- 1Beijing Earthquake Agency, Beijing, China
- 2China Earthquake Networks Center, Beijing, China
- 3The Bureau of seismology of Yanqing District of Beijing, Beijing, China
Hydrological changes in groundwater coupled with earthquakes had been documented in previous studies by global researchers. Although few reports investigate multiple geochemical parameters that respond to earthquakes, trace elements received less attention, whereas they were suggested to be more sensitive to small earthquakes than the commonly used geochemical parameters. Beijing is located in the Zhangjiakou-Bohai (Zhang-Bo) seismic belt of North China, and although the occurrence of small earthquakes is frequent, the great historic earthquake in the Sanhe-Pinggu area M8 in 1679 in the adjoining southeast of Beijing gained widespread public attention. To find effective precursors that are significant for operational earthquake forecasting of the Beijing area, we carried out a one year test research project through weekly collection of groundwater samples during June 2021 to June 2022 from the seismic monitoring well of Wuliying in northwest Beijing. The 41 trace elements chemical compositions were analyzed for each sample. During the project ongoing period, the biggest earthquake with a magnitude of ML3.3 occurred in the Chaoyang District of Beijing on 3 February 2022. The content changes in these trace elements were systematically monitored before and after the earthquake. Through retrospective research, it was found that a few sensitive trace elements were anomalous to be coupled to the earthquake, including Li, Sc, Rb, Mo, Cs, Ba, W, U, Sr, Mn, Ni, and Zn. In addition to trace elements, we examined stable isotopes of hydrogen and oxygen and the existing hydrological data on groundwater level, temperature, major ions, and gases to assess the validity of geochemistry as a monitoring and predictive tool. We only found that F- (fluorine) ions and He (helium) gas had apparent shifts related to the earthquakes, while no shifts in the groundwater level were observed. Such characteristics of multiple geochemical parameters indicate that trace elements are likely to be more sensitive to crustal strain than the groundwater level and major ions. We assumed a most likely mechanism of the combination of mixing and water–rock interactions to explain the phenomenon. The probable scenario was that minor stresses caused by the earthquakes might create micro-cracks in bedrocks, thereby leading to a small volume of chemically distinct water mixing with the original water of the aquifer, and finally, the earthquake-induced rock fractures enhance the water–rock interactions, resulting in the post-seismic recovery of trace elements and δ18O value migration to the GWML. More testing works to find other sensitive sites to investigate multiple geochemical characteristics aiming at long-term to short-term earthquake prediction in the Beijing area and Zhang-Bo seismic belt are in progress.
1 Introduction
As part of the earthquake precursor detection program, changes in groundwater levels, temperatures, and chemical parameters related to earthquakes have been documented for decades (Toutain et al., 1997; Roeloffs, 1998; Montgomery and Manga, 2003; Wang and Manga, 2010; Skelton et al., 2014; Manga and Wang, 2015; De Luca et al., 2018; Martinelli, 2020). Because of the feasibility of using automated monitoring systems, earthquake-related changes in water levels, temperatures, and radon concentrations are the most commonly reported responses (King, 1981; Kitagawa et al., 1996; Manga and Wang, 2015; Martinelli, 2020). These monitoring methods of groundwater also play key roles for precursor detection in China, and a nationwide network of monitoring wells located along strain-sensitive locations has been constructed for this purpose.
Comparisons with conventional hydrological parameters such as water level and temperatures, geochemical changes induced by earthquakes have become increasingly important (Martinelli, 2020; Claesson et al., 2004) because geochemical changes are considered to be sensitive to crustal stress and beneficial for earthquake prediction (Thomas, 1988; Wakita, 1996; Poitrasson et al., 1999; Manga and Wang, 2015; Martinelli and Dadomo, 2017; Shi et al., 2020; Kopylova et al., 2022; Chen and Liu, 2023). For example, based in part on hundreds of hydrological anomalies including geochemical changes, an imminent prediction was made before the 1975 M7.3 Haicheng earthquake in China (Wang et al., 2006). Following the 1995 M7.2 Kobe earthquake, several papers reported precursory changes in the concentrations of radon, chlorine, and sulfate ions in the groundwater (Tsunogai and Wakita, 1995; Igarashi et al., 1995). Claesson et al. (2004) observed simultaneous changes in trace elements, major elements, and isotope before and after major earthquakes. Skelton et al. (2019) found pre-seismic changes in five parameters (Na, Si, K, δ18O, and δ2H) before three earthquakes and the post-seismic changes in eight parameters (Ca, Na, Si, Cl, F, SO4, δ18O, and δ2H).
Among these geochemical parameters, trace elements were thought to be more sensitive to earthquakes. For example, Rosen et al. (2018) showed that trace elements (Al, Cu, Pb, Mn, and Sr) exhibited post-seismic peak changes in four hot springs but noted only small changes for the major ions. Barberio et al. (2017) documented another study on changes in trace elements (As, Fe, V, and Cr) that were associated with the seismic sequence, but the study showed no changes for the major ions (K, Na, Ca, Mg, and Cl). Shi et al. (2020) showed that trace element concentrations had significant decreases in response to the Tonghai earthquake but no significant changes in major ions and hydrogen and oxygen isotope concentrations. However, trace element changes induced by earthquakes have been documented relatively rarely (Martinelli, 2020; Claesson et al., 2004) because manual sampling and expensive and time-consuming laboratory analyses are required (Wang and Manga, 2010; Shi et al., 2020). Another problem is to understand which geochemical elements would be good candidates for monitoring and prediction. For this purpose, Chen and Liu (2023) continuously collected water samples for a whole year and found that Li, Sc, Ti, Pb, Cu, Nb, Th, Zn, Tl, U, and REEs responded to small earthquakes in Beijing, China.
Overall, most documented studies have used the major elements or single species geochemical indicators, and they respectively examined the major elements (Tsunogai and Wakita, 1995; Toutain et al., 1997; Kingsley et al., 2001; Woith et al., 2013), hydrogen and oxygen isotopes (Taran et al., 2005; Skelton et al., 2014), and trace elements (Chen and Liu, 2023) related to earthquakes. Few reports investigate the characteristics of multiple geochemical parameters’ response to earthquakes.
In this paper, we continuously collected water samples of the Wuliying well and analyzed multiple geochemical parameters including trace elements and hydrogen and oxygen isotopes, combining existing data on the groundwater level, temperature, major ions, and gases, to examine comprehensive hydrological changes related to earthquakes, discuss the possible mechanisms, and assess the sensitivity and validity of multi-geochemistry as a predictive tool in the future.
2 Background of geological settings
2.1 Faults and earthquakes in and around Beijing
Beijing is the capital of China with a large population, and it is located in the intersection of the North China Plain and the Zhang-Bo (Zhangjiakou-Bohai) seismic belt. North China has experienced strong earthquakes frequently over the last few decades (Chen and Liu, 2023), such as the Xingtai M7.2 earthquake in 1966, Bohai M7.4 earthquake in 1969, Haicheng M7.3 earthquake in 1975, Tangshan M7.8 earthquake in 1976, and Zhangbei M6.2 earthquake in 1998. The Zhang-Bo seismic belt is a group of NW–W orderly active fault zones, which starts from the northern margin of Taihang Mountain in the west and enters the Bohai Sea in the east. It is an important NW seismic activity zone with high seismic activity in North China (Yang et al., 2022). Hence, there is potential risk and danger of damaging earthquakes at Beijing in the following years.
Beijing also developed regional active faults, mainly including the NW orderly Nankou-Sunhe fault zone and two groups of NE orderly active fault zones named the Huangzhuang-Gaoliying fault zone and Shunyi-Liangxiang fault zone (Figure 1). All earthquakes occur along these fault zones or where they intersect (Figure 1), and almost all of these earthquakes were less than M3. Therefore, it is of great scientific and practical significance for small earthquakes monitoring and prediction in the Beijing area.
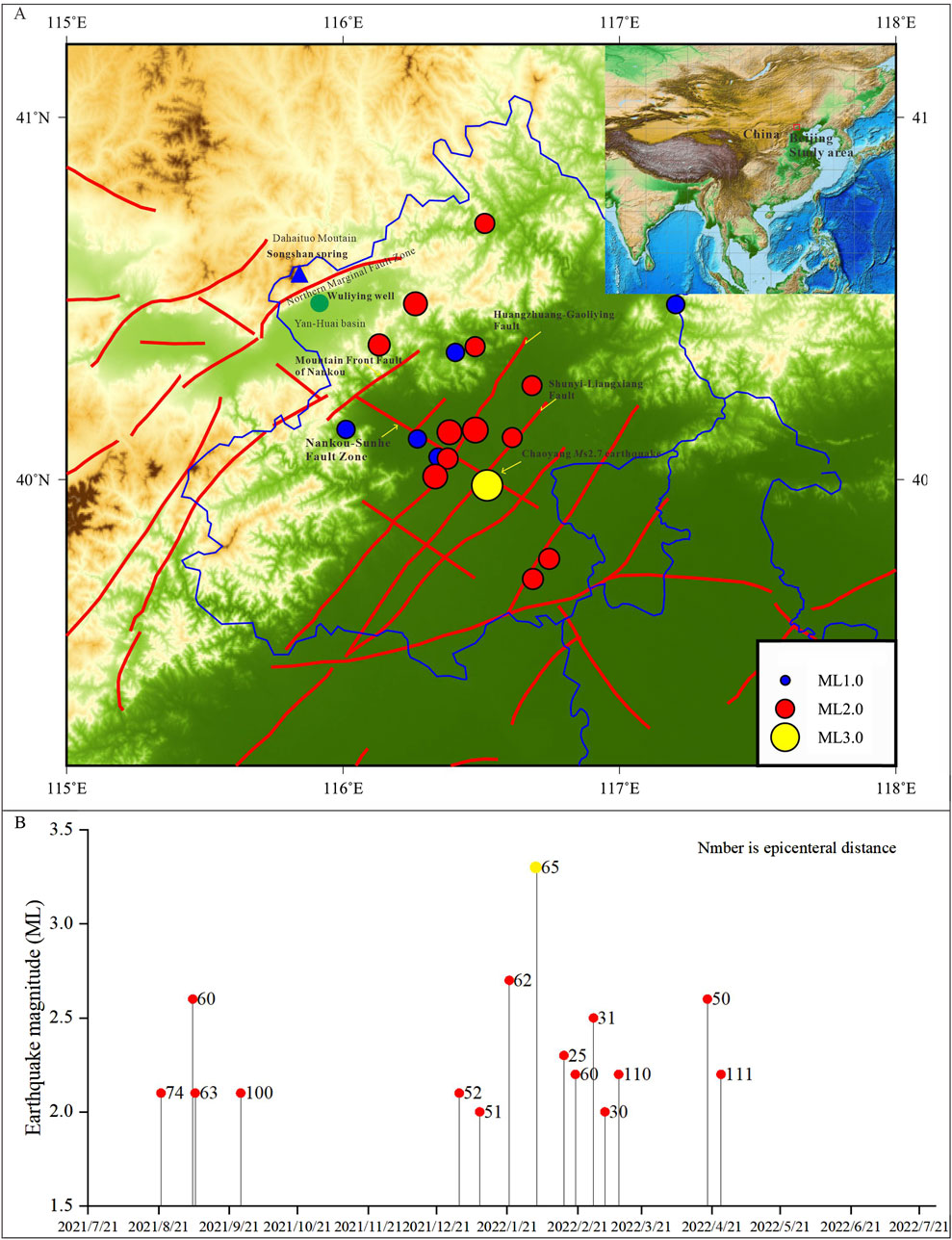
Figure 1. Earthquake distribution (A) of the Beijing area from June 2021 to June 2022 and the time sequence of earthquakes (B) above M1.0 (modified from Chen and Liu, 2023). The biggest yellow circle is Chaoyang M2.7 (ML3.3) earthquake, and the blue triangle and green circle were location of the Songshan hot spring and Wuliying monitoring well, respectively.
