- 1Chengdu Surveying Geotechnical Research Institute Co., Ltd. of MCC, Chengdu, Sichuan Province, China
- 2State Key Laboratory of Coal Mine Disaster Dynamics and Control, Chongqing University, Chongqing, China
To study the failure of red sandstone under extremely high stress during the service life of tunnels, an in-depth study was conducted on the mechanical properties of red sandstone under uniaxial loading and cyclic loading and unloading processes at different pH values using the AG-250kNIS electronic precision material testing machine and MTS815 mechanical testing machine. The results show that as the acidity and alkalinity increase, the peak stress under uniaxial loading decreases and the axial strain increases,The peak stress at failure is 9.40, 12.37, 7.18, and 5.36 MPa, respectively, accounting for 74.19%, 68.91%, 40.38%, and 36.21% of the uniaxial compressive strength; The number of cycles significantly decreases during cyclic loading and unloading fatigue failure, and the stress required for sandstone failure gradually decreases. The peak strength and elastic modulus of sandstone show a decreasing trend, indicating that the hydrochemical environment plays an accelerating role in rock degradation. During the cyclic loading and unloading process of sandstone, there is a continuous increase in dissipated energy and finally a sudden increase, the
1 Introduction
At present, in the critical period of international geotechnical engineering research, worldwide tunnel construction is at a stage where both new construction and maintenance are given prominence, and the exploration of rock mass development is continuously advancing, and the maintenance and preservation of existing tunnel structures have received unprecedened attention. The deterioration of sandstone under stress can have an impact on the deformation of tunnel lining, and different geological environments can also have certain impacts. Sandstone is subjected to loads such as vibration and machinery, as well as complex water environment erosion. The tunnel rock mass under extremely high stress is more prone to deterioration in mechanical properties under the erosion of the surrounding water environment, and the internal structure of the rock is often damaged, seriously threatening the safety and maintenance difficulty of tunnel engineering. Therefore, studying the fatigue failure of sandstone under the combined action of acid-base water environment and different load modes is of great significance.
There have been many research results on the mechanical properties of rocks in hydro-chemical environments. Wang et al. (2012) conducted corrosion tests on sandstone using different chemical solutions. The results showed that after soaking in aqueous chemical solutions, the elastic modulus, residual strength, and peak strength of the samples showed different rates of decrease. Different pH values and ion types had a significant impact on the macroscopic mechanical properties of sandstone samples. Chen et al. (2010) studied the effects of chemical solutions and stress on rocks and found that different pH values and peripheral pressures have an impact on the strength of rock samples. The results showed that the stronger the acidity and alkalinity of the solution, the lower the maximum strength and elastic modulus of the rock. Miao et al. (2016) and others simulated the changes in the physical parameters of granite and found that the higher the concentration of acid solution, the more obvious the deterioration of the rock. Researchers study the changes in the physical and mechanical properties of rocks under the influence of acidic and alkaline solution environments, as well as the effects of different hydro-chemical solutions on the internal structure of rocks (Wang et al., 2016; Liu et al., 2019; Liu et al., 2017). The mechanical properties of rocks under different stresses and frequencies under uniaxial and cyclic loads were studied using acoustic emission and CT methods. Rong et al. (2019). studied the influence of the hydro-chemical environment on the demonstration of microstructure through uniaxial experiments and mercury intrusion experiments; Li GL. et al. (2017). studied the timeliness of rocks under hydro-chemical action through uniaxial impact tests, and obtained the response law of rocks under dynamic compression after erosion; Pan et al. (2022). conducted chemical treatment on single fractured rocks, studied the deformation characteristics under uniaxial action, and proposed a constitutive model for coupled damage of hydro-chemical and fractured rocks. Yu et al. (2019). conducted mechanical tests on sandstone soaked in solutions with different pH values and subjected to freeze-thaw cycles, analyzing the mechanical degradation patterns and changes in porosity under the dual effects of hydro-chemical solutions and freeze-thaw cycles. Most of these studies have only considered the hydrochemical environment in which the rock mass is located, but rock masses under extremely high ground stress will exhibit new mechanical properties and failure characteristics, and there is currently little research on this aspect. Therefore, studying the dynamic mechanical properties and failure characteristics of rocks under extremely high stress in a hydrochemical environment can supplement the deficiencies in existing research on deep rock mechanics, and it is of great significance for deep excavation engineering.
Many scholars have also studied the physical properties of rocks under hydro-chemical corrosion conditions (Stewart and Val, 1999; Michael, 1998; Liu et al., 2018). This article takes tunnels under high stress in polar regions as the background and uses rocks eroded by hydro-chemical environments for uniaxial compression experiments and cyclic load experiments to analyze the mechanical properties and failure laws of sandstone under acidic and alkaline environments. This can provide a reference for the stability of tunnel rock masses and the evaluation and maintenance of tunnel safety.
2 Tunnel characteristics and rock sample preparation
2.1 Extreme geological characteristics of the tibet railway
The geological terrain of the Tibet Railway is complex, with active plate structures along the line, dense active cracks, strong seismic intensity, significant terrain fluctuations, and prominent natural disasters. Great technical challenges are encountered in the construction and maintenance of tunnel systems. The compressive action of crustal tectonic movements on the strata leads to the complication of the rock mass structural stress field and a state of extremely high stress; rocks under high stress often exist in a critical state after excavation, and are highly susceptible to unstable failure when subjected to dynamic loads from high-frequency mechanical drilling, blasting, and other engineering activities. At the same time, the depth of the railway tunnel entering Tibet is relatively deep, and the problems caused by high stress should not be underestimated. Weak rock masses, will slowly deform under the action of geostress, damaging tunnel support measures, causing tunnel deformation, and even leading to tunnel abandonment. Due to the complex structure and active tectonic movements in the area where the tunnel is located, the lithology of the strata along the route is uneven and the rock quality is complex. The problems of hard rock explosions and soft rock deformation are prominent, and have a significant impact on deep-buried tunnels.
The underground water reserves along the tunnel are abundant. Under high stress, rocks along the tunnel are prone to fracture and deformation, and the fractures are well-developed, creating conditions for the accumulation of groundwater. Rich groundwater not only leads to sudden water surges, affecting tunnel construction and operation but also may cause tunnel collapse accidents.
