- 1School of Geography and Sustainable Development, University of St Andrews, St Andrews, United Kingdom
- 2School of Geography, Politics and Sociology, Newcastle University, Newcastle upon Tyne, United Kingdom
- 3School of Earth and Environmental Sciences, University of St Andrews, St Andrews, United Kingdom
- 4Department of Earth Sciences, University of Geneva, Geneva, Switzerland
Volcanic ash (tephra) preserved in terrestrial environments and lake sediments contains information about volcanic processes and can be used to infer eruptive parameters and frequency of past eruptions, contributing to the understanding of volcanic hazards. However, tephra deposits can undergo transformation from their initial fallout sedimentation to being preserved as a tephra layer in the sedimentary record. The process is likely to be different in lakes and in terrestrial (soil) sequences. Here we compare the thickness, mass loading and grain size of tephra layers from the 1991 eruption of Cerro Hudson, Chile, from small lakes and adjacent terrestrial settings to measurements of the tephra made shortly after the eruption. We analysed samples from 35 cores in total from six small lakes (<0.25 km2), located 76 and 109 km from the volcano in two contrasting climatic areas (cool and humid northern site, and warm and dry southern site), and made 73 measurements of tephra thickness and 11 measurements of grain size in adjacent terrestrial areas. The major element geochemistry of our samples confirmed they were from the 1991 Hudson eruption. We found that some of the measured characteristics of the preserved tephra layers were comparable to those recorded in 1991 shortly after initial deposition, but that there was considerable variability within and between locations. This variability was not predictable and lake sediments did not preserve a notably more accurate record of the fallout than terrestrial sites. However, in aggregate the characteristics of the preserved tephra was similar to those recorded at the time of deposition, suggesting that, for palaeotephra research, a sampling strategy involving a wide range of environments is more robust than one that relies on a single sedimentary record or a single type of sedimentary environment.
1 Introduction
Tephra deposits are important for our understanding of eruptive and atmospheric processes and the hazards associated with volcanic eruptions. They are particularly useful for reconstructing the magnitude and extent of eruptions for which there are no contemporary observations – this is commonly done by applying statistical approaches to measurements of tephra thickness (e.g., Pyle, 1989; Engwell et al., 2015; Green et al., 2016; Yang and Bursik, 2016). These estimates can be further improved by the use of ash-dispersion models which incorporate grain-size data (Costa et al., 2012; Smith et al., 2020). Tephra deposits are also widely used to provide precise dating constraint in palaeoenvironmental and palaeoclimatic records; indeed, the temporal correlation among diverse sedimentary records enabled by tephrochronology has greatly enhanced our understanding of environmental change (Lowe, 2011; Davies et al., 2012; Lane et al., 2013; Timms et al., 2019). We know from process-based studies and from studies that have compared the preserved tephra layer with the properties of the initial tephra deposit, that tephra deposits are vulnerable to post-depositional reworking by wind, water and slope processes, as well as compaction effects, all of which may affect the properties of the layers that are eventually preserved in the stratigraphic record (Arnalds et al., 2013; Blong et al., 2017; Panebianco et al., 2017; Dugmore et al., 2018; Collins and Dunne, 2019). It is increasingly recognised that the period between deposition and long-term preservation in sedimentary archives is an important stage in the “life-cycle” of volcanic ash (Buckland et al., 2020; Dominguez et al., 2020; Paredes-Mariño et al., 2022).
Long term preservation of tephra layers also varies depending on the depositional environment. Lake sediments are less prone to erosion than terrestrial sediments, and many reconstructions of volcanic activity and palaeoenvironments use tephra layers in lakes for this reason (Fontijn et al., 2014; Smith et al., 2019; Hiles et al., 2021; McGuire et al., 2024). However, reworking of tephra in the lake’s catchment (and indeed within the lake itself) may mean that the thickness of a tephra layer in a sediment core does not accurately reflect the thickness originally deposited over the landscape at the time of the eruption (Bertrand et al., 2014; Boygle, 1999; McNamara et al., 2019). Preservation of tephra layers in sub-aerial locations is more variable. In some locations preservation is good and insights into long term volcanic activity or palaeoenvironmental change are possible (Oladóttir et al., 2008). More typically, only larger eruptions - ones which produce deposits several cm thick over large areas - are likely to be preserved (Fontijn et al., 2014). Where layers are thin, or the environmental conditions unfavourable for preservation, the layer may be eroded by wind and dispersed entirely (Arnalds et al., 2013; Liu et al., 2014; Panebianco et al., 2017; Dominguez et al., 2020). For these reasons, measurements of tephra thickness should ideally be taken shortly after an eruption occurs (i.e., days to weeks). However, to reconstruct eruptions for which contemporary records are not available, and in palaeoenvironmental reconstruction, we are limited to preserved tephra layers. These layers may have been modified by various processes that are not well understood, nor easy to detect in the field. In particular, we do not fully understand the extent to which post-depositional re-mobilisation results in selective thickening or thinning of tephra deposits and grainsize variations, in different depositional environments, and over what spatial and temporal scales.
Existing comparisons between tephra measurements taken at the time of the eruption and the terrestrial tephrostratigraphic record, as observed some years later, suggest that tephra thickness preservation varies across landscapes, and that this variation may be predictable. For example, it has been shown that vegetation cover (heathland vs. scrub woodland) has a strong effect on tephra preservation in Iceland (Cutler et al., 2016a; Cutler et al., 2016b; Dugmore et al., 2018; Morison and Streeter, 2022). Small (cm-m) scale surface topography may also drive variability in tephra thickness (Thompson et al., 2022). Some degree of thinning of tephra layers after deposition can be expected (due to deposit compaction), but comparisons of mass-loading measurements show that in some circumstances the preserved tephra layer differs from the original measurements even once compaction is taken into account (Cutler et al., 2018). Data are also available from lakes: for example, studies at Cordón Caulle and Calbuco in Chile suggest that some lake tephra deposits (particularly in deeper and larger lakes with significant river inflows) may systematically overestimate past eruption volumes, but that smaller and lower energy lakes are likely to provide a good record (Bertrand et al., 2014; McNamara et al., 2019).
To address these issues, we surveyed the properties (thickness, loss on ignition, mass loading, and grain size distribution) of the tephra layer formed by the 1991 eruption of Cerro Hudson, Chile, in six small lakes and across 73 sub-aerial sections. The 1991 eruption of Hudson was chosen because its large (4.35 km3) tephra deposit was widely distributed across a range of climatic zones from the Andes to the Patagonian steppe and we know the deposit has been subject to extensive aeolian remobilisation (Scasso et al., 1994; Kratzmann et al., 2010; Wilson et al., 2010). We compared our results to similar measurements made shortly after the eruption. Although the rate of transformation is likely to be highest immediately after an eruption, tephra deposits may continue to be transformed, albeit more slowly, for some years after an eruption (cf., Cutler et al., 2021). In existing comparisons between the measurements of tephra taken during the eruption and later measurements of preserved tephra deposits in lakes, the time interval between measurements is just a few years (e.g., McNamara et al., 2019). In our study the time interval between deposition and measurement was almost 30 years which means that the preserved tephra layer is more likely to resemble the deposits in older sediments.
The preservation potential of tephra varies from west to east across the Andes, with better preservation in the well vegetated and wet areas to the west, and poor to non-existent preservation in the sparsely vegetated and semi-arid areas to the east (Fontijn et al., 2014). The sampling was conducted across two contrasting climatic areas (one cool and humid, the other warm and semi-arid) to capture this variation. The semi-arid sampling area was close to Chile Chico (Figure 1), where measurements of tephra thickness and grain size distribution were made shortly after the 1991 eruption of Cerro Hudson (Scasso et al., 1994; Banks and Iven, 1991), allowing like-for-like comparison. To investigate the influence of catchment characteristics on preservation in small lakes (<0.25 km2), we examined tephra layers in sediment cores from three lakes in each area. We also surveyed the tephra layer in terrestrial locations adjacent to each lake, to assess the differences between lacustrine and terrestrial preservation. We selected terrestrial locations with contrasting vegetation cover within each lake area to test whether this factor also affected preservation. In the lakes, we anticipated better preservation (i.e., a closer resemblance to the initial deposit) in the cool, humid locations due to higher vegetation cover and greater surface stability. These factors would be expected to inhibit remobilisation. We also expected that the lakes would preserve a more complete record of the initial deposit than terrestrial settings, as the former are more likely to retain sediment than adjacent soils, which are subject to erosion by wind and water. In terrestrial settings, we anticipated that well vegetated areas would more closely resemble the initial deposit compared to sparsely vegetated areas.
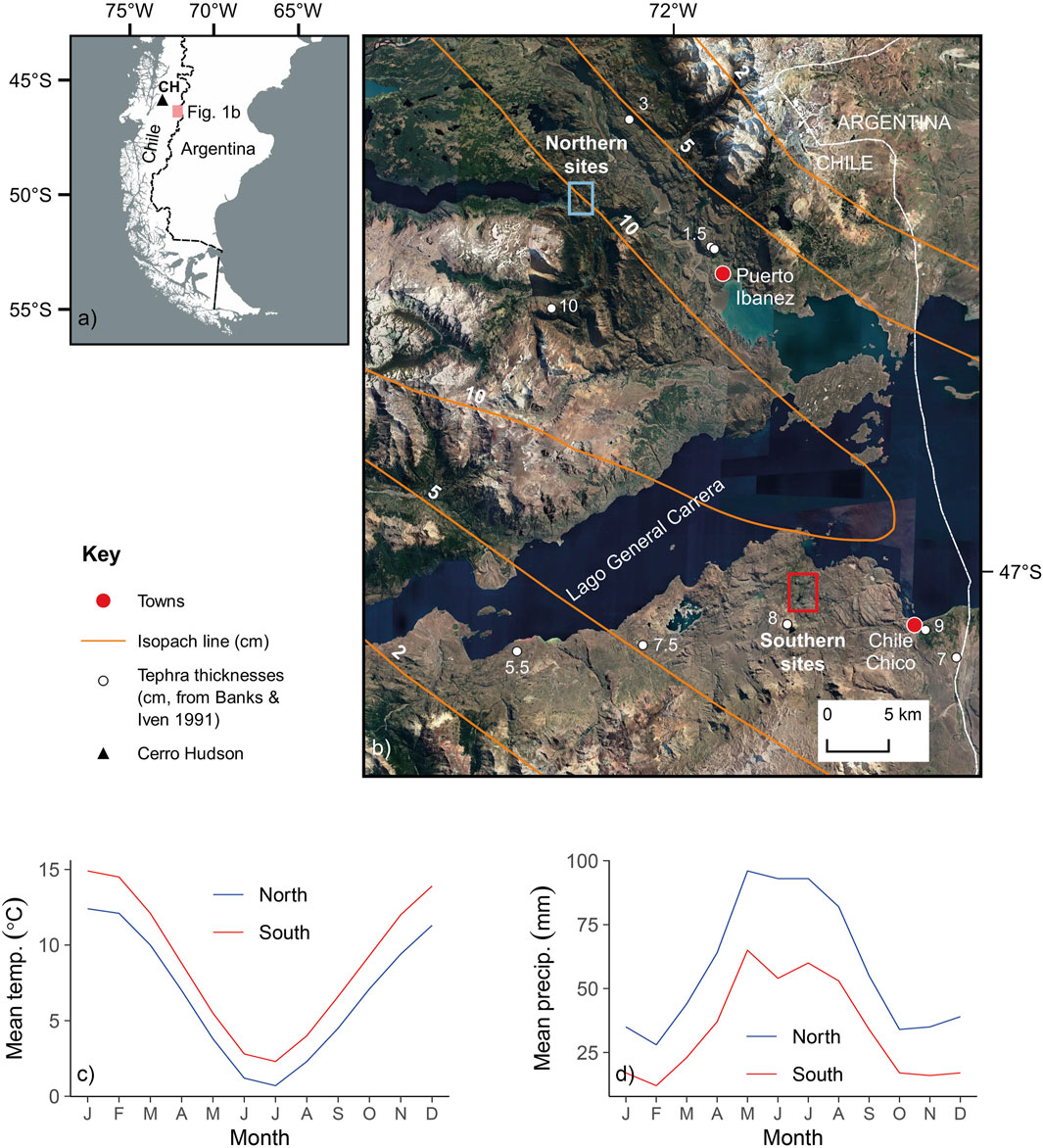
Figure 1. Overview of study location and climate. (A) Overall setting (B) Location map of study sites. Isopachs (in orange with thickness in cm) of the 1991 fallout deposit are from Scasso et al. (1994). White circular points show thickness in cm from the survey conducted by Banks and Iven (1991) in August 1991. Blue and red boxes show location of northern and southern sites respectively (C) Mean monthly temperatures for study sites, from Fick and Hijmans (2017) (D) Mean monthly precipitation for study sites, from Fick and Hijmans (2017).
2 Methods
We investigated the effect of lacustrine and terrestrial conditions on the preservation of tephra layers based on a coupled sampling strategy carried out 29 years after the 1991 Hudson eruption. In order to understand variability of tephra preservation in areas of contrasting climate, we selected two locations with multiple lakes suitable for sampling within a small area (1–2 km radius), one about 76 km from the volcano (northern sites) and the other about 109 km from the volcano (southern sites) close to locations sampled by Scasso et al. (1994) and Banks and Iven, (1991) (Figures 1–3). We sampled both the lake (cores) and the adjacent terrestrial soil/sediment to compare the preservation features in both environments.
