- 1University Grenoble Alpes, IRD, CNRS, Grenoble INP, IGE, Grenoble, France
- 2Servicio Nacional de Meteorología e Hidrología (SENAMHI), Lima, Peru
- 3Escuela Ambiental, Universidad de Antioquia, Medellín, Colombia
- 4Departamento de Meteorología, Universidad de Valparaíso, Valparaíso, Chile
- 5Center for Climate and Resilience Research (CR)2, Santiago, Chile
- 6Center for Oceanographic Research COPAS COASTAL, Universidad de Concepción, Concepción, Chile
- 7Instituto Argentino de Nivología, Glaciología y Ciencias Ambientales (IANIGLA) – CONICET, Mendoza, Argentina
- 8Facultad de Ciencias Exactas y Naturales, Universidad de Buenos Aires, Buenos Aires, Argentina
- 9CONICET ‒ Universidad de Buenos Aires, Centro de Investigaciones del Mar y la Atmósfera (CIMA), Buenos Aires, Argentina
- 10Instituto Franco-Argentino de Estudios sobre el Clima y sus Impactos (IFAECI) – IRL 3351 – CNRS-CONICET-IRD-UBA, Buenos Aires, Argentina
- 11Department of Atmospheric Processes, Brandenburg University of Technology Cottbus-Senftenberg, Cottbus, Germany
- 12Departamento de Ingeniería Civil y Ambiental, Facultad de Ingeniería Civil y Ambiental, Escuela Politécnica Nacional, Quito, Ecuador
- 13Grupo de Investigación—MetClima, Escuela Politécnica Nacional, Quito, Ecuador
- 14Grupo de Ingeniería y Gestión Ambiental (GIGA), Escuela Ambiental, Facultad de Ingeniería, Universidad de Antioquia, Medellín, Colombia
- 15Department of Geophysics, Universidad de Chile, Santiago, Chile
- 16Centro de Estudios Avanzados en Zonas Áridas, La Serena, Chile
- 17Universidad Nacional de Colombia, Sede Bogotá, Colombia
- 18Universidad Nacional de Colombia, Sede Medellín, Colombia
- 19Departamento de Ciencias de la Atmósfera y los Océanos (DCAO), Facultad de Ciencias Exactas y Naturales, Universidad de Buenos Aires, Buenos Aires, Argentina
- 20Instituto de Investigación sobre la Enseñanza de las Matemáticas, Pontificia Universidad Católica del Perú (PUCP), Lima, Peru
In the Andes, the complex topography and unique latitudinal extension of the cordillera are responsible for a wide diversity of climate gradients and contrasts. Part I of this series reviews the current modeling efforts in simulating key atmospheric-orographic processes for the weather and climate of the Andean region. Building on this foundation, Part II focuses on global and regional climate models challenging task of correctly simulating changes in surface-atmosphere interactions and hydroclimate processes to provide reliable future projections of hydroclimatic trajectories in the Andes Cordillera. We provide a review of recent advances in atmospheric modeling to identify and produce reliable hydroclimate information in the Andes. In particular, we summarize the most recent modeling research on projected changes by the end of the 21st century in terms of temperature and precipitation over the Andes, the mountain elevation-dependent warming signal, and land cover changes. Recent improvements made in atmospheric kilometer-scale model configurations (e.g., resolution, parameterizations and surface forcing data) are briefly reviewed, highlighting their impact on modeling results in the Andes for precipitation, atmospheric and surface-atmosphere interaction processes, as mentioned in recent studies. Finally, we discuss the challenges and perspectives of climate modeling, with a focus on the hydroclimate of the Andes.
1 Introduction
In the Andes, the complex topography and unique latitudinal extension of the cordillera are responsible for a wide diversity of climate gradients and contrasts. From the cold extratropical Patagonia in the south to the wet tropical Northern Andes or the hyper-arid Atacama Desert in the subtropics, the region is facing global and regional environmental changes, including climate warming, glacier retreat, and land use changes (e.g., deforestation). These changes are related to strong socio-economic impacts in terms of water resources, ecosystems, energy and agriculture, among many others. Therefore, there is an urgent need for the scientific community to understand and project climate change over this unique environment. In particular, global and regional climate models (GCMs and RCMs, respectively) face a challenging task in correctly simulating changes in surface-atmosphere interactions and hydroclimate processes to provide reliable future projections of hydroclimatic trajectories in the Andes Cordillera.
Recently, limited area models (LAM) with kilometer-scale horizontal resolution have been increasingly used to improve local-scale atmospheric processes in climate simulations in Andean regions. Compared to previous generations of simulations with grid spacing in the range 25–200 km (both from GCMs and RCMs), kilometer-scale LAM simulations with grid spacings in the 1–25 km range show overall improvements in spatial and temporal variability of main climatic features over the Andes (e.g., Bozkurt et al., 2019; Falco et al., 2020; Gutierrez et al., 2024), and better-resolved local valley-wind and slope processes (e.g., Junquas et al., 2018; Bozkurt et al., 2019; Schumacher et al., 2020; Rosales et al., 2022). Weather and climate modeling of fundamental processes such as deep convection, convective organization, land-atmosphere interactions, the effects of landscape (e.g., mountains and valleys) and land-cover heterogeneity require a high level of detail in their spatiotemporal representation (e.g., Gutowski et al., 2016; Gutowski et al., 2020; Giorgi, 2019; Palmer and Stevens, 2019; Prein et al., 2015; Lucas-Picher et al., 2021). This is particularly critical for modeling the atmospheric phenomena affected by the Andes, where local circulations such as valley-wind breezes or orographic enhancement of cloudiness and precipitation interact with regional atmospheric processes such as low-level jets and gravity waves (e.g., Junquas et al., 2018; Bozkurt et al., 2019; Posada-Marín et al., 2019; Schumacher et al., 2020; Chimborazo and Vuille, 2021). Under climate projections, these processes interplay with large-scale atmospheric circulations perturbations, local and regional land cover changes and elevation-dependent warming, among others. These processes and their interactions strongly shape weather and hydroclimate in a wide scale range of resolution (i.e., 1–200 km) throughout the Andes. With high resolutions, it is possible to achieve a better depiction of “precipitation hotspots” associated with particular topographic features (e.g., Junquas et al., 2018), and statistical distributions of simulated precipitation that are more suitable for representing extreme precipitation events (e.g., Onol, 2012; Di Luca et al., 2013; Torma et al., 2015; Lucas-Picher et al., 2017; Giorgi, 2019; Baladima et al., 2022). Therefore, the recent improvement in resolution and complexities of climate models have driven the publication of high-resolution modeling studies in the entire Andes for the analyses of hydroclimate variables, that we propose to assess in this review paper.
However, the LAM models still face challenges associated with persistent precipitation biases in temporal and spatial variability, such as an overestimation in some part of the Andes like the southern tropical highlands (e.g., Mourre et al., 2016; Junquas et al., 2018; Junquas et al., 2022; Moya-Álvarez et al., 2018a; Moya-Álvarez et al., 2018b), the central Andes of Chile and Argentina (e.g., Rojas, 2006; Schumacher et al., 2020), and Patagonia (e.g., Villarroel et al., 2013). Such precipitation biases can be due to several factors, including the use of parametrizations for deep cumulus convection that is needed for models with grid spacings larger than ∼5 km, the type/configuration of other parametrizations (e.g., microphysics, radiation, etc.), topographic and hypsometric errors, and inadequate high-quality observational data, particularly over the high Andes (e.g., Bozkurt et al., 2019; Schumacher et al., 2020; Fernández et al., 2021; Hodnebrog et al., 2021). Similar difficulties are common to regions with mountainous terrain (e.g., Maussion et al., 2011; Bozkurt et al., 2012; Onol, 2012; Solman 2013; Bieniek et al., 2016). In addition, due to the presence of steep valleys, slopes, cities, glaciers, lakes and other highly localized surface features, and considering that the size of the smallest flow structure reproduced in a dynamical model is approximately 5–10 times the grid spacing of the simulation (Pielke, 2013), even a kilometer-scale simulation can barely represent all processes and systems in the Andes. In this review, we assess the added value of the resolution increase as well as our enhanced understanding of the processes to be resolved by the dynamical atmospheric models in order to produce the most reliable and localized hydroclimate trajectories and changing signals in the Andes.