2.2 Rock preparation and experimental design
2.2.1 Sample preparation
The red sandstone used in the experiment was taken from a sloping site in Chongqing, and each group of sandstone samples was drilled from the same rock mass to ensure the relative uniformity of the sandstone. The sandstone rock samples were complete, uniform in texture, and strictly processed into standard cylinders with a diameter of 50 mm and a height of 100 mm by relevant regulations and standards. Measure the wave velocity of the considered sandstone, exclude samples with relatively large differences, retain samples with smaller differences, and group sandstones with similar wave velocities to reduce differences between samples.
2.2.2 Experimental design
In actual rock engineering, the water in contact with the rock is a hydro-chemical solution, and the ion composition in the solution is very complex. To study the effects of acidity and alkalinity on sandstone, it is necessary to consider the complex water environment’s impact on the rock (Cui et al., 2008; Zhang et al., 2022; Zhang et al., 2021). To present the process of hydro-chemical corrosion in a short period, a solution with a pH value higher or lower than the engineering groundwater is used in the experimental design to accelerate the experimental process.
Divide all sandstones into 5 groups, set different pH values, and prepare solutions with pH 3, 5, 7, 9, and 11 using a 0.1 mol/L HCl and NaOH solution. The pH error of the solution is ±0.1. To minimize experimental errors, the experiment was conducted at an ambient temperature of 20°C, with a time-sealed immersion for 21 days to simulate water chemical erosion conditions. The experimental samples are sandstone soaked in solutions of pH 3, pH 5, pH 7, pH 9, and pH 11.
The uniaxial compression test was conducted using the AG-250kNIS electronic precision material testing machine, with displacement control and a loading rate of 0.05 mm/min. The cyclic loading and unloading test was conducted using the MTS815 testing machine. MTS815 is a multifunctional electro-hydraulic servo controlled rigid testing machine specifically designed for rock and concrete experiments, produced by MTS Systems in the United States. It can test the deformation and strength characteristics as well as mechanical index parameters of rocks and concrete under various mechanical actions (uniaxial and triaxial), meeting the requirements of mechanical loading testing in this experiment. The lower limit of the load on the pH7 sample was kept constant at 2.5 MPa, and the upper limit stress increased by 2.5 MPa with each cycle level in the cyclic fatigue mechanics experiment. Perform cyclic loading and unloading stress-strain experiments on other samples, where the lower limit stress increases by 0.25 MPa per cycle level and the upper limit stress changes by 1 MPa per cycle level. Until the sandstone is destroyed, each stage is cycled 30 times with a frequency of 1 Hz.
Under uniaxial compression and cyclic loading and unloading conditions, the test results are processed to analyze changes in deformation parameters, changes in sandstone failure, study the degradation of sandstone mechanical properties under different acidic and alkaline environments and analyze the degradation of sandstone under acidic and alkaline erosion environments.
3 Analysis of experimental results
3.1 Comparison of specimens before and after erosion
Under the action of acid-base erosion, it has been observed that compared to the natural state, the surface of sandstone samples exhibits significant roughening and the formation of pores. This phenomenon indicates that the corrosive effect of acid-base solutions on rock samples progresses from the exterior towards the interior, with the outer surface of the rock samples being the first to undergo corrosion. Meanwhile, as the acidity and alkalinity of the solution increase, the surface roughness of the sample becomes more pronounced, the pore range expands, and the area increases (Meng et al., 2022; Yang et al., 2021). This indicates that the stronger the acidity and alkalinity of the solution, the higher the degree of corrosion the sample is subjected to.
3.2 Uniaxial compression test results and analysis
Based on the data obtained from uniaxial compression experiments, the mechanical parameters of sandstone soaked in different acid-base solutions were calculated, and the stress-strain curve of sandstone was plotted.
From the Table 1, it can be seen that the order of compressive strength of sandstone after soaking in different acid-base solutions is: natural state > pH7 > pH5 > pH9 > pH11 > pH3. As the acid-base properties of the solution increase, the compressive strength of the sandstone gradually decreases, and the peak strain of the sandstone gradually increases. The stronger the acidity and alkalinity of the solution, the greater the corrosive effect on sandstone. The corrosion effect of neutral to alkaline sandstone is weaker than that of an acidic environment. Macroscopically, it is manifested as a decrease in the rate of increase in peak strain of the sample compared to an acidic environment, but it has a certain corrosion effect on sandstone.
From Figure 7, it can be seen that the stress-strain curves of sandstone after soaking in different acidic and alkaline solutions exhibit consistency. According to the stress-strain curve of sandstone, it can be divided into three stages: compaction stage, elastic deformation stage, and yield failure stage (Li et al., 2020).
(1) Compression stage: At the beginning, sandstone bears relatively small stress, and small internal cracks are compacted. The stress-strain curve shows an upward concave shape, and the early stress and strain show a non-linear relationship. As shown in the figure, the natural concave segment is very short and quickly enters the elastic strain stage (Guo et al., 2021). The concave stage of the sample corroded by acid-base solution has increased compared to the natural state. The length of the concave and convex stages on the curve is related to the development of internal defects and voids. After soaking in different acidic and alkaline solutions, the internal structure of sandstone is damaged, and the pores increase. Macroscopically, it exhibits different corrosion effects, so the compaction stage of sandstone increases to varying degrees. From the graph, it can be seen that the initial elastic modulus of sandstone samples in a natural state is greater than that of sandstone samples soaked in acid-base solutions. Based on the above, it can be seen that the porosity of sandstone samples increases after chemical solution corrosion.
(2) Elastic deformation stage: In this stage, the primary cracks close, and the stress-strain curve can be approximated as a straight line, showing a linear variation (Han et al., 2013). After the sandstone is corroded, the elastic stage extends and the slope of the elastic stage decreases. After soaking in an acid-base solution, the elastic stage of the sandstone sample becomes shorter than in its natural state. With the increase of acid-base properties, the yield stress of the sandstone sample gradually decreases, and the sample enters the yield stage earlier, leading to greater degradation. Rock can be treated with solutions of different acidity and alkalinity, and the degree of deterioration can be reflected by the elastic modulus. The stronger the acidity and alkalinity, the more significant the degradation, the smaller the elastic modulus, and the neutral environment also has a certain effect on sandstone.
