- 1College of Civil and Architectural Engineering, Guangxi University of Science and Technology, Liuzhou, China
- 2Guangxi Zhuang Autonomous Region Engineering Research Center of Geotechnical Disaster and Ecological Control, Guangxi University of Science and Technology, Liuzhou, China
- 3Guangxi Zhuang Autonomous Region Engineering Research Center of Assembly Structure Safety Prevention and Control, Guangxi University of Science and Technology, Liuzhou, China
- 4Guangzhou Institute of Building Science Group Co., Ltd., Guangzhou, China
- 5Guangzhou Jianyan Engineering Technology Co., Ltd., Guangzhou, China
Soft rock undergoes internal structural redistribution and random damage under the action of dry–wet cycles, with these processes ultimately affecting its mechanical properties. In order to analyze the evolution mechanism of mineral composition inside soft rocks, an effective method for the characterization of the nonlinear damage of soft rock using a multifractal spectrum is presented. Moreover, a cross-scale correlation model of internal structural changes and strength degradation is established. Based on scanning electron microscopy (SEM) images of soft rock subjected to a varying number of dry–wet cycles, the damage propagation path was tracked via a rock-like compression failure test. The study results indicate that soft rock exhibits a random fractal damage effect under the action of dry–wet cycles. As the number of cycles increases, the multifractal spectrum becomes more asymmetric and the discretization degree becomes more uneven. The soft rock exhibits cross-scale evolution characteristics from mesostructural to macroscopic damage after encountering water. After a series of reactions between water and soft rock, the bonding between particles weakens and recombines, ultimately affecting the mechanical properties of the soft rock. The research results have enriched the research framework of soft rock failure mechanisms, and provided an effective method for quantitatively characterizing the correlation analysis between soft rock damage and damage at different scales.
1 Introduction
In many areas with soft rock formations, the natural environment and groundwater have a continuous impact on the soft rock, causing varying degrees of damage from dry–wet cycles. This damage often leads to recurrent engineering accidents and significant economic losses. The dynamic softening process mechanism of soft rock is shown in Figure 1. The mechanical properties of soft rock are maintained through effective bonding between particles within a porous medium. The compressive strength of soft rock is determined by the mineral composition and bonding strength of particles. Understanding water–rock interaction under dry–wet cycle conditions is a fundamental issue in collaborative research across different fields in academia and engineering. Research findings in this field hold significant practical value for application in engineering practice (Kaniz et al., 2023; Wagdi and Ahmed, 2023; Li W. et al., 2022; Lin et al., 2024). Revealing the failure mechanism of soft rock under dry–wet cycles and achieving the quantitative characterization of soft rock damage can offer practical and feasible solutions for controlling engineering disasters.
At present, research on the mechanism of the dry–wet cycle between soft rock and water mainly focuses on achieving relevant results at different scales. Regarding the interaction between soft rock and water, the changes in ion concentration and acid–base properties in aqueous solutions are primarily measured to determine the alterations in the mineral composition of the soft rock itself and further validate its mechanical properties (Ali and Davood, 2020; Krzysztof and Radoslaw, 2022; Sara et al., 2024; Anh et al., 2023). During dry–wet cycles, the process of electrolysis occurs between hydrogen ions and hydroxide ions within the aqueous solution. This process allows for the penetration of these ions into the interior space of soft rock, where they undergo neutralization reactions with the anions and cations that comprise the mineral components inside. The ongoing process of dissolution has an impact on the clay minerals present in the soft rock. The series of chemical reaction processes mainly involves the measurement of ion concentration in aqueous solutions, changes in mineral composition inside the soft rock, and the disclosure of damage mechanisms under dry–wet cycles based on experimental results (Zhang N. et al., 2023; Kausar et al., 2024; Huang et al., 2022; Liu C. et al., 2022). Some researchers focus their attention on the analysis of easily disintegrating soft rock, studying its softening process and exploring changes in physical and mechanical properties. They also aim to establish correlations between rock mass and its microstructural dynamics in order to elucidate collapse mechanisms. Such research is crucial for understanding the behavior of soft rock and its potential impact on geological formations (Liu and Lu et al., 2000; Huang and Che, 2007; Xu et al., 2024). The adverse effects of dry–wet cycles on soft rock are primarily evident in the ion exchange between water and rock, as well as changes in mineral composition, which represent chemical reactions at the microscopic level.
Soft rock, due to its discrete and vulnerable nature, exhibits strong overall failure and nonlinear disintegration softening characteristics during the dry–wet water cycle. These characteristics are primarily attributed to the ingress of water into the interior of soft rock, which disrupts the bonding between particles and leads to the formation of numerous cracks. Consequently, a significant number of researchers have directed their attention toward investigating the mechanism of damage caused by water–rock interaction during dry–wet cycles at both micro- and macro-scales. For example, soft rock can be subjected to triaxial compression tests and scanning electron microscopy analysis under saturated conditions, with varying confining pressures and saturation levels used. The possibility to perform such tests is based on the rock’s mechanical properties and microstructural characteristics. By subdividing the primary damage areas of soft rock samples, it is possible to analyze the two-stage progressive freeze–thaw damage process both before and after surface peeling. Furthermore, it is essential to establish a correlation between the internal constitutive model of soft rock and related variables (Zhao, 2022; Liu et al., 2021; Jiang et al., 2022; Ning et al., 2023). Uniaxial compression failure tests were conducted on dry, natural, and saturated soft rock using acoustic emission detection to examine the physical and mechanical properties, water softening characteristics, and energy damage evolution mechanism of mudstone. The peak stress and elastic modulus of soft rock decrease with increasing water content, leading to a gradual transition in deformation characteristics from brittleness to ductility (Mahbashi et al., 2023; Feng et al., 2023; Chen et al., 2018; Liu X. X. et al., 2022; Huang et al., 2023). In terms of numerical and theoretical models, discrete element simulation is primarily used to investigate the damage and fracture processes occurring between particle micro-contacts. During simulation, the correlation between micro-parameters and macro-parameters is systematically analyzed and validated. A proposed empirical formula for the shear band inclination angle takes into account the shear dilation angle. In addition, the parallel bonding model introduces tensile and shear damage variables to characterize the deformation, strength, and energy evolution characteristics of particle connection bonds (Liu et al., 2024; Jia et al., 2023; Zhou et al., 2024). Therefore, under the action of dry–wet water cycles, soft rock undergoes continuous internal particle damage and accumulation, eventually leading to the comprehensive evolution of crack formation. However, understanding of the intrinsic correlation mechanism of softening in soft rock is still in its early stages. The correlation between the characteristics of granular changes and the expansion of soft rock fractures requires further investigation.