This paper is the second part of two review papers focused on recent advances in climate modeling in the Andes. Part I (Martinez et al., in revision) proposes a review of the atmospheric processes and associated precipitation features described by recent modeling studies, such as mountain-related wind systems, low-level jets along the Andes, windstorms, gravity waves, and associated characteristics of diurnal cycle, altitudinal gradient, extreme events and orographic precipitation, among others. In this second part, we review the recent advances in atmospheric modeling to identify and produce reliable hydroclimate information in the Andes. In Section 2, we summarize modeling research on projected changes by the end of the 21st century in temperature and precipitation over the Andes, and how they are influenced by the mountain elevation-dependent warming signal and land cover changes. In Section 3, we describe the recent improvements made in atmospheric kilometer-scale model configurations (e.g., resolution, parameterizations and surface forcing data) and how they impact the modeling results in the Andes in terms of precipitation, atmospheric and surface-atmosphere interaction processes, as mentioned in previous studies. Finally, in Section 4, we discuss the challenges and perspectives of climate modeling, with a focus on the hydroclimate of the Andes.
2 Effects of climate and land use changes
In this section, we aim to review the most recent results associated with climate and land use changes in the Andes with a particular focus on modeling advances and improvements adapted to the hydroclimate complexity of the Andean regions.
2.1 Projected changes in precipitation and surface temperature
Pabón-Caicedo et al. (2020) summarized some results regarding climate projections for the Andes region. These authors compared ensembles of Coupled Model Intercomparison Project CMIP3 and CMIP5 models under historical conditions in the recent past (1971–2000) and future conditions (2071–2100) under the Representative Concentration Pathways (RCP; van Vuuren et al., 2011) RCP8.5 scenario. In general, these models agree on expecting higher temperatures over the Andes by the end of the century, although the magnitude of the change depends on the region and model (Figure 1). The robust signal of temperature increase for the end of the 21st century was confirmed by recent studies using CMIP6 models under Shared Socioeconomic Pathways (SSPs; Riahi et al., 2017) scenarios (e.g., Almazroui et al., 2021; Ortega et al., 2021; Figure 1), showing reduced biases in general with respect to CMIP5 models in South America (Ortega et al., 2021).
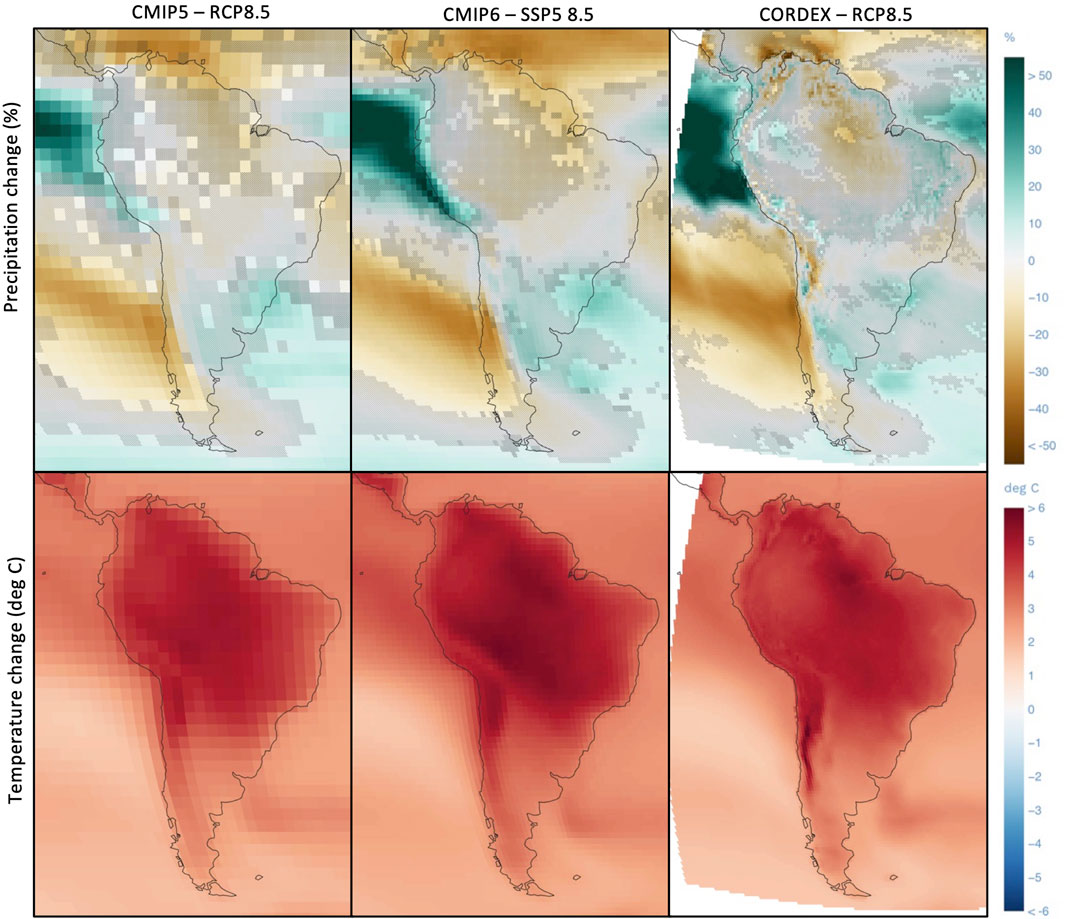
Figure 1. Long-term total annual precipitation change (top row; %) and temperature change (bottom row; °C) in South America for 2081–2100 with respect to 1995–2014 from 29 CMIP5 models (first column; 2°x2°), 33 CMIP6 models (second column; 1°x1°) and 18 CORDEX South America models (third column; 0.5°x0.5°). For precipitation, color grid point indicates that the change is robust and likely emerges from internal variability (at least 66% of the models show a change greater than the internal-variability threshold and at least 80% of the models agree on the sign of change); Light gray color indicates no change or no robust change (fewer than 66% of the models show change greater than the internal-variability threshold); Dark gray color indicates conflicting signals where at least 66% of the models show change greater than the internal-variability threshold but fewer than 80% of all models agree on the sign of change. https://interactive-atlas.ipcc.ch/.
In the case of precipitation, future climate projections are more heterogeneous in terms of sign of change among both generations of model and regions (e.g., Pabón-Caicedo et al., 2020; Almazroui et al., 2021; Ortega et al., 2021; Thaler et al., 2021). The most robust regional signal among GCMs from CMIP3 and CMIP5 in the Andes indicates a drying trend over Central Chile (e.g., Pabón-Caicedo et al., 2020; Figure 1), which is primarily attributed to the anthropogenic forcing signal (Boisier et al., 2018). While there is an overall trend of reduced rainfall throughout the year driven by greenhouse gas forcing, a summer rainfall decline in Chile is shown to be linked to the depletion of the ozone layer over Antarctica. This robust projected signal is also found with the generation of CMIP6 models (e.g., Almazroui et al., 2021; Ortega et al., 2021; Rivera and Arnould, 2020; Salazar et al., 2024), which are able to reasonably capture the long-term drying trend from the beginning of the 20th century (Rivera and Arnould, 2020). However, the observed decline is larger than simulated, suggesting future projections may underestimate the changes (Boisier et al., 2018; Rivera and Arnould, 2020).
Other projected signals of precipitation are found in CMIP6 models and consistent with earlier projections (CMIP3, CMIP5), as for example, a decrease over the east of the northern tropical Andes and northern Patagonia, and an increase over parts of the Andes in Colombia, Ecuador, Perú, Bolivia and northern Argentina (e.g., Pabón-Caicedo et al., 2020; Almazroui et al., 2021; Salazar et al., 2024; Figures 1, 2). The annual changes are mainly explained by the wetter part of the year (Almazroui et al., 2021), and opposite signals can be found considering the season of the year (Ortega et al., 2021; Thaler et al., 2021; Figure 2). In addition, most of these precipitation changes remain within the range of variability of the reference period (Almazroui et al., 2021). From an analysis of an ensemble of 7-best performing CMIP6 models, Ortega et al. (2021) found similar results for the northern-central Andes and central-southern Chile, suggesting stronger trends than the whole ensemble (Figure 2). This 7-best model selection was based on annual cycle and statistical evaluations (Taylor diagrams), in terms of precipitation and surface temperature (compared to satellite and reanalysis products, respectively) for a present period. However, the analysis of CMIP6 for Chile made by Salazar et al. (2024) suggested that caution is needed even with the best-performing models (identified based on their performance for the present climate) because among them one finds so-called “hot models” (e.g., Hausfather et al., 2022). Indeed, some CMIP6 models are found to be unrealistic high-sensitivity models with worse climate outcomes than the previous model generation (CMIP5). They exhibit an overestimated climate sensitivity, inconsistent with past assessments, resulting in future warming that may be larger than supported by the most reasonable projections (Tokarska et al., 2020).