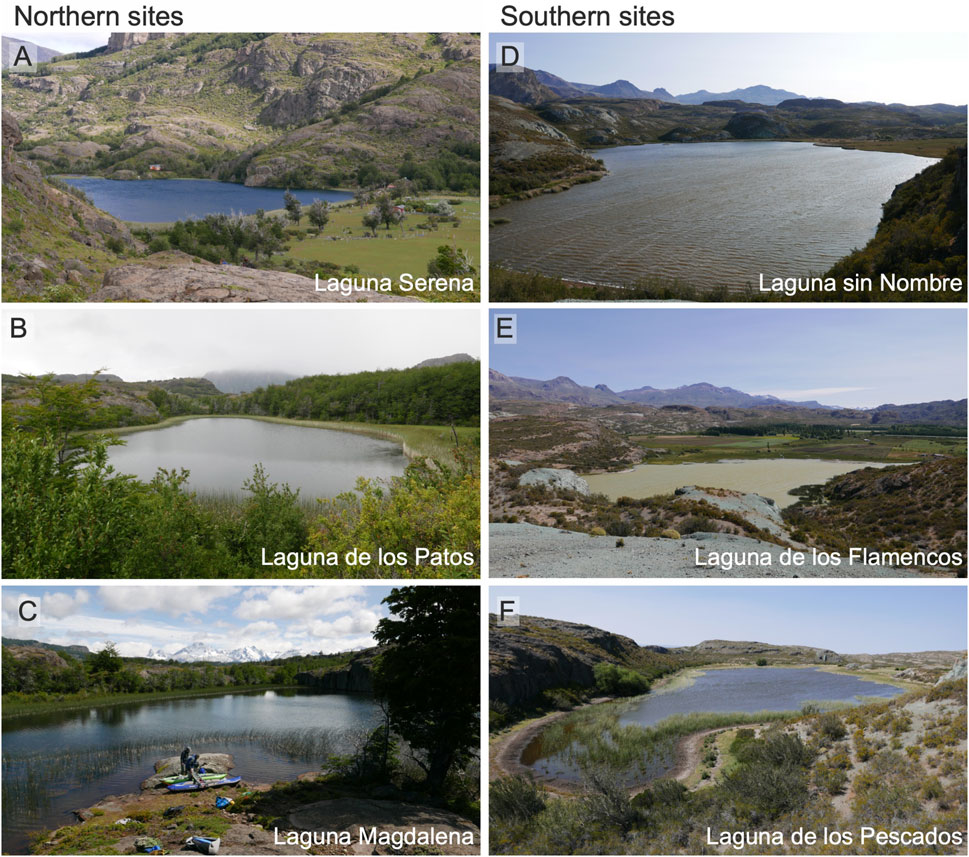
Figure 2. Photographs of surveyed lakes and surrounding landscape. Lakes are named on each photo. Typical vegetation and land surface cover is illustrated; patchy temperate forest and shrub cover with areas of exposed rock in panels (A-C), and sparse shrub cover with large areas of bare sediment in panels (D-F).
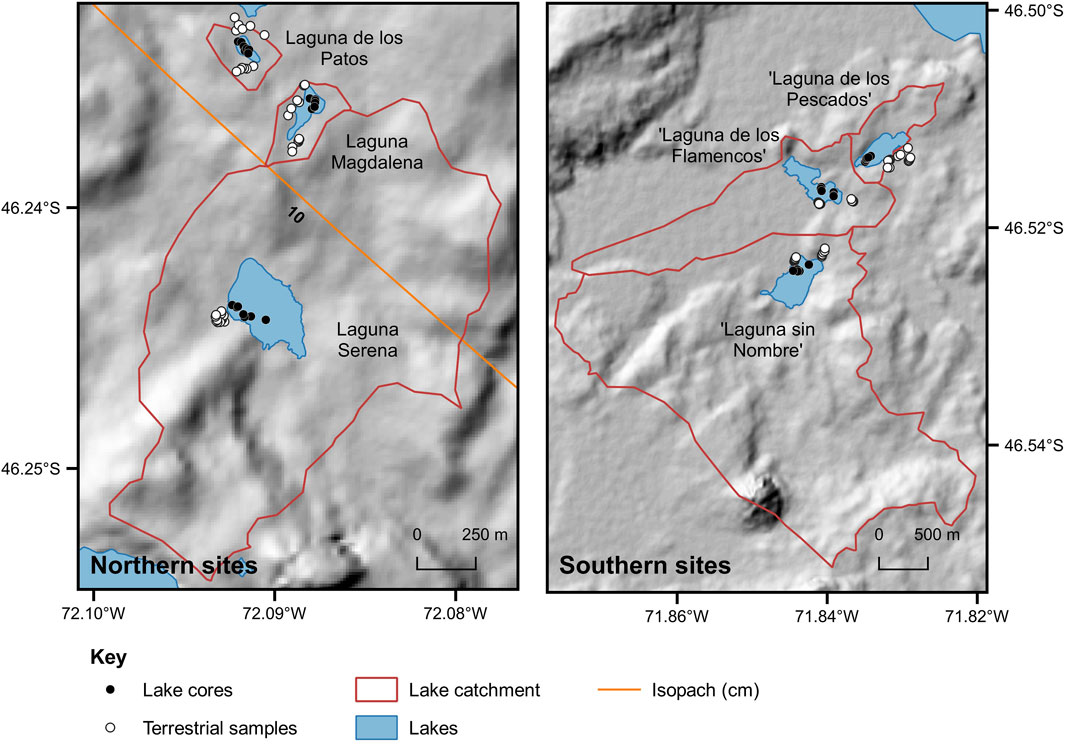
Figure 3. Detailed location map of study sites and all sampling locations. Background is a hillshade based on a 12.5 m resolution ALOS-PALSAR based Digital Elevation Model. Catchment outlines are shown in red. Isopach from Scasso et al. (1994).
2.1 Cerro Hudson
Cerro Hudson is located in the Southern Volcanic Zone of the Andes (Figure 1A) and has produced several large and widely dispersed tephra deposits throughout the Holocene (Naranjo and Stern, 1998; Stern, 2007). Activity over the last 100 years includes three moderate-to-large size eruptions that occurred in 1971 (Volcanic Explosivity Index, VEI 3), in 1991 (VEI 5) and in 2011 (VEI 2) (Global Volcanism Program, 2024).
The 1991 eruption of Cerro Hudson (hereafter referred to as CH1991) occurred in two phases. The first phase, which started on the 8 August, resulted in an eruption column height of up to 12 km above sea level, with a dispersal axis to the north (Kratzmann et al., 2010). A paroxysmal phase occurred from 12 to 15 August with a maximum plume height of about 18 km above sea level, when the bulk of material was deposited (Kratzmann et al., 2010). A total volume of 4.35 km3 of tephra dispersed mainly to the southeast of the volcano and covered an area of more than 100,000 km2 in Chile and Argentina (Scasso et al., 1994). A notable feature of the deposit was a marked bimodality in the grain size distribution attributed to a combination of alternating coarse and fine eruptive phases, with particle aggregation occurring during atmospheric sedimentation (Scasso et al., 1994).
2.2 Lake and catchment characteristics
There is a notable difference in the vegetation communities between the northern and southern sites, reflecting differences in annual precipitation and temperature (Figures 1, 2). Northern sites are markedly cooler and wetter than the southern sites. Northern sites receive around 600 mm of precipitation annually, with a mean annual temperature of 4°C–6°C. Southern sites receive around 400 mm precipitation annually, with a mean annual temperature of 8°C–10°C (Fick and Hijmans, 2017). Our sites encompass two distinct ecoregions, namely, humid Valdivian temperate forest in the north and west, and semi-arid Patagonian steppe in the south and east. These differences are typical of the main climatic and vegetation gradient observed between the Andes and the steppe in the east, although sites further west and east would show a larger range of climatic differences than among our sites. At each site we selected three small lakes which were within 1 km radius of each other, to minimise differences in primary tephra fallout. The lakes were located at 0.1–1 km (northern Sites) and 0.5–1.8 km (southern Sites) distance from farms and had evidence of grazing within the catchments. There were enclosed pastoral field systems adjacent to the lakes in the case of Laguna Serena (Northern sites), and Laguna sin Nombre and Laguna de los Flamencos (Southern sites) (Figures 2A, D, E). Local relief in the surrounding terrain was low (up to 50 m) at five of the lakes but higher, up to 250 m, at Laguna Serena (Figures 1, 3).
To understand potential sources of differences in preservation between lakes and their catchments we calculated the lake catchment area and the percentage vegetation cover surrounding each lake at the time of the eruption. We used a DEM based on ALOS-PALSAR data (ASF DAAC, 2015) with a resolution of 12.5 m to calculate the catchment area for each lake through the Hydrology Spatial Analyst Toolbox of ArcMap 10.7. The Fill function was applied to fill possible sinks. Flow Direction and Flow Accumulation were calculated from the DEM. Subsequently the pour points were selected for each lake and used to delineate the drainage basin with the Watershed tool. Due to the resolution limitations of the DEM, catchment areas were subsequently adjusted to reflect drainage routes observed on the ground and from satellite imagery.
We estimated surface cover on our sites using the Normalized Difference Vegetation Index (NDVI), a good proxy for vegetation cover (e.g., Laidler et al., 2009). In order to calculate NDVI for our sites we selected two Landsat scenes, one from December 1984 (the closest suitable image prior to the eruption) and a second captured in January 2020 during our collection of field measurements (Supplementary Table S1). For each scene, the NDVI was calculated and a threshold level of 0.2 was applied to distinguish between vegetated and non-vegetated areas. The NDVI threshold level was selected based on field observation of vegetation presence, and the level used in other studies (e.g., Durán et al., 2013). Finally, the reclassified vegetation cover image was masked with a 500 m buffer from each lake edge and subsequently the total area in each land cover category was calculated. We defined the major vegetation communities around the lakes on the basis of their structure and height, as these factors have been shown to affect tephra preservation (Cutler et al., 2016b).
2.3 Lake sampling
Using a short-drop Universal Corer (manufactured by Aquatic Research Instruments), we took short (<30 cm) cores from each lake bed to sample the sediments deposited since 1991, the tephra layer and the underlying sediments. Sedimentation rates and processes may vary within lakes, so we measured tephra thickness from 5 to 6 cores per lake. The two largest lakes (Laguna Serena and Laguna sin Nombre) had fluvial inflows at one end, and we located our core sites at the other end of the lake in these cases, to minimise the effect of fluvial inflow on tephra deposition. Additionally, we kept at least 5 m from the shore to minimize edge effects. Otherwise, core locations were located where sampling was feasible, and with a minimum of a few metres separation between cores. We re-sampled cores where the bottom contact of the tephra with the underlying sediments was not visible. The clear core tube permitted measurement of tephra thickness without extruding the sediment (Figures 4C, H). We made four measurements of tephra thickness at 90-degree intervals around the core barrel. These measurements were averaged to create a single mean thickness for each core, which was used for subsequent analysis. For a minimum of one core in each lake, the core was extruded, and we carefully collected all the material within the visual upper and lower boundaries of the tephra layer for further laboratory analysis (i.e., grain size and geochemistry). In most cases, the water depth was recorded at the core location, with at least one measurement of water depth taken per lake.
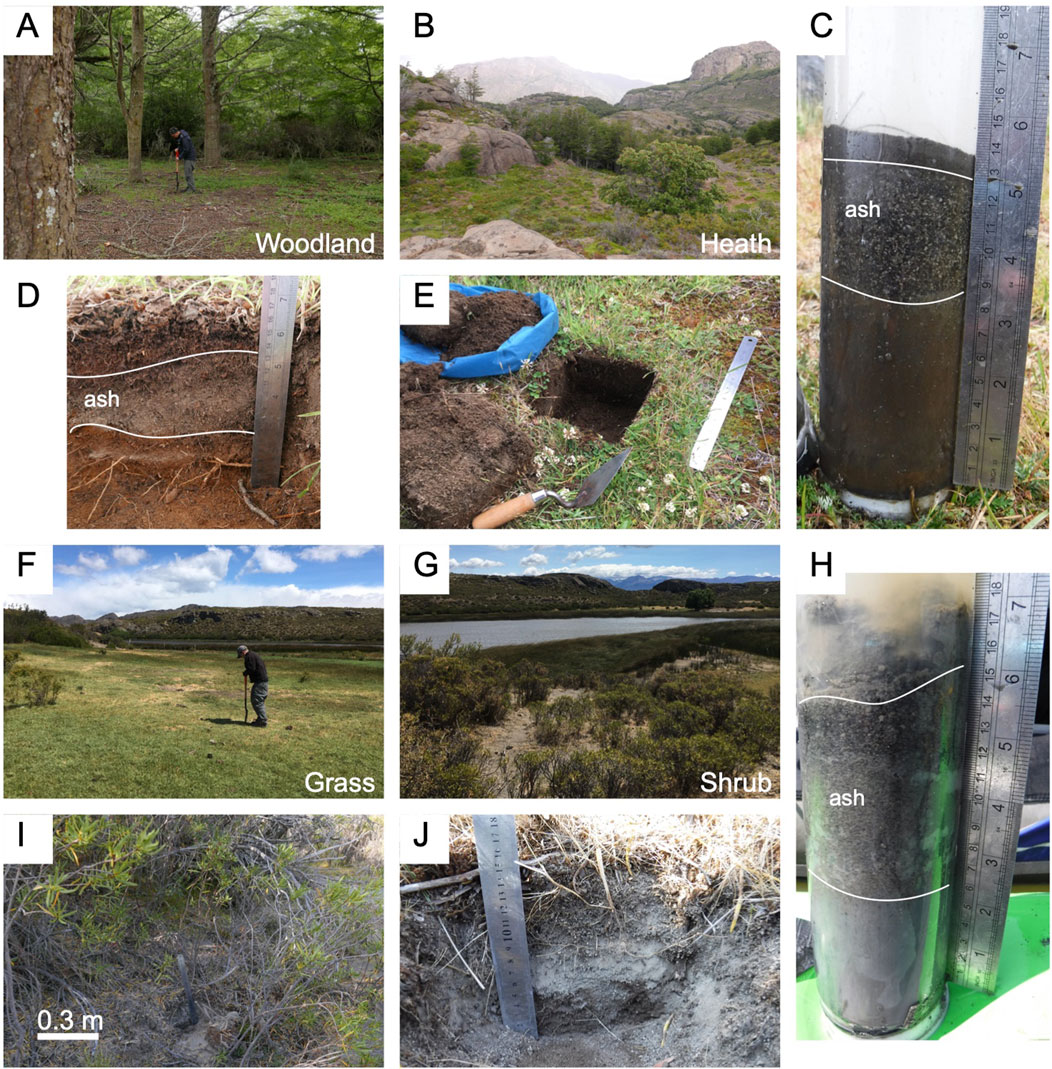
Figure 4. Sample site photographs of vegetation cover and sections. Panels (A–E) are from the Northern sites and show main vegetation cover categories (A, B), and example sections and cores (C–E). Panels (F–J) are from the Southern sites and show vegetation cover categories (F, G) and example sections and cores (H–J).