(3) Yield failure stage: At this stage, cracks begin to develop in sandstone, and small cracks gradually develop. The stress-strain curve shows a downward convex shape (Liu et al., 2014). After being subjected to acid-base environments, the yield failure stage of sandstone is more pronounced than in its natural state. In the natural state, the sample quickly reaches its peak after the elastic stage, while the sample without treatment in the yield stage is obvious. The peak strain of the processed sample has increased compared to the natural state, and the degree of degradation of the elastic modulus has also increased. In the failure stage, the stress drop is significant, and the failure rate of the specimen in its natural state is greater than that of the treated specimen. Cracking often occurs during destruction, and the stress decreases in a cliff-like manner without residual strength.
In its natural state, the peak stress of sandstone is 27.37 MPa. After soaking in solutions with pH values of 3, 5, 7, 9, and 11, the peak stress of sandstone is 12.68, 17.95, 20.99, 17.78, and 14.80 MPa, respectively, which is 53.67%, 34.41%, 23.31%, 35.04%, and 45.92% lower than that of natural sandstone. This indicates that the acid-base solution has a significant impact on the damage and deterioration of sandstone, and has created more micropores inside the sandstone, accelerating its degradation. The peak strain of the sample in the neutral solution has increased compared to the natural state, but the increase is smaller than in other solutions, indicating that the neutral solution can also affect the sample. Meanwhile, by comparing the experimental results under pH 3, pH 5, pH 9, and pH 11 conditions, it was found that acidic solutions have a greater impact on the mechanical properties of rocks than alkaline solutions.
3.3 Results and analysis of cyclic loading and unloading tests
3.3.1 Stress-strain relationship
The stress-strain curve obtained from cyclic loading and unloading mechanical tests with varying upper and lower limits on sandstone is shown in Figure 9.
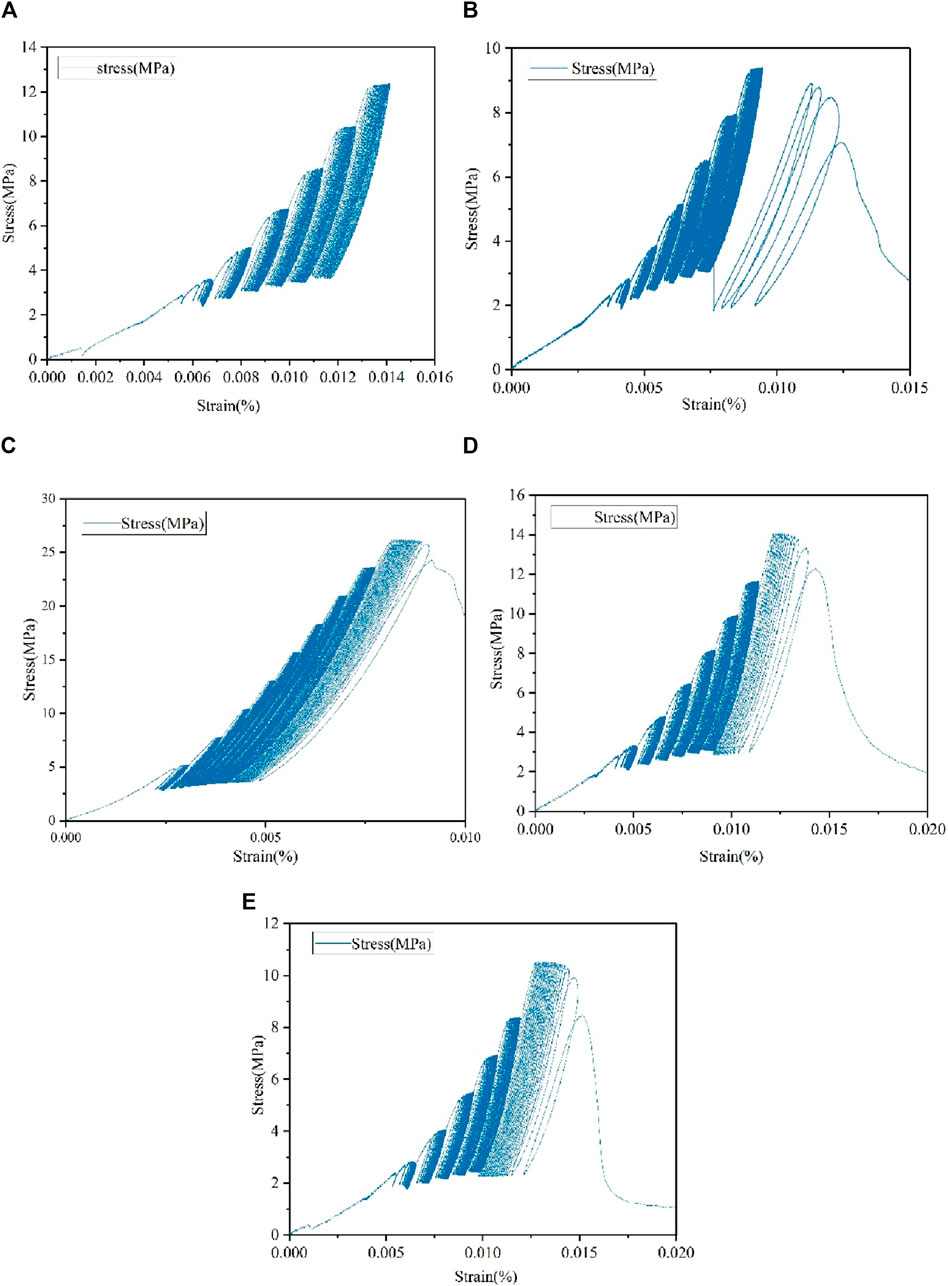
Figure 9. (A) Stress-strain curve at pH3. (B) Stress-strain curve at pH5. (C) Stress-strain curve at ph7. (D) Stress-strain curve at pH9. (E) Stress-strain curve at pH11.