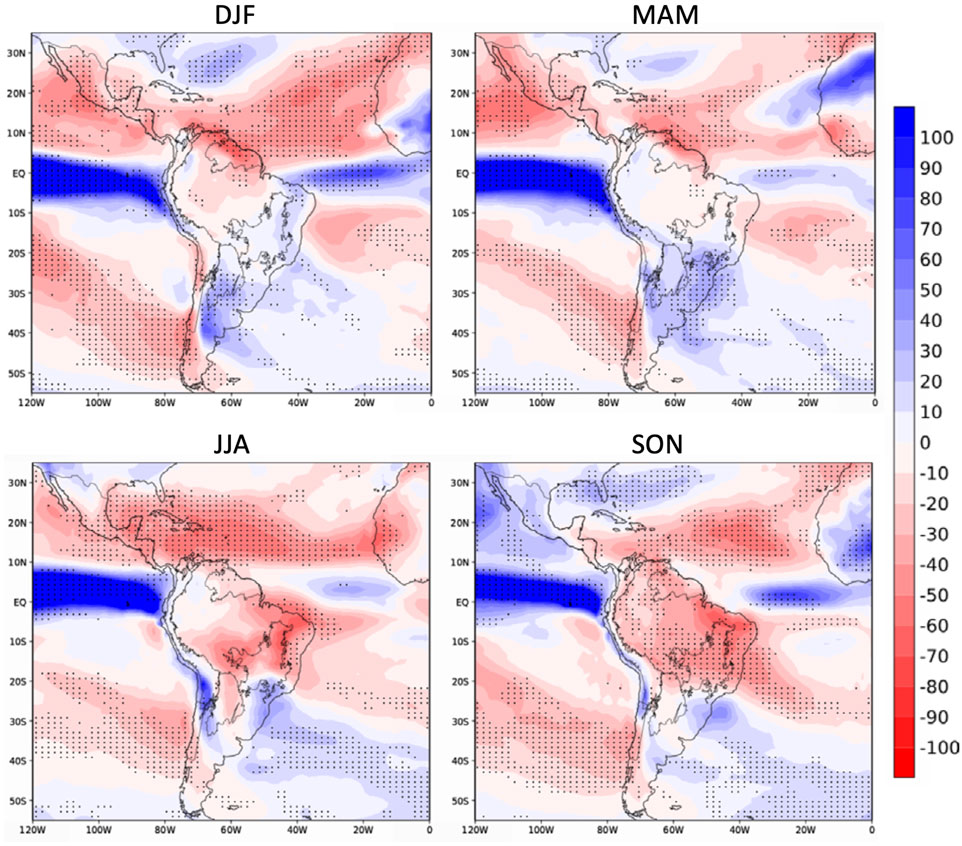
Figure 2. Seasonal projected precipitation changes (%) identified in Ortega et al. (2021) from a sub-ensemble of selected seven-best CMIP6 models between 2071–2100 and 1971–2000 following the SSP5-8.5 scenario. Dots indicate an agreement of 80% in the sign (positive in blue, negative in red) of the projected change between the models. Source: Ortega et al. (2021). © International Journal of Climatology. Reprinted by permission from Wiley.
Future projections show an increase in hydroclimate extreme events associated with a more intense hydrological cycle in South America (Almazroui et al., 2021). In the Andes, an increase of extreme drought events in central Chile, as the recent mega-drought (2010–2015), is projected to increase from one to five events every 100 years by the end of the 21st century (Bozkurt et al., 2018). In addition, Bambach et al. (2022), using a global variable-grid atmospheric model, revealed a projected decrease in the timing and magnitude of water stored as snow in the Andes of Chile, along with a decrease in the number of winter wet days with temperatures below 1°C, as well as fewer days with minimum temperatures below 0°C. In the tropical Andes, the projected increasing minimum temperature and decreasing stored snow could generate glacier mass loss (e.g., Al-Yaari et al., 2023) and a nearly elimination of the Andean Tundra or Alpine climates at high altitude (Bambach et al., 2022).
Using higher resolutions than most GCMs, regional models from Coordinated Regional Downscaling Experiment (CORDEX; Giorgi et al., 2009; grid spacing ∼50 km), also show a general projected increase in temperature over the Andes under the RCPs scenarios (Figure 1). In terms of precipitation, the sign of the change can be mixed, with a heterogeneous distribution over the Andes, sometimes in coherence with the GCM projections (e.g., in central Chile) and oftentimes showing the opposite sign of the parent GCM simulation (e.g., Pabón-Caicedo et al., 2020; Arias et al., 2021b; Hodnebrog et al., 2021; Figure 3). For example, based on a subset of CORDEX simulations, Arias et al. (2021b) found that while their parent CMIP5 simulations suggest wetter future conditions under the RCP8.5 scenario (except for the September-October-November season), RCM models from the CORDEX ensemble suggest drier conditions over parts of the Colombian and Ecuadorian Andes. An opposite signal of future climate change can also be found using kilometer-scale numerical downscaling. In eastern central Peru, a wetter future climate is simulated at 50 km for the end of the 21st century using the Weather Research and Forecasting (WRF; Skamarock et al., 2008) model (Hodnebrog et al., 2021; Figure 3). However, when using 10-km resolution with the same model, the Cumulus scheme interacting with a better resolved topography of the Andes results in a reduction of the ascent and moisture convergence over the eastern slopes of the Andes of Peru, consequently leading to a reduction in precipitation in future projections. Therefore, some regions of the Andes exhibit opposite precipitation projections, depending on the model and on the resolution used, consequently (i) highlighting the importance to be particularly cautious in the choice of the model and its configuration in modeling studies, and (ii) revealing a strong need to increase our understanding of local-regional interaction processes to help identifying the most reasonable projection signals (as discussed in section 4.1.1).
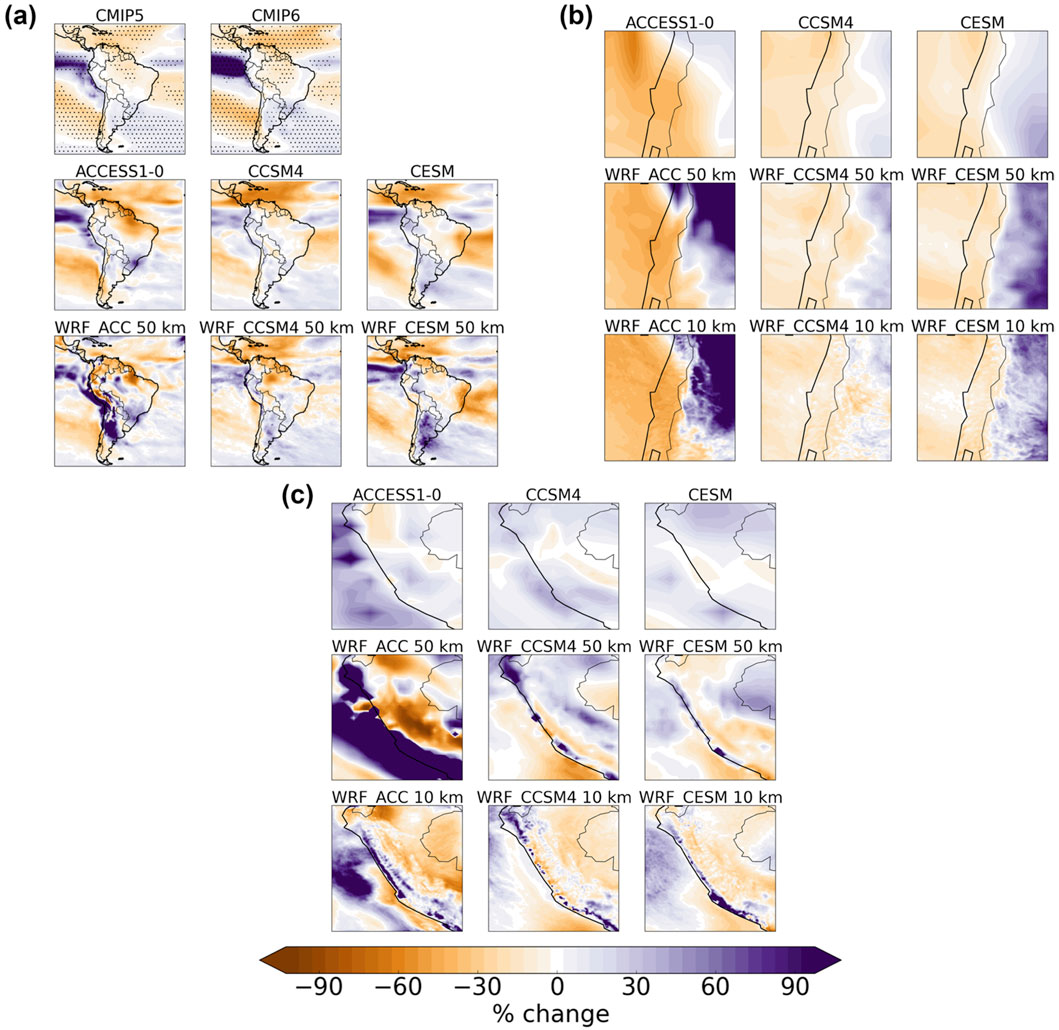
Figure 3. Projected changes in annual precipitation (%) in 1996–2005 vs 2091–2100 (RCP8.5) for South America (A), central Chile (B) and Peru (C) from three GCMs and three dynamical downscaling with the WRF model at 50 km and 10 km. Stippling in the CMIP5 and CMIP6 plots in (A) indicates where more than 80% of the models agree on the sign of change. Source: Hodnebrog et al. (2021). © Springer Nature. CC license.
