasonal patterns and the similar range of monthly mean δ18O values were observed between river water and supra-permafrost water (Figure 2). Moreover, most of the δD versus δ18O points of river water lay between the points of precipitation and supra-permafrost water (Figure 5). These indicated that the river water was mainly dominated and replenished by supra-permafrost water.
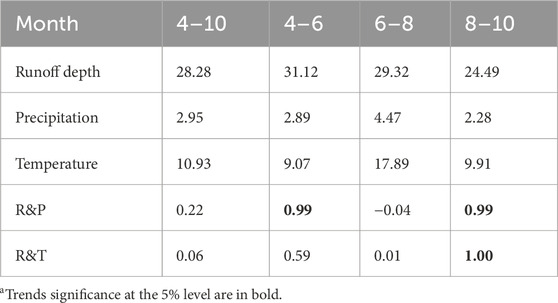
Table 3. Variation trend of monthly average runoff depth, precipitation, and temperature (°C or mm), the correlation coefficient between runoff depth and corresponding values of precipitation(R&P) and temperature (R&T).
These characteristics indicated that river water in the basin was recharged by two sources, namely supra-permafrost water and precipitation. The δ18O concentrations in river water, precipitation, and supra-permafrost water exhibited significant value variations within the basin. Thus, the δ18O concentration was selected for further analysis and to optimize the separation of water sources. The isotopic composition of river water lay along the line between supra-permafrost water and precipitation, indicating that both supra-permafrost water and precipitation served as recharge sources for river water. Supra-permafrost water was considered the first end-member, while precipitation served as the second end-member (Figure 6). The average δ18O values were determined as −11.63‰, −17.76‰, and −18.53‰ for precipitation, river water, and supra-permafrost water, respectively. The distinct δ18O values of each potential recharge source served as tracers for end-member mixing analysis (EMMA). According to the EMMA model, supra-permafrost water and precipitation accounted for 85% and 15% of the river water in the Laoyeling basin, respectively. This indicates that the primary source of runoff is supra-permafrost water.
4 Discussion
4.1 Assumptions and uncertainties of the EMMA model
The model uncertainty of EMMA is usually ignored in the hydrograph separation using stable isotope tracers (Genereux, 1998). It is necessary to make assumptions and explanations about the model before constructing it. In this study, the Laoyeling basin is regarded as a closed area. Precipitation is the input to the water cycle in the basin. Since there is no snowpack in the catchment during the sampling period, it is assumed that there is no snow recharge to runoff in the model. However, snowmelt water is one of the sources of supra-permafrost water in permafrost regions (Li et al., 2020a; Gui et al., 2024). Therefore, snowmelt water before the sampling period may have indirectly and delayed effect on surface runoff by recharging the supra-permafrost water in this study area. Permafrost degradation can significantly affect hydrological processes in winter (Niu et al., 2011). However, the Laoyeling river in this study is seasonal and does not exist in winter. Therefore, it is assumed that permafrost degradation does not recharge the river in the model. The groundwater in northeast China mainly contains three types: supra-permafrost water, sub-permafrost water, and river and lake talik water (Cheng and Jin, 2013). The river is not replenished by sub-permafrost water because it does not disappear in the winter if it is recharged by sub-permafrost water. Taliks usually exist under large rivers and lakes. However, the river in the study area is small, with a maximum runoff depth of only 57.58 cm. Therefore, it is assumed that the river is not recharged by river and lake talik water in the model. Moreover, the river’s existence coincides with the thawing period of the active layer above permafrost. Therefore, it is assumed that the river is recharged by supra-permafrost water in the model. Although there are some uncertainties for EMMA, it is still a valuable tool for quantitatively evaluating the contribution of different water bodies to runoff and improving our understanding of hydrological processes in permafrost regions.
4.2 Stable isotopes and their meteorological effects
Precipitation is an input to the terrestrial hydrological cycle, and understanding the isotopic characteristics of precipitation is very important for ecohydrological studies of wetlands (dos Santos et al., 2019; Tao et al., 2021). The stable isotopes of precipitation in the study area initially increased and subsequently decreased with time. This trend is attributed to evaporation. From June to August, the high temperatures significantly influenced precipitation, leading to secondary evaporation beneath clouds as raindrops fall. This contributed to the elevated δ18O values of precipitation. The d-excess values were closely related to the relative humidity of water vapor sources and the dynamic fractionation during evaporation (Dansgaard, 1964). The δ18O values of precipitation in the northeast China monsoon region were closely related to the advance and retreat of East Asian monsoon and the rainfall belt transfer (Liu et al., 2010). From June to August, the wet air mass not only brought a large amount of precipitation, but also led to a low d-excess. In May, September and October, air masses with low humidity, along with local water vapor recycling processes, resulted in higher d-excess values. Moreover, d-excess values were affected by the evaporation of water vapor (Aron et al., 2021). The higher temperatures from June to August led to strong evaporation, contributing to lower d-excess values. In contrast, the colder temperatures in May, September, and October, along with weaker evaporation, resulted in higher d-excess values. The meteoric water line contains a wealth of information, including the evaporation, transport, condensation, and precipitation of water vapor during the water cycle (Breitenbach et al., 2010). It not only reflects the changes in natural factors in the region but also holds significant importance for studying the variations of stable isotopes in atmospheric precipitation. The first local meteoric water line (LMWL) established for the Laoyeling basin was determined as δD = 7.37δ18O− 3.32, R2 = 0.95 (Figure 5). Compared with the meteoric water line in China, δD = 7.9δ18O+ 8.2 (Zheng et al., 1983) and the global meteoric water line (GMWL), δD = 8δ18O+ 10 (Craig, 1961), the local meteoric water line (LMWL) at Laoyeling exhibited a lower slope and intercept. The relatively low slope indicates the presence of significant secondary evaporation during the precipitation process in the basin.