From the Figure 9, it can be seen that under neutral conditions, the formation of internal fractures in sandstone is not obvious, and only a small amount of reaction occurs. Under weakly acidic conditions, the mechanical degradation of sandstone is not significant. This is because sandstone undergoes chemical reactions in acidic environments to inhibit further degradation of red sandstone, resulting in a less significant increase in peak strain. After corrosion by an acidic solution, it can be observed that due to the strengthening of acidity, the effect on sandstone becomes stronger, with a significant increase in internal pores and a significant decrease in elastic modulus. Under weakly alkaline conditions, the strain of sandstone has undergone significant changes, with the alkaline situation becoming more pronounced. This is due to reactions occurring inside the rock, increasing internal pores. The comparison results show that with the increase of the pH value of the soaking solution, the compaction and yield sections of the rock sample show a significant slowdown and elongation compared to neutral conditions, and the number of cycles of sandstone fracture decreases, leading to a decrease in peak strength.
Overall, the stress-strain curves of red sandstone in various states maintain high consistency, with significant changes compared to the uniaxial compression curve, indicating that the cyclic loading and unloading scheme promotes the development of internal cracks in the sample; After repeated loading and unloading and the coupling effect of acid-base solution, the deterioration of the rock sample becomes more pronounced (Li JC. et al., 2017).
3.3.2 Peak stress and peak strain
The peak stress and peak strain of samples soaked in different pH values are shown in the following figures.
From Figure 10, it can be seen that the sandstone soaked in pH 7 solution undergoes failure at the ninth level of cyclic loading. At the time of failure, the maximum stress is 26.18 MPa, accounting for 95.6% of the uniaxial compressive strength. From Figure, it can be seen that the sandstone undergoes failure at the seventh level of loading when soaked in pH 3, pH 5, pH 9, and pH 11 solutions. The maximum stress at failure is 9.40, 12.37, 7.18, and 5.36 MPa, respectively, accounting for 74.19%, 68.91%, 40.38%, and 36.21% of the uniaxial compressive strength. The peak stress of sandstone soaked in different solutions decreased, which is due to the promotion of internal crack propagation under multiple loading and unloading actions, resulting in a decrease in sample strength. Strong acidic and alkaline environments have a more significant impact on the strength degradation of sandstone and have a greater impact on its strength characteristics.
In neutral conditions, the peak stress under uniaxial compression is 27.37 MPa, and under cyclic loading and unloading conditions, the peak stress is 26.18 MPa, which is a decrease of 4.34% compared to uniaxial compression. This indicates that under loading and unloading, the yield stress gradually decreases and the sample strength slightly decreases.
From Figure 11, it can be seen that the solutions with pH = 3 and pH = 11 have the greatest impact on the peak strain of sandstone. This is because, in strongly acidic and alkaline environments, the degradation of the sample accelerates, leading to the gradual development of pores and an increase in strain.
3.3.3 Axial strain and loading unloading relationship
The axial strain and loading-unloading relationship of sandstone are shown in Figure 12.
As the number of loading and unloading increases, the samples soaked in different solutions show a linear growth trend. When the number of cycles is small, the axial strain remains at a lower level. As the load is applied and the number of loading and unloading increases, the axial strain rate is faster than before.
After soaking in an acid-base solution, the maximum axial strain of sandstone in each cycle stage is greater than that in a neutral environment. As the number of cycles increases, the axial strain continuously increases.
By comparing the two test methods of uniaxial loading and graded cyclic loading and unloading, it was found that during the cyclic loading and unloading test, the peak strength of sandstone showed a decreasing trend with the increase of the acidity and alkalinity of the soaking solution. The failure stress of sandstone under pH 3, pH 5, pH 9, and pH 11 solution soaking was 63.87%, 52.44%, 72.41%, and 79.56% lower than that of natural sandstone, respectively. Comparing the results of uniaxial compressive strength tests, it was found that the peak stresses of sandstone after soaking in pH 3, pH 5, pH 7, pH 9, and pH 11 solutions were 12.68, 17.95, 20.99, 17.78, and 14.80 MPa, respectively, which were 53.67%, 34.41%, 23.31%, 35.04%, and 45.92% lower than natural sandstone.
In the same acidic and alkaline environment, the peak strength of sandstone in cyclic loading and unloading tests is lower than that in uniaxial compression tests. This is due to the chemical corrosion effect of acid-base solutions on sandstone, and the fatigue damage caused by cyclic loading and unloading tests on sandstone, which accelerates the failure of sandstone. Macroscopically, the strength of sandstone is significantly reduced compared to its natural state (Shen et al., 2010).
4 Analysis of energy characteristics under cyclic loading and unloading
4.1 Calculation of energy for sandstone during the cycling process
In the stress-strain curve of cyclic loading and unloading, the loading curve, the enclosed area enclosed by the loading curve, vertical line, and horizontal axis is the total input energy; The enclosed area enclosed by the unloading curve, vertical line, and horizontal axis is the elastic performance, which is the released elastic performance stored in the rock; The area of the hysteresis loop enclosed by the loading curve, unloading curve, and horizontal axis is the dissipated energy density, which is the energy consumed by the internal damag e and plastic deformation of the rock.
In the formula:
The variation of the energy of sandstone under cyclic loading and unloading with the number of cycles is shown in Figure 10. In the early stage of cyclic loading, almost all input energy is converted into elastic energy and stored in the rock. At this stage, the damage to the rock is relatively small, and the dissipated energy is also relatively small. The total energy curve overlaps with the elastic energy curve, and the dissipated energy is almost zero; When rock damage occurs and cumulative damage increases, input energy is consumed, and the total energy curve does not coincide with the elastic energy curve, resulting in a gradual increase in dissipated energy. As the number of cycles increases, the dissipation energy grows faster and faster. At the beginning of rock loading, the energy change is not significant, and with the increase of stress, the dissipated energy rapidly increases, resulting in more severe damage; The rapid increase in dissipated energy in the final stage indicates an increase in the energy required for damage, leading to a greater degree of rock failure.
The energy variation curves of sandstone with different pH values and cyclic levels are shown in Figure 13.