The CORDEX simulations were also used to study the mechanisms associated with precipitation change. For example, Torres-Alavez et al. (2021) found that the South American Low-Level Jet (SALLJ; e.g., Berbery and Barros, 2002; Vera et al., 2006; see Part I) could become stronger and exhibit a westward expansion under the RCP8.5 scenario, due to stronger warming over continental areas and an intensification of the geopotential gradient. Soares and Marengo (2008) also identified an intensification of the SALLJ under a high emission scenario associated with an increase of the meridional temperature gradient between tropical and subtropical South America east of the Andes. Although a characterization of the uncertainty range in the simulated SALLJ responses would be needed, these changes may be related to projected changes in precipitation over the eastern slopes of the central Andes. However, results from Blázquez and Solman (2023), based on the CORDEX-CORE RCMs, highlighted that seasonal mean precipitation is overestimated throughout the Andes range probably due to models failing in representing the interaction between the flow and the topography. Additional kilometer-scale downscaling experiments are needed to better understand the atmospheric processes associated with climate change signals at local scale in the Andes, particularly in the tropics where most of the uncertainties among models are found.
2.2 Elevation-dependent warming projected signals
In mountainous terrain, changes in temperature under future conditions might vary with altitude, exhibiting an enhancement in warming at higher elevations (known as Elevation-Dependent Warming, EDW), which has been observed and projected in some of the major mountain chains in the world (e.g., Palazzi et al., 2019; Pepin et al., 2022). In the case of the Andes, the lack of a dense network with long-term records complicates robust trend estimations in the recent past. In addition, the high resolution needed for better representing the topography for assessing EDW also poses a challenge for estimating trends under current conditions and climate change scenarios (e.g., Pabón-Caicedo et al., 2020).
We highlight two studies devoted to EDW over the Andes based on atmospheric dynamic models. Based on CORDEX simulations with the Rossby Centre Atmospheric (RCA) regional model, Toledo et al. (2021) studied EDW in the Andes between Ecuador and Chile. The RCA simulations, with a resolution of 0.44° (∼50 km), involved an ensemble of eight members (downscaled from different GCMs) representing present-day (1976–2005) conditions, and the RCP8.5 scenario by the end of the century (2071–2100). Toledo et al. (2021) found a consistent increase in warming with altitude in the subtropical Andes, while in the tropical Andes, minimum temperature exhibits a decrease in EDW. The drivers of EDW were found to vary between the subtropical and tropical Andes, with a significant influence of the snow-albedo feedback in the subtropics, where seasonal snow cover exists, and more significant changes in downward long- and short-wave radiation in the tropics.
Based on higher resolution (10 km grid spacing) 30-year simulations, Chimborazo et al. (2022) focused on EDW in the Ecuadorian Andes. The authors found that the simulations agree with the observations in showing positive trends in EDW for the recent past. In addition, for the mid-21st century (2041–2060), they project positive trends in EDW, especially on the western slopes of the Ecuadorian Andes. The study identified enhanced upper-tropospheric warming at high elevations on both sides of the cordillera, changes in mid-tropospheric zonal circulation inducing subsidence and drying, reduced cloudiness over the western slopes, and the snow-albedo feedback at higher elevations as key drivers of EDW.
These studies provide clues about the features of EDW in the Andes but also emphasize on the need of higher resolution simulations to better represent the effects of the complex terrain and to test different land-surface models given their instrumental role in the simulation of the snow-albedo feedback.
2.3 Deforestation effects on the Andean climate
The impact of Amazon deforestation over the Andes climate was analyzed through modeling studies (e.g., Sun and Barros, 2015a; 2015b; Eghdami and Barros, 2019; Sierra et al., 2021). Sierra et al. (2021) focused on the austral summer precipitation (December-January-February; DJF) of the Amazon-Andes transition region, particularly influenced by a large portion of recycled moisture coming from the Amazonian rainforest transpiration (e.g., Staal et al., 2018). High-resolution experiments in the Bolivian Amazon-Andes transition region show a decrease in precipitation associated with regional Amazon deforestation particularly over the lower parts of the Amazonian valleys and during the night (Sierra et al., 2021; Figure 4). This is due to a strong reduction of the moisture transport linked to a weakened regional SALLJ. The local moisture circulation also showed a weakening of the daytime upslope wind. Similar results were found in quasi-idealized experiments in the eastern flank of the tropical Andes, where surface evapotranspiration removal weakened upslope flows by increasing the static stability of the lower troposphere (Sun and Barros, 2015b). In addition, the local deforestation in the Andean piedmont is also responsible for a local feedback between atmospheric conditions and the rainforest through a soil moisture reduction, leading to decreased rainfall (Eghdami and Barros, 2019). In addition, Sun and Barros (2015a) found a precipitation dipole-like anomaly on the eastern flank of the Andes associated with Amazon deforestation experiments, with decreased precipitation over the Amazon lowlands and increased precipitation in surrounding mountains due to a convergence-divergence anomaly. Therefore, it is expected that both climate change and Amazon deforestation could significantly affect future projections of precipitation in the Amazon-Andes transition region but more experiments would be needed in order to (i) identify robust signals of precipitation change associated with the Amazon deforestation in the Andes highlands and (ii) properly distinguish the different effects of climate change and deforestation over the future Andean climate.