To better monitor the seismic activity in the capital area, the Chinese Earthquake Administration (CEA) started to deploy the Beijing metropolitan digital Seismic Network (BSN) in the late 90s. Currently, the network consists of broadband, borehole, and surface short-period stations that cover Beijing and the surrounding areas densely and uniformly (Jiang et al., 2008; Li and Huang, 2014). Since 2001, 107 stations have been installed in the Chinese capital region, which is the most advanced and densest digital seismic network in Mainland China. A large number of high quality waveforms have been recorded, which provides an opportunity to improve the location accuracy of small earthquakes in the region (Jiang et al., 2008).
Beijing hosted the 2022 Winter Olympic Games from February 4 to 20. For security purpose, continuous monitoring of possible earthquake areas had been strengthened, which provided an opportunity to investigate trace element variation during this time. There were approximately 400 earthquakes that were recorded in Beijing during the study period (Figure 1A), and only 22 of them were above M1.0. On 3 February 2022, one of the biggest earthquakes with a magnitude of ML3.3 occurred in the Chaoyang District of Beijing (Figure 1). Furthermore, there were 10 earthquakes above M1.5 that occurred densely during January to March 2022 (Figure 1B), but spatial distribution of these earthquakes was not clustered and spread along the NW orderly Nankou-Sunhe fault and NE orderly Huangzhuang-Gaoliying fault (Figure 1).
2.2 Hydrogeological condition of the Wuliying well and the monitoring techniques
The Wuliying well is located in the north of Wuliying village, Yanqing District, Beijing. The well is 2 km west of the Yanqing seismic monitoring station and has an epicentral distance of ∼65 km from the Chaoyang earthquake (Figure 1). Geologically, the Wuliying well is located in the Yan-Huai (Yanqing-Huailai) sediment basin, where lies in the junction area of Yanshan Mountain and Zhang-Bo seismic belt. The northern marginal fault zone of the Yan-Huai Basin is 3 km northwest of the well, and pressure faults have developed around the well area, so the structural site of the well is sensitive to stress changes.
The Wuliying well was drilled in 1984 with a depth of 533 m, and the lithology consists of Quaternary sediment thickness of 330 m, including clay, sand, and gravel. Below the sediment are Jurassic basalt, then Sinian (Precambrian) limestone, and dolostone (Figure 2). The wellbore is open to Sinian dolostone from 505 m to the bottom of the well, and the only water inlet is at carbonate conditions, including dolomite and calcite. The well is a flowing artesian geothermal well with a flow rate 2.5 L/s, temperature 33.4°C, pH 7.3, and the water type is HCO3–Na (Chen, 2023).
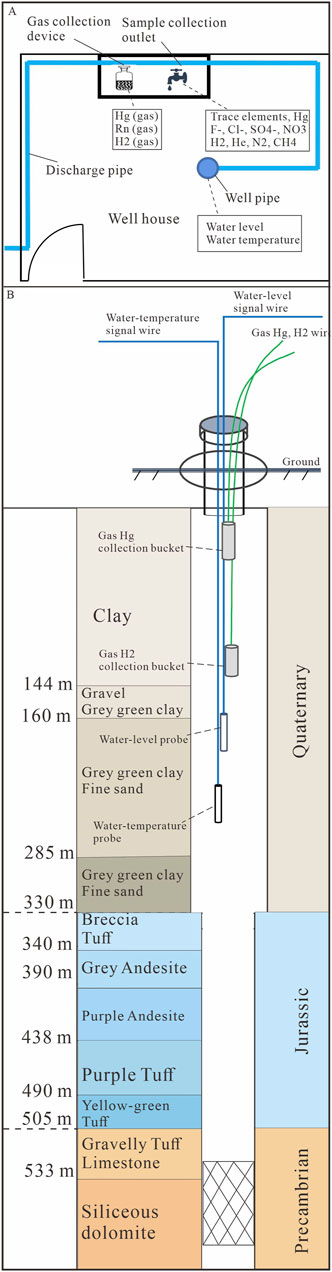
Figure 2. Distribution of gas and water sample gathering devices of the Wuliying well (A) and the lithology of the wellbore (B). The grid-like section in (B) indicates the screen section in the well.
The Wuliying seismic monitoring well in Yanqing district, Beijing has established continuous and long-term monitoring systems on comprehensive hydrological parameters, including water level, temperature, Hg (mercury), Rn (radon), major ions, and gases (dissolved and degassing gases from water). Comprehensive monitoring methods have been measured daily in the Wuliying well for decades, including the water level, deep water temperature, four major ions (F−, Cl−, SO4−, and NO3−), five dissolved gases in water (Hg, H2, He, N2, and CH4), and three degassing gases (Hg (gas), Rn (gas), and H2 (gas)) (sample gathering device in Figure 2). The sufficient data provided an important foundation for earthquake monitoring and prediction in Beijing. In this research, we compare the seismic sensitivity of trace elements to all geochemical parameters already available of the Wuliying wells. Hence, the Wuliying well provided an opportunity to investigate multiple parameter variations coupled to earthquakes.
In addition, the Songshan hot spring is ∼10 km from the Wuliying well. The spring is an artesian geothermal type, and the water comes from the granite rock body of Dahaituo Mountain that is also near the northern marginal fault of the Yan-Huai Basin (Figure 1). Investigation of trace elements in the Songshan spring had been first documented in detail by Chen and Liu, 2023.
3 Methodology
3.1 Data collection
The groundwater level and deep water temperature observation of the Wuliying well are usually recorded at 1 minute interval by an SWY-2 and SZW-2 digital instrument, respectively. The instruments were developed and produced by the Institute of Crustal Dynamics, China Earthquake Administration. The water-level sensor has a range of 0–50 m, resolution of 1 mm, sampling interval of 1 s, precision of 0.02 percent, and accuracy of 0.05 percent (Chen, 2023).
Daily geochemical observations are conducted by the staff working at Yanqing monitoring station every day, including four major anions (F−, Cl−, SO4−, and NO3−), five dissolved gases in water (Hg, H2, He, N2, and CH4), and three degassing gases (Hg (gas), Rn (gas), and H2 (gas)). Major anions were analyzed by ion chromatography (CIC-200), dissolved gases in water were analyzed by gas chromatography (SP-3400), dissolved Hg in water was measured by mercury-measuring instruments (RG-BS) using atomic absorption, Hg (gas) degassing from the well was measured by a digital instrument (ATG-6138M), Rn (gas) was measured by a digital instrument (BG 2015R), and H2 (gas) was measured by a digital instrument (ATG-6118H).
In addition to the above measurements, we collected water samples with relatively high sample rates for trace element analysis. The sampling and analytical procedures were described by Chen and Liu, 2023: water samples were collected in relatively high and regular sample rates every 7–10 days, from June 2021 to June 2022, and a total of 61 water samples were collected during the study period (almost 1 year). Each sample was stored in 200-mL polyethylene bottles. All samples were sent monthly to the Beijing Research Institute of Uranium Geology for 41 trace element constituent analysis, including Li, Ti, Pb, Sc, Ni, Cu, Nb, Th, Zn, Tl, U, Ga, Mo, Ba, Rb, Cs, Sr, Be, Mn, Cr, Co, Y, Bi, and rare earth elements (La, Ce, Pr, Nd, Sm, Eu, Gd, Tb, Dy, Y, Ho, Er, Tm, Yb, and Lu) (Supplement.xls). Each sample was filtered with 0.45-μm membranes and then acidified with 2% nitric acid before testing it on the machine (An ELEMENT XR mass spectrometer). Measurements were repeated four times for each sample and then averaged to get the results; relative standard deviation (RSD) of each sample was better than 5%. The concentration units were ug/L (ppb).
The stable isotopes of oxygen (δ18O) and deuterium (δ2H) were also analyzed at the Beijing Research Institute of Uranium Geology using a liquid-water isotope analyzer (MAT253) with an accuracy of 0.2‰ for δ18O and δD.
3.2 Statistical analyses
We used data smoothing methods including LOWESS, rLOWESS, LOESS, and rLOESS (Chen and Liu, 2023). The LOWESS (locally weighted scatterplot smoothing) means local regression using weighted linear least squares and a first-degree polynomial model. The rLOWESS means a robust version of LOWESS that assigns lower weight to outliers in the regression. The LOESS means local regression using weighted linear least squares and a second-degree polynomial model. rLOESS means a robust version of LOESS. Hydrological variations and anomalies were considered apparent through smoothing.
We also calculate a Pearson correlation matrix (r values matrix) of 41 trace elemental compositions, and the r-values were considered statistically significant if p < 0.05. The significant r values can quantitatively indicate the correlation between each element. The correlation matrix was converted into a visual network by R program to check geochemical elemental clustering and grouping. In the network, each geochemical element is a node, and each correlation is an edge, in which the width of the edges according to the magnitude of the correlation and the placement of the nodes is a function of the pattern of correlations (Epskamp et al., 2012). This means that stronger correlations have shorter and wider edges and closer placement of nodes (Chen and Liu, 2023).