2.4 Terrestrial sampling
For each vegetation community around each lake we selected six locally flat sampling locations within areas of mature vegetation to record tephra thickness. At the southern sites vegetated areas were frequently interrupted by patches of bare sediment. These bare areas often had tephra visible at the surface. Sampling locations were selected to avoid bare areas and we only sampled where there was evidence that the surface was generally stable, such as the presence of moss cover or biocrust. All sampling locations were located either within the catchment of the adjacent lake or close to limits of the lake catchment (Figure 3). To record the tephra thickness we made a small (c. 20 cm × 20 cm) shallow excavation to expose the tephra and underlying sediments (Figure 4E). Where the soil and root mat were coherent, we were able to make four measurements of tephra thickness. In some locations the unconsolidated nature of the sediments meant we were only able to make two thickness measurements at each sampling location. In each case, thickness measurements were averaged to create a single mean for each site and this mean was used in subsequent analysis. Other characteristics of the tephra layer (grain size, colour, identifiable sub-units) were also noted, as well as any notable features of the vegetation community (proximity to large plants or roots) and local small-scale topography. For each vegetation community at each lake we retained a sample of the tephra from at least one sampling point for further analysis. In order to determine the significance of differences between tephra thickness in the different types of environment we first performed a non-parametric Kruskal-Wallis H-test to determine if there was a difference in thickness across all the types of environment. If this showed a difference in thickness, we then performed a post hoc Wilcoxon rank sum test with Bonferroni correction on the thicknesses from each combination of environment (e.g., heath compared to lake) to identify which types of environments had thicknesses significantly different from each other. Further details of the statistical analysis can be found in the supplementary text.
2.5 Mass loading and organic content
Lake and terrestrial tephra samples were transported to the University of St Andrews for further laboratory analysis. We used Loss On Ignition (LOI) to establish the proportion of organic material in the tephra layer. Samples were dried at 105°C for a minimum of 8 h, and then cooled in a dessicator, to remove remaining moisture, and then weighed. Samples were then heated to 550°C for 4 h to drive off organic material, and the difference in weight before and after was used to calculate the percentage of organic material. Mass loading was calculated by dividing the mass of tephra (i.e., the material remaining after the LOI processing) by the cross-sectional area of the lake core tube. Deposit dry bulk density was calculated from the mass of the dried material (tephra and organic material) and the volume of material collected in the lake core tube.
2.6 Grain size analysis
All lake and terrestrial samples were analysed using a Beckman Coulter LS230 Particle-Size Analyser using laser techniques to obtain the Grain Size Distribution (GSD) of 0.4–2000 µm range (Blott et al., 2004). Subsamples were pre-treated with 30% hydrogen peroxide, to remove residual organic matter remaining after LOI, and 10% hydrochloric acid, to remove carbonates (e.g., Morison and Streeter, 2022). For each sample three subsamples were analysed, and each subsample was measured in triplicate for 60 s each. Each distribution was an average of the three subsample grain-size distributions generated for each sample (e.g., Etyemezian et al., 2019). Sorting was calculated based on the Inman graphic standard deviation in phi units (Inman, 1952). We calculated the proportion of each sample in the size range 63–500 μm as this represents the size range prone to aeolian remobilisation (Dominguez et al., 2020), so could indicate enrichment by aeolian remobilised tephra or depletion of the tephra by wind.
2.7 Geochemistry
To confirm that the surveyed tephra layer was from the 1991 eruption of Cerro Hudson we undertook major element geochemical analysis of samples from each of the lakes and associated terrestrial areas (see tephra data repository dataset for details). Initial preparation was the same as the grain size distribution analysis. Only the 63–500 µm size fraction was analysed, to avoid the potential complications of geochemistry varying with grain size, and to ensure that all glass shards were a suitable size for analysis. Selected shards were mounted in epoxy resin stubs (using EpoThin resin), the surfaces ground down to expose shards and polished using diamond paste. Major element concentrations were determined at the University of St Andrews using a JEOL iSP100 Electron Probe Micro Analyser with 5 wavelength-dispersive X-Ray spectrometers. The accelerating voltage was 15 kV, the current was 5 nA and the beam was 5 μm. All elements were counted on-peak for 20 s (Si, Al, Fe, Mg, Ca, K), except for Na (10 s, and analysed first to minimise alkali loss), and P, Mn and Ti (30 s). Off-peak background counting times were 10 s except for Na (5 s).
We evaluated instrument accuracy throughout each session using the glass standards ML3B-G (basalt), StHs6/80-G (andesite/dacite) and Lipari obsidian ID3506 (rhyolite). At least 20 measurements of each of these standards were made throughout the run and average values were mostly within two standard deviations (2σ) and within ±2% of published values (see tephra data repository dataset and Supplementary Table S2 for details). Analytical uncertainties are represented in figures by the mean and 1 SD of two replicate analyses of StHs6/80-G standard glass (one per analytical session), which is the most compositionally similar standard to our unknowns. We removed measurements with analytical totals <97% from our analysis and data were normalised to 100 wt% on an anhydrous (volatile-free) basis to aid comparison between sessions and different studies. The results of these analyses were compared to previous analyses of tephra from the 1991 eruption (Kratzmann et al., 2009).
3 Results
3.1 Lake and lake catchment characteristics
The degree of vegetation cover around the lakes varies considerably between the two regions (Table 1), with the northern lake catchments having higher proportions of vegetation cover (62%) compared to the southern lakes (19%). At the northern sites vegetation cover prior to the 1991 eruption was 6%–20% lower than in 2020, whereas it has remained broadly constant at the southern sites. Catchment areas varied considerably by lake, but on average were larger at the southern sites (Table 1). Maximum measured lake depth was notably greater (>8 m) at the northern lakes than at the southern lakes, which were uniformly shallow (1.6 m or less) (Table 1).
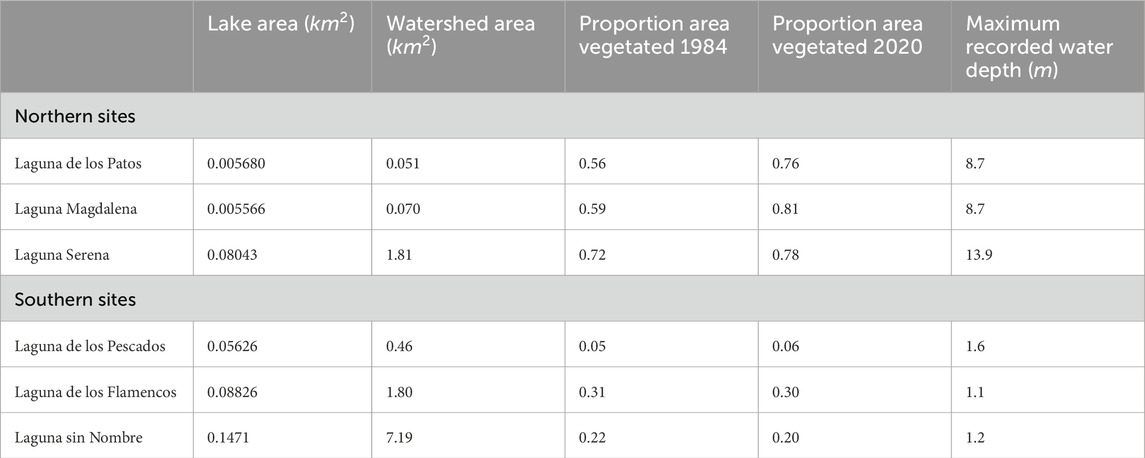
Table 1. Lake and catchment characteristics. The proportion of area vegetated is calculated using the area enclosed by a 500 m wide buffer zone from the lake edge.
We defined divided vegetation cover for northern sites into two categories, woodland and heath. Woodland cover consists of a mixture of Nothofagus woodland (N. pumilio and N. antarctica), primarily, with a sparse ground layer, and grassland/heath cover is characterised by shrubby plants (Embothrium coccineum, Gaultheria mucronata, Baccharis magellanica, and Escalonia species). Expanses of bare rock were common, and the soil cover was often less than 30 cm deep (Figures 4A, B).
In the southern sites, two categories of vegetation cover were identified. The first category consisted of shrubs (up to a few m high), with large patches of open ground and limited moss ground cover (Figures 2D, 4G). The second category comprised areas dominated by a uniform grass and forb sward Figure 4F. Vegetation cover on the southern sites was shorter in stature (trees were sparse) and patchier. Vegetation patches were frequently separated by areas of unconsolidated sediments (coarse sand mixed with tephra grains); a layer of moss growing under shrubs sometimes stabilised the sediments. Vegetation cover was dominated by thorny shrubs (notably Colliguaja integerrina, with Berberis microphylla, Shinus johnstonii and Adesmia boronoides also present), tussocky grasses (e.g., Stipa sp.) and drought-tolerant herbaceous varieties (e.g., Chuquiraga aurea, Senecio spp., Grindelia chiloensis).
3.2 Tephra layers characterisation
We took 35 lake cores in total, from six lakes (Figure 3). A coherent layer of tephra was found 0.5–2 cm below the lake-sediment interface. In most cases the tephra layer was readily apparent through the core tube and had clear contacts with adjacent sediment at the base and top of the layer (Figures 4C, H). Typically, 5–15 cm of the underlying sediment was retrieved from each core. No other tephra layers were observed in the sampling core column.
At our northern sites, tephra in both the lake cores (17 samples) and terrestrial sections (36 samples) was a mixture of fine and coarse ash to lapilli, ranging in colour from dark to light grey (Figures 4C, D). The tephra had no discernible sub-units within either the lake cores or terrestrial sections. At several of the terrestrial woodland sections we observed a 0.5–1.5 cm thick fine tephra layer 2–3 cm below the 1991 tephra layer, presumed to be from the 1971 eruption of Cerro Hudson.
Tephra in the southern lakes (18 core samples) was visually similar to the tephra observed in the northern sites. The tephra deposit was underlain with grey clay in lake samples from Laguna sin Nombre and Laguna de los Flamencos (Figure 4H). Within the terrestrial sections (37 samples) the tephra was distinct from the surrounding sediments, and there was a higher proportion of fine particles than observed at the northern sites (Figures 4I, J). At some of the shrub sites the tephra varied in colour and grainsize through the layer, but these units were not consistent among sites.
3.3 Tephra thickness and mass loading
Based on published isopachs and the nearest available thickness measurements, the primary tephra fallout deposit was in the region of 50–100 mm thick at our northern sites at the time of the eruption (Banks and Iven, 1991; Scasso et al., 1994; Figure 1). Tephra thickness in the northern lake cores spanned 43–168 mm, with the median thickness across all lakes of 86 mm (n = 17 cores) (Figure 5A). There was no significant difference in the mean thickness of tephra among the lakes as determined by a one-way H-test (p=0.07). Mean thickness at Laguna de los Patos, where tephra was thickest, was 105 ± 38 mm compared to 66 ± 15 mm at Laguna Serena, where tephra was thinnest (Figure 5A, in green). Tephra thickness across all terrestrial sections spanned 20–103 mm.