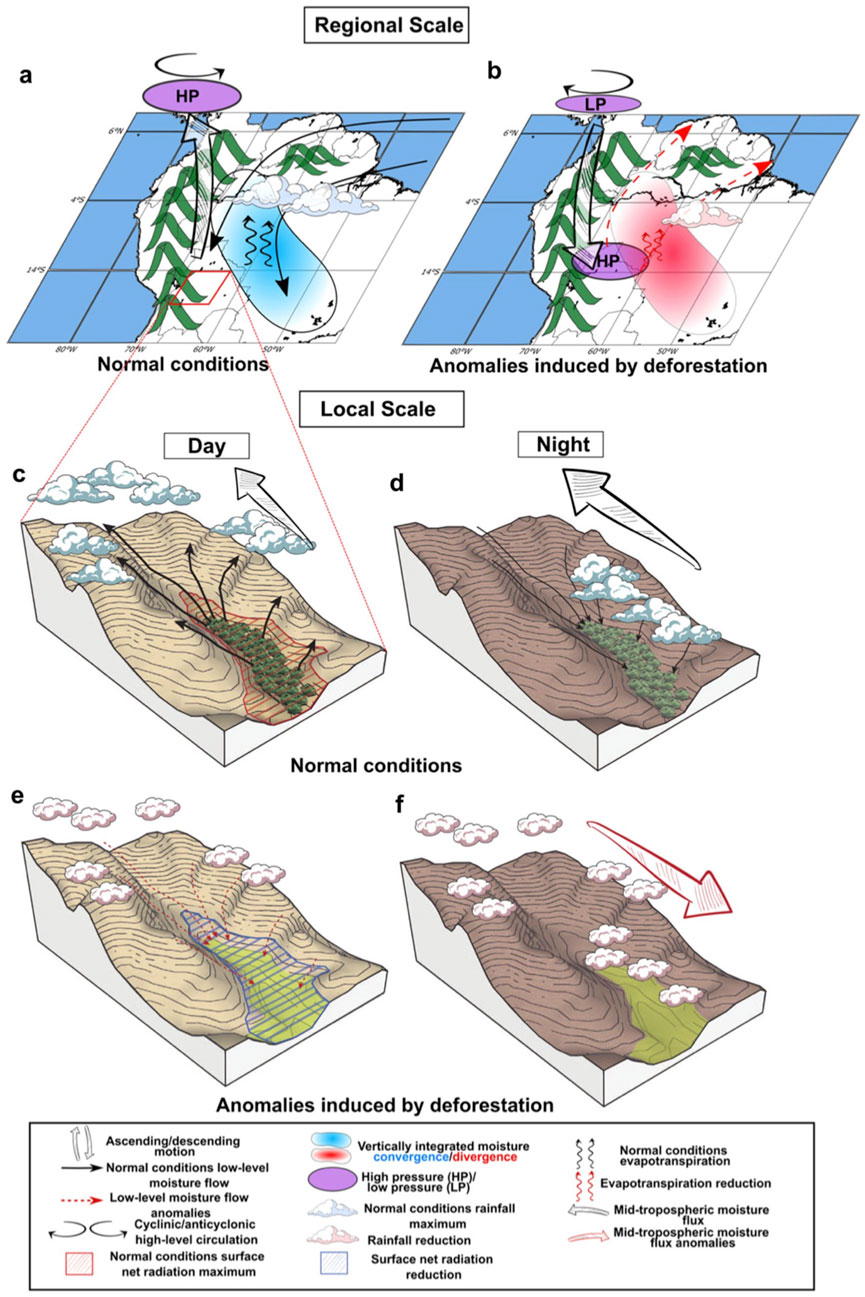
Figure 4. Schematic representation of DJF climatological atmospheric conditions at regional (A, B) and local scales (C–F) for normal conditions (A,C,D) and Amazon deforestation induced anomalies (B,E,F) in WRF simulations. The red square in (A) indicates the approximated location of the Zongo Valley sketched in (C–F). Black solid (red dashed) thin arrows represent surface moisture transport under normal conditions (deforestation anomalies). Black (red) thick arrows represent mid-tropospheric moisture flux under normal conditions (deforestation anomalies). Vertical thick arrows represent ascending and descending motions. Black (red) curved arrows represent evapotranspiration under normal conditions (deforestation anomalies). Blue and red shaded areas indicate vertically integrated moisture convergence and divergence, respectively. Purple areas indicate pressure changes. Blue and red clouds indicate rainfall maximum and rainfall reductions under normal and deforested conditions, respectively. Red (blue) hatched area in (C, E) indicates surface net radiation maximum (reduction) under normal (deforestation) conditions. Source: Sierra et al. (2021). © Climate Dynamics. Reprinted by permission from Springer Nature.
Regional changes in circulation patterns have been identified recently in association with projected deforestation patch scenarios like a large-scale subsidence suppressing the low-level convection during the dry-to-wet transition season (e.g., Sierra et al., 2023). However, it is not known how the Andean seasonal variability of precipitation could be affected by this regional change at local scale. More high-resolution experiments are needed in order to identify the effect of the Amazon deforestation over the annual precipitation regimes in the Andes and to increase the robustness of this signal.
Non-Amazonian Andes have also been subjected to a consistent historic process of land use and land cover changes. In particular, Chilean matorral are among the most affected non-Amazonian ecosystems in South America where the conversion of wooded grassland to croplands has resulted in a warmer climate, as suggested by early modeling studies (Salazar et al., 2015 and references therein). Using a three-members ensemble with a climate model at a 25-km resolution over a recent 30-year period, Salazar et al. (2016) also suggested that the loss of natural vegetation in this semi-arid region has increased the drying and potentially desertification process due to changes in the hydrological cycle including surface temperature, evaporation, soil moisture and atmospheric humidity.
3 How model configurations impact atmospheric modeling results
The design of atmospheric simulations requires important decisions about the configuration of the model and the numerical experiment, including a definition of the type of grid and grid spacing, various physical parameterizations for sub-grid processes, a version of the static forcing data, among others (e.g., initial and boundary conditions). For a given model (e.g., with a given dynamical core), different configurations may produce substantially different patterns of winds, clouds, temperature, precipitation, and other variables. In this section we briefly comment on issues related to grid spacing (or equivalently, resolution), parameterizations and static forcing data (e.g., land cover) based on studies about the Andes.
3.1 Role of grid spacing
The grid spacing is directly related to the resolution of the simulation. The smallest flow structure resolved in a numerical simulation has an approximate scale of 5–10 times the grid spacing (e.g., Pielke, 2013). Thus, if properly configured, a smaller grid spacing facilitates a numerical solution that resolves smaller details in the flow and other atmospheric conditions, especially when the topography has important details at smaller scales. As already described with multiple examples in Part I of this review, using a small grid spacing is particularly relevant for atmospheric simulations in the Andes region. At the time of writing, high resolution refers to horizontal grid spacings at the kilometer scale, with simulations mostly in convection-permitting (CP) mode (i.e., without the use of a deep cumulus convection scheme) when lower than 5 km. Simulations with grid spacings approximately in the range 9–18 km can represent some of the major Andean features and their effects on atmospheric flow (e.g., low-level jets and wind channelization by major inter-Andean valleys) and distribution of precipitation (e.g., Insel et al., 2010; Junquas et al., 2018; Moya-Álvarez et al., 2018a; Posada-Marín et al., 2019; Bozkurt et al., 2019; Flores-Rojas et al., 2019; Jiménez-Sánchez et al., 2019; 2020; Schumacher et al., 2020; Sierra et al., 2021; Fernández et al., 2021; Martinez et al., 2022). However, grid spacings below 6 km have been found necessary for resolving phenomena like organized deep convection, orographically forced or enhanced convection, rain shadows, thermally forced circulations like slope flows (including katabatic winds) and mountain-valley winds, other mountain circulations like gravity waves and foehn-like winds (see for example, Trachte et al., 2010; Lenaerts et al., 2014; Junquas et al., 2018; 2022; Schumacher et al., 2020; Sauter, 2020; Yepes et al., 2020; Rosales et al., 2022; Gomez-Rios et al., 2023; see also Part I of this review, and references therein).
Although there are clear arguments in favor of resolutions of the order of a few kilometers to reduce persistent and systematic model biases over the Andes, there still exist some uncertainties in high resolution RCM experiments such as precipitation overestimation in the Andes, which is one of the most common uncertainty sources. As seen in other parts of the world, an increased spatial resolution of RCMs does not always improve all statistics in simulated precipitation (Mass et al., 2002; Schwartz et al., 2009; Rauscher et al., 2010). The added value of the resolution increase in the Andes depends on various factors, including the driving fields, the surface characteristics of the region, the season, the variable considered and the temporal variability mode considered (e.g., Falco et al., 2020; Gutierrez et al., 2024). For example, a 20-km RCM simulation is able to better resolve the climatological mean of orographic precipitation “hotspots” over the southern tropical Andes-Amazon transition zone than 50-km RCM CORDEX simulations (Gutierrez et al., 2024), and 9-km simulations better reproduce its localization along the eastern Andean slope than 27-km simulations (Junquas et al., 2018). Otherwise, over central Chile an improvement is found after two consecutive downscaling at 50 km and 10 km from a forcing GCM (MPI-ESM-MR; Figure 4). While the GCM under-estimates precipitation, the 50-km RCM simulation induces a positive bias that is then reduced with the 10-km downscaled simulation (Bozkurt et al., 2019; Figure 5). However, in the southern tropical Andes, a 2-km simulation shows improvements in the diurnal cycle of precipitation when compared to a 6-km simulation, but with a persistent precipitation overestimation (Rosales et al., 2022). Furthermore, the number of RCM studies with CP horizontal resolutions (e.g., ∼ 1–4 km) is still limited, and even at such high resolutions might still be too coarse to deactivate cumulus schemes for a correct representation of deep convection over the eastern Ecuadorian Andes (Junquas et al., 2022), where a 1-km resolution is needed to reproduce the slope and valley processes associated with the nighttime orographic convection, while not reproduced at 4 km (Trachte et al., 2010).