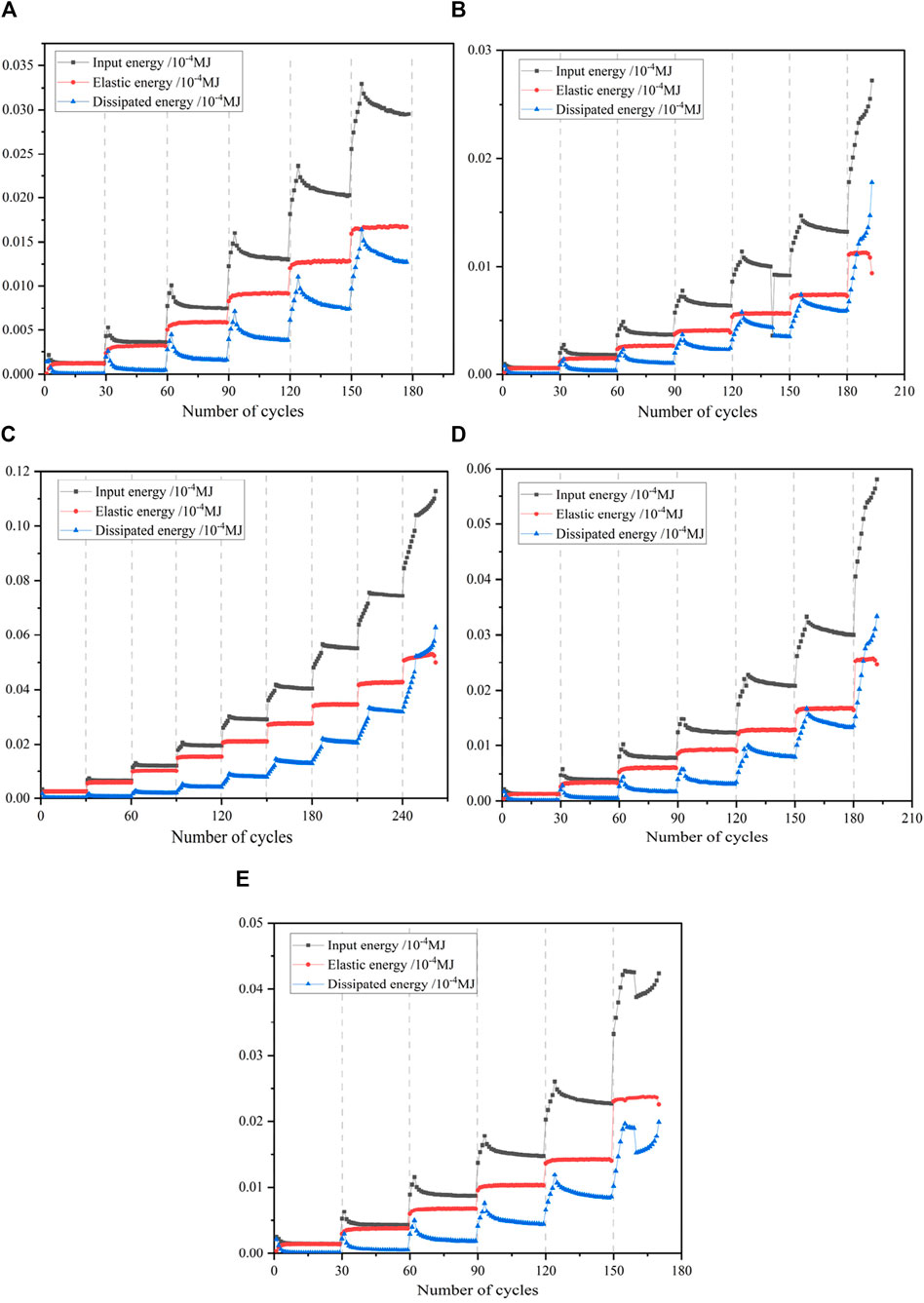
Figure 13. (A) Energy variation of sandstone at pH3. (B) Energy variation of sandstone at pH5. (C) Energy variation of sandstone at pH7. (D) Energy variation of sandstone at pH9. (E) Energy variation of sandstone at pH11.
Table 2 shows the strain energies of red sandstone at peak points under different pH water chemical solution states. As shown in the table, the strain energy of sandstone after corrosion by different pH water chemical solutions shows varying degrees of reduction compared to the natural state, indicating that different water chemical solutions have a significant impact on the strain energy of sandstone samples. According to numerical calculations, the
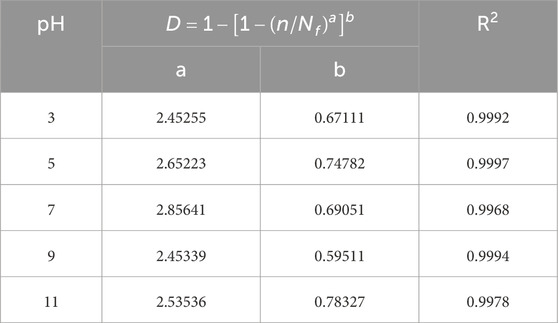
Table 3. Fitting relationship between cumulative damage and relative cycle during rock sample deformation process.
The cumulative dissipated energy in this context refers to the total energy consumed by the rock specimen during the process of cyclic loading due to internal damage, plastic deformation, and crack propagation. This energy is not fully released during the unloading process but is dissipated as heat, sound, or other forms of energy, leading to the gradual degradation of the material’s performance. In fatigue testing, the cumulative dissipated energy is a crucial parameter as it is directly related to the material’s fatigue life and the accumulation of fatigue damage. The higher the cumulative dissipated energy, the more severe the fatigue damage to the material, and consequently, the shorter its lifespan. The energy curve during the loading phase is shown in Figure 14. As the level of cyclic loading increases, the input energy is converted into elastic performance and dissipated energy, and the proportion of dissipated energy increases, leading to an increase in cumulative damage and an increase in dissipated energy. During the fatigue deformation process of rocks, most of the energy is dissipated, leading to the full development of internal damage.