The dry–wet cycle has a direct impact on the cementation effect of clay mineral particles during the failure process of soft rock. As the interconnection between skeletal particles weakens, microcracks persist in occurring and expanding, ultimately leading to a reduction in the compressive strength of the rock mass. Existing research on this topic has primarily focused on quantitatively characterizing soft rock structures. The porosity and arrangement of particles within soft rock undergo changes, leading to the formation of different shapes (Li Z. S. et al., 2022; Zhang Z. P. et al., 2023; Dong et al., 2024; Lai et al., 2024; Wang et al., 2023). Furthermore, as surface cracks develop in soft rock, there is continued appearance and accumulation of blocks of rock and soil failure. Analyzing the fractal path and fractal dimension of cracks and paths can provide a better understanding of the macroscopic failure mechanism of soft rock (Xin et al., 2023; Liu and Kang, 2023). Therefore, quantitative characterization using fractals can effectively capture both the discrete and distributed characteristics of rock damage within soft rock. Based on the fractal dimension and fractal path, the damage mechanism of soft rock under the action of dry–wet cycles can be further elucidated.
Based on the above research findings, it is evident that soft rock undergoes internal structural changes evolving into external macroscopic damage under the action of dry–wet cycles. The chemical reactions between water and soft rock, along with the exacerbation and accumulation of damage between the particles of soft rock, ultimately lead to the overall failure of the soft rock. However, the damage mechanism of soft rock is extremely complex, and further research on related scientific issues is still required. Research on the damage mechanism of soft rock is typically divided into two levels: mesoscopic and macroscopic. Mesoscopic research primarily focuses on the mineral composition and microstructure of soft rocks, while macroscopic research centers on the mechanical properties and deformation and failure characteristics of soft rocks. However, previous studies have often failed to effectively integrate the meso and macro levels, resulting in a lack of comprehensive understanding of the mechanism of soft rock damage. For instance, further research is needed to investigate how changes in mesostructure affect the macroscopic mechanical properties of soft rocks, as well as the evolution laws of mesostructure during macroscopic deformation and failure processes. Such factors therefore raise questions: for example, how can we quantitatively characterize the damage variable of soft rock and then invert the evolution law of the internal structure of soft rock based on this information? How can we determine the intrinsic relationship between particle damage and macroscopic cracks in the process of soft rock damage and thereby reveal the dynamic reorganization behavior of the internal structure of soft rock? To address the above two issues, a more comprehensive prediction of the strength variation law under the action of water in soft rock needs to be achieved. This prediction can provide more accurate theoretical support for practical engineering. Therefore, in this paper, the number of dry–wet cycles of soft rock is taken as the initial external condition. The number of cracks after the failure of soft rock was quantitatively characterized based on fractal theory and multifractal features. Simultaneously, the correlation between changes in the internal structure of soft rock and macroscopic failure was revealed.
2 The fractal mechanism of soft rock structure
Soft rock consists of hydrophilic clay particles and non-hydrophilic skeleton particles, which exhibit obvious dispersion. Unlike hard rock, the compressive strength of soft rock is not determined by the bearing capacity of the particles themselves. Under the action of external loads, there are no force chains between particles. A large quantity of hydrophilic clay particles surround non-hydrophilic skeleton particles. These two types of particles, along with hydrophilic particles, are interconnected through cementation, collectively influencing the compressive strength and other mechanical properties of soft rock. There are numerous gaps of varying sizes on the outer surface and inside of soft rock, which indicate the initial level of damage to the soft rock. In addition, there are two different types of contact modes between particles in soft rock, a parallel bonding mode and a rigid connection mode, as shown in Figure 2.
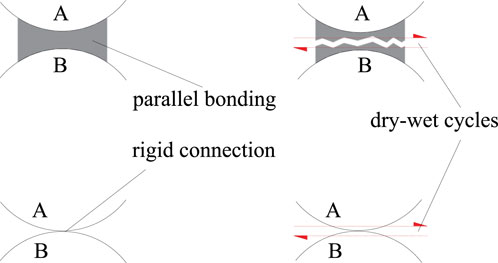
Figure 2. The particle connection mode and dry-wet cycles effect inside soft rock (both A and B in the figure are particles).
The particles are bonded together to create a strong bonding effect, which is a concentrated manifestation of the parallel bonding mode. When water infiltrates the rock, these cementitious substances are susceptible to chemical reactions with water, which disrupts the cementation process and ultimately leads to particle separation and sliding. In the case of rigid connections, there is no apparent bonding mineral between particles. The outer surface and interior of soft rock contain gaps of different sizes, reflecting the level of initial damage.
The strength of soft rock is influenced by a combination of factors such as mineral composition, particle bonding properties, and grain size. These factors interact in a complex manner to determine the overall strength of the rock. Due to the independent nature of these factors and their statistical regularity, the strength of soft rock can be described using statistical distribution patterns. Assuming that the strength of soft rock follows a Weibull distribution, the probability density function is given by (Yi et al., 2022; Bai et al., 2023)
In Formula 1,
The majority of non-hydrophilic skeletal particles in soft rock are enveloped by clay minerals. The damage caused to clay minerals by dry–wet cycles is random (Formula 2), representing the concentrated manifestation of random fractal evolution within soft rock in terms of the action and intensity of failure. As illustrated in Figure 3, the interior surface of soft rock undergoes changes when exposed to dry–wet cycles. Both the external and internal surfaces exhibit varying degrees of damage, with the void volume gradually increasing and the internal solid volume decreasing. This state can be considered as the initial element of the soft rock. The internal structure distribution is highly irregular and disordered, with fragmented void distribution demonstrating significant randomness. Because of the unpredictable nature of damage caused by dry–wet cycles, the deterioration of soft rock particles demonstrates significant discreteness and probability distribution. Through repeated destructive effects and continuous fractal processes, the degree of deterioration in soft rock continues to intensify.
3 Soft rock failure test under dry-wet cycles
3.1 Test equipment and soft rock samples
The dry–wet cycle test performed in the present study was conducted using an NT-3A digital-display electric constant-temperature blast-drying oven. The compression failure test performed on the rock samples was conducted using the Matest mechanical performance testing machine developed in Italy. As part of the present study, we focused on investigating the impact of dry–wet cycles on the mechanical properties of soft rock, specifically mudstone and siltstone with a high clay mineral composition. The experiments focuse on the silty mudstones in southern China, which belong to moderately weathered soft rock. The minerals inside this type of soft rock mainly comprise quartz and feldspar, with mud calcium cement. Its natural density is 2.211 g/cm3. Standard cylindrical specimens were prepared by processing drilled rock cores using automatic core-taking machines, automatic rock-cutting machines, and double-end grinding machines. The sample size was Φ 50 mm × 100 mm.