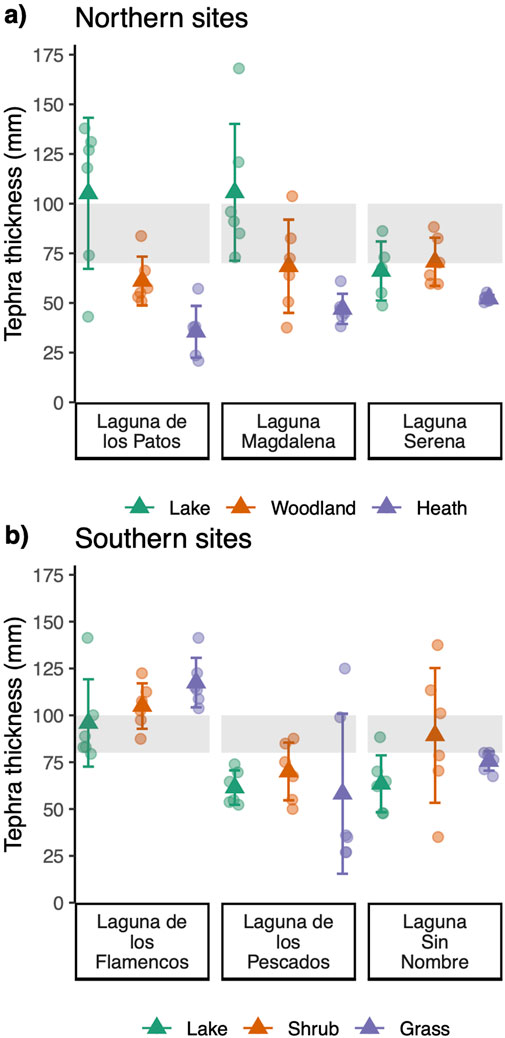
Figure 5. Variation in tephra thickness for both lake and terrestrial environments in (A) northern sites, and (B) southern sites. Circles show individual thickness measurements, triangles show mean thicknesses and error bars show one standard deviation. Grey rectangles show a range of possible thicknesses at the site based on nearest measurements from 1991 (Banks and Iven, 1991; Scasso et al., 1994).
There was a significant difference in tephra thicknesses among woodland (Figure 5A, in orange) and grass/shrub sites (Figure 4A, in purple), and those in the lakes (Figure 5A, in green), determined by one-way H-test (p=<0.001). A Wilcoxon rank-sum test showed a significant difference between thickness in lakes and woodland (p=0.03), lakes and grass/shrub sites (p=<0.01) and between areas of woodland (67 ± 16 mm, n = 16) and grass/shrub (45 ± 11 mm, n = 16, p = <0.01). Mass loading and organic content were variable through the lake cores. As an example, within Laguna Magdalena mass loading ranged from 1.39 to 6.07 g cm−2 and organic content from 1.4% to 23.6%, and had no apparent correlation with tephra thickness (Table 2).
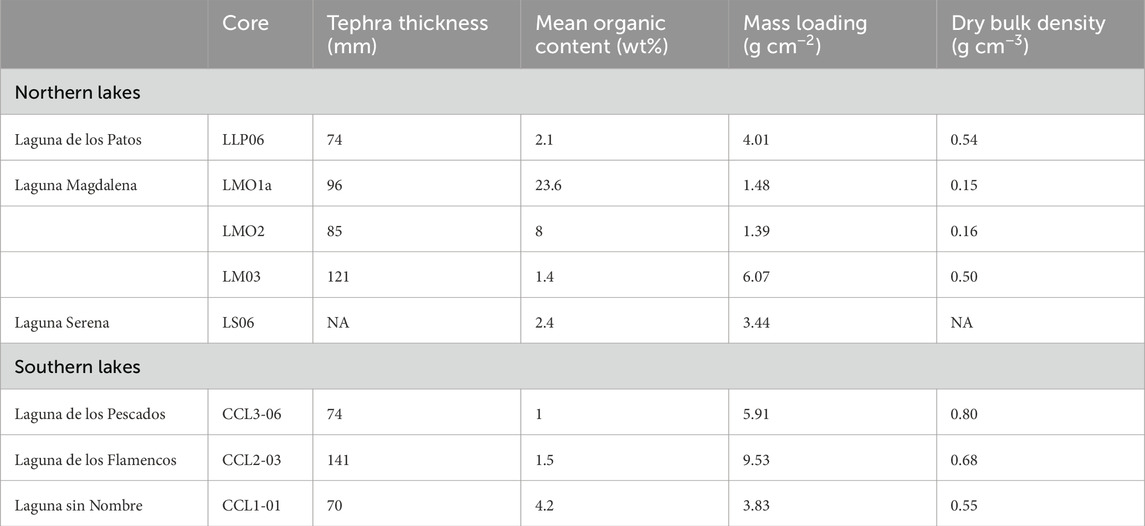
Table 2. Tephra layer mass loading, organic content, dry bulk density and thickness from lake cores.
At our southern sites primary fallout thickness was around 80 or 90 mm [derived from Scasso et al. (1994) isopach map and the nearby measurements of Banks and Iven (1991); Figure 1]. Tephra thickness measured in the 18 lake cores spanned 48–141 mm (Figure 5B), with the median thickness across all the lakes of 70 mm (n = 18). A one-way H-test showed significant variation in thickness among the lakes (p=0.008) (Figure 5B), with a Wilcoxon rank-sum-test showing that only the difference between thickness in Laguna de los Flamencos and Laguna de los Pescados was significant (p = 0.01).
A one-way H-test showed no significant difference between tephra thickness in the lakes and the two terrestrial vegetation categories (p = 0.18). In the terrestrial sections, tephra thickness spanned 27–141 mm (Figure 5B). The mean thickness of tephra found in areas of shrub cover (88 ± 27 mm, n=18) was similar to that found in grass cover (83 ± 35 mm, n = 18), although there were notable differences among lake catchments with thickness values in all categories tending to be larger at Laguna de los Flamencos than the other lake catchments (Figure 5B). In the bare areas between shrubs there were patches of exposed tephra on the surface (we did not sample these areas), but coherent layers of tephra were found amongst the shrubs where the surface was stable, as indicated by the presence of a moss layer. Mass loading was determined in cores from five lakes and tended to be higher than at northern sites (Table 2). Percentage organic content was lower (<5%) (Table 2).
3.4 Tephra grain size distribution
A total of 21 GSD of both lake and terrestrial samples are summarised in Table 3; Figure 6.
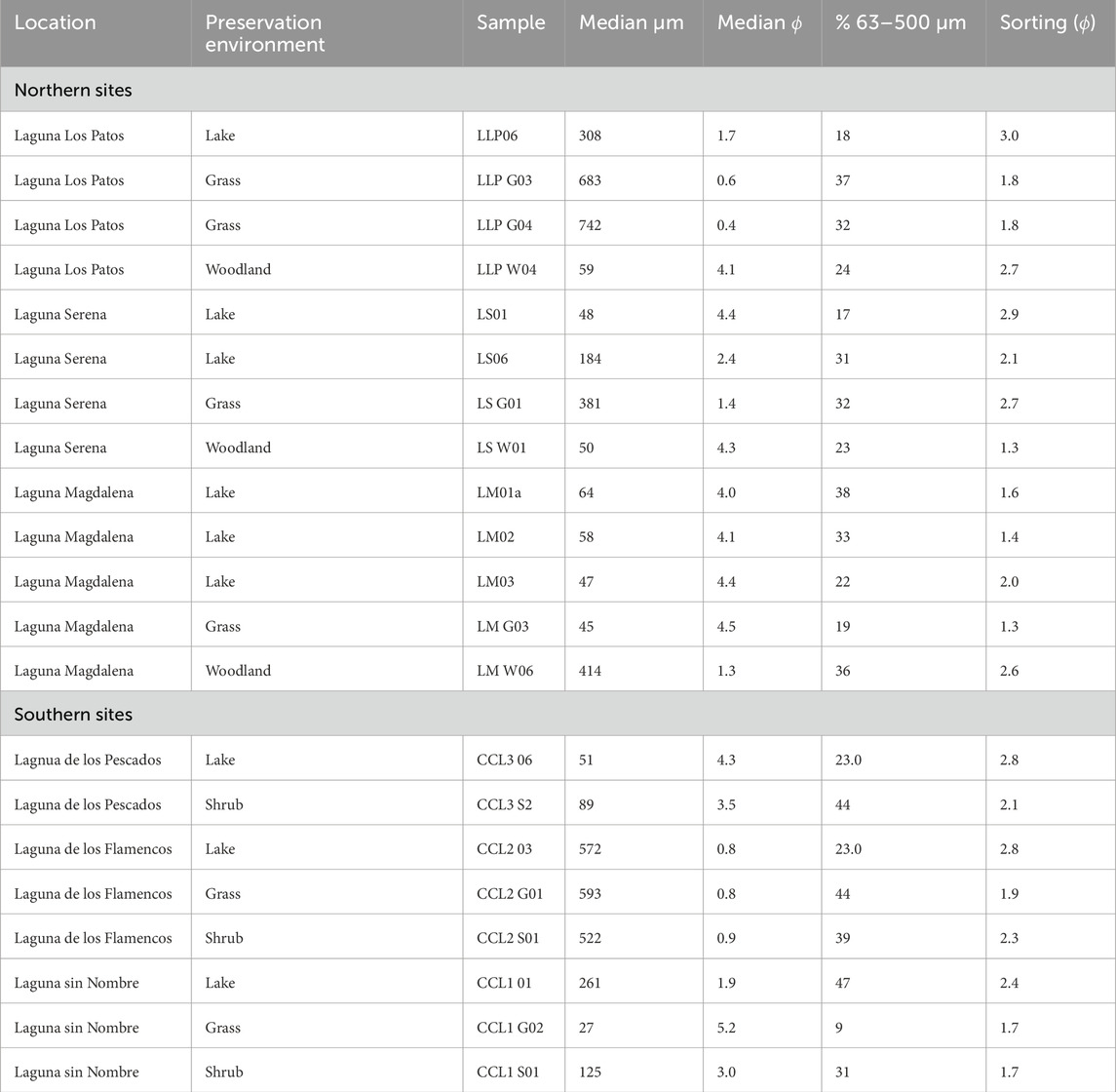
Table 3. Summary grain size characteristics of tephra. Sorting is calculated using the method of Inman (1952).
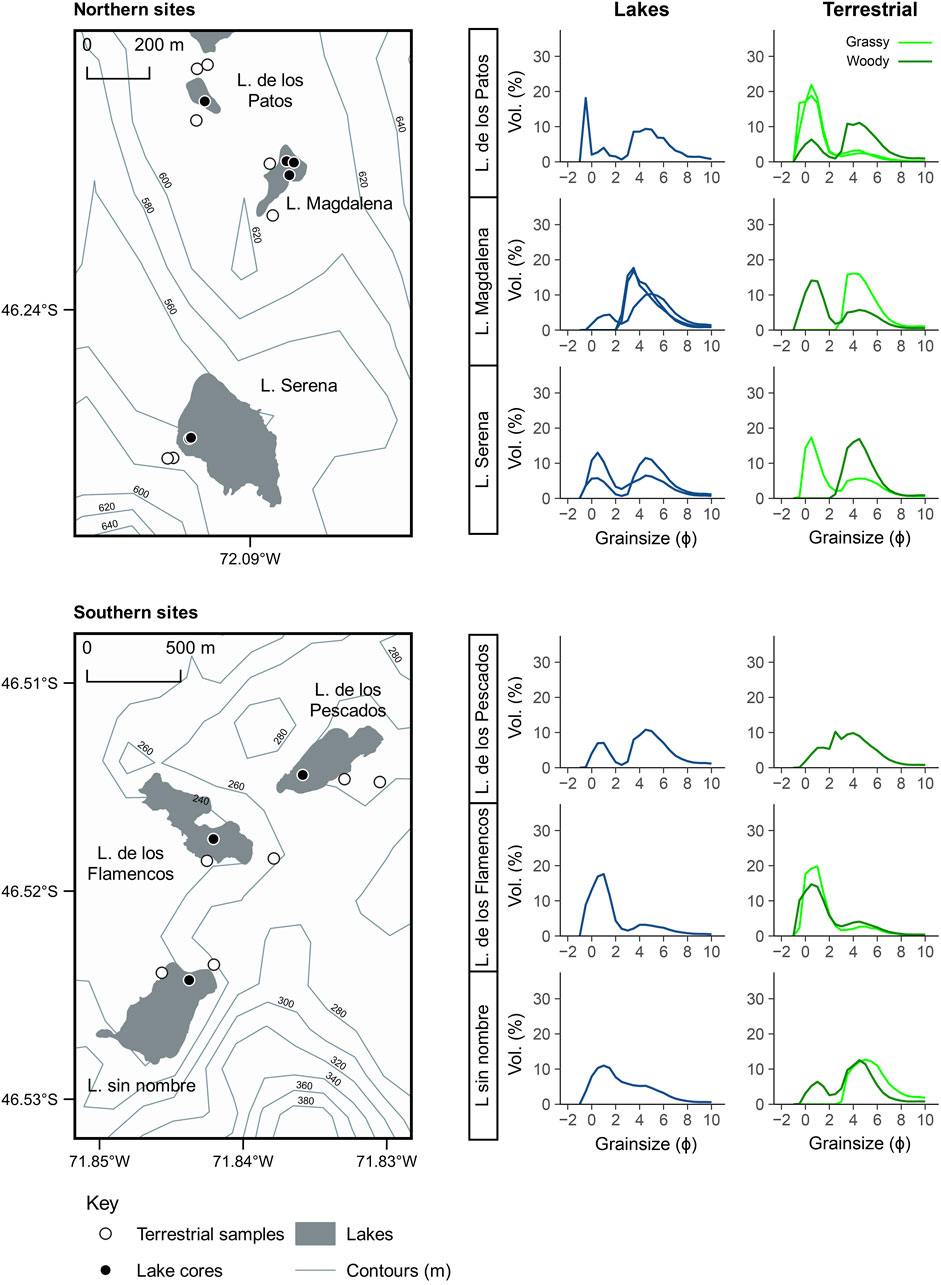
Figure 6. Grain size distribution for sampled tephra. Maps on left show sample locations, with open circles terrestrial samples and closed circles lake sediment samples. Multiple lines on graphs indicate multiple samples collected and grains size measured.