The temporal variation of δ18O in river water and permafrost is not obvious. This is consistent with the temporal variation of river water and permafrost water in other areas where supra-permafrost water participates in river recharge (Gui et al., 2019; Li Z. X. et al., 2020). Previous study determined that river water with high d-excess values represents the isotopic signature of spring or summer precipitation, while a medium d-excess value indicates the recharge signal of supra-permafrost water or active layer water to river (Yang et al., 2019). The precipitation amount in July is the largest, and the d-excess of river also shows a high value (Figure 2), indicating that precipitation may play a more important role in the process of replenishing river water at this time. With the decrease of precipitation from August to October, the d-excess value of river water decreased compared with that from May to July, indicating that supra-permafrost water as a recharge source may have a greater impact on the composition of river water. The LEL of river water and supra-permafrost water were δD = 3.12δ18O – 68.93 (R2 = 0.63) and δD = 6.39δ18O- 10.40 (R2 = 0.771),respectively. The low slopes indicated that both of them were subject to strong evaporation, especially as the river is more sensitive to evaporation due to its narrow channel and small discharge. Theoretically, increased temperature would enhance evaporation, resulting in higher δ18O values in the water (Li Y. et al., 2023). However, we found that the highest δ18O value of the river water did not occur in July, the hottest month, but in August(Figure 2). The reason was that the highest precipitation occurred in July, counteracting the effect of evaporation on δ18O in the river water.
Temperature is a crucial factor influencing the isotopic composition of precipitation (Matthews et al., 2016). Near-surface temperature is linked to the condensation temperature of the corresponding air mass, impacting the stable isotope values in atmospheric precipitation. Rayleigh fractionation, driven by temperature, results in lower stable isotope concentrations in precipitation as temperature decreases. Under the influence of temperature, sub-cloud evaporation and water vapor cycles are vital factors affecting isotope evolution during precipitation (Li et al., 2015; Sun et al., 2015; Li Z. J. et al., 2019). The temperature effect on the stable isotopes of precipitation in the Laolaoling basin was found to be smaller than in inland areas of China (Wu and Zhang, 2010; Li et al., 2012; Zhu et al., 2015). This difference may be attributed to the wetter climate in the study area compared to inland areas. In addition, the sampling period in the study area took place from May to October, characterized by high temperatures and abundant precipitation. Stable isotope enrichment caused by intense sub-cloud evaporation was counteracted by depletion caused by water vapor recirculation. This result aligns with previous studies indicating that temperature is the primary factor controlling changes in the stable isotope composition of precipitation in middle and high latitudes, with the temperature effect becoming more pronounced further inland (Li et al., 2011).
The significant negative correlation between stable isotope concentration and precipitation, known as the amount effect, is observed in coastal areas of middle and low latitudes and regions influenced by monsoons. This effect is likely associated with intense convective weather (Dansgaard, 1964; Yapp and Epstein, 1982). The formation mechanism of the amount effect is complex and depends on three precipitation formation processes: evaporation conditions in the water vapor source area, the water vapor transport process, and the degree of condensation during precipitation. The study area has a temperate continental monsoon climate, characterized by the influence of the East Asian monsoon, westerlies, and local evaporation. The alternation of air masses from different sources, combined with temperature effects, leads to seasonal variations in stable isotopes of atmospheric precipitation.
4.3 Importance of supra-permafrost water
The supra-permafrost water plays a crucial role as a source of runoff in the cryosphere, which holds significant importance for local hydrological processes and ecosystems. In the three-river headwater region, known as “Chinese Water Tower,” supra-permafrost water accounts for 39% of river water (Li Z. X. et al., 2023). In the source regions of the Yangtze River, the river water contains 42.21% and 69.54% of supra-permafrost water in the glacier permafrost area and the permafrost area, respectively (Li et al., 2020b). Supra-permafrost water is the main water source of Tuotuo River on the Qinghai-Tibet Plateau, accounting for 51% (Li Z. X. et al., 2020).