3.2 Experimental methods
As illustrated in Figure 4, it is the entire process of the experiments. The below are the brief explanations of each step.
Dry–wet cycle tests: The rock samples were first soaked in natural water for 48 h and then dried in an oven at a temperature of 105°C for 24 h. After reaching room temperature, the rock samples were removed, marking the completion of one dry–wet cycle process. Each rock sample was subjected to zero, one, three, and seven dry–wet cycles. Parallel experiments were carried out on three rock samples within each group.
Uniaxial compression failure tests: After undergoing a series of dry–wet cycles, uniaxial compression failure tests were conducted on each rock sample. The loading method employed displacement control, with a loading rate set at 0.03 mm/min. Axial loading was applied to the rock samples using a testing machine until failure occurred. This experimental process enabled the collection of dynamic stress–strain data during the loading process of the rock samples, as well as the capture of images of rock samples after failure.
Tests on microscopic structure and mineral composition: To analyze the influence of dry–wet cycles on the internal microstructure of the rock samples, scanning electron microscopy (SEM) experiments were conducted on sliced rock samples. Scanning images of the microstructure of the rock samples at 2,000 times magnification were obtained after zero, one, three, and seven dry–wet cycles.
4 Results
The test results of the uniaxial compression failure test of the rock samples after zero, one, three, and seven dry–wet cycles are shown in Figure 5. From the figure, it is evident that the peak compressive strength of the rock samples gradually decreases as the number of dry–wet cycles increases. The peak compressive strength of the dry rock samples is 25.06 MPa. After seven dry–wet cycles, the rock samples’ compressive strength decreased by 5.29 MPa–19.77 MPa. The change in the stress–strain curve is notable. When the number of dry–wet cycles is 0, the compressive strength of the rock samples rapidly decreases from the highest stress, exhibiting strong brittle characteristics. This finding indicates that the density of the dry rock samples is high. Following a varying number of dry–wet cycles, the rate of decrease in maximum stress decreases and the maximum strain increases. The rock samples undergo a gradual transition from brittle to plastic characteristics. The primary reason for this finding is that the internal structure of the rock samples becomes loose after undergoing a cycle of dry and wet conditions. This phenomenon occurs due to the interaction of water with the rock samples, leading to the weakening of its internal integrity. Furthermore, the bonding effect between mineral particles within the rock samples gradually decreases over time, leading to the lubrication and softening of hydrophilic clay mineral particles. This phenomenon is indicative of a continuous decrease in the interparticle bonding strength within the rock samples.
As shown in Formula 3, in order to analyze the changes in the mechanical properties of soft rock under the action of dry–wet cycles, the degradation degree
In Formula 3,
Based on the results presented in Table 1, the degradation of the peak compressive strength of the rock sample demonstrates an increasing trend from fast to slow as the number of dry–wet cycles increases. Compared to the initial state, after one, three, and seven dry–wet cycles, the cumulative increment of peak compressive strength degradation during uniaxial compression is 12.76%, 17.63%, and 21.58%, respectively. From the degree of degradation in the peak compressive strength stage, it is evident that the first three dry–wet cycles have a significant impact on the peak compressive strength. The relatively fast degradation rate accounts for approximately 80% of the total degradation degree. As the number of dry–wet cycles increases, there is no significant change in the degradation degree during the peak compressive strength stage. After one dry–wet cycle, a deterioration of 12.76% in the peak compressive strength during this stage was identified, which gradually decreased thereafter. After three dry–wet cycles, this decrease reached 4.87%. Therefore, the above results indicate that these three dry–wet cycles had a significant impact on the compressive strength of the rock samples. Subsequently, an increase in the number of dry–wet cycles did not have a significant impact on the compressive strength of the rock samples.
As the number of dry–wet cycles increases, the overall degradation of the elastic modulus of the rock samples shows a trend of increasing from fast to slow. Compared to the initial state, after one, three, and seven dry–wet cycles, the total degradation increment of the elastic modulus of the rock samples is 8.156%, 14.98%, and 16.84%, respectively. From the perspective of elastic modulus degradation, it is evident that the initial three dry–wet cycles have a significant impact on the elastic modulus of the rock samples. The degradation rate is relatively fast, accounting for approximately 88% of the total degree of degradation. However, as the number of dry–wet cycles increases, there is a rapid decrease in this degradation trend. After one dry–wet cycle, there was an 8.156% deterioration in the strength stage, which gradually decreased thereafter. After seven dry–wet cycles, this deterioration rapidly decreased to only 1.86%. It can be concluded that dry–wet cycling has a significant impact on the elastic modulus and ultimately leads to a substantial decrease in the compressive strength of the rock samples.
5 Discussion
5.1 Bonding mechanism between particles of soft rock
To analyze the microstructural changes in the rock samples after a varying number of dry–wet cycles, the internal distribution characteristics of the structure were determined using scanning electron microscopy (SEM). The distribution morphology of the internal structure of the rock samples was determined, as shown in Figure 6. The image was magnified 1,000 times by an electron microscope.
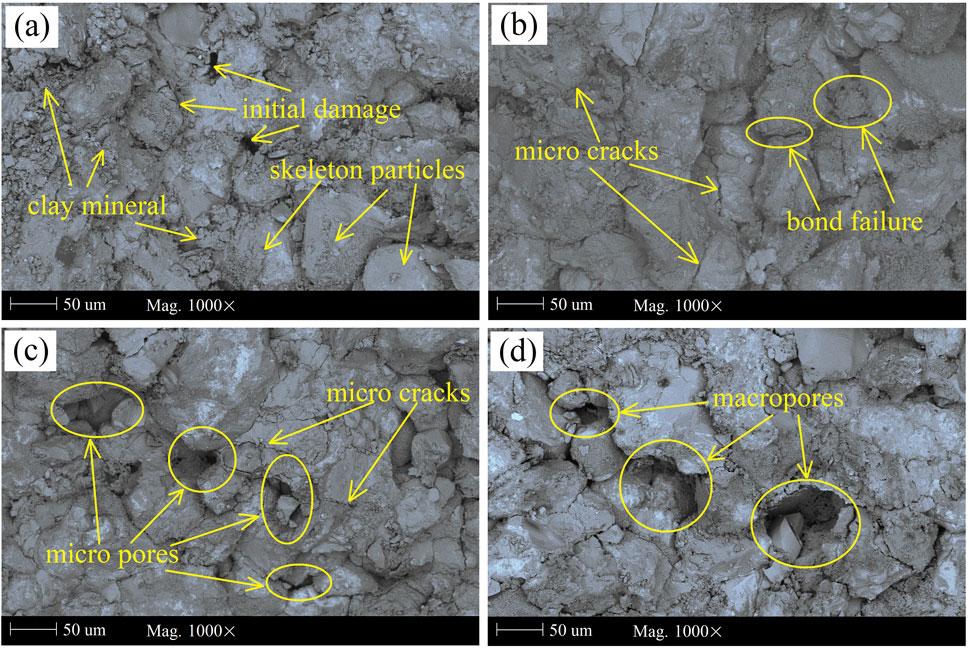
Figure 6. Internal microstructure of rock samples after different dry-wet cycles. (A) 0 times. (B) 1 time. (C) 3 times. (D) 7 times.