Grain size distributions from the northern lake sites were variable. A majority of samples (nine out of 13) across all sample environments (lake, grass and woodland) had a bimodal distribution with peaks around 1 ϕ and 4-5 ϕ and a long tail up to 9 ϕ. Four samples (two lake, one terrestrial grass and one terrestrial woodland) had a unimodal distribution with a modal grain size around 4-5 ϕ and a notable absence of grains coarser than 2-3 ϕ (Figure 6A). Sorting values for all northern samples averaged 2.2 and ranged between 1.3 and 3 (Table 3). The proportion of the deposit in the range 63–500 μm was fairly consistent between samples, averaging 28% (Table 3).
For the southern lake sites grain size distributions were reasonably consistent, with six out of eight samples having a bimodal distribution with peaks around 1 ϕ and 5 ϕ. The remaining two samples (both from terrestrial sections) had a broad unimodal distribution, with a modal peak around 4-5 ϕ (Figure 6B). The proportion of the deposit in the range 63–500 μm was variable and ranged between 9% and 47% (Table 3). The mean sorting value of 2.2 is the same value reported by Scasso et al. (1994) at their nearby sampling location. The grain size distribution of our samples was slightly finer than that measured by Scasso et al. (1994) at Chile Chico, 8 km south-east from our sampling locations (Figure 7).
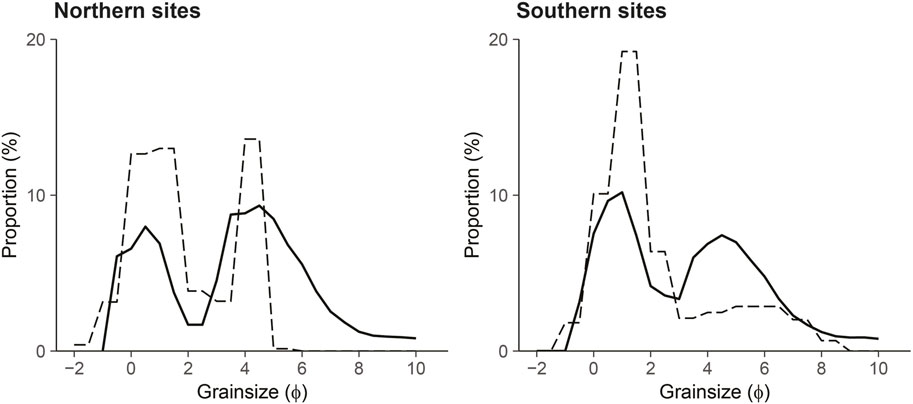
Figure 7. Mean grain size distribution for our sites plotted against grain size distributions from samples collected at Sthe time of the eruption. Our data is shown as a solid line and is binned in 0.5 phi increments and was collected using a laser-diffraction particle size analyser. Comparison data is shown with dashed lines and comes from the nearest available location. For northern sites, data from site NB91HD14 in Banks and Iven (1991) and data for southern sites is from a site at Chile Chico reported in Scasso et al., 1994. Comparison data is determined in 1 ϕ increments based on sieved weight, and in the case of the Banks and Iven (1991) data it only spans the grain size range −2 to 6 ϕ. To increase comparability between datasets the percentage values for the comparison data were adjusted to match the 0.5 ϕ range of our data.
There was no clear relationship between the overall shape of the grain size distributions (bimodal or unimodal) and depositional setting (Figure 6), although overall the proportion of terrestrial sites with a unimodal distribution (33%) was slightly higher than the equivalent proportion of lake sites (22%). The mean distributions from each area resemble the shape of the distribution from the closest available measurements taken at the time of the eruption (Figure 7).
3.5 Geochemistry
Major element geochemical analysis of 367 tephra shards from 20 sampling locations, incorporating all lakes and their catchments, overlapped substantially in composition when compared to published data from phase two of the 1991 eruption of Hudson (Figure 8; Kratzmann et al., 2009).
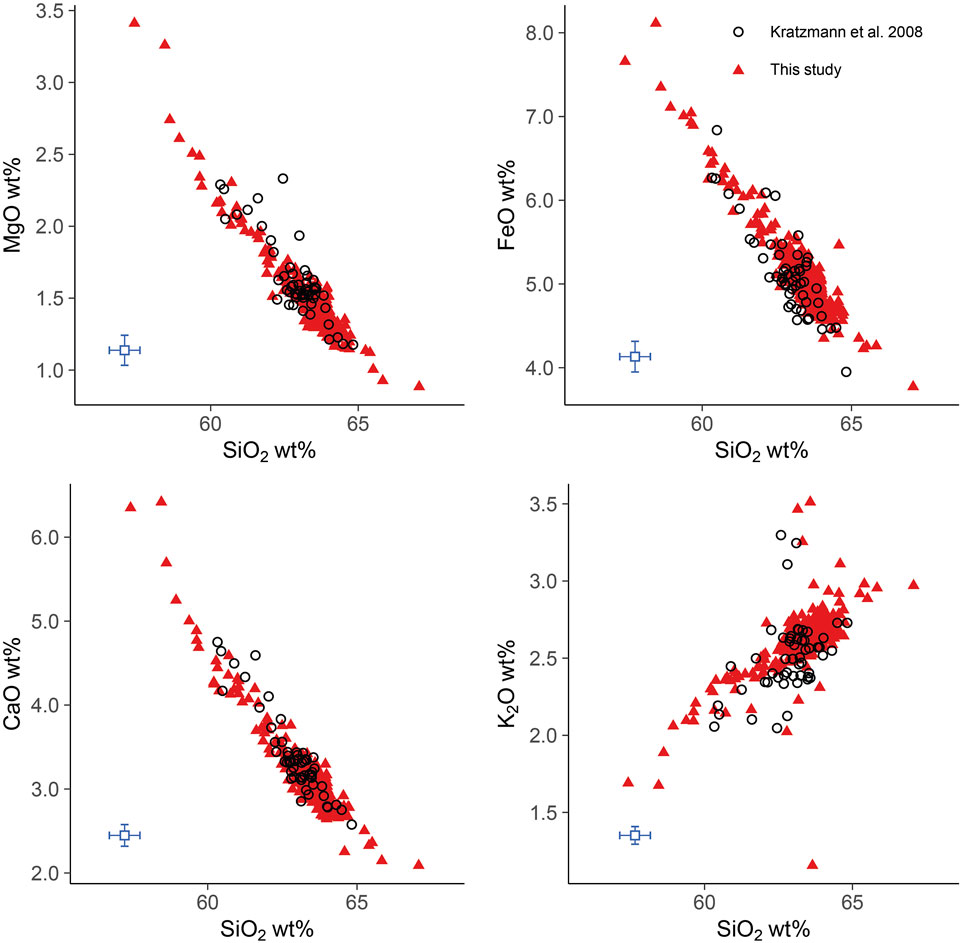
Figure 8. Major elements geo-chemistry of collected tephra samples, plotted with reference data of previously collected material (Kratzmann et al., 2009). Error bars show two standard deviation values from on the closest matrix matched secondary standard (StHs6/80-G, Jochum et al., 2006).
4 Discussion
Geochemical results and the stratigraphic position of the tephra layer near the surface or lake bed confirmed that we found the CH1991 tephra in all the locations we surveyed. However, there are differences in the preservation of primary tephra in both lake and terrestrial settings and in northern versus southern sites. These differences are plausibly associated with meteorological conditions, vegetation cover and depositional processes in the contrasting environments that are discussed below. We evaluate the preservation of tephra in three ways: 1) the quality of preservation, which is the correspondence between the preserved deposits and the characteristics of the original fallout, 2) the ubiquity of the preservation, which is how widely across the landscape a deposit is preserved, and 3) the longevity of the deposit, which is how likely that deposit is to be preserved over geological timescales. We consider these aspects of tephra preservation first in terms of the quality of the preservation (Section 4.1), then look at lakes vs. terrestrial in terms of all three aspects (quality, ubiquity, longevity) (Section 4.2), and finally look at differences in these three aspects between lakes (Section 4.3). We summarise the different aspects of preservation for our environments in Table 4; Figure 9, and discuss below.
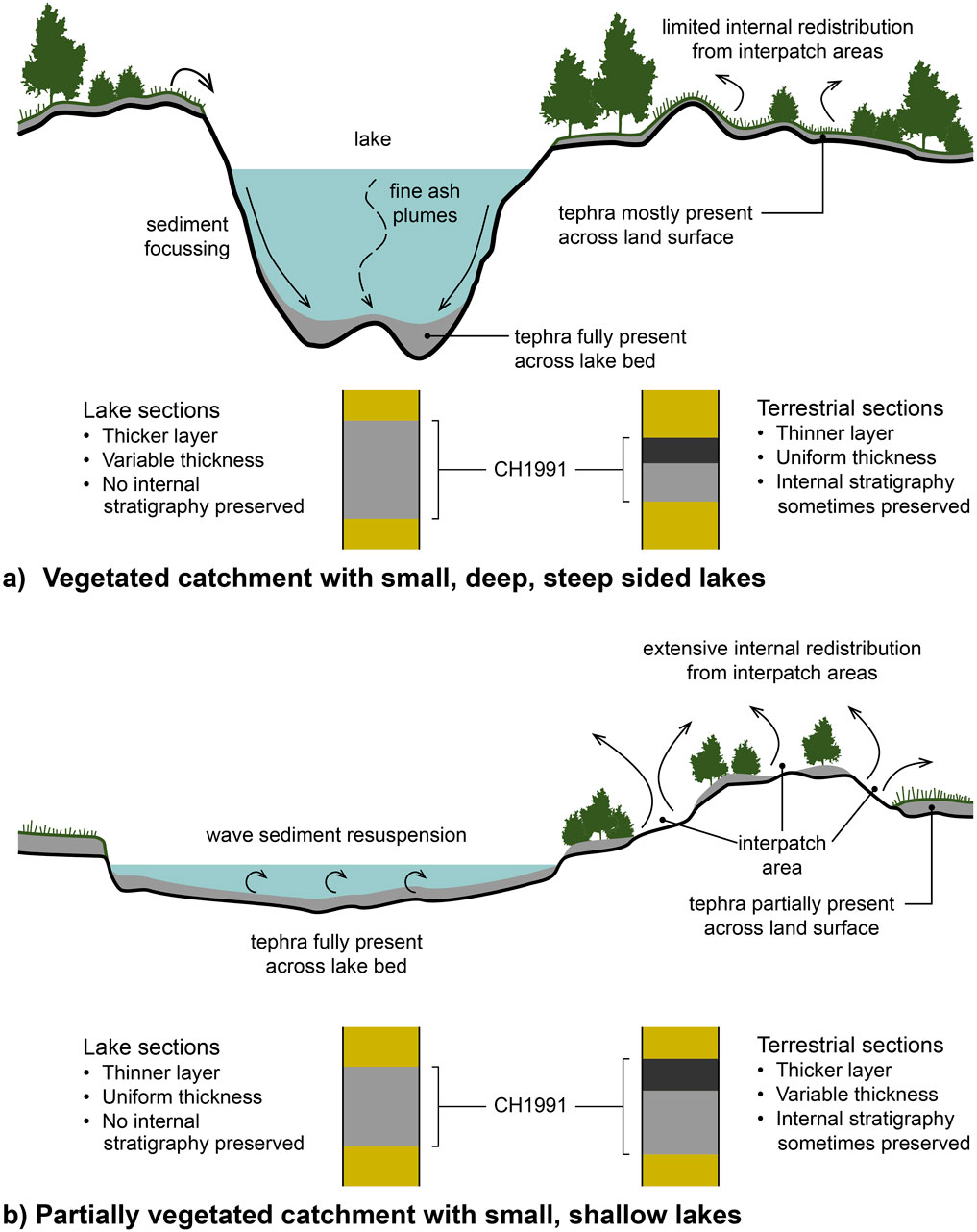
Figure 9. Schematic illustration showing tephra preservation of Cerro Hudson 1991 across a range of environments. Panel (A) shows the typical environments and tephra preservation found in the northern sites and (B) shows the typical environments and tephra preservation found in the southern sites.
4.1 Preservation of original fallout characteristics
The utility of tephra layers for reconstructing eruptions depends on the preserved deposits reflecting primary volcanological and depositional processes. However, secondary post-eruptive processes (e.g., aeolian remobilisation) can obscure this signal. Tephra layers formed by the localised redistribution of tephra from an earlier eruption have been observed in many palaeoenvironmental sequences (Thompson et al., 1986; Boygle, 1999; McGuire et al., 2022) and are a major challenge for constructing chronologies and for correlating among cores (Freundt et al., 2023). Secondary processes have been neglected in the past but are now a topic of increased interest (Jarvis et al., 2020). Previous comparisons of extant tephra deposits and syn-eruptive measurements have found variable, but generally good, levels of preservation, in terms of the correspondence between thickness, mass loading and GSD (Cutler et al., 2018; Cutler et al., 2020; Cutler et al., 2021). We consider the quality of preservation of the original primary tephra fallout deposit in contrasting environments, humid northern vs. dry southern sites, and lacustrine vs. terrestrial, based on the GSD and thickness, discussed in Section 4.2.