4.2 Analysis of damage evolution characteristics of sandstone
Rock is a typical brittle material with non-uniformity, discontinuity, and nonlinear characteristics. Under cyclic loading, the mechanical properties of rock materials gradually weaken and their strength gradually decreases, exhibiting a process of gradual damage. Theoretical analysis of the damage evolution process of rock materials and prediction of rock failure trends plays an important role in evaluating the stability of engineering rock masses. Damage refers to the phenomenon that under monotonic or cyclic loading, the micro defects of a material weaken its progressive cohesion, leading to the failure of volume units and the deterioration of the macroscopic mechanical behavior of the material (Wang et al., 2022).
For rock damage caused by fatigue loading tests, a fatigue damage variable that reflects the cumulative damage evolution during the fatigue loading process is the key to predicting fatigue instability. Different physical and mechanical parameters are used to define damage variables, such as ultrasonic velocity, elastic modulus, acoustic emission, density, hardness, resistance, dissipation energy, etc. At present, most damage variables defined from an energy perspective are based on dissipated energy. Xu et al. (2019) believe that the accumulation of dissipated energy can characterize the accumulation of damage, and define the ratio of the accumulated dissipated energy in the i-cycle to the accumulated dissipated energy in the final state of the damage variable as the damage variable; Yang et al. (2019) characterized the current damage development of rocks using the energy consumption ratio of the i-th cycle. Zhang et al. (2017) defined the ratio of the accumulated dissipated energy Nd in the i-cycle to the total input energy in the final stage as the damage variable. The calculation method for the damage D of sandstone in this article adopts the dissipative energy method: according to the principle of energy conservation, the damage and failure of materials are caused by the continuous accumulation of dissipative energy. The ratio of cumulative dissipative energy to cumulative total strain energy is defined as the damage D.
The formula for calculating cumulative dissipated energy is:
In the formula:
The formula for calculating the cumulative total strain energy is:
In the formula:
In the formula:
Calculate the damage variable at the end of each cycle loading level and draw a damage evolution diagram as shown in Figure 15. The results indicate that rock damage is greater after being corroded by a hydrochemical environment. The fitting curve shows a trend of stable increase followed by an accelerated increase, indicating that hydrochemical environmental treatment has a significant impact on the fatigue life of rocks. Propose a new damage accumulation model based on energy dissipation and release to describe the degradation process of red sandstone. The form of the proposed function is:
In the formula: D represents the damage caused by cyclic loading, N represents the number of loading cycles,
As the cyclic level increases, the slope of the curve gradually increases, indicating that the degree of sandstone damage is getting higher and the damage growth is getting faster, which can better reflect the damage evolution process of sandstone under cyclic loading and unloading. During the cycling process, damage gradually accumulates and rapidly increases as damage approaches.
4.3 Sandstone failure mode
Figure 16 shows the failure mode of sandstone samples under hydrochemical erosion conditions. As shown in the figure, the failure modes of sandstone under hydrochemical erosion conditions are different. Under natural conditions and pH = 7, sandstone specimens mainly undergo splitting failure, with macroscopic cracks penetrating the specimen along the central axis; When pH = 5, the sandstone sample begins to exhibit shear failure, with macroscopic cracks forming a shear angle of approximately 75° with the central axis; When pH = 3, the sandstone sample undergoes X-shaped failure, with shear failure particularly evident at the top and bottom of the sample; When pH = 9, the sandstone sample mainly exhibits shear failure, with macroscopic cracks forming a shear angle of about 85° with the central axis; When pH = 11, the sandstone sample undergoes shear and splitting failure, and the sample is more severely damaged. Therefore, with the increase of acid-base strength, the failure of sandstone samples transitions from axial splitting failure to shear failure, and even fracture occurs.
5 Discussion
The specimen was subjected to uniaxial loading and cyclic loading and unloading experiments to study the rock failure process under high stress. In actual tunnel operation, rocks are subjected to dynamic loads such as blasting and mechanical vibration, as well as corrosion from chemical water environments. The stress disturbance acting on rocks is not constant, but variable, so the applied load is not a constant stress load, but a graded cyclic load with varying upper and lower limits. We studied the stress-strain relationship and found that corrosion by aqueous chemical solutions can affect the strain of rocks. The stronger the acidity and alkalinity, the greater the axial strain. We studied the characteristics of energy dissipation and release and found that hydrochemical solutions have a significant impact on the mechanical properties and energy evolution characteristics of rocks. Rocks are more prone to structural damage under acid-base corrosion conditions. The input energy required for failure of the processed rock decreases, and the proportion of dissipated energy continues to increase. Therefore, the damage variable is defined based on the input energy dissipated energy. From the rock failure morphology, it can be seen that the stronger the acidity and alkalinity, the higher the degree of fracture, and the higher the dissipation energy, which promotes the increase of cracks. The failure mode of the corroded specimen ultimately resulted in multiple axial cracks, and after chemical corrosion, the failure characteristics and methods were basically the same.
The damage characteristics of rocks under multi-stage cyclic loading and unloading conditions are different from those of Li et al. (2021), Miao et al. (2021), and others. In a cyclic loading level, the damage steadily increases. In the final loading stage, there is a sudden increase in damage accumulation. The damage of rock under multi-stage cyclic loading can be divided into two stages. In the first few loading stages, microcracks are compacted, and most of the input energy is converted into elastic energy for storage. The energy consumed by internal damage or deformation only accounts for a small part. The stored large amount of elastic energy is rapidly released, causing the stress of the sample to rapidly decrease, leading to the rapid expansion of internal cracks until failure. With the development of cracks, the dissipation energy increases, the crack evolution is obvious, and the rate of increase accelerates. So when rocks approach failure, the dissipated energy transforms from a steady increase to a rapid increase, which is a precursor to rock fracture. With the increase of loading and unloading times, the expansion and connection of cracks, the energy of crack propagation and penetration increases faster, and the elastic energy is transformed into dissipated energy.