From Figure 6, it is evident that the interior surface of the soft rock primarily consists of block-like particles, which are non-hydrophilic and predominantly composed of quartz. Surrounding these non-hydrophilic minerals are hydrophilic clay minerals, closely interconnected as a whole. In the absence of dry–wet cycles, the initial damage to the rock samples is minimal, with fewer microcracks and micropores. At this stage, the integrity of the rock samples is good and the bonding between particles is relatively strong. However, as the number of dry–wet cycles increases, there is a significant increase in internal damage to the rock samples. Microcracks continue to proliferate and lengthen, while micropores also increase accordingly. Specifically, after seven cycles of dry and wet conditions, the internal structure of the rock samples becomes very loose. This phenomenon leads to the expansion and aggregation of cracks in addition to further enlargement of pores, creating favorable channels for water infiltration. The end result is a decrease in mechanical properties such as the compressive strength and shear strength of the rock samples.
However, under the action of dry-wet cycles, there is always energy dissipation due to the interaction between the destruction of particles inside soft rocks and water. Researchers such as Bai (Bai et al., 2022) have confirmed that when hydrated minerals undergo dissociation, their internal particles exhibit recombination characteristics and cause corresponding energy dissipation. Under this destructive mechanism, the bonding force between particles decreases. Meanwhile, the findings of Bai (Bai et al., 2020) indicate that the movement of water can cause the migration of mineral chemical composition. Due to the significant driving effect of temperature gradient, moisture promotes the gradual movement of relevant ions towards distant areas and forms an uneven distribution. Therefore, the dissipation and abrupt changes of energy, as well as the driving force of temperature gradients, can cause random damage paths in soft rocks, ultimately leading to deformation and failure of soft rocks.
5.2 Failure mode of soft rock
Figure 7 illustrates the final morphology of the rock samples after uniaxial compression failure following a varying number of dry–wet cycles. With an increasing number of dry–wet cycles, the rock samples undergo a transition from tensile fracture along the axial direction to shear failure, resulting in an increased degree of failure. During tensile failure, macroscopic cracks in the rock samples align with the direction of principal stress. Furthermore, as the number of dry–wet cycles increases, more and wider cracks are generated during loading and failure of the rock samples.
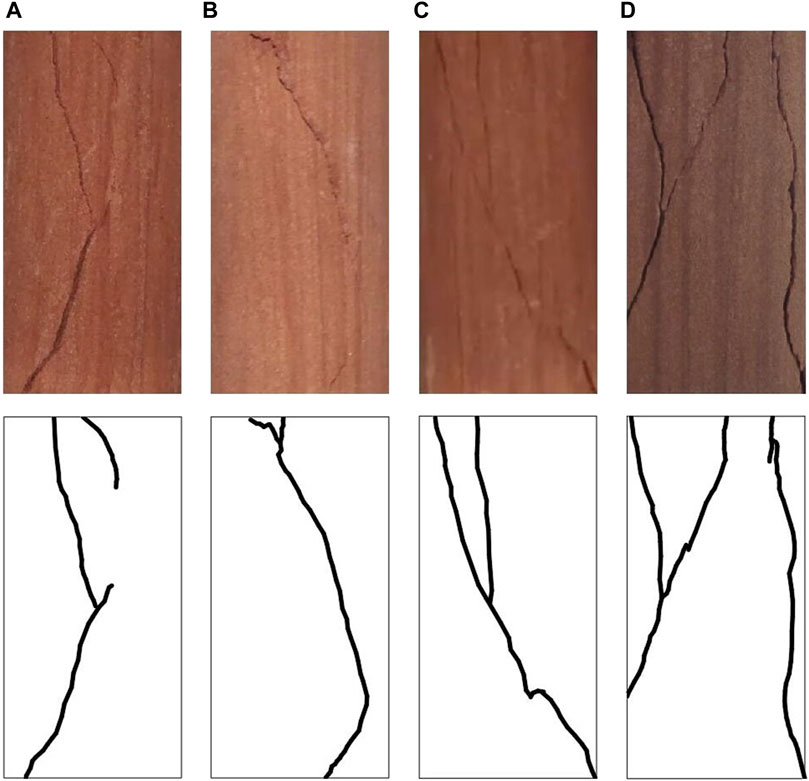
Figure 7. Uniaxial compression failure mode of rock samples. (A) 0 dry-wet cycles. (B) one dry-wet cycle. (C) three dry-wet cycles. (D) seven dry-wet cycles.