4 Results
The results of all samples are listed in Supplement.xls. The groundwater level in the Wuliying well continuously increased from 1.3 to 1.8 m during July 2021 to August 2022 (Figure 3A). The deep water temperature in the Wuliying well also consecutively increased from 33.0°C to 33.22°C during the study period (Figure 3B). The changes in the water level and temperature have no relationship to precipitation as there is only large amount of precipitation during summer (from June to August every year) (Figure 3C). No abnormal changes for the groundwater level and temperature were found temporally related to earthquakes (Figure 3).
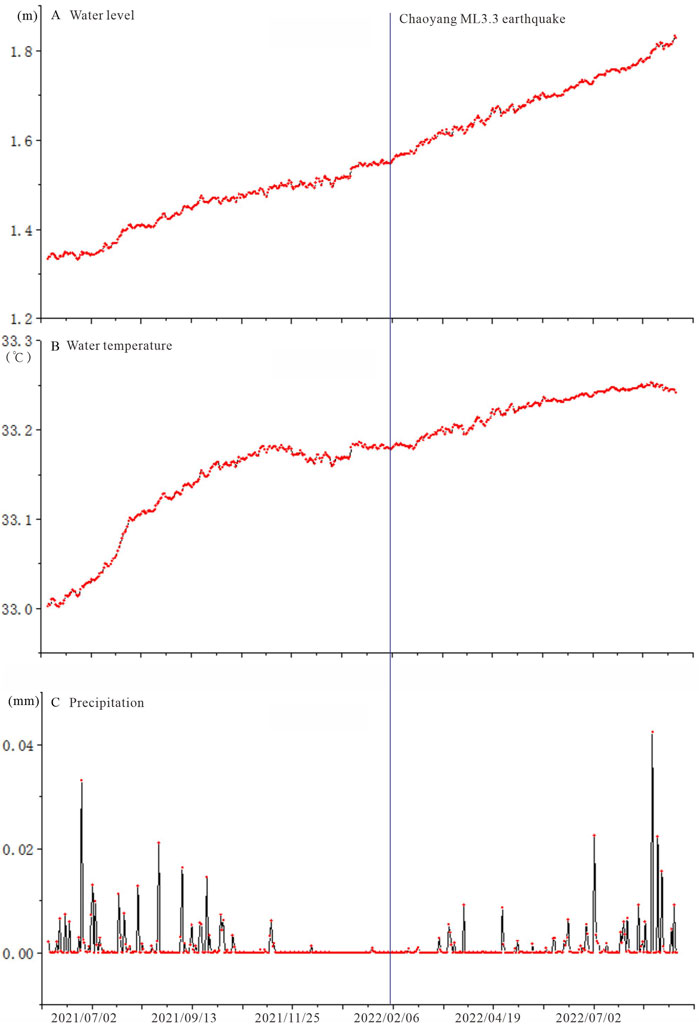
Figure 3. Water level and deep water temperature changes and precipitation during the study period (modified from Chen and Liu, 2023). The blue vertical line shows the time of the Chaoyang ML3.3 earthquake.
The trends of the major ions (F−, Cl−, SO4, and NO3−) are different (Figure 4). F- had an obvious decrease from 3.6 mg/L to 2.9 mg/L (Figure 4) during October to December 2021 before the Chaoyang earthquake and then had stable concentrations from January to June 2022 during and after the earthquakes. Cl− was stable for most of the year, but it declined rapidly in June 2022. SO4− was stable during the sampling period, and only some minor fluctuations in the months before and after the earthquake were observed. NO3− continued to rise during July 2021 to January 2022, but then it trends down. Changes in NO3− have a good temporal correlation with the earthquake, but NO3− is easily contaminated by organic matter, so this correlation is not certain.
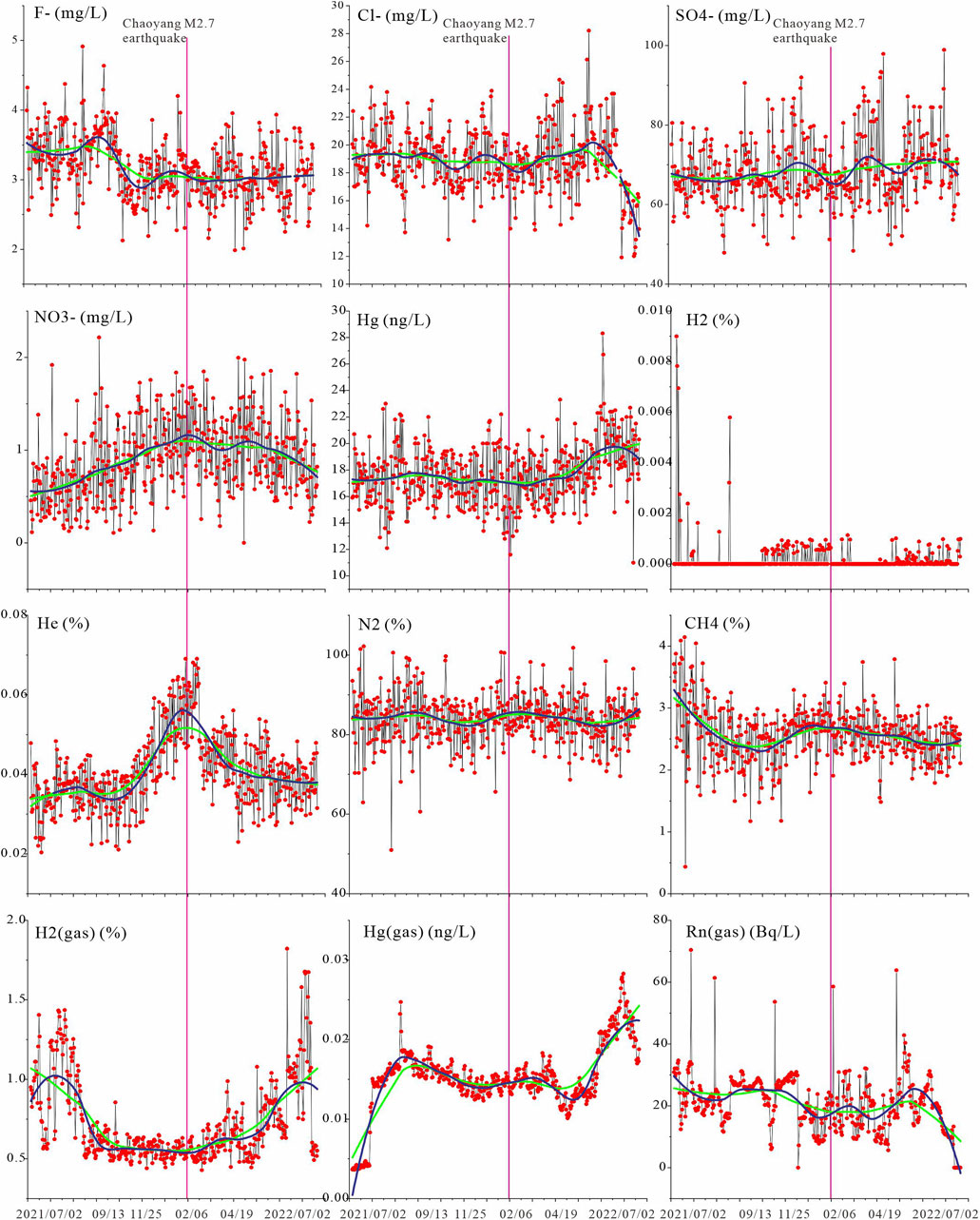
Figure 4. Content changes in 12 multiple parameters in the Wuliying well during the study period, including four major ions (F−, Cl−, SO4−, and NO3−), five dissolved gases in water (Hg, H2, He, N2, and CH4), and three gases (H2 (gas), Hg (gas), and Rn (gas)). The green line and blue line indicate the LOWESS and LOESS fitting methods, respectively. The pink vertical line shows the time of the Chaoyang ML3.3 earthquake. The x-axis of figures was a convenient conversion of actual dates to decimal for mathematical smoothing and fitting.
Dissolved gases (Hg, H2, He, N2, and CH4) in water also have different trends (Figure 4). Hg was stable for most of the year, but it increased rapidly in April–June 2022. H2 had high values in July–August 2021 and then stabilized at low values from September 2021 to June 2022. Notably, He distinctly increased from 0.03% to 0.05% during November 2021 to February 2022 before the Chaoyang earthquake and decreased from 0.05%–0.03% from March 2022 to June 2022 (Figure 4) after the earthquake. N2 was relatively stable during the study period. CH4 declined rapidly from June to November 2021, and then, it was relatively stable from January to June 2022.
Three degassing/escaping gases (Hg (gas), Rn (gas), and H2 (gas)) from water were relatively stable from October 2021 to March 2022. Notably, H2 (gas) and Hg (gas) have peaks in July each year (Figure 4), which were likely to be related to the season but not to earthquakes. When the air temperature is high in summer, these gases’ concentrations increased degassing, and when the air temperature is low in winter, these gas concentrations decreased.
The average concentration of rare earth elements (REE) is low at 0.001ug/L. The PAAS-normalized REE display remarkable positive Eu anomalies and LREE (light REE) depletion or HREE (heavy REE) enrichment distribution patterns (Figure 5A). Eu concentrations showed obvious periodic changes in the time series (Figure 5B), the period was more evident when data smoothing was done using the mathematic method (LOESS), and the Eu element concentration formed three convex peaks in September 2021, February 2022, and July 2022, respectively (Figure 5B).