The evolution of rock damage in this article is different from the three-stage trend of deceleration accumulation, stable accumulation, and rapid accumulation proposed by Li X W. The rock damage model based on energy dissipation and release established in this article takes into account the effect of chemical erosion on rocks and the accumulation of damage under cyclic loading. The results indicate that the model is in good agreement with experimental data. The loading frequency of the rock in this experiment is constant, and the upper and lower limits of cyclic stress change. In further research, the energy dissipation of rocks under frequency variation conditions and their degradation mechanisms in more complex hydrochemical environments should be studied.
6 Conclusion
This article combines the characteristics of tunnel rock mass under high stress to study the mechanical properties of red sandstone under uniaxial loading and cyclic loading and unloading conditions. The main conclusions are as follows:
(1) Explored the mechanical properties of red sandstone under uniaxial loading in acidic and alkaline environments. The peak stresses of sandstone soaked in pH 3, pH 5, pH 7, pH 9, and pH 11 solutions were 12.68, 17.95, 20.99, 17.78, and 14.80 MPa, respectively, which were 53.67%, 34.41%, which were lower than natural sandstone.
(2) Explored the fatigue characteristics of red sandstone under cyclic loading and unloading in acidic and alkaline hydrochemical environments. Sandstone underwent damage at the 7th level of loading after soaking in pH 3, pH 5, pH 9, and pH 11 solutions. The maximum stresses during failure are 9.40 MPa, 12.37, 7.18, and 5.36 MPa, respectively. As the pH of the soaking solution increases, the number of cycles of sandstone fracture decreases, and the peak strength also decreases. Under the coupled uniaxial and cyclic loading and unloading conditions of hydrochemical solutions, the degradation of sandstone gradually becomes significant with the increase of acidity and alkalinity, and the number of cycles, accelerating the development of pores in the sample. The fatigue damage caused by cyclic loading and unloading results in higher uniaxial compressive strength than uniaxial loading and unloading.
(3) Hydrochemical erosion has a significant impact on the energy evolution characteristics of sandstone. The total input energy, elastic energy, and dissipated energy change with pH value, and an increase or decrease in pH will cause them to decrease, and the energy gradually decreases with the enhancement of acidity and alkalinity.
(4) Establishing a sandstone damage degree model under hydrochemical erosion conditions can better reflect the damage degree of sandstone caused by acid-base and cyclic loads, and reflect the process of damage accumulation.
Data availability statement
The original contributions presented in the study are included in the article/supplementary material, further inquiries can be directed to the corresponding author.
Author contributions
TP: Data curation, Writing–original draft. DR: Conceptualization, Investigation, Writing–review and editing. FH: Formal Analysis, Methodology, Writing–review and editing. BL: Data curation, Formal Analysis, Writing–review and editing. FW: Methodology, Writing–review and editing. HZ: Validation, Visualization, Writing–review and editing.
Funding
The author(s) declare that financial support was received for the research, authorship, and/or publication of this article. This work is supported by Major R&D Projects of China Metallurgical (YFXM-20230410-0001).
Conflict of interest
Authors TP, DR, FH, and BL were employed by Chengdu Surveying Geotechnical Research Institute Co., Ltd. of MCC.
The remaining authors declare that the research was conducted in the absence of any commercial or financial relationships that could be construed as a potential conflict of interest.
Publisher’s note
All claims expressed in this article are solely those of the authors and do not necessarily represent those of their affiliated organizations, or those of the publisher, the editors and the reviewers. Any product that may be evaluated in this article, or claim that may be made by its manufacturer, is not guaranteed or endorsed by the publisher.
References
Chen, B. R., Feng, X. T., Yao, H. Y., and Xu, S. C. (2010). Study on mechanical behavior of limestone and simulation using neural network model under different water-chemical environment. Rock Soil Mech., 31(4): 1173–1180. doi:10.16285/j.rsm.2010.04.046
Cui, Q., Feng, X. T., Xue, Q., Zhou, H., and Zhang, Z. H. (2008). Mechanism study of porosity structure change of sandstone under chemical corrosion. Chin. J. Rock Mech. Eng., 27(6): 1209–1216.
Guo, H., Ji, M., Sun, Z., and Zhou, Z. (2021). Energy evolution characteristics of red sandstone under cyclic load. J. Min. Strat. Control Eng., 3: 15–23. doi:10.13532/j.jmsce.cn10-1638/td.20211008.001
Han, T. L., Chen, Y. S., Shi, J. P., Yu, Z., and He, M. M. (2013). Experimental study of mechanical characteristics of sandstone subjected to hydro chemical erosion. Chin. J. Rock Mech. Eng., 32(S2): 3065–3072.
Li, G. L., Yu, L. Y., Jing, H. W., Su, H. J., Zhang, T., and Li, M. (2017a). Experimental study of dynamic compressive mechanical properties of limestone after acid corrosion. Rock Soil Mech., 38(11): 3247–3254. doi:10.16285/j.rsm.2017.11.021
Li, J. C., Xu, Y., and Li, P. J. (2017b). Characteristics of hysteresis of sandstone deformation under constant and cyclic loading. Sci. Technol. Eng.
Li, L. F., Zhang, X. H., Deng, H. L., and Han, L. P. (2020). Mechanical properties and energy evolution of sandstone subjected to uniaxial compression with different loading rates. J. Min. Strata Control Eng., 2(4): 043037. doi:10.13532/j.jmsce.cn10-1638/td.20200407.001
Li, X. W., Yao, Z. S., Huang, X. W., Liu, Z. X., Zhao, X., and Mu, K. H. (2021). Investigation of deformation and failure characteristics and energy evolution of sandstone under cyclic loading and unloading. Rock Soil Mech. doi:10.16285/j.rsm.2020.1463
Liu, X. R., Zhang, L., and Fu, Y. (2014). Experimental study of mechanical properties of argillaceous sandstone under wet and dry cycle in acid environment. Rock Soil Mech., 35(2): 45–52. doi:10.16285/j.rsm.2014.s2.003
Liu, Y., Li, J., and Wu, Y. (2017). Study on the effect of acidic environment on mechanical properties of rock. Sci. Technol. Eng., 17: 196–201.
Liu, Y., Yang, G., Wang, J. X., and Jiang, A. N. (2018). Fatigue deformation characteristics of limestone under the combined action of hydro chemical solution and cyclic loading[J]. Dalian City, Liaoning Province: Journal of Dalian Maritime University.