Our results also showed that supra-permafrost water is the main source of river water in the mountains small river basin, but it is different from high mountainous arid inland river basins, where precipitation is the main source of rivers (Li et al., 2015; Li et al., 2016b; Gui et al., 2019). Water vapor can be lifted by the blocking effect of mountains to a high altitude where temperatures are low, forming local precipitation that replenishing rivers. However, the elevation of the mountains in the study area is relatively low, and thus the effect on the local water vapor lifting is not significant. In addition, precipitation may experience more soil infiltration and interception by vegetation roots during its downward flow along mountains and recharge the rivers due to the higher vegetation coverage and looser soil of the mountains in the study area. Besides supra-permafrost water, snowmelt water and glacier melt water are also important components of surface water supply in cold regions (Penna et al., 2014; Rai et al., 2019; Kong and Pang, 2012; Wang et al., 2015). However, our results are not consistent with those of the previous studies. This is due to the lack of perennial glaciers and snow cover in the permafrost area of the northern Greater Khingan Mountains during the sampling period of this study. Previous research identified subsurface water as the main source of rivers in Northeast China (Kendall, 1993). This agrees with the current study, which we identified supra-permafrost water as the main source of seasonal runoff in the permafrost region of the northern Greater Khingan Mountains. Dense vegetation and loose soil provide favorable conditions for the conversion of precipitation into subsurface water.
The groundwater in northeast China mainly contains three types: supra-permafrost water, sub-permafrost water, and river and lake talik water (Cheng and Jin, 2013). Interestingly, a previous study showed that Huma river in the permafrost region of northeast China was mainly recharged by sub-permafrost water, rather than supra-permafrost water (Ma et al., 2021). The river will not disappear in the winter if it is recharged by sub-permafrost water. Huma river is a perennial river, and runoff remains in the winter when the active layer freezes. This indicates that the river is recharged by sub-permafrost water. However, the Laoyeling river in the study area is seasonal, and its presence and disappearance coincide with the thawing and freezing of the active layer on the permafrost. Therefore, the river in the study area is not recharged by sub-permafrost water. River and lake talik water vary with the areal extent of surface-water bodies. It is more likely to be found under large rivers and lakes (Cheng and Jin, 2013). Since there is no lake and the river is very small in our study area, we speculate that river and lake talik water may not exist. Due to the significant difficulty of sampling, the isotope data on river and lake talik water is still unclear. We will continue to explore in the future.
4.4 Implications of supra-permafrost water on runoff
There has been a notable upward trend in global temperatures over the past 100 years (Pu et al., 2017). In the context of global warming, there has been a continuous acceleration in the degradation of permafrost, resulting in a gradual increase in permafrost thawing water. This situation has led to changes in the spatial and temporal distribution of water resources and water cycle, ultimately exerting a profound impact on the ecological environment. Permafrost acts as a hydrological barrier, impeding the lateral and vertical movement and recharge of groundwater. Previous studies have also confirmed the effect of permafrost degradation on runoff, with its degradation reducing soil impermeability and enhancing the connection between surface water and groundwater (Li et al., 2016a; Li et al., 2016b; Li et al., 2016c).
The Laoyeling basin is a typical permafrost area at middle and high latitudes, belonging to the Xing’an-Baikal permafrost in northeast China. Xing’an-Baikal permafrost is located at the southeast edge of the Eurasian permafrost zone, and its total permafrost area is about 2.57 × 105 km2 with the stable permafrost area (mean annual ground temperature ≤1.0°C) of 1.07 × 105 km2 (Wei et al., 2011). The supra-permafrost water accounts for 85% of runoff, indicating that supra-permafrost water is a vital resource in the Laoyeing basin. The majority of precipitation freezes underground or is used to convert into soil water, rather than contributing to surface runoff directly in permafrost regions (Li et al., 2009). The direct contribution of precipitation to runoff in the Laoyeling basin is relatively small, and it has an insignificant impact on the total annual runoff in the study area. Furthermore, the temperature in the northeastern permafrost region shows an increasing trend, and this trend is expected to continue in the future, which is consistent with the global trend of rising temperatures (Shan et al., 2023). The river water is influenced by both precipitation and temperature, with the latter showing a positive correlation to the amount of water released by the melting of ice above the permafrost in the northeastern permafrost region (Shan et al., 2023). Therefore, the freezing and thawing of the active layer play a crucial role in the hydrological processes within the study area.
In the context of global warming, there is increasing focus on permafrost degradation. In the past 20 years, climate change has caused the area of permafrost in northeastern China to shrink from 3.31 × 105 km2 to 2.70 × 105 km2, a degradation rate of 18.43%, and the ice stored in the permafrost is showing an increasingly rapid rate of release. The total ice volume in the permafrost of northeast China is 3.178 × 1011 m3, and it is projected to decrease to 6.641 × 1010 m3 after 100 years, representing a reduction of 2.514 × 1011 m3. The released water volume from permafrost degradation accounts for 79.11% of the current total ice volume, with a release rate of 2.51 × 109 m3/a (Shan et al., 2023). The distribution and recharge-discharge processes of groundwater in cold regions are significantly influenced by permafrost. Permafrost degradation alters soil connectivity, thereby influencing the interaction between surface water and groundwater (Li Z. X. et al., 2020). Therefore, the degradation of permafrost is expected to significantly impact the regional water cycle in the future. As the thickness of the active layer increases and the area of permafrost decreases, the contribution of water from the frozen soil layer to runoff is expected to initially increase and subsequently decrease as permafrost diminishes. Changes in hydrological processes will have complex impacts on the environment. Therefore, future research should pay more attention to the environmental response to permafrost degradation, which will provide more insights for environmental protection and economic development in the permafrost region of northeastern China.