5.3 Fractal dimension of cracks in rock sample
Based on the image captured of the rock samples after failure, we focused on tracking the distribution characteristics of surface cracks. The final crack condition of the rock samples is mainly characterized by the number and length of cracks. The evolution process of cracks provides the most direct basis for understanding its failure. Therefore, a quantitative characterization of the fractal dimension of the final fracture morphology was carried out to further explore the damage law of soft rock. Assuming that a small square with a side length of
In Equation 4,
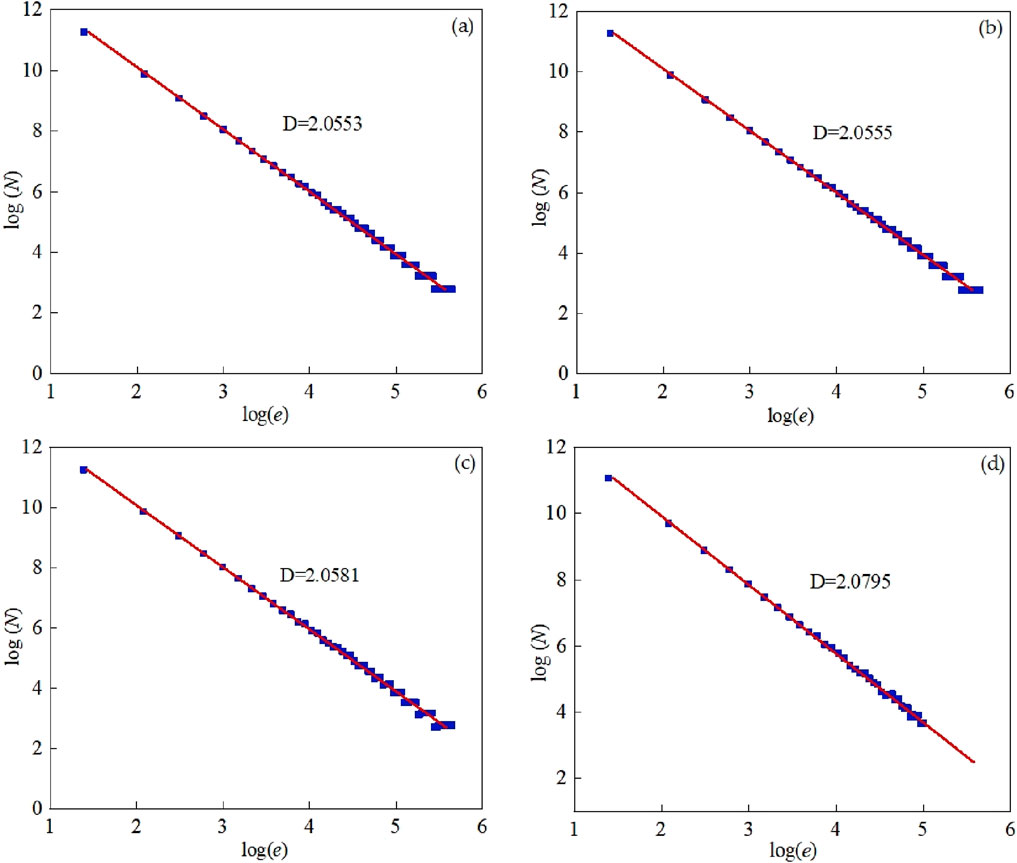
Figure 8. The fractal dimension of the final fracture of rock samples. (A) 0 dry-wet cycles. (B) one dry-wet cycle. (C) three dry-wet cycles. (D) seven dry-wet cycles.
From Figure 8, it is evident that the fractal dimension of rock sample cracks varies with the number of dry–wet cycles. This finding suggests that the damage circumstances and crack distribution characteristics of rock samples vary due to the different effects of water. When comparing the fractal dimension under different dry–wet cycles, an overall increasing trend was observed. This finding indicates that as the number of dry–wet cycles increases, the pore structure inside the soft rock becomes more complex, leading to a broader distribution of cracks. Consequently, this process results in more pronounced brittle characteristics in the rock samples. Regarding the results described above, we only observed the overall characteristics of soft rock during a specific saturated time period, without capturing its specific detailed structure. Soft rock, as a dispersed body, possesses an extremely complex and diverse internal structure. Merely studying its overall condition may lead to overlooking many important details. Therefore, it is imperative to delve into the specific details and conduct more in-depth research.
5.4 Multifractal characteristics of soft rock cracks
In the above fractal dimensions, the overall structural failure of the rock samples can be efficiently obtained. However, for the distribution of cracks, it is more difficult to analyze more detailed structures using fractal dimension. For example, important information such as the uniformity, distribution probability, and location of cracks can better reflect the mechanical properties of soft rock. In order to comprehensively analyze the cracks in rock samples, we adopted the multifractal analysis method in our study. By selecting the range of variation in statistical moments’ orders, the multifractal characterization parameters were calculated for void structures during soft rock failure. According to the relevant literature on multifractal theory provided in this field, we followed the calculation methods stated therein (Hadrien et al., 2017). When determining the order
In Formula 5,
In Formula 6,
According to the Legendre transformation, we have
Using the Formulas 7, 8, we selected the order variation range of from −10 to 10, with a variable of 1. The distribution function, generalized fractal dimension, and multifractal spectrum of surface cracks on rock samples were calculated according to relevant programs; the results are shown in Figures 9–11.
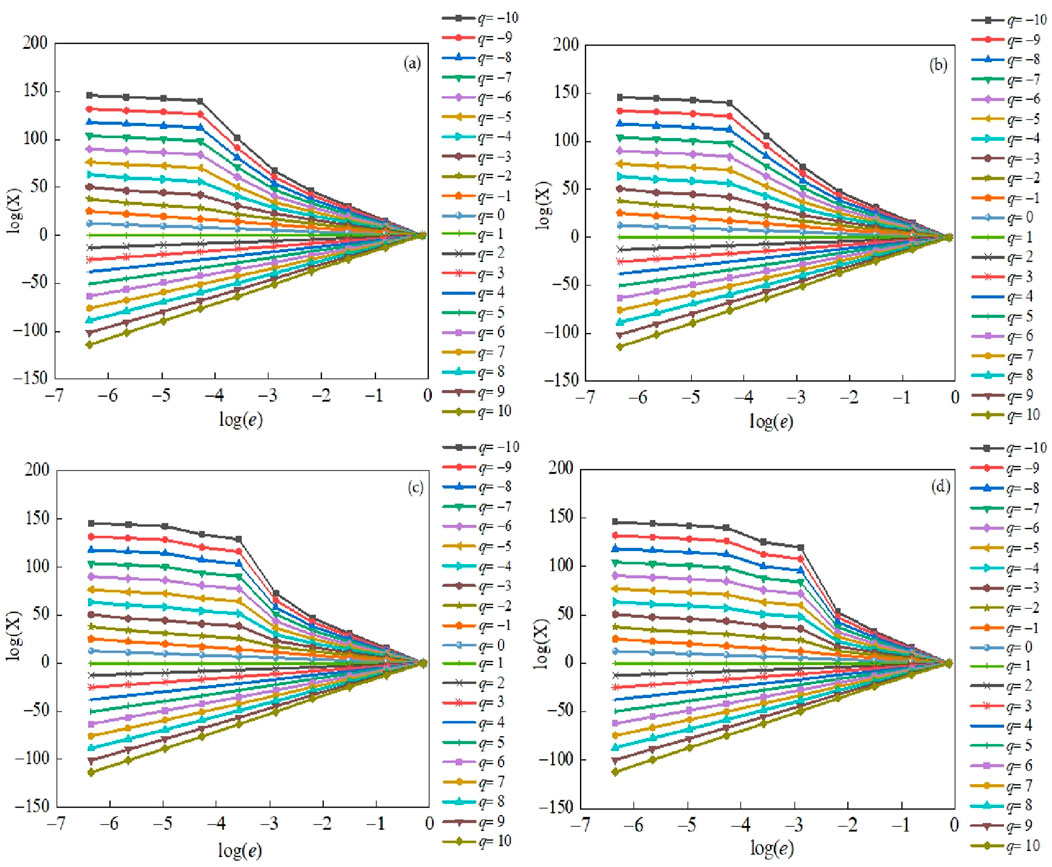
Figure 9. The cracks distribution function for the ultimate failure of rock samples. (A) 0 dry-wet cycles. (B) one dry-wet cycle. (C) three dry-wet cycles. (D) seven dry-wet cycles.