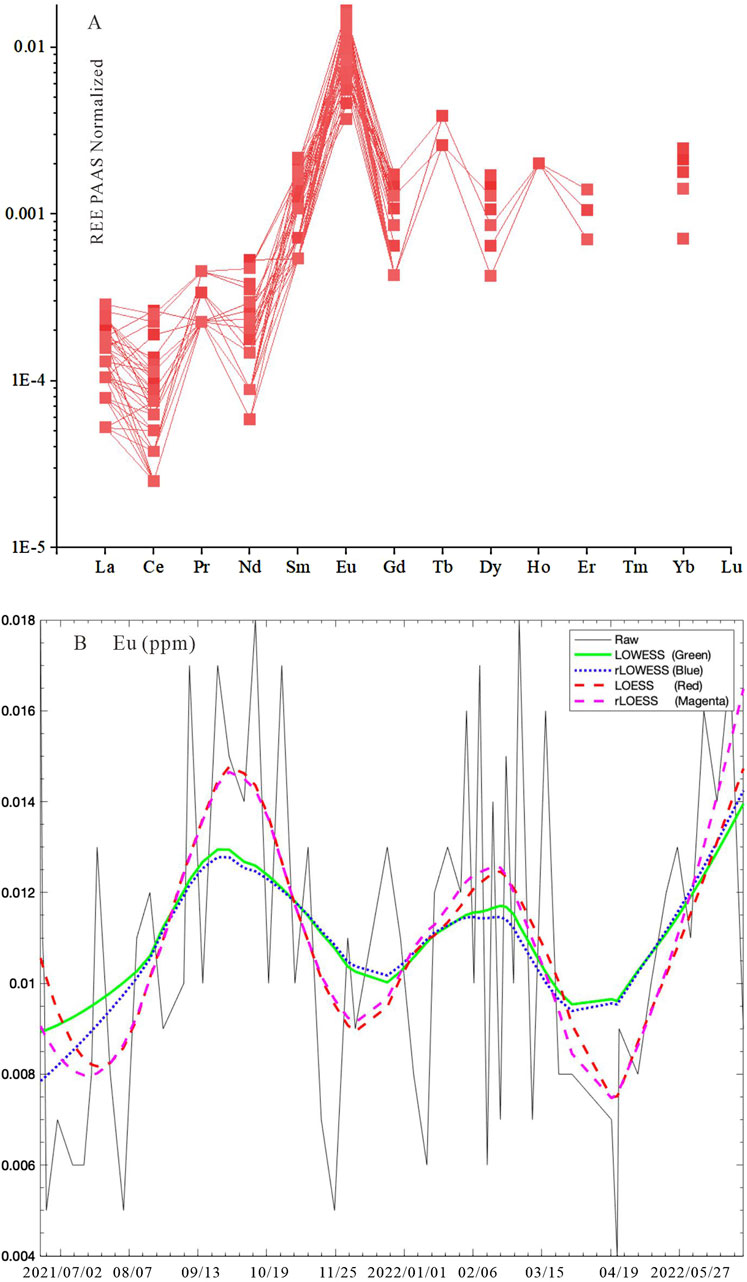
Figure 5. PAAS shale-normalized REE patterns (A) and variation in Eu (B) during the study period. The x-axis of figures was convenient conversion of actual dates to decimal for mathematical smoothing and fitting.
Apart from REE, other 27 trace elements show different time series patterns. Based on the element correlation matrix (Figure 6A, gray box), these elements can be broadly classed into two groups (Figure 6B). The first group consists of Li, Sc, Rb, Mo, Cs, Ba, W, U, Sr, Mn, Ni, and Zn, which cluster each other in the correlation network, drawing pink circles in Figure 6B. These elements had a consistent trend: rapidly decreased from December 2021 to early February 2022 (Figure 7), before the earthquake, and then increased in later time. The decreased changes were marked when smoothing methods were used (Figure 7, LOWESS and LOESS methods). The small variations in the first group trace elements might be due to the measurement error of each sample; however, since the trends are clearly evident, the overall trend is more relevant than individual variations, so the variations are believable.
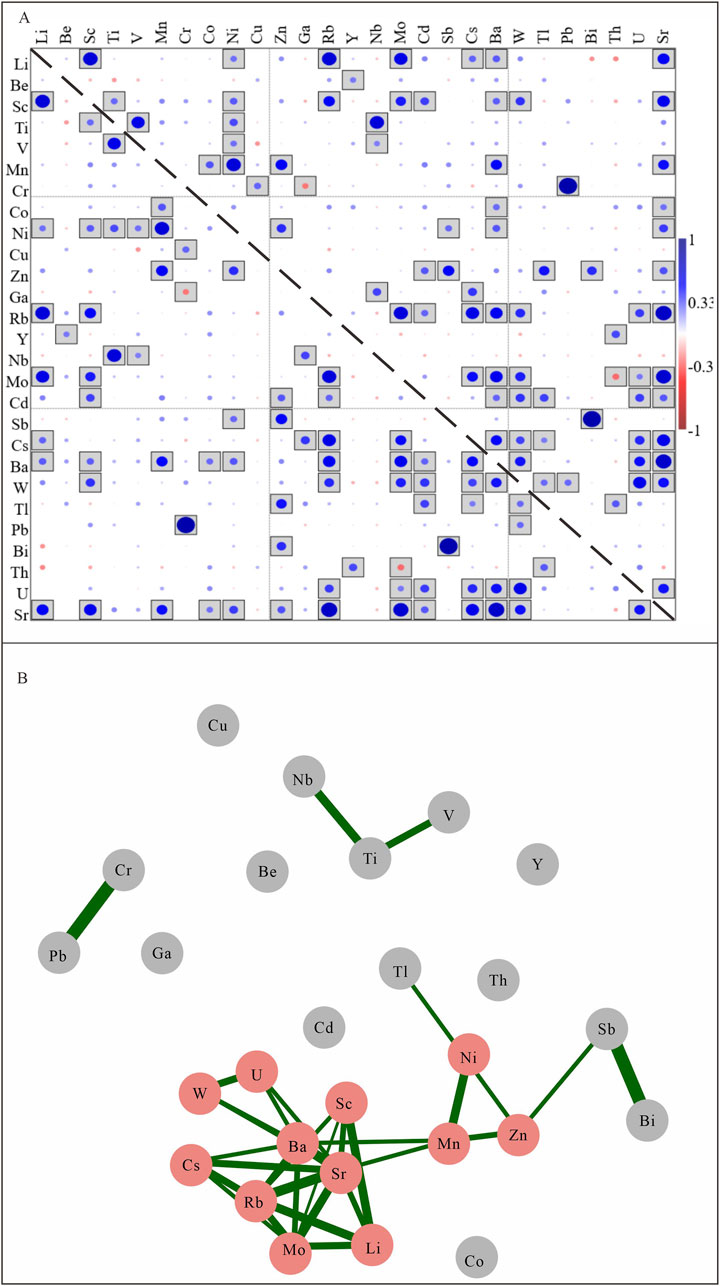
Figure 6. Trace element correlation matrix (A) and elemental correlation cluster (B). Each circle in A represents the strength of the element correlation; the larger the circle, the stronger the correlation, and the box represents that the correlation has statistical significance. Network structures in B based on the correlation matrix of (A). Each geochemical element is a node, and each correlation is an edge. Stronger correlations have shorter and wider edges and a closer placement of nodes.
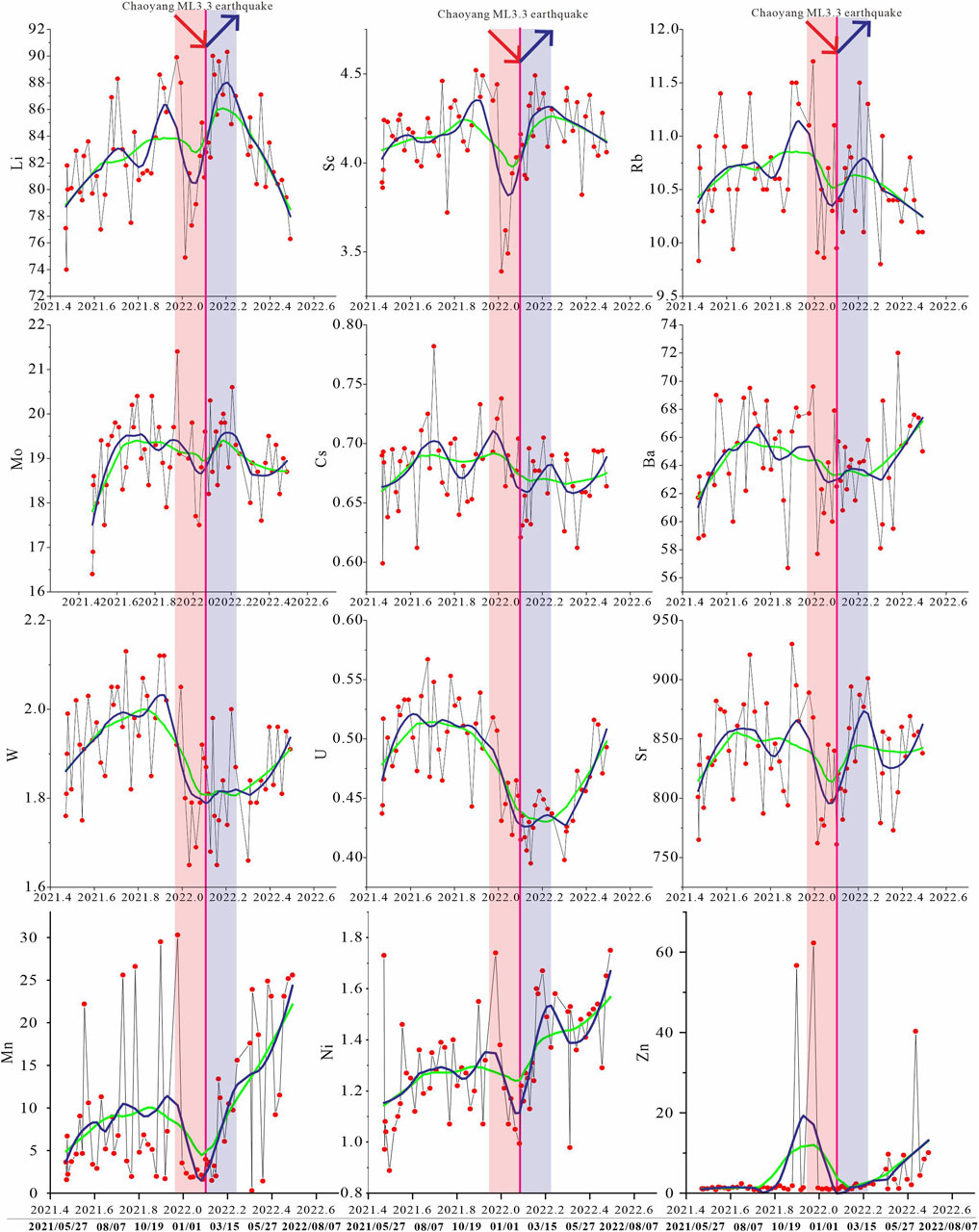
Figure 7. Time series of trace elements in the Wuliying well during the study period, including Li, Sc, Rb, Mo, Cs, Ba, W, U, Sr, Mn, Ni, and Zn (unit, in ug/L). The green line and blue line indicate the LOWESS and LOESS fitting methods, respectively. The pink shadow shows the time period of decline of trace elements. The blue shadows the show time period of increase of trace elements. The purple vertical line shows the time of the Chaoyang ML3.3 earthquake. The date of the x-axis is decimal for mathematical smoothing and fitting. The bolded font at the bottom is the actual date.