5 Conclusion
In this study, we examined the different isotopes of precipitation, supra-permafrost water and river water in a small catchment during 2021. The results showed that the isotopes displayed distinct seasonal variations. The d-excess followed the opposite trend compared to that of δ18O. The relatively low slope of the local meteoric water line (LMWL) indicated pronounced evaporation during the descent of precipitation. A significant positive correlation was determined between precipitation isotopes and temperature. The relationship between precipitation isotopes and precipitation amount was not significant, possibly due to either the mixing of evaporated local water vapor with falling raindrops or strong secondary evaporation affecting raindrops. The isotopes suggested a close hydraulic connection among river water, precipitation, and supra-permafrost water. Compared to precipitation, isotopes in river water and supra-permafrost water exhibited smaller changes, with similar variation ranges and patterns. This implies that direct precipitation recharge to river water was not substantial. Instead, supra-permafrost water served as the primary recharge source. End-member mixture analysis identified supra-permafrost water and precipitation as the first and second end-members, contributing 85% and 15% to river water composition, respectively. In the context of global warming, permafrost is expected to continue to degrade, and this will have significant impacts on the water resource balance and ecological environment in northeastern China. Therefore, future research will focus on investigating the impact of permafrost melting due to climate warming on the water cycle mechanisms and the environmental impact of permafrost degradation, particularly over extended periods.
Data availability statement
The raw data supporting the conclusions of this article will be made available by the authors, without undue reservation.
Author contributions
BZ: Conceptualization, Data curation, Formal Analysis, Investigation, Writing–original draft, Writing–review and editing. SZ: Funding acquisition, Project administration, Resources, Supervision, Writing–review and editing. LZ: Conceptualization, Supervision, Writing–review and editing. XW: Supervision, Validation, Writing–review and editing. RL: Investigation, Writing–review and editing. XD: Investigation, Writing–review and editing. DY: Software, Writing–review and editing. XC: Writing–review and editing.
Funding
The author(s) declare that financial support was received for the research, authorship, and/or publication of this article. This study was supported by the National Natural Science Foundation of China (U20A2082), and the Science & Technology Fundamental Resources Investigation Program (2022FY100701), Natural Science Foundation of China (41941015, 32061143032).
Acknowledgments
We thank the Mohe Forest Ecological Research Station for supporting our fieldwork. Suggestions from reviewers for the improvement of our paper are also appreciated.
Conflict of interest
The authors declare that the research was conducted in the absence of any commercial or financial relationships that could be construed as a potential conflict of interest.
Publisher’s note
All claims expressed in this article are solely those of the authors and do not necessarily represent those of their affiliated organizations, or those of the publisher, the editors and the reviewers. Any product that may be evaluated in this article, or claim that may be made by its manufacturer, is not guaranteed or endorsed by the publisher.
References
Adomako, D., Maloszewski, P., Stumpp, C., Osae, S., and Akiti, T. T. (2010). Estimating groundwater recharge from water isotope (δ2H, δ18O) depth profiles in the Densu River basin, Ghana. Hydrological Sci. J. 55 (8), 1405–1416. doi:10.1080/02626667.2010.527847
Ala-aho, P., Soulsby, C., Pokrovsky, O. S., Kirpotin, S. N., Karlsson, J., Serikova, S., et al. (2018). Using stable isotopes to assess surface water source dynamics and hydrological connectivity in a high-latitude wetland and permafrost influenced landscape. J. Hydrology 556, 279–293. doi:10.1016/j.jhydrol.2017.11.024
Aron, P. G., Poulsen, C. J., Fiorella, R. P., Levin, N. E., Acosta, R. P., Yanites, B. J., et al. (2021). Variability and controls on δ18O, d-excess, and increment ′17O in southern Peruvian precipitation. J. Geophys. Research-Atmospheres 126 (23). doi:10.1029/2020jd034009
Bolduc, C., Lamoureux, S. F., and Franssen, J. (2018). Thermal and isotopic evidence for surface and subsurface water contributions to baseflow in a high Arctic river. Hydrol. Process. 32 (5), 602–616. doi:10.1002/hyp.11427
Boucher, J. L., and Carey, S. K. (2010). Exploring runoff processes using chemical, isotopic and hydrometric data in a discontinuous permafrost catchment. Hydrology Res. 41 (6), 508–519. doi:10.2166/nh.2010.146
Breitenbach, S. F. M., Adkins, J. F., Meyer, H., Marwan, N., Kumar, K. K., and Haug, G. H. (2010). Strong influence of water vapor source dynamics on stable isotopes in precipitation observed in Southern Meghalaya, NE India. Earth Planet. Sci. Lett. 292 (1-2), 212–220. doi:10.1016/j.epsl.2010.01.038