It seems likely, based on the high degree of sorting, lack of clear stratigraphy and variable GSDs, that many of the tephra deposits we observed consisted, at least partially, of reworked, secondary material. Measurements of the 1991 Hudson deposit by Scasso et al. (1994) and Banks and Iven (1991) were taken so soon after the eruption that the authors could clearly distinguish between primary and reworked deposits (though some post-depositional processes such as compaction can begin very rapidly after tephra fall: Blong et al., 2017). A strong windstorm started on 16 August 1991, directly after the end of the eruption, and remobilised the freshly deposited tephra (Scasso et al., 1994). We also know from more recent observations that tephra deposits from CH1991 were remobilised by wind for years after the eruption (Wilson et al., 2010). However, this does not seem to have affected our deposits so much that the original volcanological signal of the eruption is totally obscured. This observation accords with similar studies in other areas. For example, contemporary observations of tephra deposits from the 1980 eruption Mt St Helens found that the deposits were initially highly mobile but after 6 years had become stabilised, even in areas where there was little to no vegetation cover (Collins and Dunne, 2019). Recent measurements of Mt St Helens deposits in sparsely vegetated areas of eastern Washington State concluded that there had been relatively little alteration in the nearly 40 years since the original fallout (Cutler et al., 2018; Cutler et al., 2021). A study of the 2011 Cord\xF3n Caulle eruption, about 600 km north of Cerro Hudson, found that aeolian remobilisation of tephra reduced dramatically after a significant rainfall event, but this may reflect local circumstances rather than a more general outcome (Forte et al., 2018). Altogether, these studies suggest that even in environments prone to aeolian remobilisation, preservation of the original fallout characteristics, under certain conditions (e.g., vegetated areas, humid conditions), can be good.
Perhaps surprisingly, given the sparse vegetation and windy climate of the region, and despite direct observation in the field of patches of the CH1991 tephra being eroded at the ground surface today, the CH1991 tephra still presented as a single, discrete layer at all of our (carefully selected) sampling locations. At least five lines of evidence suggest that the tephra layer we observed was the result of a combination of primary airfall deposition and/or reworking during a short period (weeks to months) after the event, rather than a wholesale re-working of the original deposit over years or decades: a) the layer was geochemically homogenous; b) clear contacts between tephra and overlying non-tephra sediment were observed in the majority of sections; c) there was only one tephra layer representing CH1991 at our sites (thinner deposits presumably from earlier eruptions were present at depth at a few sites); d) the tephra layer thicknesses were typically similar to measurements taken at the time of the eruption and e) we did not observe sedimentary structures indicative of wholesale or extended remobilisation at the terrestrial sites (although we did not sub-sample layers for grain-size analysis, or analyse particle shape, approaches that might find evidence of remobilisation). Together, these lines of evidence suggest that the landscape stabilized quite rapidly after the eruption and that ensuing reworking has been patchy in time and space. Certainly, we saw no evidence of discrete layers representing episodes of secondary remobilisation and redeposition, as has been claimed in some previous studies (Thompson et al., 1986; Boygle, 1999; McGuire et al., 2022).
We could not discern well defined stratigraphic units within the CH1991 tephra layer in most lake cores or terrestrial sections. In a few instances, colour or GSD changes were visible, but at most two or three units could be differentiated (e.g., the colour change at top of the core illustrated in Figure 4H). This contrasts with the distinct stratigraphic units observed near our southern locations in the weeks following the 1991 eruption: up to ten units were recorded at Chile Chico (Scasso et al., 1994). The units recorded in 1991 typically alternated between fine light-grey ash and coarse grey ash and have been assumed to reflect variations in eruption intensity. Scasso et al. (1994) also noted that laminated wind-remobilised deposits were present at the top of many sections in this area; we did not observe this feature. In lakes, the particle settling regime, which is largely dependent on particle size and water depth, determines whether the tephra fallout stratigraphy is preserved (McNamara et al., 2019). McNamara et al. (2019) found good preservation of stratigraphy in their lake cores from the Chilean Andes, including in lakes of similar depth to ours (10–20 m), which they attributed to volcanic particles settling individually through the water column, rather than settling collectively in plumes. That we did not see any clearly identifiable units in our lake cores might be because the GSD in our samples was much finer than the tephra studied by McNamara et al. (2019), as our lakes were further from the volcano (75–106 km compared to 13–20 km). Fine ash is more likely to be affected by mixing during deposition due to its significantly longer residence time in the atmosphere and in the water column (Rose and Durant, 2009; Freundt et al., 2023). In particular circumstances, ash can be transported through the water column as gravitationally unstable dense plumes of particles, moving at far faster speeds than individual particles (Rose and Durant, 2009; Manville and Wilson, 2004; Freundt et al., 2023). Deposits resulting from sediment plumes will preserve overall GSDs but not the stratigraphic structures of fallout landing on the water surface. It seems plausible that at least the finer tephra at our sites may have settled from plumes, as whilst the overall GSD is similar to the primary deposit, the preserved stratigraphy does not reflect the primary deposit. Our results could also suggest that much (perhaps most) of the tephra in lake cores we studied had been remobilised or modified to some extent, either during the transport to the lake bed or shortly after its deposition on the lake bed, e.g., by bioturbation at our shallower southern lakes. Finally, it is possible that the lack of any clearly identifiable units in our samples could result from the sampling method we used (i.e., difficulties observing the tephra through the clear core tube), and the fact that we did not sub-sample the lake cores to look for grain size distribution changes through the layer, or undertake particle shape analysis, which has been used elsewhere to investigate processes of tephra reworking (Dominguez et al., 2020). Generally, the preservation of units within the CH1991 layer was poor in all deposits, terrestrial and lacustrine, limiting the extent to which these tephra deposits could be used to reconstruct eruptive styles, for instance variations in eruption intensity noted by Scasso et al. (1994).
4.2 Preservation in lakes compared to terrestrial sites
We aimed to compare preservation of tephra among small lakes, which are known to be good long-term preservation environments but not always common in a landscape, and soil sections, which are usually more widespread. Lakes do not passively accumulate tephra; its deposition and preservation involve a range of processes which are distinct from those that apply to soils and sub-aerial sediments. For example, lakes may recruit tephra by inwash from their catchments, particularly if they are fed by inflowing streams (Bertrand et al., 2014). We chose small lakes with small catchments and no or few inflows, to minimize the possibility of reworking, but nonetheless the quality of the preservation in our lakes is not notably better (measured by the degree of correspondence between our measurements of tephra thickness and GSD, and the records from 1991) than in nearby terrestrial environments, with only minor differences (Table 4; Figure 9). Previous studies have also shown that terrestrial sites can preserve tephra similarly well to lake records. For instance, in Iceland, Boygle (1999) found that no single lake core had a complete record of tephra fall in the area, and that preservation was not notably better in lake sediments than on land. Watson et al. (2016), studying cryptotephra preservation in peatlands and lakes in northwestern Europe, showed that preservation was similar in both kinds of site (albeit with much variability and incompleteness in individual records). Similarly, for the Mt Mazama tephra in North America, Buckland et al. (2020) found that lakes did not have notably better preservation than terrestrial sites. However, the difference in the quality of preservation between lake and terrestrial sites is likely to be sensitive to the properties of different terrestrial environments (e.g., rainfall, level of vegetation cover, surface roughness, windspeed), the properties of different lakes (e.g., basin morphology, currents, inflows).
We expected the tephra layer in the lakes to be more consistent and more similar to the initial deposit than the terrestrial samples in terms of thickness and grain size distribution (stratigraphy has been discussed in Section 4.1), on the grounds that tephra deposited on land should be more prone to (spatially variable) reworking by wind in the months and years following the eruption. However, this was not the case. Although the tephra layer in the northern lakes was slightly thicker and more variable in thickness than the tephra in adjacent terrestrial sites, the difference in thickness was small in absolute terms and the tephra layer in all three settings (lake, heath and woodland) approximated the initial deposit thickness (Figures 5, 9). In the south, the tephra layer in the lake was thinner than in terrestrial locations (Figures 5, 9). Calculations of mass loading suggest that the apparent thinning of the tephra in the southern lakes may simply reflect a greater degree of deposit compaction in the deposits in the southern lakes (Table 2); a possible mechanism for this is discussed in Section 4.3. The grain size distributions were similarly complex. We expected the lakes to be more effective than terrestrial surfaces at retaining fine material (both from the original fallout and from remobilised material); as such, we expected lacustrine sediments to be enriched in finer fractions and adjacent terrestrial sites to be depleted (winnowed). We did not observe this, with a similar proportion of lake and terrestrial sites having a GSD similar to the original fallout (Figure 6). Overall, there were no systematic differences in the quality of preservation between lake and terrestrial sites (although see Section 4.3 for further discussion of the variability between different lakes).
Where terrestrial environments are unstable or frequently disturbed, for example, in the semi-desert areas to east of the Andes in our study areas, lakes may be the only landscape locations where tephra is preserved reliably in the long run, especially from smaller eruptions and over centennial to millennial timescales (Fontijn et al., 2014; Smith et al., 2019). However, our study suggests that, over shorter periods (on the order of decades), lakes do not necessarily provide a more accurate record of the initial tephra fallout than the best preservation sites on land; in some respects (e.g., the detailed stratigraphy) they are inferior. Further research which aims to understand the stability of terrestrial records over longer timescales (e.g., Bolos et al., 2021) is needed, not least because, for older eruptions, the contrast between incomplete preservation on land and more reliable preservation in lakes is likely to become more pronounced over time, especially in arid or disturbed areas.
Understanding the difference in preservation potential matters because sampling terrestrial sites offers several advantages over lakes; they are generally more easily and widely found; easier to sample, and easier to collect observations with a wider spatial coverage. An exception to this is where lake levels have changed over time and there are now extensive lacustrine sediments on land, allowing observations to be collected over large spatial areas (e.g., Fontijn et al., 2018). Studying the lateral variations in these deposits offers an opportunity to better understand the variations observed in lake cores. The degree of spatial coverage is an important consideration when trying to reconstruct isopachs (Cutler et al., 2020). It is also easier at terrestrial sites to observe any relevant factors which have affected the preservation such as vegetation cover and local topography. Equivalent factors may be hard or impossible to observe on a lake bed. We did not select terrestrial sites at random within our study areas, so we almost certainly selected locations in the landscape with favourable characteristics for tephra preservation (e.g., more sheltered locations with high levels of vegetation cover). There were many locations on land which had not preserved any record of the eruption 29 years previously, but within the lake sediments tephra was found almost everywhere we sampled (on the few occasions we did not find tephra, this was likely due to problems with the application of the corer, or proximity to the lake shore). It may be there is a cut-off point in time where the better preservation of the layer in lakes becomes more important than the extra challenges that lake coring (and interpretation of lake records) presents. In this sense, the lakes did have a more robust and ubiquitous (within the lake sediments) record of the tephra fallout, and one which is likely to persist over a long period (Table 4; Figure 9).
4.3 Role of lake and catchment characteristics
Our two study areas received comparable amounts of tephra during the 1991 eruption, but the lakes that we sampled differ in terms of surface area and catchment size, bathymetry, and catchment vegetation cover. Here we explore how these factors have affected the preservation of tephra in these lakes over the period between the eruption in 1991 and our survey 29 years later.
There was some variation in the characteristics of the lakes that we sampled, though all were small compared with some that have been studied previously. Three of them (Laguna de los Patos and Laguna Magdalena in the northern study area, and Laguna de los Pescados in the south) were very small (<0.05 km2), had catchments of less than 0.5 km2 and lacked notable inflows. The other three lakes (Laguna Serena in the north, Laguna de los Flamencos and Laguna sin Nombre in the south) were larger (up to 0.5 km2), had larger catchments (all >1.8 km2) and had small inflows. In the northern lakes, tephra thickness tended to be similar to, or higher than, the original fallout thickness (Figures 5A, 9). In the southern lakes, the layer was similar or slightly thinner than the original fallout (Figures 5B, 9). In both cases the measurements from 1991 are not directly comparable to ours as they were taken several kilometre distance from our sites, but the differences between the original measurements and our measurements are small. We did not observe significant over-thickening in most instances: the thickest layer we observed represented 168% of the probable fallout thickness at the lake, and all other layers were <140%. This is in contrast to reported tephra deposits in larger lakes (>150 km2) elsewhere in Patagonia, where tephra layers >200% of the fallout thickness have been observed (Bertrand et al., 2014; McNamara et al., 2019). These over-thickened deposits were attributed to within-lake turbidity currents and extensive inflows from fluvial systems (Bertrand et al., 2014; McNamara et al., 2019). The lack of such over-thickened deposits at our sites likely reflects the small size and limited or lack of inflows of the lakes we sampled, and supports the notion that records from lakes like these can reliably be used to estimate past tephra fallout thickness.