The second group consists of Pb, Cr, Ga, Cu, Be, Nb, Ti, V, Y, Cd, Tl, Th, Sb, Bi, and Co, which were dispersed among each other in the correlation network (gray circles in Figure 6B). These elements had no regular changes and no temporal coincidence with earthquakes.
For the hydrogen (δ2H) and oxygen (δ18O) isotopes, we analyzed five samples (2 December 2021, 3 January, 3 February, 3 March, and 3 April 2022) of the Wuliying well and Songshan hot spring, respectively. The hydrogen and oxygen isotopes exhibited small fluctuations during the study period. At the Wuliying well, δ2H and δ18O values range from −89.0‰ to −90.5‰ and from −13.4‰ to −12.5‰, respectively. At Songshan spring, δ2H and δ18O values range from −89.7‰ to −91.0‰ and from −12.8‰ to −13.0‰, respectively. These values all plot to the left of the GMWL (the global meteoric water line from Craig, 1961) and LMWL (the local meteoric water line from Yu et al., 1987).
5 Discussions
5.1 Water level and temperature
During the study period, the Chaoyang ML3.3 earthquake occurred in the region, but the epicentral distance was ∼65 km, and the seismic energy density produced by the earthquake at Wuliying well was ∼0.03*10−4 J/m3 (calculation according to the method from Wang, 2007), which is much smaller than the minimum energy density (10−4 J/m3) that was required for triggering hydrological responses based on global observations (Wang, 2007). The calculation of the above empirical formula was consistent with the actual observations, that is, no abnormal changes in the groundwater level and temperature to the earthquake, and they had no temporal relationship.
Many previous studies have documented co-seismic groundwater level changes, following earthquakes, and have proposed several mechanisms to explain the responses, such as co-seismic static strain-induced dilation and compaction (Ge and Stover, 2000), liquefaction or consolidation of sediments (Wang et al., 2001), and permeability changes caused by shaking (Brodsky et al., 2003). Among these mechanisms, the crustal strain dilation or aquifer permeability changes causing mixtures of different waters are usually considered to be the mechanism that explains these responses (Woith et al., 2013; Skelton et al., 2014). For our case, the absence of concomitant shifts in the water level excluded the previously proposed mechanisms in aquifer dilation/compaction and permeability changes, which would cause abundant groundwater movement and the massive addition of additional water into the wellbore, causing an increase in water levels.
5.2 Major ions and gases
In our data, three major ions (F−, Cl−, and NO3−) had changed during the study period; however, the change in Cl− did not correspond to the time of the earthquake, NO3− is easily contaminated by organic matter, and the correlation is not certain, so only F- had an obvious decrease approximately one month before the Chaoyang earthquake, which were temporal-related, and the tendency is consistent with results of the previous studies. For example, Wei et al. (1991) also found that F- concentrations appeared clearly negative anomalies around the Sihai (northwest of Beijing) M3.0 earthquake in 1986 and Zhangjiawan (Xuanhua city near Beijing) M4.2 earthquake in 1987. They suggested that the probable mechanism is the aquifer mixing process, that is, large volumes of chemically distinct groundwater enter the well and mix with the original water, so that the mixing ratio is changed. Skelton et al. (2019) also found that F- from one site in northern Iceland had decreased response to the M5.3 earthquake in 2013. They also infer mixing of groundwater sources caused by the rupture of a hydrological barrier between sources.
The mechanism of major ions was suggested to be affected by many factors, and considerably larger changes in aquifer properties or large volumes of mixing would be needed (Rojstaczer and Wolf, 1992). Variation in major ion concentrations in groundwater can record mixing and/or switching between different groundwater sources (Skelton et al., 2019), water–rock interactions, or some combination of both the processes. This is because groundwater sources tend to be chemically distinct from one another and because water–rock interactions can cause species release into (or removal from) groundwater. Hence, in this study, F- ion had an obvious decrease, meaning a small mixing of groundwater sources reflecting the leakage along a fracture system or a minor switching between sources reflecting the rupture of a hydrological barrier between sources.
Geochemical gases involved in the Earth’s degassing activity are considered responsible for water-gas-rock interaction processes able to induce chemical variations in groundwater composition (King, 1986; Martinelli, 2015). About all geochemical anomalies have been directly or indirectly attributable to deep fluid pressure variations induced by crustal deformative processes since fluid pressure is proportional to stress and volumetric strain (Petrini et al., 2012). From the results (Figure 4), four dissolved gases (Hg, H2, He, and CH4) in water had changed during the study period, while the changes in Hg, H2, and CH4 did not correspond to the time of the earthquake, only He (helium) increased approximately two months before the Chaoyang earthquake and then decreased and returned to the original value a few months after the earthquake, so they had a strong temporal relationship. Due to its deep origin and characteristics, helium appears as a powerful indicator of deep and early demixing processes, and it appears as an exceptional marker of crustal discontinuities, using faults, tiny fractures, and paths to rise to the surface (Wakita et al., 1978; Toutain and Baubron, 1999). Note that Gao and Xing (2011) also found an abnormal increase of He in the Wuliying well before the Wenchuan M8.0 earthquake in 2008. In this study, He had an obvious increase, implying deep rooting of the fractures, and the fractures had occurred at the microscale, and gas leakage along fracture pathways formed because of crustal dilation associated with the stress build-up before the earthquake.
Three degassing/escaping gases (Hg (gas), Rn (gas), and H2 (gas)) from water were relatively stable. The high values of these gases in summer may be related to air temperature and do not correspond to the time of the earthquakes.
5.3 Hydrogen and oxygen isotopes
Broadly speaking, the usefulness of using stable isotopes to predict earthquakes due to these responses is less likely to be site-specific. Specifically, variations in δ2H in groundwater records mixing and/or switching between different groundwater sources, whereas variation in δ18O in groundwater records the water–rock interaction (Skelton et al., 2019). This is because rocks, compared with water, contain proportionally more oxygen than hydrogen (Taylor, 1977). Hence, groundwater δ18O values are strongly affected by water–rock interactions. δ2H values are largely unaffected by the water–rock interaction, and can, therefore, provide a faithful record of mixing and/or switching between different groundwater sources (Skelton et al., 2019).
In our data, the δ18O and δ2H values of groundwater from both sites (Wuliying well and Songshan hot spring) plot to the left of the GMWL and LMWL (Figure 8A) and exhibited small variations. Furthermore, note that the variation in δ18O of the Wuliying well had a small increasing trend, varied from −13.4‰ to −12.5‰ (Figure 8B), and shifted from a less negative δ18O value toward GMWL, implying the strengthened degrees of the water–rock interaction. δ2H had low values compared with values in rivers (∼-85 to −65‰ in the Chaobai river (Song et al., 2007); −70 to −60‰ in the Yongding river (Liu et al., 2008)), meaning that there was no leakage of surface water (river) into the borehole. δ2H had a small downward trend and varied from −89.0‰ to −90.5‰ (Figure 8C), indicating minor mixing between older (isotopically lighter) groundwater sources and original water. Hence, stable isotope data point to the combination of groundwater mixing and water–rock interaction processes.
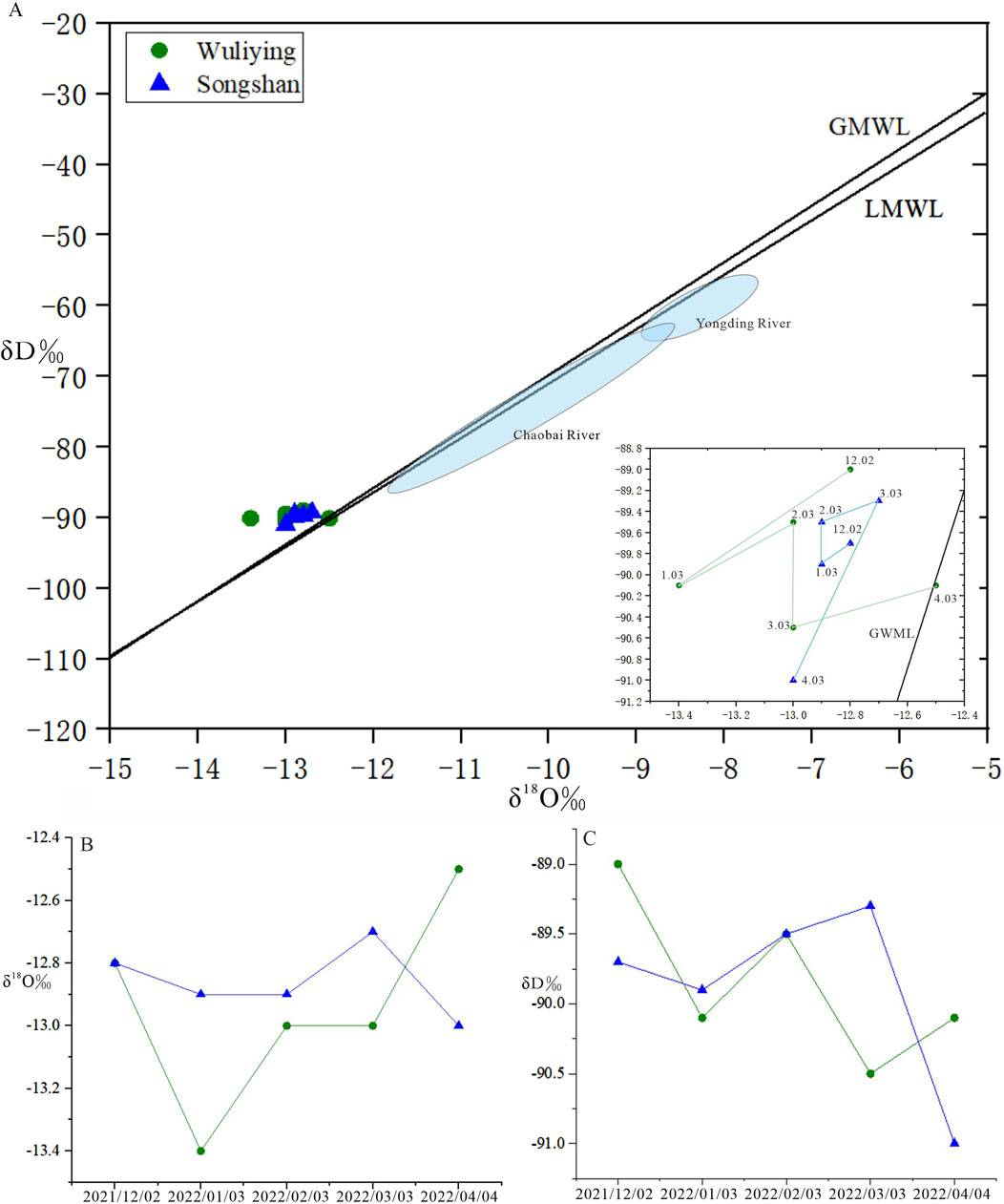
Figure 8. (A). Bi-variant plot of δD and δ18O in the five samples (2 December 2021, 3 January, 3 February, 3 March, and 3 April 2022) in the Wuliying well and Songshan spring in Yanqing District, Beijing. The δD and δ18O of the Chaobai River cited from Song et al. (2007). The δD and δ18O of the Yongding River cited from Liu et al. (2008). The inset shows the enlarged δD and δ18O variation. (B) Time series of δ18O. (C) Time series of δD.