Carey, S. K., Boucher, J. L., and Duarte, C. M. (2013). Inferring groundwater contributions and pathways to streamflow during snowmelt over multiple years in a discontinuous permafrost subarctic environment (Yukon, Canada). Hydrogeology J. 21 (1), 67–77. doi:10.1007/s10040-012-0920-9
Chai, M. T., Luo, Y., Gao, Y., Ma, W., and Mu, Y. H. (2023). Seepage influence of supra-permafrost groundwater on thermal field of embankment on Qinghai-Tibet Plateau, China. Res. Cold Arid Regions 15 (3), 132–140. doi:10.1016/j.rcar.2023.06.004
Chang, J., Ye, R. Z., and Wang, G. X. (2018). Review: progress in permafrost hydrogeology in China. Hydrogeology J. 26 (5), 1387–1399. doi:10.1007/s10040-018-1802-6
Chen, J. S., and Wang, C. Y. (2009). Rising springs along the silk road. Geology 37 (3), 243–246. doi:10.1130/g25472a.1
Cheng, G. D., and Jin, H. J. (2013). Permafrost and groundwater on the Qinghai-Tibet Plateau and in northeast China. Hydrogeology J. 21 (1), 5–23. doi:10.1007/s10040-012-0927-2
Craig, H. (1961). Isotopic variations in meteoric waters. Science 133 (346), 1702–1703. doi:10.1126/science.133.3465.1702
Dansgaard, W. (1964). Stable isotopes in precipitation. Tellus 16 (4), 436–468. doi:10.1111/j.2153-3490.1964.tb00181.x
Dedieu, J. P., Lessard-Fontaine, A., Ravazzani, G., Cremonese, E., Shalpykova, G., and Beniston, M. (2014). Shifting mountain snow patterns in a changing climate from remote sensing retrieval. Sci. Total Environ. 493, 1267–1279. doi:10.1016/j.scitotenv.2014.04.078
dos Santos, V., Gastmans, D., Sánchez-Murillo, R., Gozzo, L. F., Batista, L. V., Manzione, R. L., et al. (2019). Regional atmospheric dynamics govern interannual and seasonal stable isotope composition in southeastern Brazil. J. Hydrology 579, 124136. doi:10.1016/j.jhydrol.2019.124136
Duan, L. L., Man, X. L., Kurylyk, B. L., and Cai, T. J. (2017). Increasing winter baseflow in response to permafrost thaw and precipitation regime shifts in northeastern China. Water 9 (1), 25. doi:10.3390/w9010025
Gat, J. R., Klein, B., Kushnir, Y., Roether, W., Wernli, H., Yam, R., et al. (2003). Isotope composition of air moisture over the Mediterranean Sea: an index of the air-sea interaction pattern. Tellus Ser. B-Chemical Phys. Meteorology 55 (5), 953–965. doi:10.1034/j.1600-0889.2003.00081.x
Genereux, D. (1998). Quantifying uncertainty in tracer-based hydrograph separations. Water Resour. Res. 34 (4), 915–919. doi:10.1029/98wr00010
Gibson, J. J., Birks, S. J., Yi, Y., and Vitt, D. H. (2015). Runoff to boreal lakes linked to land cover, watershed morphology and permafrost thaw: a 9-year isotope mass balance assessment. Hydrol. Process. 29 (18), 3848–3861. doi:10.1002/hyp.10502
Gui, J., Li, Z. J., Yuan, R. F., and Xue, J. (2019). Hydrograph separation and the influence from climate warming on runoff in the north-eastern Tibetan Plateau. Quat. Int. 525, 45–53. doi:10.1016/j.quaint.2019.09.002
Gui, J., Li, Z. X., Xue, J., Du, F., and Cui, Q. (2024). The effect of freeze-thaw action on the dynamic change of supra-permafrost water sources: a stable isotope perspective. J. Environ. Manag. 356, 120536. doi:10.1016/j.jenvman.2024.120536
Hooper, R. P., Christophersen, N., and Peters, N. E. (1990). Modelling streamwater chemistry as a mixture of soilwater end-members — An application to the Panola Mountain catchment, Georgia, U.S.A. J. Hydrology 116 (1-4), 321–343. doi:10.1016/0022-1694(90)90131-g
Hren, M. T., Bookhagen, B., Blisniuk, P. M., Booth, A. L., and Chamberlain, C. P. (2009). δ18O and δD of streamwaters across the Himalaya and Tibetan Plateau: Implications for moisture sources and paleoelevation reconstructions. Earth Planet. Sci. Lett. 288 (1-2), 20–32. doi:10.1016/j.epsl.2009.08.041
Jin, L., Siegel, D. I., Lautz, L. K., and Lu, Z. L. (2012). Identifying streamflow sources during spring snowmelt using water chemistry and isotopic composition in semi-arid mountain streams. J. Hydrology 470, 289–301. doi:10.1016/j.jhydrol.2012.09.009
Kane, D. L., Yoshikawa, K., and McNamara, J. P. (2013). Regional groundwater flow in an area mapped as continuous permafrost, NE Alaska (USA). Hydrogeology J. 21 (1), 41–52. doi:10.1007/s10040-012-0937-0
Kendall, C. (1993). Impact of Isotopic Heterogeneity in Shallow Systems on Modeling of Stormflow Generation.
Kong, Y. L., and Pang, Z. H. (2012). Evaluating the sensitivity of glacier rivers to climate change based on hydrograph separation of discharge. J. Hydrology 434, 121–129. doi:10.1016/j.jhydrol.2012.02.029
Langman, J. B., Martin, J., Gaddy, E., Boll, J., and Behrens, D. (2022). Snowpack Aging, Water Isotope Evolution, and Runoff Isotope Signals, Palouse Range, Idaho, USA. Hydrology 9 (6), 94. doi:10.3390/hydrology9060094
Li, T., Wang, G., Hu, H., Liu, G., Ren, D., and Wang, Y. (2009). Hydrological Process in a Typical Small Permafrost Watershed at the Headwaters of Yangtze River. J. Glaciol. Geocryol. 31 (1), 82–88.