From Figure 9, it is evident that the crack distribution of the rock samples demonstrates a strong linear relationship between scale and frequency, indicating a typical first-order power-law distribution. Particularly for the part with order
From Figure 10, it is evident that the variation in the generalized fractal dimension
From Figure 11, it is evident that the multifractal spectral function curve exhibits an upward convex trend with consistently occurring maximum values. The maximum value of
5.5 Mechanism of soft rock damage evolution
Based on the results of the experiments described above and a comprehensive analysis of multifractals, it can be concluded that the effect of damage on soft rock exhibits randomness and follows relevant probability distributions. For soft rock, the process of dry–wet cycles represents a complex form of deterioration. The extent of this destructive effect is closely related to the path and intensity of the fractal. Furthermore, localized damage within the soft rock accumulates and evolves continuously, ultimately leading to the failure of the rock mass. This phenomenon is mainly related to the inherent evolution of the relationship between micro- and macro-perspectives.
As shown in Figure 12, the soft rock has local damage in its initial state. At this point, the number of internal gaps is minimal, and the bonding between particles is relatively tight. When soft rock is subjected to dry–wet cycles, water repeatedly breaks down the bonding material between particles. This kind of destruction is a concentrated manifestation of fractals. In the initial state, fractal initial units exist in soft rock. As the dry–wet cycles continue, these initial units expand and extend to different extents and in different directions, generating secondary elements and new initial elements. Due to the fractal effect having a certain probability distribution, during low-order dry–wet cycles, damage to soft rock typically occurs locally. After multiple cycles of high-level moisture and dryness, the continuous expansion and accumulation of the damaged area ultimately lead to an increase in macroscopic cracks in soft rock. This finding precisely validates the experimental results and the distribution pattern of the multifractal spectrum, demonstrating the inherent correlation mechanism between meso- and macroscopic damage in soft rock.
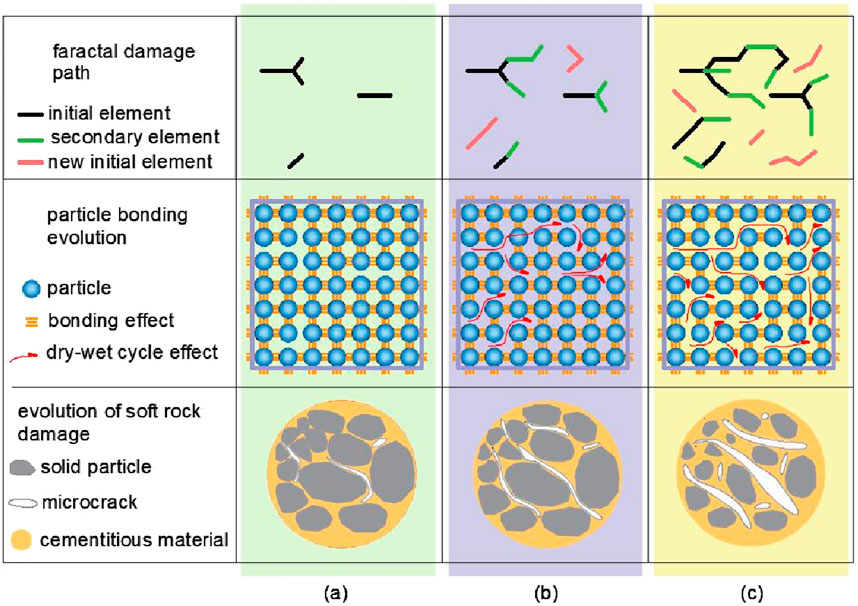
Figure 12. The intrinsic correlation mechanism of fractal damage in soft rocks. (A) initial state. (B) low dry-wet cycles. (C) high order dry-wet cycles.
6 Conclusion
In the study presented herein, the multi-scale failure mechanism and fractal damage effect of soft rock were investigated through the use of dry–wet cycle and uniaxial compression failure tests. In this study, the structural changes and correlations between different scales in the process of soft rock failure are explored. The main conclusions of this study are as follows.
The damage to soft rock is a concentrated manifestation of the fractal effect, which is subject to randomness. The greater the number of dry–wet cycles, the greater the fractal dimension of crack damage in soft rock and the greater the extent of damage to the soft rock. The failure pattern of soft rock exhibits obvious fractal structural characteristics in terms of the distribution function of cracks and the generalized fractal dimension. With an increasing number of dry–wet cycles, the distribution of cracks in soft rock becomes more widespread, and the difference in generalized fractal dimension becomes larger. This finding indicates a greater degree of deterioration of soft rock.
Under the action of dry–wet cycles, the strength and direction of fractal pathways can alter the structural distribution of soft rock. The bonding between particles within the soft rock is disrupted and reorganized. The continuous increase and accumulation of internal damage in soft rock lead to the generation and propagation of macroscopic cracks. This phenomenon ultimately results in a reduction in the elastic modulus and compressive strength of the soft rock. Soft rock exhibits both meso- and macroscopic damage characteristics under the action of dry–wet cycles, and there exists a fractal intrinsic correlation between these two scales.
Data availability statement
The original contributions presented in the study are included in the article/Supplementary Material, further inquiries can be directed to the corresponding author..
Author contributions
NL: Conceptualization, Formal Analysis, Funding acquisition, Methodology, Software, Writing–original draft, Writing–review and editing. TJ: Data curation, Methodology, Validation, Writing–original draft. DS: Data curation, Formal Analysis, Supervision, Writing–review and editing.
Funding
The author(s) declare that financial support was received for the research, authorship, and/or publication of this article. This research was funded by the Guangxi Science and Technology Base Talent Project (AD21220126), the Central guidance local science and technology development fund projects (2022SRZ0101), the Basic Ability Enhancement Program for Young and Middle-Aged Teachers of Guangxi (2021KY0358, 2023KY0346), and the Guangxi Univer-sity of Science and Technology Doctoral Fund Project (21z53), Guangdong Provincial Department of Housing and Urban-Rural Development Science and Technology Plan Project under Grant (2021-K1-140626), and the China Postdoctoral Science Foundation Funded Project (2020M682671).