5.4 Rare earth elements (REEs)
The REE content in the Wuliying site was very low (ug/L), which eliminated the influence of surface water and soil REE, because surface water and soil had a relatively high REE content (mg/L). The low REE content indicated that the confined water was sealed and was not affected by the surface environment.
In our case, the water of the Wuliying well displayed remarkable positive Eu anomalies and HREE (heavy REE) enrichment distribution patterns (Figure 5A), which indicated that REE in water came from feldspar and calcite minerals. Eu is a redox-sensitive REE, and in reducing settings, Eu (III) can be reduced to Eu(II), creating the potential for fractionation relative to the other REEs (Elderfield, 1988). However, this redox transformation requires both low redox potential and high temperature, a combination most commonly found in magmatic systems rather than in Earth-surface environments (Bau, 1991). Feldspar commonly becomes Eu-enriched during magmatic crystallization, owing to ready substitution of Eu2+ for Ca2+ (Taylor and McLennan, 1985; Chen et al., 2021). The calcite usually had an HREE-enriched pattern because of stronger carbonate complexation of HREEs (Webb and Kamber, 2000; Chen et al., 2021). The strength of REE-carbonate complexation increases from La to Lu as a result of a systematic increase in the stability constant of ligand complexes with increasing atomic number. The above REE results coincide with petrological evidence as the aquifer in the Wuliying well is made of limestone and dolostone containing a large amount of calcite. The overlying Jurassic basaltic rocks contain a lot of feldspar, so REE features indicated that groundwater in the Wuliying well was a mixture that came from different host rocks, implying that some fractures cut through both aquifers dominated by the limestone and overlying basaltic rocks.
The REE can trace the host rocks from which the water originated. The Eu concentrations showed obvious periodic changes with no response to the earthquake. The mechanism might be related to rock weathering rather than the earthquake. Because the concentration of Eu elements released by feldspar minerals was related to the strength of rock weathering caused by periodic temperature changes, it is most likely that weathering causes the periodic feldspar minerals to release REE to water.
5.5 Trace element characterization
Mechanism explanations for geochemical anomalies are relatively rare and based on co-seismic geochemical changes, following the earthquake (Claesson et al., 2007; Skelton et al., 2019). We considered the previously proposed mechanisms for changes in geochemical compositions. For example, Favara et al. (2001) collected geochemical composition (Ca, Cl, and SO4) at three thermal springs in central Italy in the 1997–1998 seismic swarm. The authors suggested that the recorded variations actually seem to have been induced by permeability variations related to crustal deformation in the absence of elastic energy release. Rosen et al. (2018) proposed that the changes in trace elements were likely related to the release of groundwater of different residence times from pore spaces or fractures. Shi et al. (2020) suggested that the changes in trace elements were the combined result of the addition of small amounts of water from the reservoirs isolated by the hydraulic barrier and the changes in the physical–chemical environment, following the earthquake.
Most of above mechanisms were proposed based on studies of co-seismic changes. In our study, we investigate characteristics of trace elements on pre-seismic changes, and based on smoothing methods, we can confirm probable associations between pre-seismic shifts of trace elements and the earthquake (Figure 7). These trace elements have pre-seismic downward changes, which were similar with the previous observations. For example, Claesson et al. (2004) reported pre-seismic rapidly decreased anomalies in the concentrations of Cr and Fe before the Mw 5.8 earthquake in 2002, and they interpreted that the earthquake ruptured a hydrological barrier, permitting a rapid influx of water of other aquifers. The pattern of the post-seismic recovery of chemical compositions could refer to Claesson et al. (2007); they proposed that the increase in the reactive surface area would shift the system out of chemical equilibrium and lead to a more extensive water–rock interaction, and increases in concentrations of some dissolved cations would be expected.
Furthermore, it is noteworthy that these sensitive trace elements originated from different rocks. For examples, Li and Sc probably came from basaltic rocks as Li is a typical lithophile and often enriched in mica and other silicate minerals, and Sc has a very high partition coefficient in granites (Mou, 1999). Rb, Cs, Ba, Sr, Mn, and Mo most likely came from carbonate of limestone and dolostone because these elements are closely located in the periodic table nearby the Ca element, indicating that these elements have very similar chemical properties as Ca. According to petrology, elements of Ba, Rb, Cs, and Sr often replace Ca in the calcite mineral with isomorphism (Mou, 1999). W, U, Ni, and Zn are transition elements in the periodic table, which were present in both basaltic rock and dolostone. The characteristics of different origins of the trace elements imply the ruptures cutting through strata, including different lithologies dominated by dolostone and basaltic rocks, which was consistent with REE features and the lithology of the Wuliying well.
Hence, the mechanism of trace element response in the Wuliying well may be explained as follows: the pre-seismic minor stress/strain changes caused by the Chaoyang earthquake led to small (micro) fractures cutting aquifers and overlying basaltic rocks, and maybe other nearby small isolated reservoirs, thereby leading to the mixing of chemically distinct water with the original water. The small amount of water with different trace element concentrations added, which diluted the element concentration, because the concentrations of trace elements were usually very low, and below the detection limits in many groundwater sources, the trace elements concentrations could change easily (Rosen et al., 2018). Finally, the increase in the reactive surface area caused by fractures would enhance the water–rock interactions, resulting in the post-seismic recovery of trace elements. After the earthquake, sawtooth patterns emerged in some trace elements, most likely caused by undulant changes in sealing off of the fracture with time. Thus, the mechanism of trace elements in the Wuliying well may have been the combined result of mixing and water–rock interaction processes.
We need to note that trace elements showed different responses in nearby sites. Trace elements in the Songshan hot spring near the Wuliying well had significantly upward or downward changes based on different groups in response to the Chaoyang earthquake (same earthquake in this paper), which are documented in detail by Chen and Liu, 2023, meaning that the sensitivity of trace elements in different sites may depend on many factors that could affect the fluid chemistry in a specific aquifer system. We infer that the site-specific behavior of species concentrations reflects, differing rock mineral assemblages. This is not surprising, given the differing groundwater source. For example, the aquifer of the Wuliying well came from dolomite and basaltic host rocks, while the aquifer of Songshan spring came from the granite host rocks. Furthermore, the two monitoring sites are on different structural locations; the Wuliying well is located in the sediment basin at the lower plate of the northern marginal fault, while the location of Songshan spring is on the bedrock at the upper plate of the northern marginal fault zone, implying different stress/strain sensitivities. So, trace element species were site-specific. More trace element data are needed to provide an additional understanding of the sensitivity of different trace elements to earthquake stress.
5.6 Mechanism of multiple geochemical parameters
In summary, most of those mechanisms were proposed based on studies related to springs with a single hydrological parameter, usually using the water level or major ions. Few studies have examined both groundwater levels and multiple geochemical constituents in response to earthquakes (Barberio et al., 2017; Rosen et al., 2018; Skelton et al., 2019; Shi et al., 2020). In our study, we investigate characteristics of multiple hydrological parameters. We found no shift in the water level and temperature related to earthquake, F- of major ions decreased and He gases increased before the earthquake, and some trace elements decreased before the earthquake and then returned to initial concentration, including Li, Sc, Rb, Mo, Cs, Ba, W, U, Sr, Mn, Ni, and Zn.
Hence, the mechanism for multiple hydrological parameters in this study most likely can be explained as a combination result of mixing and water–rock interactions. The mixing could be examined by no shift in the groundwater level, small decrease in the hydrogen isotopes (δ2H) and major ion concentration (F-), and pre-seismic decrease in trace elements (including Li, Sc, Rb, Mo, Cs, Ba, W, U, Sr, Mn, Ni, and Zn). The probable scenario was that minor stresses caused by the Chaoyang earthquake might create micro-cracks in bedrocks, thereby leading to mixing between chemically distinct water and the original aquifer. Because the volume of water added to the system was small, there would be slight changes in the above hydrological parameters. Finally, the increase in the reactive surface area caused by fractures would enhance the water–rock interactions, resulting in the post-seismic recovery of trace elements. This interpretation is also supported by concomitant shifts of δ18O values, and the shift from a less negative δ18O value toward GMWL also indicates strengthening degrees of the water–rock interaction.
5.7 Coupling between geochemical changes and earthquakes
As mentioned above, a group of small earthquakes above M1.0, mainly the biggest Chaoyang M2.7 earthquake, had small magnitudes and longer epicenter distance, while the small earthquakes caused several abnormal shifts of multiple geochemical changes, such as, F-, He, hydrogen and oxygen isotopes, particularly trace element changes. The sensitive geochemical parameters of the Wuliying well had strong temporal correspondence with the Chaoyang earthquake.
They also had a strong spatial correlation because these seismic activities in Beijing mainly occurred on one NW orderly fault zone orderly on a regional scale. The Wuliying well was also located in the northwest direction of the Chaoyang earthquake, and the direction was consistent with the NW orderly fault zone. Furthermore, the local fault zone was controlled by the large-scale NW orderly Zhang-Bo seismic belt in North China, so the associations indicated that the Zhang-Bo seismic zones were strongly active from January to March 2022.