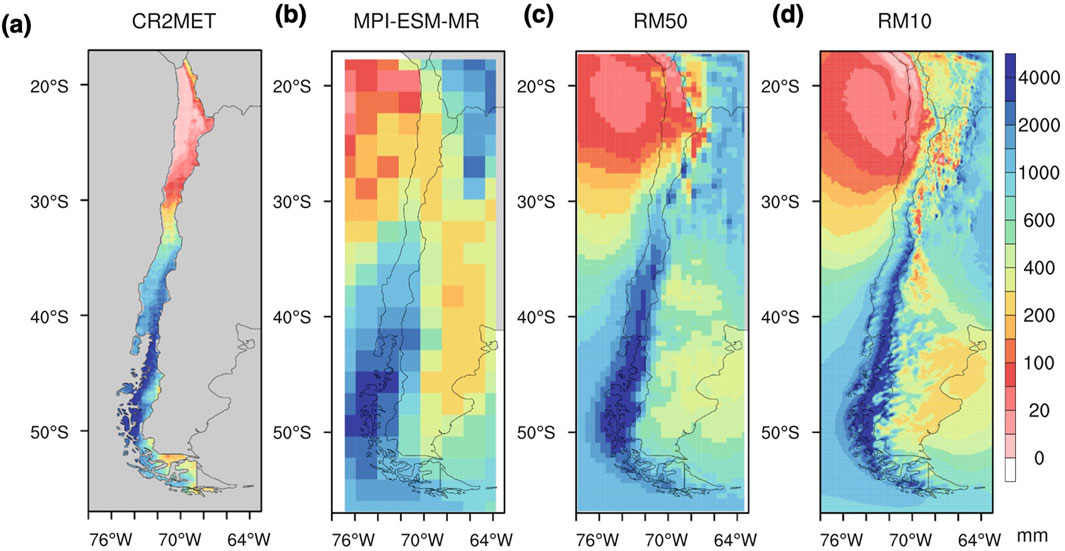
Figure 5. Climatological mean 1980–2005 of precipitation (mm) in the Andes of Chile from (A) gridded observation (CR2MET), (B) MPI-ESM-MR (∼220 km), (C) dynamically downscaled simulations of MPI-ESM-MR at 50-km (RM50) and (D) 10-km (RM10) resolutions. Source: Bozkurt et al. (2019). © Climate Dynamics. Reprinted by permission from Springer Nature.
3.2 Influence of the physical parameterization choices over modeled atmospheric processes
Even using a very fine grid (e.g., kilometer scale), some physical processes will not be explicitly resolved, because they take place in spatial scales ranging between a few meters down to the molecular scale. These sub-grid processes have to be represented by sub-models or parameterizations, and they are associated with radiative transfer, cloud microphysics, planetary boundary layer, exchanges (fluxes) between the first levels of the atmosphere and the bottom boundary (i.e., land surface or the ocean), among others. For larger grid spacings, a parameterization for deep cumulus convection is also needed. For each type of parameterization (e.g., cloud microphysics), different sub-model versions (schemes) exist, with a broad spectrum of complexity. The impact of individual schemes on the emergent patterns of simulated precipitation, temperature, winds, etc. is very difficult to assess, given the strong coupling between parameterizations (see e.g., Dudhia, 1989). In this section we very briefly comment on particular results or trends associated with different parameterizations in modeling studies over the Andes.
When the grid spacing is relatively large (e.g., >10 km), a deep cumulus convection scheme is recommended to be used in order to represent sub-grid-scale effects of deep and/or shallow clouds. Some schemes are relatively simpler than others. In the case of the tropical Andes, the Kain-Fritsch scheme has been widely used, which is a low-layer controlled scheme, with detailed representation of the mass flux between different levels in a given atmospheric column. This approach has often produced a better simulation of precipitation, compared to deep-layer convective adjustment schemes (e.g., Betts-Miller-Janjic; Janjic, 1994), or ensemble schemes (e.g., Grell; Grell and Freitas, 2013; Posada-Marín et al., 2019; Ochoa et al., 2016; Mamani and Hendrick, 2021). However, the latter might produce a good representation of precipitation over parts of the Peruvian Andes (Hodnebrog et al., 2021; González-Rojí et al., 2022). At kilometer-scale grid spacings, it is usual to configure the simulations without a deep cumulus scheme (i.e., CP configuration), although the range 2–10 km is still a “gray-zone” for deep convection (e.g., Honnert et al., 2020). In particular, for grid spacings below 5 km, atmospheric models would be able to explicitly resolve the major convective elements and processes associated with deep convection (e.g., Prein et al., 2015; Kendon et al., 2021). Some studies have tested how this CP configuration might affect precipitation performance in the Andes, with conclusions differing by region. While some studies find improvement with a CP configuration (e.g., Posada-Marín et al., 2019), others find no particular benefit (e.g., Moya-Álvarez et al., 2018a), while others find better results using a cumulus convection scheme even with grid spacings as small as 1–3 km (e.g., Junquas et al., 2022).
For simulations over the Andes, most of the recent studies use full double-moment or partial-double moment (i.e., double moment only for two water species) microphysics schemes. While double-moment schemes (simulating both the mixing ratio and particle number density) can lead to improvement in the simulation of precipitation (e.g., Junquas et al., 2022; Moya-Álvarez et al., 2018a; Martínez-Castro et al., 2019) and surface solar radiation (e.g., Urrego-Ortiz et al., 2019), they can lead to overestimation of precipitation over parts of the extratropical Andes (e.g., Comin et al., 2018); in these latter regions, single-moment schemes have been found to produce better patterns of precipitation (e.g., Comin et al., 2018). In the case of the Planetary Boundary Layer (PBL) parameterizations, non-local schemes like YSU (Yonsei University; Hong et al., 2006) are the most widely used in simulations for the Andes, because of their good performance in the simulation of precipitation and temperature (Supplementary Table S1; Ochoa et al., 2016; Moya-Álvarez et al., 2018a; González-Rojí et al., 2022). However, 1.5 order schemes (the most recent including a mass-flux component) can oftentimes lead to a better simulation of different variables, including precipitation (e.g., Moya-Álvarez et al., 2020; Henao et al., 2020). In addition, in the Peruvian Andes, Gonzalez-Roji et al. (2022) found that the choice of parameterization, and particularly the microphysics could affect differently the performance of a simulation depending on the season considered, to correctly reproduce the orographic precipitation gradient in the “hotspot” region of the Amazon-Andes transition slope (Figure 6).
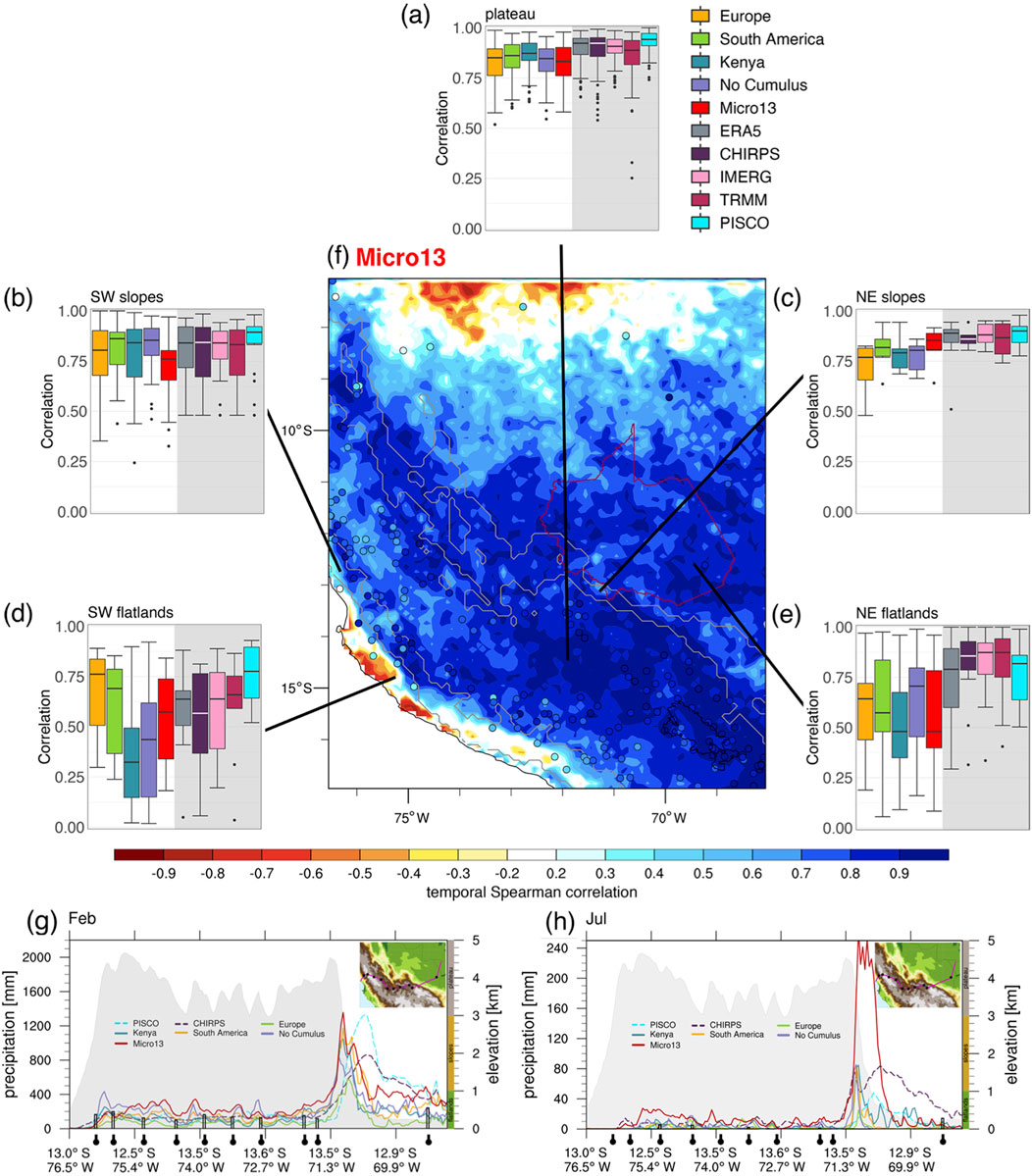
Figure 6. Temporal Spearman correlation of five different parameterizations of Cumulus, microphysics and PBL of a 5-km WRF simulation of the year 2008 against five data products in whisker plots (A–E), and against weather station data and the PISCO dataset in the central map (F). Andes-Amazon cross-section for the monthly accumulated precipitation for the months of February (G) and July (H) of 2008 are represented for each dataset and simulations following the color legend indicated in each panel. Source: Gonzalez-Roji et al. (2022). © Copernicus Publications. CC license.