Conflict of interest
Author DS was employed by Guangzhou Institute of Building Science Group Co., Ltd. Author DS was employed by Guangzhou Jianyan Engineering Technology Co., Ltd.
The remaining authors declare that the research was conducted in the absence of any commercial or financial relationships that could be construed as a potential conflict of interest.
Publisher’s note
All claims expressed in this article are solely those of the authors and do not necessarily represent those of their affiliated organizations, or those of the publisher, the editors and the reviewers. Any product that may be evaluated in this article, or claim that may be made by its manufacturer, is not guaranteed or endorsed by the publisher.
Supplementary material
The Supplementary Material for this article can be found online at: https://www.frontiersin.org/articles/10.3389/feart.2024.1466304/full#supplementary-material
References
Ali, S., and Davood, Z. (2020). Detailed analysis of the brine-rock interactions during low salinity water injection by a coupled geochemical-transport model. Chem. Geol. 537, 119484. doi:10.1016/j.chemgeo.2020.119484
Anh, T. D., Nadine, G., and Nico, G. (2023). Use of major ion chemistry and trace and rare earth elements to characterize hydraulic relations, mixing processes and water-rock interaction in the Dong Van Karst aquifer system, Northern Vietnam. Hydrogeol. J. 31, 1735–1753. doi:10.1007/s10040-023-02689-4
Bai, B., Xu, T., Nie, Q. K., and Li, P. P. (2020). Temperature-driven migration of heavy metal Pb2+ along with moisture movement in unsaturated soils. Int. J. Heat. Mass Transf. 153, 119573. doi:10.1016/j.ijheatmasstransfer.2020.119573
Bai, B., Zhou, R., Yang, G. C., Zou, W. L., and Yuan, W. (2022). The constitutive behavior and dissociation effect of hydrate-bearing sediment within a granular thermodynamic framework. Ocean. Eng. 268, 113408. doi:10.1016/j.oceaneng.2022.113408
Bai, Y., Zhao, K. P., Liu, Y., Hu, Z. H., Bai, S., and Yang, W. (2023). Shale dynamic damage constitutive model based on Weibull distribution under variable confining pressure. Eng. Blasting. 29, 61–67+99. doi:10.19931/j.EB.20220086
Chen, Z. Q., He, C., Dong, W. J., Ma, G. Y., Pan, X. Y., and Pei, C. Y. (2018). Physico-mechanical properties and its energy damage evolution mechanism of the Jurassic and Cretaceous argillaceous sandstone. Rock Soil Mech. 39, 2873–2885. doi:10.16285/j.rsm.2018.0212
Dong, H. M., Luo, B., Dang, C. Y., Xu, S., Wang, F., and Chi, P. (2024). Quantitative characterization of the carbonate rock microstructure considering topological features: a case study from the Gaoshiti-Moxi block of the Sichuan Basin. Front. Earth Sci. 12, 1375637. doi:10.3389/feart.2024.1375637
Feng, X. L., Ma, Z. Q., and Chen, A. M. (2023). Study on mechanical and energy characteristics of red shale under wetting-drying cycle. Min. Saf. Environ. Prot. 50, 47–54. doi:10.19835/j.issn.1008-4495.2023.04.009
Hadrien, S., Roberto, M., and Elsa, A. (2017). Multifractal methodology. Phys. A Amst. Neth. 473, 467–487. doi:10.1016/j.physa.2017.01.041
Huang, H. W., and Che, P. (2007). Research on micro-mechanism of softening and argillitization of mudstone. J. Tongji Univ. Nat. Sci. . 35, 866–870. doi:10.3321/j.issn:0253-374X.2007.07.002
Huang, K., Dai, Z. J., Meng, Y. Y., Yu, F., Yao, J. K., Zhang, W., et al. (2023). Mechanical behavior and fracture mechanism of red-bed mudstone under varied dry-wet cycling and prefabricated fracture planes with different loading angles. Theo. Appl. Fract. Mec. 127, 104094. doi:10.1016/j.tafmec.2023.104094
Huang, T. M., Li, Z. B., Long, Y., Zhang, F., and Pang, Z. H. (2022). Role of desorption-adsorption and ion exchange in isotopic and chemical (Li, B, and Sr) evolution of water following water-rock interaction. J. Hydrol. 610, 127800. doi:10.1016/j.jhydrol.2022.127800
Jia, Y. F., Bai, Y. X., Xia, D., Li, F. P., and Liang, B. (2023). Energy dissipation and damage evolution during dynamic fracture of muddy siltstones containing initial damage under the freeze thaw effect. Materials 16, 16010120. doi:10.3390/ma16010120
Jiang, J., Hou, Z. M., Hou, K. P., Lu, Y. F., Sun, H. F., and Niu, X. D. (2022). The damage constitutive model of sandstone under water-rock coupling. Geofluids 2022, 1–12. doi:10.1155/2022/1731254
Kaniz, R., Shaini, A. H., Melissa, M. L., Tang, C. S., Xue, W., and Zhu, C. (2023). Desiccation cracking remediation through enzyme induced calcite precipitation in fine-grained soils under wetting drying cycles. Biogeotechnics 1, 100049. doi:10.1016/j.bgtech.2023.100049
Kausar, S. S., Mohd, H. B. M. H., Hafeezur, R., and Kamar, S. A. (2024). Effect of wet-dry cycling on the microstructure of various weathering grade sandstone. Appl. Mech. Mater. 920, 183–187. doi:10.4028/p-103kzt
Krzysztof, L., and Radoslaw, T. (2022). Modeling hydrogen-rock-brine interactions for the Jurassic reservoir and cap rocks from Polish Lowlands. Int. J. Hydrogen Energy. 47, 10947–10962. doi:10.1016/j.ijhydene.2022.01.134
Lai, Q., Wu, Y. Y., Zeng, Y., Xie, B., Jiang, Y. K., Chen, L., et al. (2024). Quantitative characterization of fractures and holes in core rolling scan images based on the MFAPNet deep learning model. Front. Earth Sci. 11, 1331391. doi:10.3389/feart.2023.1331391
Lin, T., Wang, W. W., Ma, Q., Kang, J. L., Yang, R. Z., and Liu, X. H. (2024). Formation mechanism and implication of analcime in the sandstone reservoirs of the Permian Jingjingzigou formation in the Jinan sag, southern Junggar basin, NW China. Front. Earth Sci. 12, 1416594. doi:10.3389/feart.2024.1416594