Hence, the monitoring of trace element changes has temporal–spatial significance in the Beijing area, where small earthquakes occur frequently. Furthermore, we propose to find other sensitive sites to investigate continuous and long-term multiple geochemical characteristics in Beijing and the Zhang-Bo seismic belt that would be helpful for deepening the understanding of the mechanism of geochemistry changes in response to earthquake stress.
6 Wider implications
Overall, we can state that past earthquakes in our study area were probably associated with multiple pre-seismic and post-seismic geochemical shifts, and the Chaoyang earthquake had been recorded at two sites (Wuliying well and Songshan hot spring). A few trace elements are inferred to bound geochemical anomalies coupled to earthquakes, and trace elements offer the greatest potential as a precursor of M < 3 earthquakes in Beijing, confirming the findings of Chen and Liu, 2023. Trace elements might be more sensitive to small earthquake stress and would be good candidates to monitor small earthquake activities. Several other geochemical parameters for coupling with earthquakes could be shown and can offer valuable insights about the causes of coupling between geochemical changes and earthquakes. Hence, the monitoring of multiple geochemical compositions has great scientific and practical significance in the Beijing area, where small earthquakes occur frequently. Furthermore, it is possible that with further extension of our time series, trace element shifts can also be used to verify the association with other earthquakes; therefore, we suggest that the monitoring of multiple geochemical compositions should be sufficiently long so that random coincidences can be ruled out and the longevity of the time series permits statistical verification of coupling between geochemical changes and earthquakes.
7 Conclusion
In this study, we have examined the sensitivity of multiple geochemical constituents to earthquakes, including trace elements, four major anions (F−, Cl−, SO4−, and NO3−), five dissolved gases in water (Hg, H2, He, N2, and CH4), and three degassing gases (Hg (gas), Rn (gas), and H2 (gas)). Based on mathematical smoothing and fitting methods, we can better observe the association between geochemical shifts to the earthquakes. This study has the following three conclusions:
1. Although the Chaoyang earthquake had a small magnitude, long distance, and small energy density, the F-, He, and oxygen isotopes of the Wuliying well had strong temporal correspondence with the earthquakes. A few sensitive trace elements also are anomalies coupled to the earthquakes, including Li, Sc, Rb, Mo, Cs, Ba, W, U, Sr, Mn, Ni, and Zn. These trace elements are likely to be sensitive to a crustal minor strain by the small earthquakes, which offer the greatest potential as a precursor of M < 3 earthquakes in Beijing.
2. We attribute source mixing and the water–rock interaction caused by micro-scale fractures as the mechanism of coupling between changes in trace elements, oxygen isotopes, and earthquakes in this study. Our explanation provided the possibility of geochemical signals to small physical perturbations occurring at a depth.
3. This work assessed the sensitivity and validity of multi-geochemical parameters as monitoring and predictive tools in Beijing as a key seismic monitoring area. Trace elements might be more sensitive to small crustal strain and would be good candidates for short-term seismic precursor anomalies, which should be considered a priority for monitoring the tectonic activities and establishment of seismic monitoring networks along faults. Thus, the construction of continuous and long-term monitoring system for trace elements will be necessary in Beijing even on the Zhang-Bo seismic belt in the future. On the other hand, trace element monitoring could be used not only as a precursor before earthquakes but also as a good way to assess environmental changes caused by earthquakes, thus evaluating the magnitude of the disaster after earthquakes by changes in the water quality.
Data availability statement
The datasets presented in this study can be found in online repositories. The names of the repository/repositories and accession number(s) can be found in the article/supplementary material.
Author contributions
YC: formal analysis, funding acquisition, investigation, methodology, supervision, validation, visualization, writing–original draft, and writing–review and editing. GL: formal analysis, supervision, validation, and writing–review and editing. FH: formal analysis, supervision, validation, and writing–review and editing. ZW(4th author): funding acquisition, supervision, and writing–review and editing. LH: funding acquisition, supervision, and writing–review and editing. MY: supervision and writing–review and editing. XS: writing–review and editing. PH: writing–review and editing. SZ: writing–review and editing. YAZ: writing–review and editing. XW: investigation and writing–review and editing. ZW(12th Author): investigation and writing–review and editing. LX: investigation and writing–review and editing. KH: writing–review and editing. BC: writing–review and editing. HD: writing– review and editing. YOZ: investigation and writing–review and editing.
Funding
The author(s) declare that financial support was received for the research, authorship, and/or publication of this article. This work was supported by grants from the Project of Technology Innovation of Beijing Earthquake Agency (BJWC-2022017), the Beijing Earthquake Short-Term Tracking and Anomaly Verification Program (ZQDL05), and the Science and Technology Project of Beijing Earthquake Agency (Grant No.BJMS-2024001).
Acknowledgments
The authors express their sincere thanks to editors and reviewers for valuable constructive comments on this manuscript that greatly enhanced the clarity and improved this work.
Conflict of interest
The authors declare that the research was conducted in the absence of any commercial or financial relationships that could be construed as a potential conflict of interest.
Publisher’s note
All claims expressed in this article are solely those of the authors and do not necessarily represent those of their affiliated organizations, or those of the publisher, the editors, and the reviewers. Any product that may be evaluated in this article, or claim that may be made by its manufacturer, is not guaranteed or endorsed by the publisher.
Supplementary material
The Supplementary Material for this article can be found online at: https://www.frontiersin.org/articles/10.3389/feart.2024.1448035/full#supplementary-material
References
Barberio, M. D., Barbieri, M., Billi, A., Doglioni, C., and Petitta, M. (2017). Hydrogeochemical changes before and during the 2016 Amatrice-Norcia seismic sequence (central Italy). Sci. Rep. 7 (1), 11735. doi:10.1038/s41598-017-11990-8
Bau, M. (1991). Rare-earth element mobility during hydrothermal and metamorphic fluid-rock interaction and the significance of the oxidation state of europium. Chem. Geol. 93, 219–230. doi:10.1016/0009-2541(91)90115-8
Brodsky, E. E., Roeloffs, E., Woodcock, D., Gall, I., and Manga, M. (2003). A mechanism for sustained groundwater pressure changes induced by distant earthquakes. J. Geophys. Res. 108 (B8), 2390. doi:10.1029/2002JB002321
Chen, Y. X., and Liu, J. B. (2023). Groundwater trace element changes were probably induced by the ML3.3 earthquake in Chaoyang district, Beijing. Front. Earth Sci. 11, 1260559. doi:10.3389/feart.2023.1260559
Chen, Y. (2023). Time-frequency characteristics of groundwater in Wuliying monitoring well, Yanqing, Beijing and its relationship with regional seismic activity (in Chinese). North China Earthq. Sci. 41 (2), 58–68. doi:10.3969/j.issn.1003–1375.2023.02.000
Chen, Y., Jianbo*, L., BingShen, Y. W., Wu, R., Zhan, R., and Zhan, R. (2021). Geochemistry of lower ordovician microbialites on the yangtze platform, south China: implications for oceanic oxygenation at the onset of the GOBE. Palaeogeogr. Palaeoclimatol. Palaeoecol. 578, 110564. doi:10.1016/j.palaeo.2021.110564
Claesson, L., Skelton, A., Graham, C., Dietl, C., Mörth, M., Torssander, P., et al. (2004). Hydrogeochemical changes before and after a major earthquake. Geology 32 (8), 641–644. doi:10.1130/g20542.1
Claesson, L., Skelton, A., Graham, C., and M, C. M. (2007). The timescale and mechanisms of fault sealing and water-rock interaction after an earthquake. Geofluids 7 (4), 427–440. doi:10.1111/j.1468-8123.2007.00197.x
Craig, H. (1961). Isotopic variations in meteoric waters. Science 133 (3465), 1702–1703. doi:10.1126/science.133.3465.1702
De Luca, G., Di Carlo, G., and Tallini, M. (2018). A record of changes in the Gran Sasso groundwater before, during and after the 2016 Amatrice earthquake, central Italy. Sci. Rep. 8, 15982. doi:10.1038/s41598-018-34444-1
Elderfield, H. (1988). The oceanic chemistry of the rare-earth elements. Philos. Trans. R. Soc. Lond. A325, 105–126. doi:10.1098/rsta.1988.0046
Epskamp, S., Cramer, A., Waldorp, L., Schmittmann, V., and Borsboom, D. (2012). Qgraph: network visualizations of relationships in psychometric data. J. Stat. Softw. 48, 1–18. doi:10.18637/jss.v048.i04
Favara, R., Italiano, F., and Martinelli, G. (2001). Earthquake-induced chemical changes in the thermal waters of the Umbria region during the 1997-1998 seismic swarm. Terra nova. 13 (3), 227–233. doi:10.1046/j.1365-3121.2001.00347.x
Gao, L., and Xing, C. Q. (2011). Two abnormalities in fluid precursor observation in beijing wuliying well and related discussion (in Chinese). Northwest. Seismol. J. 33 (3), 644–652. doi:10.3969/j.issn.0253-4967.2011.03.013
Ge, S., and Stover, S. C. (2000). Hydrodynamic response to strike-and dip-slip faulting in a halfspace. J. Geophys. Res. 105 (B11), 25513–25524. doi:10.1029/2000JB900233
Igarashi, G., Saeki, S., Takahata, N., Sumikawa, K., Tasaka, S., Sasaki, Y., et al. (1995). Groundwater radon anomaly before the Kobe earthquake in Japan. Science 269, 60–61. doi:10.1126/science.269.5220.60
Igarashi, G., and Wakita, H. (1995). Geochemical and hydrological observations for earthquake prediction in Japan. J. Phys. Earth 43, 585–598. doi:10.4294/jpe1952.43.585
Jiang, C.-sheng, Zhong-liang, Wu, and Li, Y.-T. (2008). Estimating the location accuracy of the Beijing Capital Digital Seismograph Network using repeating events. Chin. J. Geophys. 51 (3), 817–827. doi:10.3321/j.issn:0001-5733.2008.03.022
King, C. Y. (1986). Gas geochemistry applied to earthquake prediction: an overview. J. Geophys. Res. Solid Earth 91 (B12), 12269–12281. doi:10.1029/jb091ib12p12269
Kingsley, S., Biagi, P., Piccolo, R., Capozzi, V., Ermini, A., Khatkevich, Y., et al. (2001). Hydrogeochemical precursors of strong earthquakes: a realistic possibility in Kamchatka. Part C. Sol. Terr. Planet. Sci. 26, 769–774. doi:10.1016/s1464-1917(01)95023-8
Kitagawa, Y., Koizumi, N., and Tsukuda, T. (1996). Comparison of postseismic groundwater temperature changes with earthquake-induced volumetric strain release: yudani Hot Spring. Japan. Geophys Res. Lett. 23 (22), 3147–3150. doi:10.1029/96gl02517
Kopylova, G. N., Boldina, S. V., and Serafimova, Y. K. (2022). Earthquake precursors in the ionic and gas composition of groundwater: a review of world data. Geochem. Int. 60 (10), 928–946. doi:10.1134/s0016702922100056
Li, Z.-chao, and Huang, Q.-hua (2014). Assessment of detectability of the Capital circle Seismic Network by using the probability-based magnitude of completeness (PMC) method. Chin. J. Geophys. 57 (8), 2584–2593. doi:10.6038/cjg20140818
Liu, F., Li, Y. H., and Lin, J. (2008). A hydrogen and oxygen isotope study of groundwater in the Yongding River drainage of Beijing and its environmental significance (in Chinese). Acta Geosci. Sin. 29 (2), 161–166.