Li, X. F., Zhang, M. J., Ma, Q., Li, Y. J., Wang, S. J., and B, L. W. (2012). Characteristics of Stable Isotopes in Precipitation over Northeast China and Its Water Vapor Sources. Environ. Sci. 33 (09), 2924–2931. doi:10.13227/j.hjkx.2012.09.009
Li, Y., Huo, S. Y., Guo, J., Sun, J., Pan, J., Wang, D., et al. (2023a). Using hydrogen and oxygen stable isotopes to estimate soil water evaporation loss under continuous evaporation conditions. Hydrol. Process. 37 (5). doi:10.1002/hyp.14885
Li, Y. J., Zhang, M. J., Wang, S. J., Li, Z. Q., and Wang, F. T. (2011). Progress of the Research of Stable Isotope in Precipitation in China: A Review. J. Glaciol. Geocryol. 33 (03), 624–633.
Li, Z. J., Li, Z. X., Fan, X. J., Wang, Y., Song, L. L., Gui, J., et al. (2020a). The sources of supra-permafrost water and its hydrological effect based on stable isotopes in the third pole region. Sci. Total Environ. 715, 136911. doi:10.1016/j.scitotenv.2020.136911
Li, Z. J., Li, Z. X., Song, L. L., Gui, J., Xue, J., Zhang, B. J., et al. (2020b). Hydrological and runoff formation processes based on isotope tracing during ablation period in the source regions of Yangtze River. Hydrology Earth Syst. Sci. 24 (8), 4169–4187. doi:10.5194/hess-24-4169-2020
Li, Z. J., Li, Z. X., Yu, H. C., Song, L. L., and Ma, J. Z. (2019a). Environmental significance and zonal characteristics of stable isotope of atmospheric precipitation in arid Central Asia. Atmos. Res. 227, 24–40. doi:10.1016/j.atmosres.2019.04.022
Li, Z. X., Feng, Q., Wang, Q. J., Yong, S., Cheng, A. F., and Li, J. G. (2016a). Contribution from frozen soil meltwater to runoff in an in-land river basin under water scarcity by isotopic tracing in northwestern China. Glob. Planet. Change 136, 41–51. doi:10.1016/j.gloplacha.2015.12.002
Li, Z. X., Gui, J., Feng, Q., Zhang, B. J., and Cui, Q. (2023b). Contribution of the cryosphere to runoff in “Chinese water tower” based on environmental isotopes. Geosci. Front. 14 (5), 101613. doi:10.1016/j.gsf.2023.101613
Li, Z. X., Gui, J., Wang, X. F., Feng, Q., Zhao, T. T. G., Ouyang, C. J., et al. (2019b). Water resources in inland regions of central Asia: Evidence from stable isotope tracing. J. Hydrology 570, 1–16. doi:10.1016/j.jhydrol.2019.01.003
Li, Z. X., Li, Z. J., Feng, Q., Zhang, B. J., Gui, J., Xue, J., et al. (2020c). Runoff dominated by supra-permafrost water in the source region of the Yangtze river using environmental isotopes. J. Hydrology 582, 124506. doi:10.1016/j.jhydrol.2019.124506
Li, Z. X., Qi, F., Li, J. G., Pan, Y. H., Wang, T. T., Li, L., et al. (2015). Environmental significance and hydrochemical processes at a cold alpine basin in the Qilian Mountains. Environ. Earth Sci. 73 (8), 4043–4052. doi:10.1007/s12665-014-3689-4
Li, Z. X., Qi, F., Wang, Q. J., Song, Y., Li, H. Y., and Li, Y. G. (2016b). The influence from the shrinking cryosphere and strengthening evopotranspiration on hydrologic process in a cold basin, Qilian Mountains. Glob. Planet. Change 144, 119–128. doi:10.1016/j.gloplacha.2016.06.017
Li, Z. X., Qi, F., Wang, Q. J., Song, Y., Li, J. G., Li, Y. G., et al. (2016c). Quantitative evaluation on the influence from cryosphere meltwater on runoff in an inland river basin of China. Glob. Planet. Change 143, 189–195. doi:10.1016/j.gloplacha.2016.06.005
Liu, J. R., Song, X. F., Yuan, G. F., Sun, X. M., Liu, X., and Wang, S. Q. (2010). Characteristics of δ18O in precipitation over Eastern Monsoon China and the water vapor sources. Chin. Sci. Bull. 55 (2), 200–211. doi:10.1007/s11434-009-0202-7
Liu, J. S., Hayakawa, N., Lu, M. J., Dong, S. H., and Yuan, J. Y. (2003). Hydrological and geocryological response of winter streamflow to climate warming in Northeast China. Cold Regions Sci. Technol. 37 (1), 15–24. doi:10.1016/s0165-232x(03)00012-0
Ma, F. Y., Chen, J. S., Zhan, L. C., Zhang, X., Yan, J. H., and Wang, W. F. (2021). New insights into water cycle in permafrost region of northern Greater Khingan Mountains, China. J. Radioanalytical Nucl. Chem. 330 (3), 631–642. doi:10.1007/s10967-021-08013-2
Matthews, C. J. D., Longstaffe, F. J., and Ferguson, S. H. (2016). Dentine oxygen isotopes (δ 18O) as a proxy for odontocete distributions and movements. Ecol. Evol. 6 (14), 4643–4653. doi:10.1002/ece3.2238