PBL schemes may lead to substantial differences in the vertical profiles of winds in the vicinity of the Andes, especially in the boundary layer, impacting the distribution of the simulated precipitation (e.g., Martinez et al., 2022). In addition, as grid spacing is reduced, better results for the complex terrain of the Andes might be obtained with a scale-aware PBL scheme (e.g., Henao et al., 2020). Note that these PBL schemes are one-dimensional, i.e., column models. At higher resolution (e.g., a few hundred meters), these approaches would have to be reconsidered.
The most recent modeling studies over the Andes also tend to use the more sophisticated radiation schemes, i.e., those including more spectral bands and interactions with a larger number of types of gases, hydrometeors and aerosols. An example of such a comprehensive scheme is the Rapid Radiative Transfer Model for GCMs (RRTMG). The skill of radiation schemes has been often assessed by how realistic the resulting precipitation patterns are (e.g., Ochoa et al., 2016; Moya-Álvarez et al., 2018a; Gonzalez-Rojí et al., 2022). An example of the skill of the RRTMG in the simulation of surface solar radiation can be found in the studies by Urrego-Ortiz et al. (2019) and Cano et al. (2022), who reported a general overestimation over an intra-Andean valley in the tropics. On the other hand, land surface schemes are directly related to the simulation of surface fluxes and near surface temperature. One example of the direct effects of the land-surface scheme is the overestimation of surface temperature over parts of the higher Andes, depending on the modeling of the partitioning of precipitation into rainfall and snowfall (Mourre et al., 2016; Junquas et al., 2022). Another example includes regional scale changes in the low-level winds because of differences in the simulated gradients of surface fluxes in the vicinity of the Andes (e.g., Martinez et al., 2022).
3.3 Surface-atmosphere interaction processes influenced by surface forcing data
3.3.1 Topography and land use
Regional climate models use two types of forcing data: the boundary forcing data, generally from reanalysis data or GCM, and the terrestrial data (e.g., orography, land cover, coastlines, etc.). Different experiments have been performed to test different forcing data in the Andes and their impacts on model performance. For instance, Comin et al. (2018) demonstrated that using ERA-Interim as boundary forcing improves the performance of the WRF model in terms of snowfall events for the central Andes of Chile-Argentina.
In the Andes, the surface-atmosphere interactions in high-resolution modeling are strongly controlled by terrestrial forcing data, in particular topography and land use. Several studies have tested different digital elevation models (DEM) as topography forcing with the WRF model. The Shuttle Radar Topography Mission (SRTM; Farr et al., 2007) DEM was identified as a better topography product than the default DEM (USGS; United States Geological Survey) in the WRF versions prior to 3.8 in terms of precipitation and surface temperature (Figure 7) because of a more realistic mountain and slope forcing over the local atmospheric circulation (e.g., Saavedra et al., 2020). In addition, Junquas et al. (2022) found that using a non-smoothed SRTM DEM (instead of a default smoothed orography) with WRF version 3.7.1 improves the performance of the model, in terms of precipitation in a glacier region of Ecuador. A more realistic representation of the maximum and minimum of the topography (summits and valleys) lead to a better representation of valley-wind and slope circulations controlling the local precipitation. The SRTM DEM was also used as topography forcing by the RACMO2 model in a precipitation study in Patagonia (Lenaerts et al., 2014).
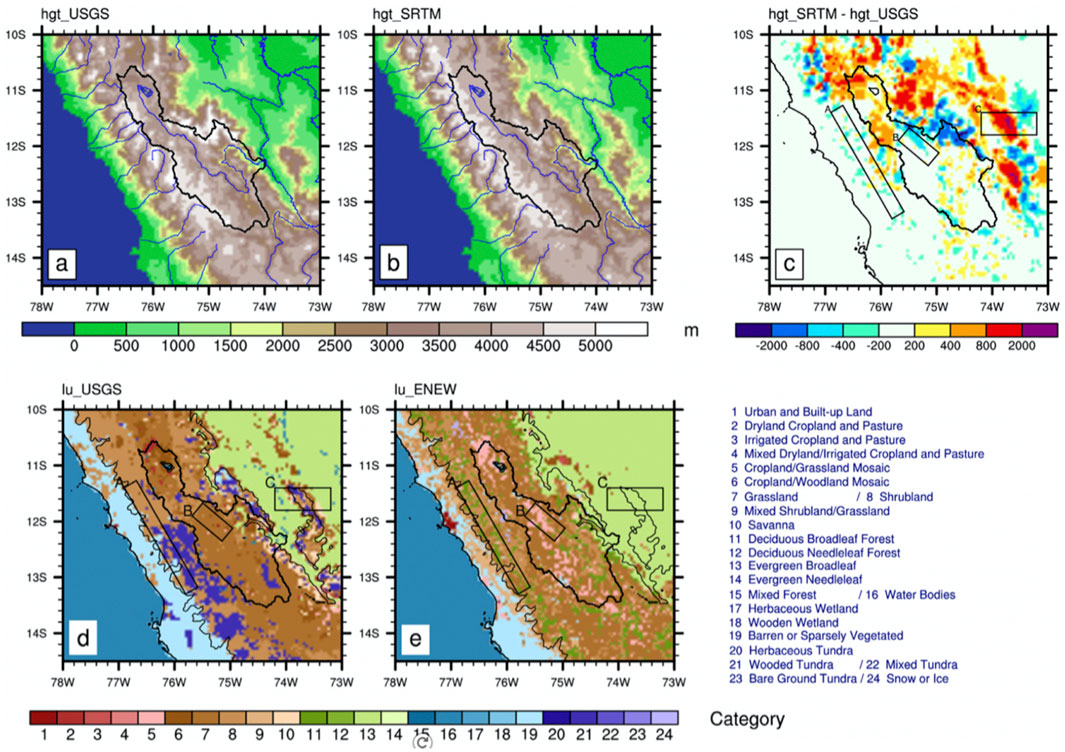
Figure 7. Topography of 3-km spatial resolution from (A) USGS and (B) SRTM in the Mantaro valley region, in Peru; (C) shows the difference SRTM- USGS. In (A) and (B) the Mantaro river system is represented with blue lines. (D) and (E) show the land use of 3-km spatial resolution from (D) USGS and (E) Eva et al. (2004). Categories of land use are indicated according to USGS definitions. The delimitation of the Mantaro basin and 2000 m. a.s.l. are also indicated with thin and thick lines, respectively. Source: Saavedra et al. (2020). © Atmospheric Research. Reprinted by the permission of Elsevier.