Manga, M., and Wang, C.-Y. (2015). Earthquake hydrology, treatise on geophysics. Amsterdam: Elsevier.
Martinelli, G. (2015). Hydrogeologic and geochemical precursors of earthquakes: an assessment for possible applications. Boll. Geofis. Teor. Appl. 56, 83–94. doi:10.4430/bgta0146
Martinelli, G. (2020). Previous, current, and future trends in research into earthquake precursors in geofluids. Geosciences 10, 189. doi:10.3390/geosciences10050189
Martinelli, G., and Dadomo, A. (2017). Factors constraining the geographic distribution of earthquake geochemical and fluid-related precursors. Chem. Geol. 469, 176–184. doi:10.1016/j.chemgeo.2017.01.006
Montgomery, D. R., and Manga, M. (2003). Streamflow and water well responses to earthquakes. Science 300, 2047–2049. doi:10.1126/science.1082980
Petrini, R., Italiano, F., Riggio, A., Slejko, F. F., Santulin, M., Buccianti, A., et al. (2012). Coupling geochemical and geophysical signatures to constrain strain changes along thrust faults. Boll. Geof. Teor. Appl. 53, 113–134. doi:10.4430/bgta0017
Poitrasson, F., Dundas, S. H., Toutain, J.-P., Munoz, M., and Rigo, A. (1999). Earthquake-related elemental and isotopic lead anomaly in a springwater. Earth. Planet. Sci. Lett. 169 (3), 269–276. doi:10.1016/s0012-821x(99)00085-0
Roeloffs, E. A. (1998). Presistent water level changes in a well near Parkfield, California, due to lacal and distant earthquakes. J. Geophys. Res. 103 (B1), 868–889. doi:10.1029/97JB02335
Rojstaczer, S., and Wolf, S. (1992). Permeability changes associated with large earthquakes:An example from Loma Prieta, California. Geology 20 (3), 211–214. doi:10.1130/0091-7613(1992)020<0211:pcawle>2.3.co;2
Rosen, M. R., Binda, G., Archer, C., Pozzi, A., Michetti, A. M., and Noble, P. J. (2018). Mechanisms of earthquake-induced chemical and fluid transport to carbonate groundwater springs after earthquakes. Resour. Res. 54 (8), 5225–5244. doi:10.1029/2017wr022097
Shi, Z. M., Zhang, H., and Wang, G. C. (2020). Groundwater trace elements change induced by M5.0 earthquake in Yunnan. J. Hydrology 581 (2020), 124424. doi:10.1016/j.jhydrol.2019.124424
Skelton, A., Andrén, M., Kristmannsdóttir, H., Stockmann, G., Mörth, C. M., Sveinbjörnsdóttir, Á., et al. (2014). Changes in groundwater chemistry before two consecutive earthquakes in Iceland. Nat. Geosci. 7, 752–756. doi:10.1038/ngeo2250
Skelton, A., Liljedahl-Claesson, L., Wästeby, N., Andrén, M., Stockmann, G., Sturkell, E., et al. (2019). Hydrochemical changes before and after earthquakes based on long-term measurements of multiple parameters at two sites in northern Iceland—a review. J. Geophys. Res. 124, 2702–2720. SolidEarth(ja). doi:10.1029/2018JB016757
Song, X. F., Li, F. D., Yu, J. J., Tang, C. Y., Yang, C., Liu, X. C., et al. (2007). Characteristics of groundwater cycle using deuterium, oxygen-18 and hydrochemistry in Chaobai River Basin (in Chinese). Geogr. Res. 26 (1), 11–21.
Taran, Y. A., Ramirez-Guzman, A., Bernard, R., Cienfuegos, E., and Morales, P. (2005). Seismic-related variations in the chemical and isotopic composition of thermal springs near Acapulco, Guerrero, Mexico. Geophys. Res. Lett. 32 (14). doi:10.1029/2005gl022726
Taylor, H. P. (1977). Water/rock interactions and the origin of H2O in granitic batholiths: thirtieth WilliamSmith lecture. J. Geol. Soc. 133 (6), 509–558. doi:10.1144/gsjgs.133.6.0509
Taylor, S. R., and McLennan, S. M. (1985). The continental crust: its composition and evolution. Oxford: Blackwell.
Thomas, D. (1988). Geochemical precursors to seismic activity. Pure. Appl. Geophys. 126 (2–4), 241–266. doi:10.1007/bf00878998
Toutain, J., Munoz, M., Poitrasson, F., and Lienard, A. (1997). Springwater chloride ion anomaly prior to a M L = 5.2 Pyrenean earthquake. Earth. Planet. Sci. Lett. 149 (1), 113–119. doi:10.1016/s0012-821x(97)00066-6
Toutain, J.-P., and Baubron, J.-C. (1999). Gas geochemistry and seismotectonics: a review. Tectonophysics 304, 1–27. doi:10.1016/s0040-1951(98)00295-9
Tsunogai, U., and Wakita, H. (1995). Precursory chemical changes in ground water: Kobe earthquake, Japan. Jpn. Sci. 269, 61–63. doi:10.1126/science.269.5220.61
Wakita, H. (1996). Geochemical challenge to earthquake prediction. PNAS 93 (9), 3781–3786. doi:10.1073/pnas.93.9.3781
Wakita, H., Fujii, N., Matsuo, S., Notsu, K., Nagao, K., and Takaoka, N. (1978). ‘Helium spots’: caused by a diapiric magma from the upper mantle. Science 200, 430–432. doi:10.1126/science.200.4340.430
Wang, C.-Y. (2007). Liquefaction beyond the near field. Seismol. Res. Lett. 78, 512–517. doi:10.1785/gssrl.78.5.512
Wang, C.-Y., Cheng, L. H., Chin, C. V., and Yu, S. B. (2001). Coseismic hydrologic response of an alluvial fan to the 1999 Chi-Chi earthquake. Taiwan. Geol 29 (9), 831–834. doi:10.1130/0091-7613(2001)029<0831:chroaa>2.0.co;2
Wang, K., Chen, Q.-F., Sun, S., and Wong, A. (2006). Predicting the 1975 Haicheng earthquake. Bull. Seism. Soc. Am. 96, 757–795. doi:10.1785/0120050191
Webb, G. E., and Kamber, B. S. (2000). Rare earth elements in Holocene reefal microbialites: a new shallow seawater proxy. Geochim. Cosmochim. Acta 64, 1557–1565. doi:10.1016/s0016-7037(99)00400-7
Wei, J.-zhen, An-qi, J., and Si-yuan, F. (1991). Abnormal variation of F-, Cl-, SO4- inos in Wuliying well, Yanqing county, Beijing (in Chinese). Earthquake, 37–42.
Woith, H., Wang, R., Maiwald, U., Pekdeger, A., and Zschau, J. (2013). On the origin of geochemical anomalies in groundwaters induced by the Adana 1998 earthquake. Chem. Geol. 339, 177–186. doi:10.1016/j.chemgeo.2012.10.012
Yang, M., Liu, G., Liu, Z., Ma, J., Li, L., Wang, Z., et al. (2022). Geochemical characteristics of geothermal and hot spring gases in Beijing and Zhangjiakou Bohai fault zone. Front. Earth Sci. 10, 933066. doi:10.3389/feart.2022.933066
Keywords: Zhang-Bo (Zhangjiakou-Bohai) seismic belt, earthquake precursor, trace elements, hydrogen and oxygen stable isotopes, gases
Citation: Chen Y, Liu G, Huang F, Wang Z, Hu L, Yang M, Sun X, Hua P, Zhu S, Zhang Y, Wu X, Wang Z, Xu L, Han K, Cui B, Dong H and Zhou Y (2024) Multiple geochemical parameters of the Wuliying well of Beijing seismic monitoring networks probably responding to the small earthquake of Chaoyang, Beijing, in 2022. Front. Earth Sci. 12:1448035. doi: 10.3389/feart.2024.1448035
Received: 12 June 2024; Accepted: 12 August 2024;
Published: 06 September 2024.
Edited by:
Pierpaolo Zuddas, Sorbonne Universités, FranceReviewed by:
Marcella Barbera, University of Palermo, ItalyFernando Lopes, Muséum National d'Histoire Naturelle, France
Copyright © 2024 Chen, Liu, Huang, Wang, Hu, Yang, Sun, Hua, Zhu, Zhang, Wu, Wang, Xu, Han, Cui, Dong and Zhou. This is an open-access article distributed under the terms of the Creative Commons Attribution License (CC BY). The use, distribution or reproduction in other forums is permitted, provided the original author(s) and the copyright owner(s) are credited and that the original publication in this journal is cited, in accordance with accepted academic practice. No use, distribution or reproduction is permitted which does not comply with these terms.
*Correspondence: Yuxuan Chen, Y3l4NjMwQDE2My5jb20=; Guiping Liu, bGl1Z3VpcGluZ0BianNlaXMuZ292LmNu; Fuqiong Huang, aGZxaW9uZzEyNkAxMjYuY29t; Leyin Hu, aHVsZXlpbkBianNlaXMuZ292LmNu