Miller, S. A., Mercer, J. J., Lyon, S. W., Williams, D. G., and Miller, S. N. (2021). Stable isotopes of water and specific conductance reveal complimentary information on streamflow generation in snowmelt-dominated, seasonally arid watersheds. J. Hydrology 596, 126075. doi:10.1016/j.jhydrol.2021.126075
Mul, M. L., Mutiibwa, R. K., Uhlenbrook, S., and Savenije, H. H. G. (2008). Hydrograph separation using hydrochemical tracers in the Makanya catchment, Tanzania. Phys. Chem. Earth 33 (1-2), 151–156. doi:10.1016/j.pce.2007.04.015
Niu, L., Ye, B. S., Li, J., and Sheng, Y. (2011). Effect of permafrost degradation on hydrological processes in typical basins with various permafrost coverage in Western China. Sci. China-Earth Sci. 54 (4), 615–624. doi:10.1007/s11430-010-4073-1
Pang, H., He, Y. Q., Theakstone, W. H., and Zhang, D. D. (2007). “Soluble ionic and oxygen isotopic compositions of a shallow firn profile, Baishui glacier No. 1, southeastern Tibetan Plateau,” in International Symposium on cryospheric Indicators of global climate change, 325–+.
Penna, D., Engel, M., Mao, L., Dell, A., Bertoldi, G., and Comiti, F. (2014). Tracer-based analysis of spatial and temporal variations of water sources in a glacierized catchment. Hydrology earth Syst. Sci. 11 (12), 5271–5288. doi:10.5194/hess-18-5271-2014
Piao, S. L., Zhang, X. Z., Wang, T., Liang, E. Y., Wang, S. P., Zhu, J. T., et al. (2019). Responses and feedback of the Tibetan Plateau's alpine ecosystem to climate change. Chin. Sci. Bulletin-Chinese 64 (27), 2842–2855. doi:10.1360/tb-2019-0074
Pu, T., Qin, D. H., Kang, S. C., Niu, H. W., He, Y. Q., and Wang, S. J. (2017). Water isotopes and hydrograph separation in different glacial catchments in the southeast margin of the Tibetan Plateau. Hydrol. Process. 31 (22), 3810–3826. doi:10.1002/hyp.11293
Rai, S. P., Singh, D., Jacob, N., Rawat, Y. S., and Arora, M.BhishmKumar (2019). Identifying contribution of snowmelt and glacier melt to the Bhagirathi River (Upper Ganga) near snout of the Gangotri Glacier using environmental isotopes. Catena 173, 339–351. doi:10.1016/j.catena.2018.10.031
Ren, W., Tian, L. D., and Shao, L. L. (2021). Regional moisture sources and Indian summer monsoon (ISM) moisture transport from simultaneous monitoring of precipitation isotopes on the southeastern and northeastern Tibetan Plateau. J. Hydrology 601, 126836. doi:10.1016/j.jhydrol.2021.126836
Shan, W., Wang, Y., Guo, Y., Zhang, C. C., Liu, S., and Qiu, L. S. (2023). Impacts of Climate Change on Permafrost and Hydrological Processes in Northeast China. Sustainability 15 (6), 4974. doi:10.3390/su15064974
Sugimoto, A., Naito, D., Yanagisawa, N., Ichiyanagi, K., Kurita, N., Kubota, J., et al. (2003). Characteristics of soil moisture in permafrost observed in East Siberian taiga with stable isotopes of water. Hydrol. Process. 17 (6), 1073–1092. doi:10.1002/hyp.1180
Sun, C. J., Chen, Y. N., Li, W. H., Li, X. G., and Yang, Y. H. (2016). Isotopic time series partitioning of streamflow components under regional climate change in the Urumqi River, northwest China. Hydrological Sci. J. 61 (8), 1443–1459. doi:10.1080/02626667.2015.1031757
Sun, C. J., Li, W. H., Chen, Y. N., Li, X. G., and Yang, Y. H. (2015). Isotopic and hydrochemical composition of runoff in the Urumqi River, Tianshan Mountains, China. Environ. Earth Sci. 74 (2), 1521–1537. doi:10.1007/s12665-015-4144-x
Tao, S. Y., Zhang, X., Pan, G. Y., Xu, J., and Zeng, Z. Y. (2021). Moisture source identification based on the seasonal isotope variation of precipitation in the Poyang Lake Wetland, China. J. Hydrology-Regional Stud. 37, 100892. doi:10.1016/j.ejrh.2021.100892
Turner, K. W., Edwards, T. W. D., and Wolfe, B. B. (2014). Short Communication Characterising Runoff Generation Processes in a Lake- Rich Thermokarst Landscape (Old Crow Flats, Yukon, Canada) using d18O, d2H and d-excess Measurements. Permafr. Periglac. Process. 25 (1), 53–59. doi:10.1002/ppp.1802
Uhlenbrook, S., and Hoeg, S. (2003). Quantifying uncertainties in tracer-based hydrograph separations: a case study for two-three- and five-component hydrograph separations in a mountainous catchment. Hydrol. Process. 17 (2), 431–453. doi:10.1002/hyp.1134