Liu, C., Liu, X. L., Peng, H. Y., Wang, E. Z., and Wang, S. J. (2024). Discrete element study on the mechanical response of soft rock considering water-induced softening effect. Appl. Sci. 14, 14093918. doi:10.3390/app14093918
Liu, C., Liu, X. L., Zhang, D., Wu, C. L., Wang, E. Z., and Wang, S. J. (2022). Dynamic model for water-rock interface of softening of soft rock and its evolution law. Chin. J. Geotech. Eng. 44, 2280–2289. doi:10.11779/CJGE202212015
Liu, C. W., and Lu, S. L. (2000). Research on mechanism of mudstone degradation and softening in water. Rock Soil Mech. 21, 28–31. doi:10.16285/j.rsm.2000.01.008
Liu, J., Zhang, H., Wang, R. H., Wang, F., and He, Z. W. (2021). Investigation of progressive damage and deterioration of sandstone under freezing-thawing cycle. Rock Soil Mech. 42, 1381–1394. doi:10.16285/j.rsm.2020.0803
Liu, X. X., Li, Y., Fan, Z. J., Li, S. N., Wang, W. W., and Dong, P. (2022). Energy evolution and failure characteristics of single fissure carbonaceous shale under drying-wetting cycles. Rock Soil Mech. 43, 1761–1771. doi:10.16285/j.rsm.2021.1657
Liu, Y. D., and Kang, H. P. (2023). Study on cross-scale fractal law of disintegration and failure of water-bearing mudstone under different stress paths. Chin. J. Rock Mech. Eng. 42, 4162–4173. doi:10.13722/j.cnki.jrme.2022.0608
Li W., W., Liu, S. S., Wang, Y. C., Qin, T. L., Zhang, X., Li, C. H., et al. (2022). Experimental study of rock wool on the farmland soil erosion and crop growth of winter wheat and its comprehensive evaluation. Front. Environ. Sci. 10, 1090604. doi:10.3389/fenvs.2022.1090604
Li Z. S., Z. S., Yi, H. Y., Zhu, C., Zhuo, Z., and Liu, G. S. (2022). Randomly generatin the 3D mesostructure of soil rock mixtures based on the full in situ digital image processed information. Fractal Fract. 6, 6100570. doi:10.3390/fractalfract6100570
Mahbashi, A. M., Elkady, T., and Al-Shamrani, M. (2023). The role of stress states on the hysteric behavior of expansive soil under multiple drying-wetting cycles. Buildings 13, 13071619. doi:10.3390/buildings13071619
Ning, Z. G., Li, D. P., Yang, K. C., Yu, Y. B., and Zhou, Z. (2023). Dry-wet cycling damage mechanism of argillaceous siltstone in gaogou formation of upper cretaceous. J. Highw. Transp. Res. Dev. 40, 237–245. doi:10.3969/j.issn.1002-0268.2023.11.028
Sara, W., Alexis, N. S., and Kamini, S. (2024). Water-rock interactions drive chemostasis. Hydrol. Process. 38, 15078. doi:10.1002/hyp.15078
Wagdi, H., and Ahmed, A. (2023). Evaluation of the durability and strength of stabilized sabkha soil with geopolymer. Case Stud. Constr. Mat. 18, 02051. doi:10.1016/j.cscm.2023.e02051
Wang, Z. F., Yao, Y. B., Ma, R. Y., Zhang, X. N., and Zhang, G. B. (2023). Application of multifractal analysis theory to interpret T2 cutoffs of nmr logging data: a case study of coarse clastic rock reservoirs in southwestern bozhong sag, China. Fractal Fract. 7, 7010057. doi:10.3390/fractalfract7010057
Xin, Z. P., Chai, Z. Y., Sun, H. C., Li, T. Y., Liu, X. Y., and Duan, B. Y. (2023). Post-peak fracture-bearing characteristics and fragmentation distribution sandy mudstone. Rock Soil Mech. 44, 2369–2380. doi:10.16285/j.rsm.2022.1366
Xu, J., Tian, W. L., Bu, Y. S., and Yang, J. (2024). Experimental study on mechanical properties and microscopic mechanisms of layered sandstone after high temperature water cooling. Fron. Earth Sci. 12, 1394855. doi:10.3389/feart.2024.1394855
Yi, M., Zhao, T., Ma, F. F., Wang, L., and Yuan, C. (2022). Study of constitutive model of frozen sandstone damage based on Weibull distribution. Coal Geol. Explor 50, 116–124. doi:10.12363/issn.1001-1986.22.02.0070
Zhang, N., Wang, S. D., Li, Z., Guo, S. H., and Wang, R. C. (2023). Application of multifractal theory for determination of fluid movability of coal-measure sedmentary rocks using nuclear magnetic resonance (NMR). Fractal Fract. 7, 7070503. doi:10.3390/fractalfract7070503
Zhang, Z. P., Fu, X. D., Yuan, W., Sheng, Q., Chai, S. B., and Du, Y. X. (2023). The influence of the fractal dimension on the mechanical behaviors of the soil-rock mixture: a case study from southwest China. Fractal Ffract 7, 7020106. doi:10.3390/fractalfract7020106
Zhao, L. C. (2022). Experimental study on mechanical property softening of water saturated sandstone. Chin. J. Undergr. Space Eng. 18, 154–162. doi:10.3969/j.issn.1673-0836.2022.1.dxkj202201018
Zhou, X., Ju, N. P., Cai, J. C., He, C. Y., Wang, J., Wang, H., et al. (2024). Damage creep model of viscoelastic rock based on fractional derivative and experimental verification. Front. Environ. Sci. 12, 1338016. doi:10.3389/fenvs.2024.1338016
Keywords: soft rock, dry-wet cycles, damage, multifractal, failure mechanism
Citation: Liang N, Jin T and Su D (2024) Quantitative characterization of damage and the cross-scale evolution mechanism of soft rock under dry-wet cycles. Front. Earth Sci. 12:1466304. doi: 10.3389/feart.2024.1466304
Received: 17 July 2024; Accepted: 21 November 2024;
Published: 06 December 2024.
Edited by:
Yongqiang Zhou, Chinese Academy of Sciences (CAS), ChinaCopyright © 2024 Liang, Jin and Su. This is an open-access article distributed under the terms of the Creative Commons Attribution License (CC BY). The use, distribution or reproduction in other forums is permitted, provided the original author(s) and the copyright owner(s) are credited and that the original publication in this journal is cited, in accordance with accepted academic practice. No use, distribution or reproduction is permitted which does not comply with these terms.
*Correspondence: Dingli Su, c3VkaW5nbGlAbWFpbDIuc3lzdS5lZHUuY24=