There was considerable variation in tephra thickness measurements within and between lakes (Figure 5). The variability between the lakes was only slightly lower in the south (coefficient of variation, CV = 31%) than in the north (CV = 37%). Tephra thickness in Laguna Serena – the largest of the northern lakes by a factor of six – was notably less variable than in the other lakes in this location (Figure 5A). Wave height is likely to be higher in the larger lakes due to a longer fetch, increasing the likelihood of sediment mixing at the lake bed (assuming a similar lake depth), perhaps reducing thickness variability. Lake bathymetry is also known to affect within-lake rates of sediment accumulation (e.g., Blais and Kalff, 1995). The northern lakes were in deep, rocky basins (Table 1) and our depth measurements indicated variability in the bottom profile – for instance, beyond the immediate lake shore area, Laguna Magdalena varied in depth over 4.5–8.5 m. Deeper (and therefore steeper) basins within the lakes could have concentrated tephra in some areas, leading to higher variability in tephras layer thickness and perhaps explaining the slight tendency for tephra in these lakes to be thicker than was recorded in 1991. In contrast, the southern lakes were uniformly shallow. Lake depth will also determine the degree to which surface processes (e.g., wind-induced wave action) rework tephra deposits. The lake bed sediments in the shallow southern lakes were therefore more vulnerable to disturbance by wave action. The lower variability in tephra thickness in the southern lakes may be because the tephra deposit has been resuspended and homogenised over the whole lake bed. The mass loading and dry bulk density data (Table 2) support this idea, both being greater at the southern sites than at the northern sites (in contrast to the generally thinner deposits in the south). This suggests greater levels of compaction of the deposit in the southern lakes. One plausible explanation could be that degradation of organic material in the lake sediments affects the degree of compaction (Maier et al., 2013), with higher rates of degradation in the shallower southern sites. Alternatively, repeated resuspension and settling of sediment in these shallower sites might have encouraged more efficient sediment packing. However, the mechanics of compaction in lake sediments are generally poorly known (Bennett and Buck, 2016).
Catchment vegetation cover varied between the northern and southern sampling locations. The northern catchments experience relatively cool, humid conditions, with correspondingly dense vegetation cover by comparison with the more arid southern sites, which have large areas of exposed soil/sediment. Vegetation is known to trap and stabilise fine tephra by reducing aeolian remobilisation, with taller/denser vegetation leading to more tephra retention (Cutler et al., 2016a; Morison and Streeter, 2022); the presence of moisture can also bind tephra grains together and encourage the development of stabilising biocrusts (Cutler et al., 2018). Therefore, one would expect a greater degree of aeolian remobilisation in the southern sites. Somewhat surprisingly, we found that tephra thicknesses measured in the southern lakes were thinner than in the terrestrial sections in their catchments; these terrestrial records were also more variable in thickness than their northern equivalents. This suggests that the relatively small areas of the catchment that are vegetated and able to retain tephra have a tendency to capture material reworked from nearby unvegetated areas and become “overthickened”; an effect that has been observed extensively in Iceland (Cutler et al., 2016b; Dugmore et al., 2020). The lake records did not seem to suffer from this effect appreciably.
4.4 Implications for tephrochronology and volcanology
Individual samples from both lake and terrestrial environments were highly variable, varying by up to a factor of three within a given lake or catchment. This result strongly indicates that taking a single core in a suitable lake is not sufficient to reliably estimate the properties of past eruptions. However, aggregating the data from several cores or sections gave a more coherent signal. The mean layer thickness approximated the 1991 deposit thicknesses in all of our study locations. Moreover, the aggregate grain size distribution was a good match for the records made by Scasso et al. (1994) and Banks and Iven (1991), once differences in GSD measurement methods are taken into account, with a clear bimodal distribution (Figure 6). Averaging measurements in this way appears to allow the signal of the initial deposit to be extracted from the noise generated by site-specific processes. Accordingly, we propose that the most robust sampling strategy for is to make multiple (>5) measurements from a range of preservation environments.
Overall, our small lakes proved to be good repositories for tephra layers. We found CH1991 tephra in almost every location that we sampled. Small lakes have a considerable advantage over larger lakes in terms of ease of sampling, and perhaps also by their relative simplicity in terms of sedimentary processes. However, detailed preservation of tephrostratigraphy is unlikely in lake sediments where a) the fallout contains a high proportion of fine ash and b) lakes are shallow and/or have uneven bed profiles. While very thin tephra layers might thus be difficult to sample reliably, tephra of 2 cm thickness or more can be expected to be preserved indefinitely in lakes, and for at least several decades even at erosion-prone terrestrial sites like ours, with a fidelity that can be usefully exploited for palaeovolcanological and geochronological applications.
The findings of this and other studies suggest that we are beginning to be able to characterise the ‘quality’ of preservation in different environments in terms of (a) the faithfulness of the preservation of the fallout, (b) the ubiquity of the record and (c) its longevity (Table 4). The likely range of preservation potential in terrestrial environments spans the excellent preservation of well vegetated, humid, terrestrial environments such as Icelandic woodland and temperate forest in the USA (Morison and Streeter, 2022; Cutler et al., 2018) to the relatively poor preservation in arid terrestrial settings (Dominguez et al., 2020). Our Patagonian sites sit somewhere in between these end members. Characterizing the quality of preservation in lake settings is harder, due to the extra complexity of within-lake processes and the sensitivity to the deposit characteristics (especially GSD). Preservation is more variable in large lakes with inflows, probably a result of the complex interaction of processes which affect deposits there (McNamara et al., 2019; Bertrand et al., 2014). This study suggests that preservation is generally good in smaller lakes, albeit affected by the lake and catchment characteristics.
5 Conclusion
With the exception of within-layer stratigraphy, other aspects of tephra preservation (thickness, GSD) were good at all the sites (lacustrine, terrestrial, northern and southern), despite the extensive aeolian remobilisation of tephra which has occurred since the 1991 eruption, particularly in our southern sites.
We anticipated that preservation quality of the tephra deposit would be better in the northern lakes than those to the south due to the more favourable climatic and vegetation cover conditions; there was some evidence to support this (i.e., the thickness data was closer to that on the published isopach map), suggesting that catchment characteristics have some bearing on the tephra signal retained by lakes. However, there was relatively little difference in the quality of preservation between southern and northern sites. Preservation of the tephra deposit was highly idiosyncratic, and the lake deposits were not necessarily more faithful to the original fallout, but we expect the lake records to preserved over much longer time periods. Overall, our findings indicate that tephra layer formation is a complex process that is highly variable on small spatial scales. Given the spatial variability in preservation, it is unlikely that a single sample will be representative of the initial tephra deposit in any given area. However, a systematic sampling strategy that combines measurements from different settings may provide key information about the primary deposit and post-depositional processes. We therefore recommend that, where possible, volcanological reconstruction from tephra layers utilises averaged measurements, preferably from different sedimentary archives, to infer the properties of the initial deposit.
Data availability statement
The datasets presented in this study can be found in online repositories. The names of the repository/repositories and accession number(s) can be found below: The geochemical data generated in this paper are fully available in the EarthChem data repository (https://doi.org/10.60520/IEDA/113146). Grain size distribution and thickness data from this study can be accessed at the University of St Andrews Research Portal https://doi.org/10.17630/c8d9774d-e669-433e-9da7-53038c5c81d1.
Author contributions
RS: Conceptualization, Data curation, Formal Analysis, Funding acquisition, Investigation, Methodology, Writing–original draft, Writing–review and editing. NC: Conceptualization, Formal Analysis, Investigation, Methodology, Visualization, Writing–original draft, Writing–review and editing. IL: Investigation, Writing–review and editing. WHu: Formal Analysis, Writing–review and editing. LD: Writing–review and editing. WHi: Investigation, Writing–review and editing.
Funding
The author(s) declare that financial support was received for the research, authorship, and/or publication of this article. This work was funded by a Research Incentive Grant RIG008652 from the Carnegie Trust to RS, NC and IL. The use of the Electron Microprobe at the University of St Andrews was supported by the EPSRC Light Element Analysis Facility Grant EP/T019298/1 and the EPSRC Strategic Equipment Resource Grant EP/R023751/1. William Hutchison is funded by a UKRI Future Leaders Fellowship (MR/S033505/1).
Acknowledgments
We would like to thank Brian Reid of Centro de Investigación en Ecosistemas de la Patagonia (CIEP) for advice in planning the fieldwork and on local sites, and to thank the landowners for generously allowing permission to access the sites. We would also like to thank Roberto Scasso for discussions about the observations of the tephra shortly after the eruption, Sam Engwell for discussions in the early part of the project, and Georg Kodl for assistance with the remote sensing datasets.
Conflict of interest
The authors declare that the research was conducted in the absence of any commercial or financial relationships that could be construed as a potential conflict of interest.
Publisher’s note
All claims expressed in this article are solely those of the authors and do not necessarily represent those of their affiliated organizations, or those of the publisher, the editors and the reviewers. Any product that may be evaluated in this article, or claim that may be made by its manufacturer, is not guaranteed or endorsed by the publisher.
Supplementary material
The Supplementary Material for this article can be found online at: https://www.frontiersin.org/articles/10.3389/feart.2024.1433960/full#supplementary-material
References
Arnalds, O., Thorarinsdottir, E. F., Thorsson, J., Waldhauserova, P. D., and Agustsdottir, A. M. (2013). An extreme wind erosion event of the fresh Eyjafjallajökull 2010 volcanic ash. Sci. Rep. 3, 1257. doi:10.1038/srep01257
ASF DAAC (2015). ALOS PALSAR_Radiometric_Terrain_Corrected_high_res; includes material ©JAXA/METI 2007. doi:10.5067/Z97HFCNKR6VA
Banks, N. G., and Iven, M. (1991). Report of the united nations mission to Volcán Hudson, Chile, 20 August-15 September 1991. Report, US geol surv. US: Cascades Volcano Observatory, 1–61.
Bennett, K., and Buck, C. E. (2016). Interpretation of lake sediment accumulation rates. Holocene 26, 1092–1102. doi:10.1177/0959683616632880
Bertrand, S., Daga, R., Bedert, R., and Fontijn, K. (2014). Deposition of the 2011-2012 Cordón Caulle tephra (Chile, 40°S) in lake sediments: implications for tephrochronology and volcanology: tephra deposition in lake sediments. J. Geophys. Research-Earth Surf. 119, 2555–2573. doi:10.1002/2014jf003321
Blais, J. M., and Kalff, J. (1995). The influence of lake morphometry on sediment focusing. Limnol. Oceanogr. 40, 582–588. doi:10.4319/lo.1995.40.3.0582
Blong, R., Enright, N., and Grasso, P. (2017). Preservation of thin tephra. J. Appl. Volcanol. 6, 10. doi:10.1186/s13617-017-0059-4
Blott, S. J., Croft, D. J., Pye, K., Saye, S. E., and Wilson, H. E. (2004). Particle size analysis by laser diffraction. Geol. Soc. Lond Spec. Publ. 232, 63–73. doi:10.1144/gsl.sp.2004.232.01.08
Bolós, X., Macias, J. L., Ocampo-Díaz, Y. Z. E., and Tinoco, C. (2021). Implications of reworking processes on tephra distribution during volcanic eruptions: the case of Parícutin (1943–1952, western Mexico). Earth Surf. Process. 46, 3143–3157. doi:10.1002/esp.5222
Boygle, J. (1999). Variability of tephra in lake and catchment sediments, Svinavatn, Iceland. Glob. Planet. Change 21, 129–149. doi:10.1016/s0921-8181(99)00011-9