Liu, Y. S., Zou, J. Y., Wu, Q. L., Li, J., et al. (2019). Properties of layered composite rock under chemical corrosion. J. Southwest Univ. Sci. Ed. doi:10.13718/j.cnki.xdzk.2019.02.017
Meng, Q. B., Liu, J. F., Xie, L. X., Pu, H., Yang, Y. G., Huang, B. X., et al. (2022). Experimental mechanical strength and deformation characteristics of deep damaged–fractured rock. Bull. Eng. Geol. Environ. 81, 32–27. doi:10.1007/s10064-021-02529-3
Miao, S. J., Cai, M. F., and Ji, D. (2016). Damage effect of granite’s mechanical properties and parameters under the action of acidic solutions. J. China Coal Soc., 41(4): 829–835. doi:10.13225/j.cnki.jccs.2015.0845
Miao, S. J., Liu, Z. J., Zhao, X. G., and Huang, Z. J. (2021). Energy dissipation and damage characteristics of Beishan granite under cyclic loading and unloading. Chin. J. Rock Mech. Eng., 40(05): 928–938. doi:10.13722/j.cnki.jrme.2020.0953
Michael, P. E. (1998). Time- variant reliability of reinforced concrete bridges under environmental attack[D]. Denver: University of Colorado.
Pan, J., Cai, M., Li, P., and Guo, Q. f. (2022). A damage constitutive model of rock-like materials containing a single crack under the action of chemical corrosion and uniaxial compression. J. Central South Univ. 29 (2), 486–498. doi:10.1007/s11771-022-4949-1
Rong, H., Wang, H., Cao, H., Wang, Z. S., Wang, D. L., and Feng, M. M. (2019). Influence of pH value on mechanical properties and microstructure of sandstone[J]. J. Yangtze River Sci. Res. Inst., 36(3): 116.
Shen, L. F., Feng, X. T., Pan, P. Z., and Zhou, H. (2010). Experimental research on mechano-hydro-chemical coupling of granite with single fracture. Chin. J. Rock Mech. Eng. 29 (7), 1379–1388.
Stewart, M. G., and Val, D. V. (1999). Role of load history in reliability based decision analysis of aging bridges. J. Struct. Eng. 125 (7), 776–783. doi:10.1061/(asce)0733-9445(1999)125:7(776)
Wang, W, Liu, T G, Lu, J, et al. (2012). Experimental study of influence of water-rock chemical interaction on mechanical characteristics of sandstone. Chin. J. Rock Mech. Eng. 31(a02): 3607-3617.
Wang, J., Yang, Y., Ding, J. W., and Diao, X. H. (2022). Study on failure and acoustic emission characteristics of sandstone under incremental loading and unloading. J. Railw. Sci. Eng. 19 (06), 1605–1615. doi:10.19713/j.cnki.43-1423/u.t20210599
Wang, Z. J., Fu, Y., and Liu, X. X. (2016). Erosion analysis of argillaceous sandstone under dry-wet cycle in two pH conditions. Rock Soil Mech., 37(11): 3231–3239. doi:10.16285/j.rsm.2016.11.024
Xu, Y., Li, C. J., Zheng, Q. Q., et al. (2019). Analysis of energy evolution and damage characteristics of mudstone under cyclic loading and unloading. Chin. J. Rock Mech. Eng. 38 (10), 2084–2091. doi:10.13722/j.cnki.jrme.2019.0153
Yang, J. X., Luo, M. K., Zhang, X. W., Huang, N., and Hou, S. J. (2021). Mechanical properties and fatigue damage evolution of granite under cyclic loading and unloading conditions[J]. J. Min. Strata Control Eng., 3(3): 033016. doi:10.13532/j.jmsce.cn10-1638/td.20210510.001
Yang, X. B., Cheng, H. M., Lv, J. Q., Hou, X., and Nie, C. G. (2019). Energy consumption ratio evolution law of sandstones under triaxial cyclic loading. Rock Soil Mech. doi:10.16285/j.rsm.2018.2166
Yu, J., Cai, Y. Y., Liu, S., Zhang, X., Tu, B. X., and Fu, G. F. (2019). Microscopic damage and mechanical properties degradation of sandstone under the combined effect between water chemical corrosion and freeze-thaw cycles. Yantu Lixue/Rock Soil Mech., 40(2):454–464. doi:10.16285/j.rsm.2017.1450
Zhang, G. H., Ou Yang, Z. H., Deng, Z. G., Yin, W., and Bo, L. (2017). Study on energy dissipation and damage evolution of bump proneness coal under cyclic loadings. Coal Sci. Technol. doi:10.13199/j.cnki.cst.2017.02.010
Zhang, X. W., Xu, J. H., Huang, N., Sun, L., and Cao, Y. (2022). Mechanical properties and energy damage characteristics of sandstone subjected to hydro chemical erosion. J. Min. Strat. Control Eng., 4: 79–89. doi:10.13532/j.jmsce.cn10-1638/td.20220915.001
Keywords: red sandstone, fatigue loading, tunnel safety maintenance, damage characteristics, hydro-chemical environment
Citation: Peng T, Ren D, He F, Li B, Wu F and Zhou H (2024) Study on fatigue characteristics of red sandstone under extremely high stress in the hydro-chemical environment. Front. Earth Sci. 12:1453080. doi: 10.3389/feart.2024.1453080
Received: 22 June 2024; Accepted: 12 August 2024;
Published: 02 September 2024.
Edited by:
Weiyao Guo, Shandong University of Science and Technology, ChinaReviewed by:
Yixian Wang, Hefei University of Technology, ChinaQian Yin, China University of Mining and Technology, China
Copyright © 2024 Peng, Ren, He, Li, Wu and Zhou. This is an open-access article distributed under the terms of the Creative Commons Attribution License (CC BY). The use, distribution or reproduction in other forums is permitted, provided the original author(s) and the copyright owner(s) are credited and that the original publication in this journal is cited, in accordance with accepted academic practice. No use, distribution or reproduction is permitted which does not comply with these terms.
*Correspondence: Fei Wu, wufei3616@cqu.edu.cn