Vodila, G., Palcsu, L., Futó, I., and Szántó, Z. (2011). A 9-year record of stable isotope ratios of precipitation in Eastern Hungary: Implications on isotope hydrology and regional palaeoclimatology. J. Hydrology 400 (1-2), 144–153. doi:10.1016/j.jhydrol.2011.01.030
Wang, S. Y., He, X. B., Kang, S. C., Fu, H., and Hong, X. F. (2022). Estimation of stream water components and residence time in a permafrost catchment in the central Tibetan Plateau using long-term water stable isotopic data. Cryosphere 16 (12), 5023–5040. doi:10.5194/tc-16-5023-2022
Wang, X. Y., Li, Z. Q., Ross, E., Tayier, R., and Zhou, P. (2015). Characteristics of water isotopes and hydrograph separation during the spring flood period in Yushugou River basin, Eastern Tianshans, China. J. Earth Syst. Sci. 124 (1), 115–124. doi:10.1007/s12040-014-0517-x
Wei, Z., Jin, H. J., Zhang, J. M., Yu, S. P., Han, X. J., Ji, Y. J., et al. (2011). Prediction of permafrost changes in Northeastern China under a changing climate. Sci. China-Earth Sci. 54 (6), 924–935. doi:10.1007/s11430-010-4109-6
Wu, P., Tang, C. Y., Zhu, L. J., Liu, C. Q., Cha, X. F., and Tao, X. Z. (2009). Hydrogeochemical characteristics of surface water and groundwater in the karst basin, southwest China. Hydrol. Process. 23 (14), 2012–2022. doi:10.1002/hyp.7332
Wu, Q. B., and Zhang, T. J. (2008). Recent permafrost warming on the Qinghai-Tibetan plateau. J. Geophys. Research-Atmospheres 113 (D13), 22. doi:10.1029/2007jd009539
Wu, Q. B., and Zhang, T. J. (2010). Changes in active layer thickness over the Qinghai-Tibetan Plateau from 1995 to 2007. J. Geophys. Research-Atmospheres 115, 12. doi:10.1029/2009jd012974
Yang, Y. Z., Wu, Q. B., Jin, H. J., Wang, Q. F., Huang, Y. D., Luo, D. L., et al. (2019). Delineating the hydrological processes and hydraulic connectivities under permafrost degradation on Northeastern Qinghai-Tibet Plateau, China. J. Hydrology 569, 359–372. doi:10.1016/j.jhydrol.2018.11.068
Yao, T. D., Masson-Delmotte, V., Gao, J., Yu, W. S., Yang, X. X., Risi, C., et al. (2013). A review of climatic controls on δ18o in precipitation over the tibetan plateau: observations and simulations. Rev. Geophys. 51 (4), 525–548. doi:10.1002/rog.20023
Yapp, C. J., and Epstein, S. (1982). Climatic significance of the hydrogen isotope ratios in tree cellulose. Nature 297 (5868), 636–639. doi:10.1038/297636a0
Ye, B. S., Yang, D. Q., and Kane, D. L. (2003). Changes in Lena River streamflow hydrology: Human impacts versus natural variations. Water Resour. Res. 39 (7). doi:10.1029/2003wr001991
Zheng, Q. P. (1980). Hydrogeological charicteristics of permafrost and cold regions in Dahinganling. J. Glaciol. Geocryol.
Zheng, S. H., Hou, F. G., and Ni, B. L. (1983). Studies on hydrogen and oxygen stable isotopes of atmospheric precipitation in China. Chin. Sci. Bull. 28 (13), 801–806. doi:10.1360/csb1983-28-13-801
Keywords: stable isotopes, runoff, hydrograph separation, permafrost, greater Khingan mountains
Citation: Zhang B, Zang S, Zhao L, Wu X, Liu R, Dong X, Yang D and Cheng X (2024) Characteristics of water stable isotopes and runoff sources of a small permafrost basin in northeastern China. Front. Earth Sci. 12:1476783. doi: 10.3389/feart.2024.1476783
Received: 06 August 2024; Accepted: 05 December 2024;
Published: 18 December 2024.
Edited by:
Fengjing Liu, Michigan Technological University, United StatesReviewed by:
Wei Shan, Northeast Forestry University, ChinaGopal Krishan, National Institute of Hydrology (Roorkee), India
Copyright © 2024 Zhang, Zang, Zhao, Wu, Liu, Dong, Yang and Cheng. This is an open-access article distributed under the terms of the Creative Commons Attribution License (CC BY). The use, distribution or reproduction in other forums is permitted, provided the original author(s) and the copyright owner(s) are credited and that the original publication in this journal is cited, in accordance with accepted academic practice. No use, distribution or reproduction is permitted which does not comply with these terms.
*Correspondence: Shuying Zang, enN5NjMxMUBocmJudS5lZHUuY24=