Buckland, H. M., Cashman, K. V., Engwell, S. L., and Rust, A. C. (2020). Sources of uncertainty in the Mazama isopachs and the implications for interpreting distal tephra deposits from large magnitude eruptions. B Volcanol. 82, 23. doi:10.1007/s00445-020-1362-1
Collins, B. D., and Dunne, T. (2019). Thirty years of tephra erosion following the 1980 eruption of Mount St. Helens. Earth Surf. Process. Landf. 44, 2780–2793. doi:10.1002/esp.4707
Costa, A., Folch, A., Macedonio, G., Giaccio, B., Isaia, R., and Smith, V. C. (2012). Quantifying volcanic ash dispersal and impact of the Campanian Ignimbrite super-eruption. Geophys. Res. Lett. 39. doi:10.1029/2012gl051605
Cutler, N. A., Bailey, R. M., Hickson, K. T., Streeter, R. T., and Dugmore, A. J. (2016a). Vegetation structure influences the retention of airfall tephra in a sub-Arctic landscape. Prog. Phys. Geogr. 40, 661–675. doi:10.1177/0309133316650618
Cutler, N. A., Shears, O. M., Streeter, R. T., and Dugmore, A. J. (2016b). Impact of small-scale vegetation structure on tephra layer preservation. Sci. Rep. 6, 37260–37313. doi:10.1038/srep37260
Cutler, N. A., Streeter, R. T., Dugmore, A. J., and Sear, E. R. (2021). How do the grain size characteristics of a tephra deposit change over time? B Volcanol. 83, 45. doi:10.1007/s00445-021-01469-w
Cutler, N. A., Streeter, R. T., Engwell, S. L., Bolton, M. S., Jensen, B. J. L., and Dugmore, A. J. (2020). How does tephra deposit thickness change over time? A calibration exercise based on the 1980 Mount St Helens tephra deposit. J. Volcanol. Geoth Res. 399, 106883. doi:10.1016/j.jvolgeores.2020.106883
Cutler, N. A., Streeter, R. T., Marple, J., Shotter, L. R., Yeoh, J. S., and Dugmore, A. J. (2018). Tephra transformations: variable preservation of tephra layers from two well-studied eruptions. Bull. Volcanol. 80, 77. doi:10.1007/s00445-018-1251-z
Davies, S. M., Abbott, P. M., Pearce, N. J. G., Wastegård, S., and Blockley, S. P. E. (2012). Integrating the INTIMATE records using tephrochronology: rising to the challenge. Quat. Sci. Rev. 36, 11–27. doi:10.1016/j.quascirev.2011.04.005
Dugmore, A., Streeter, R., and Cutler, N. (2018). The role of vegetation cover and slope angle in tephra layer preservation and implications for Quaternary tephrostratigraphy. Palaeogeogr. Palaeoclimatol. Palaeoecol. 489, 105–116. doi:10.1016/j.palaeo.2017.10.002
Dugmore, A. J., Thompson, P. I. J., Streeter, R. T., Cutler, N. A., Newton, A. J., and Kirkbride, M. P. (2020). The interpretative value of transformed tephra sequences. J. Quat. Sci. 35, 23–38. doi:10.1002/jqs.3174
Durán, A. P., Casalegno, S., Marquet, P. A., and Gaston, K. J. (2013). Representation of ecosystem services by terrestrial protected areas: Chile as a case study. PLoS ONE 8, e82643. doi:10.1371/journal.pone.0082643
Dominguez, L., Bonadonna, C., Forte, P., Jarvis, P. A., Cioni, R., and Mingari, L. (2020). Aeolian remobilisation of the 2011-Cord\xF3n Caulle tephra-fallout deposit: example of an important process in the life cycle of Volcanic Ash Frontiers. Earth. Sci. 7, 343. doi:10.3389/feart.2019.00343
Engwell, S. L., Aspinall, W. P., and Sparks, R. S. J. (2015). An objective method for the production of isopach maps and implications for the estimation of tephra deposit volumes and their uncertainties. Bull. Volcanol. 77, 61–18. doi:10.1007/s00445-015-0942-y
Etyemezian, V., Gillies, J. A., Mastin, L. G., Crawford, A., Hasson, R., Eaton, A. R. V., et al. (2019). Laboratory experiments of volcanic ash resuspension by wind. J. Geophys Res. Atmos. 124, 9534–9560. doi:10.1029/2018jd030076
Fick, S. E., and Hijmans, R. J. (2017). WorldClim 2: new 1-km spatial resolution climate surfaces for global land areas. Int. J. Climatol. 37, 4302–4315. doi:10.1002/joc.5086
Fontijn, K., Lachowycz, S. M., Rawson, H., Pyle, D. M., Mather, T. A., Naranjo, J. A., et al. (2014). Late Quaternary tephrostratigraphy of southern Chile and Argentina. Quat. Sci. Rev. 89, 70–84. doi:10.1016/j.quascirev.2014.02.007
Fontijn, K., McNamara, K., Tadesse, A. Z., Pyle, D. M., Dessalegn, F., Hutchison, W., et al. (2018). Contrasting styles of post-caldera volcanism along the Main Ethiopian Rift: implications for contemporary volcanic hazards. J. Volcanol. Geotherm. Res. 356, 90–113. doi:10.1016/j.jvolgeores.2018.02.001
Forte, P., Domínguez, L., Bonadonna, C., Gregg, C. E., Bran, D., Bird, D., et al. (2018). Ash resuspension related to the 2011-2012 Cordón Caulle eruption, Chile, in a rural community of Patagonia, Argentina. J. Volcanol. Geotherm. Res. 350, 18–32. doi:10.1016/j.jvolgeores.2017.11.021
Freundt, A., Schindlbeck-Belo, J. C., Kutterolf, S., and Hopkins, J. L. (2023). Tephra layers in the marine environment: a review of properties and emplacement processes. Geol. Soc. Lond., Spéc. Publ. 520, 595–637. doi:10.1144/sp520-2021-50
Global Volcanism Program (2024). Cerro Hudson (358057) in [database] volcanoes of the world (v. 5.1.7; 26 apr 2024). Distributed by smithsonian institution, compiled by venzke, E. doi:10.5479/si.GVP.VOTW5-2023.5.1
Green, R. M., Bebbington, M. S., Jones, G., Cronin, S. J., and Turner, M. B. (2016). Estimation of tephra volumes from sparse and incompletely observed deposit thicknesses. Bull. Volcanol. 78, 25. doi:10.1007/s00445-016-1016-5
Hiles, W., Lawson, I. T., Roucoux, K. H., and Streeter, R. T. (2021). Late survival of woodland contrasts with rapid limnological changes following settlement at Kalmanstjörn, Mývatnssveit, northeast Iceland. Boreas 50, 1209–1227. doi:10.1111/bor.12529
Inman, D. L. (1952). Measures for describing the size distribution of sediments. J. Sediment. Res. 22, 125–145. doi:10.1306/d42694db-2b26-11d7-8648000102c1865d
Jarvis, P. A., Bonadonna, C., Dominguez, L., Forte, P., Frischknecht, C., Bran, D., et al. (2020). Aeolian remobilisation of volcanic ash: outcomes of a workshop in the argentinian Patagonia. Front. Earth Sci. 8. doi:10.3389/feart.2020.575184
Jochum, K. P., Stoll, B., Herwig, K., Willbold, M., Hofmann, A. W., Amini, M., et al. (2006). MPI-DING reference glasses for in situ microanalysis: new reference values for element concentrations and isotope ratios. Geochem., Geophys., Geosystems 7. doi:10.1029/2005gc001060
Kratzmann, D. J., Carey, S., Scasso, R., and Naranjo, J.-A. (2009). Compositional variations and magma mixing in the 1991 eruptions of Hudson volcano, Chile. B Volcanol. 71, 419–439. doi:10.1007/s00445-008-0234-x
Kratzmann, D. J., Carey, S. N., Fero, J., Scasso, R. A., and Naranjo, J.-A. (2010). Simulations of tephra dispersal from the 1991 explosive eruptions of Hudson volcano, Chile. J. Volcanol. Geotherm. Res. 190, 337–352. doi:10.1016/j.jvolgeores.2009.11.021
Laidler, G. J., Treitz, P. M., and Atkinson, D. M. (2009). Remote sensing of arctic vegetation: relations between the NDVI, spatial resolution and vegetation cover on boothia peninsula, nunavut. ARCTIC 61, 1–13. doi:10.14430/arctic2
Lane, C. S., Brauer, A., Blockley, S. P. E., and Dulski, P. (2013). Volcanic ash reveals time-transgressive abrupt climate change during the Younger Dryas. Geology 41, 1251–1254. doi:10.1130/g34867.1
Liu, E. J., Cashman, K. V., Beckett, F. M., Witham, C. S., Leadbetter, S. J., Hort, M. C., et al. (2014). Ash mists and brown snow: remobilization of volcanic ash from recent Icelandic eruptions, 1–18. doi:10.1002/(issn)2169-8996
Lowe, D. J. (2011). Tephrochronology and its application: a review. Quat. Geochronol. 6, 107–153. doi:10.1016/j.quageo.2010.08.003
Maier, D. B., Rydberg, J., Bigler, C., and Renberg, I. (2013). Compaction of recent varved lake sediments. GFF 135, 231–236. doi:10.1080/11035897.2013.788551
Manville, V., and Wilson, C. J. N. (2004). Vertical density currents: a review of their potential role in the deposition and interpretation of deep-sea ash layers. J. Geol. Soc. 161, 947–958. doi:10.1144/0016-764903-067
McGuire, A. M., Lane, C. S., Roucoux, K. H., Albert, P. G., and Kearney, R. (2022). The dating and correlation of an eastern Mediterranean lake sediment sequence: a 46–4 ka tephrostratigraphy for Ioannina (NW Greece). J. Quat. Sci. 37, 1313–1331. doi:10.1002/jqs.3452
McGuire, A. M., Lane, C. S., Roucoux, K. H., Lawson, I. T., Koutsodendris, A., Pross, J., et al. (2024). Campanian Ignimbrite tephra reveals asynchronous vegetation responses to abrupt climate change in the eastern Mediterranean region. Quat. Sci. Rev. 334, 108714. doi:10.1016/j.quascirev.2024.108714
McNamara, K., Rust, A. C., Cashman, K. V., Castruccio, A., and Abarzúa, A. M. (2019). Comparison of lake and land tephra records from the 2015 eruption of Calbuco volcano, Chile. Bull. Volcanol. 81, 10. doi:10.1007/s00445-019-1270-4
Morison, C., and Streeter, R. (2022). The influence of vegetation cover on the grain-size distributions and thicknesses of two Icelandic tephra layers. Volcanica 5, 227–248. doi:10.30909/vol.05.02.227248
Naranjo, J. A., and Stern, C. R. (1998). Holocene explosive activity of Hudson volcano, southern Andes. Bull. Volcanol. 59, 291–306. doi:10.1007/s004450050193
Oladóttir, B. A., Sigmarsson, O., Larsen, G., and Thordarson, T. (2008). Katla volcano, Iceland: magma composition, dynamics and eruption frequency as recorded by Holocene tephra layers. B Volcanol. 70, 475–493. doi:10.1007/s00445-007-0150-5
Panebianco, J. E., Mendez, M. J., Buschiazzo, D. E., Bran, D., and Gaitán, J. J. (2017). Dynamics of volcanic ash remobilisation by wind through the Patagonian steppe after the eruption of Cordón Caulle, 2011. Sci. Rep. 7, 45529. doi:10.1038/srep45529
Paredes-Mariño, J., Forte, P., Alois, S., Chan, K. L., Cigala, V., Mueller, S. B., et al. (2022). The lifecycle of volcanic ash: advances and ongoing challenges. B Volcanol. 84, 51. doi:10.1007/s00445-022-01557-5
Pyle, D. M. (1989). The thickness, volume and grainsize of tephra fall deposits. B Volcanol. 51, 1–15. doi:10.1007/bf01086757
Rose, W. I., and Durant, A. J. (2009). Fine ash content of explosive eruptions. J. Volcanol. Geotherm. Res. 186, 32–39. doi:10.1016/j.jvolgeores.2009.01.010
Scasso, R. A., Corbella, H., and Tiberi, P. (1994). Sedimentological analysis of the tephra from the 12–15 August 1991 eruption of Hudson volcano. Bull. Volcanol. 56, 121–132. doi:10.1007/bf00304107
Smith, R. E., Smith, V. C., Fontijn, K., Gebhardt, A. C., Wastegård, S., Zolitschka, B., et al. (2019). Refining the late quaternary tephrochronology for southern south America using the Laguna potrok aike sedimentary record. Quat. Sci. Rev. 218, 137–156. doi:10.1016/j.quascirev.2019.06.001
Smith, V. C., Costa, A., Aguirre-Díaz, G., Pedrazzi, D., Scifo, A., Plunkett, G., et al. (2020). The magnitude and impact of the 431 CE tierra blanca joven eruption of ilopango, El Salvador. Proc. Natl. Acad. Sci. 117, 26061–26068. doi:10.1073/pnas.2003008117
Stern, C. R. (2007). Holocene tephrochronology record of large explosive eruptions in the southernmost Patagonian Andes. Bull. Volcanol. 70, 435–454. doi:10.1007/s00445-007-0148-z
Thompson, P. I. J., Dugmore, A. J., Newton, A. J., Streeter, R. T., and Cutler, N. A. (2022). Variations in tephra stratigraphy created by small-scale surface features in sub-polar landscapes. Boreas 51, 317–331. doi:10.1111/bor.12557
Thompson, R., Bradshaw, R. H. W., and Whitley, J. E. (1986). The distribution of ash in Icelandic lake sediments and the relative importance of mixing and erosion processes. J. Quat. Sci. 1, 3–11. doi:10.1002/jqs.3390010102
Timms, R. G. O., Matthews, I. P., Lowe, J. J., Palmer, A. P., Weston, D. J., MacLeod, A., et al. (2019). Establishing tephrostratigraphic frameworks to aid the study of abrupt climatic and glacial transitions - a case study of the Last Glacial-Interglacial Transition in the British Isles (c. 16-8 ka BP). Earth Sci. Rev. 192, 34–64. doi:10.1016/j.earscirev.2019.01.003
Watson, E. J., Swindles, G. T., Lawson, I. T., and Savov, I. P. (2016). Do peatlands or lakes provide the most comprehensive distal tephra records?. Quat. Sci. Rev. 139, 110–128. doi:10.1016/j.quascirev.2016.03.011
Wilson, T. M., Cole, J. W., Stewart, C., Cronin, S. J., and Johnston, D. M. (2010). Ash storms: impacts of wind-remobilised volcanic ash on rural communities and agriculture following the 1991 Hudson eruption, southern Patagonia, Chile. Bull. Volcanol. 73, 223–239. doi:10.1007/s00445-010-0396-1
Keywords: tephra, preservation, lake core, aeolian remobilisation, tephrochronology
Citation: Streeter RT, Cutler NA, Lawson IT, Hutchison W, Dominguez L and Hiles W (2024) Variable preservation of the 1991 Hudson tephra in small lakes and on land. Front. Earth Sci. 12:1433960. doi: 10.3389/feart.2024.1433960
Received: 16 May 2024; Accepted: 23 September 2024;
Published: 03 October 2024.
Edited by:
Stephen Self, University of California, Berkeley, United StatesReviewed by:
Alison Claire Rust, University of Bristol, United KingdomPaul Albert, Swansea University, United Kingdom
Copyright © 2024 Streeter, Cutler, Lawson, Hutchison, Dominguez and Hiles. This is an open-access article distributed under the terms of the Creative Commons Attribution License (CC BY). The use, distribution or reproduction in other forums is permitted, provided the original author(s) and the copyright owner(s) are credited and that the original publication in this journal is cited, in accordance with accepted academic practice. No use, distribution or reproduction is permitted which does not comply with these terms.
*Correspondence: Richard T. Streeter, cnRzM0BzdC1hbmRyZXdzLmFjLnVr