In Peru, Saavedra et al. (2020) tested a new land-use product from Eva et al. (2004) and found performance improvements in terms of precipitation and surface temperature when compared to the default land use data of the WRF model version 3.7.1 (USGS; Figure 7). The changes in land use produced locally shifts in the moisture flux and its associated convergence, as well as a change of local evaporation. Such changes in surface-atmosphere interactions in relation to the land use forcing was also identified at a regional scale in experiments including deforested Amazon (see Section 2.3).
3.3.2 Sea surface temperature
The weather and climate of the Andes are strongly influenced by Sea Surface Temperature (SST) conditions in the Pacific Ocean. In particular, the impact of ENSO in the Andes from Colombia to Chile has been extensively studied, due to its relevance in relation to infrastructure planning to cope with its societal impacts (e.g., Cai et al., 2020; Poveda et al., 2020). For instance, El Niño 1997–1998 caused ca. 23,000 deaths and USD 33 billion losses, in addition to its scientific importance with marked contrasting impacts across regions and temporal scales.
At the western slopes of the Andes in Peru, Trachte et al. (2018) analyzed cross-scale precipitation variability related to atmospheric circulation, local-scale mountain breezes and SST patterns using the WRF model. Although a link to ENSO could not be established, the authors demonstrated the impact of varying SST values in the Nino1+2 region on the precipitation gradient as a result of alterations in the southeastern low-level winds and upslope flows. To capture the intricate influence of ENSO in the Andes, high resolution modeling and more complex regional models, such as regional Earth System Models in which the ocean component is coupled with the atmospheric model are necessary, although broad features of its impacts can be studied using GCMs. For instance, GCMs capture present ENSO rainfall anomalies, and projected changes of ENSO mean state point to warming of eastern tropical Pacific SST, drying of Central America and northern Colombia, and wetting of southwest Colombia and Ecuador (Steinhoff et al., 2015).
In a modeling study focusing in the northern Andean regions, Martinez et al. (2019) showed that a correct representation of the SST variability is essential as it can affect the regional circulation, convergence zones and moisture availability, particularly at inter-annual scale. In addition, Martinez et al. (2019) found that a lack of coupling between SSTs and low-level winds could be associated with very low correlations between precipitation in the Colombian Andes and SSTs in the Pacific, contrary to observations. In the Andes of Peru and Chile, the SST variability is also strongly affected by the Humboldt current system (HCS), also called the Peru-Chile current system, among the foremost maritime ecosystems in the world. The HCS is a relevant oceanic forcing that affects the climate and weather of the Andes. The upwelling is localized, with continuous influence in Peru and northern Chile, shifting to a more seasonal influence in central and southern Chile. The Andes uplift affects the dynamics of the lower troposphere over the Pacific Ocean, which in turn influences the physical features of the HCS (Sepulchre et al., 2009). Modeling with lower Andes height shows that the latitudinal patterns of temperature changes up to 3°C. Also the Andes uplift forces a southward shift of the HCS affecting the position and strength of the HCS (Sepulchre et al., 2009). Thus, the feedback mechanisms between the atmosphere and the ocean may be better captured with improved SST prescriptions in high-resolution regional climate models, or with fully coupled atmosphere-ocean regional climate models.
4 Challenges and perspectives
Numerical modeling for the weather and climate of the Andes faces different challenges, including the need for more model experiments for improving our understanding of different processes and the uncertainties for different variables, the scarcity of observations for model validation and data assimilation studies and a substantial lack of funding for local modeling efforts. Additionally, the development of methodologies for the use of modeling results for impact studies and policy making is discussed in this section.
4.1 Recent modeling efforts
We can distinguish two main categories of numerical experiments that cover the Andean region. Local efforts (i.e., by South American universities and/or small scientific research teams) tend to cover small spatial areas at various resolutions due to computational constraints. Meanwhile, international coordinated efforts like CORDEX, or major projects by institutions like the National Center for Atmospheric Research (NCAR) and the Met Office, are capable of covering the entire Andean range thanks to the ample resources available (see produced data-sets in Supplementary Table S2). We can also identify two main types of numerical experiments: (1) climate-oriented studies from which long period data-sets are produced, and (2) analysis of specific processes via short term simulations.
4.1.1 Long-term climate change simulations and applications
The outputs of long-term dynamical atmospheric modeling under a scenario of climate change can be used for various purposes. These simulation outputs can be used for the proper analysis of climate statistics and atmospheric processes, but the atmospheric variables can also be used as the atmospheric forcing for the modeling of other earth components (hydrology, glaciology, ecological systems, etc.), including other dynamical atmospheric models at higher resolution or for statistical downscaling. However, various types of atmospheric modeling exist and the choice of the model outputs to be used has to be determined in function of the objective of each study.
The most important group of regional models providing long-term climate change simulations for the Andean scientific community is CORDEX for the SAM region with resolution from 0.20° to 0.44° (Supplementary Table S2). Each of them is a product of a dynamical downscaling technique using CMIP5 GCM outputs as forcing variables for a RCM at higher resolution than the forcing model, under a RCP scenario. A large number of CORDEX-SAM models exist (35), allowing to reduce uncertainties and identify statistically significant climate change signals (e.g., Falco et al., 2020; Solman and Blázquez, 2019; Blázquez and Solman, 2020). Note that even if both GCMs and RCMs provide long-term runs, only GCMs or global stretching-grid models include large-scale climate change feedback that could affect some regional circulation patterns in South America and could in turn affect the Andes future climate (ex. Walker and Hadley cells, Rossby wave-trains, etc.; e.g., Junquas et al., 2016). However, these global climate change feedbacks have been scarcely studied in South America, although the representation of correct altitudes of the Andean highlands, which is rarely the case in GCMs (e.g., see Figure 1 of Part I), could particularly impact these global teleconnections.
In addition, as already mentioned, in some regions the projected signal of precipitation is the opposite in GCM and RCM outputs (e.g., Hodnebrog et al., 2021; Arias et al., 2021b; see Section 2.1). Therefore, using one single model output for analyzing future climate impacts should not be considered as a robust source for inferring the future climate change signal. It is clear that the computational resources do not always allow to use a large number of model outputs, and some applications require to initially perform a selection of the best models adapted to the region and variable of interest. In this case, in order to reduce uncertainties, a coherent ensemble of RCMs or GCMs should be used, particularly models showing a correct present climate representation, as mentioned in the previous section, and reasonable future tendencies in the studied region and for the variable of interest.
The local projections of precipitation can vary depending not only on the choice of the model, but also on the resolution of the model, even when the downscaled simulation employs the same model configurations as the forcing output model (see Section 2.1; Hodnebrog et al., 2021). This result reveals the importance in attributing the relative influence of regional processes (e.g., SALLJ, SAMS, etc.) and local processes (e.g., thermally-driven winds, slopes, etc.) on a given local projection signal. While projections of regional processes in South America can be generally analyzed from GCM outputs, the high-resolution of an RCM is needed to explore and increase our understanding of the influence of climate change over local processes in the Andes. However, the interactions and potential feedbacks between the local and regional/global scales are little studied in the region (e.g., Sierra et al., 2021; Sierra et al., 2023; Ruiz-Vásquez et al., 2020). To conclude, an important part of the challenge of better identifying the most reasonable local projected changes in the Andes could be to better understand local-regional-global scale interactions processes and how much each of these processes could explain the local projected signals of precipitation.
Assessment studies using RCMs and GCMs in South America including Andean regions exist mainly for surface temperature and precipitation that could help in the choice of the models (e.g., Blázquez and Solman, 2020; Díaz et al., 2020; Almazroui et al., 2021; Ortega et al., 2021; Thaler et al., 2021; Olmo et al., 2022). The Working Group I (WGI) of the Intergovernmental Panel on Climate Change (IPCC) Interactive Atlas is also a valuable tool to assess GCMs and CORDEX simulations (https://interactive-atlas.ipcc.ch/). However, the sub-regions defined in the sixth IPCC assessment report (AR6) offer limited applications in the Andes, given the large variety of processes and climate change signals included in each individual domain. For example, the dry Altiplano system is included in its majority in the same domain that the Southern Amazon domain (SAM), where a wet climate regime dominates. On the other hand, the Northwestern Andes domain (NWS) includes a large part of the tropical Andes, where different interannual climate variability and rainfall annual regimes co-exist (e.g., unimodal and bimodal seasonal variability; Segura et al., 2019), limiting the study of seasonal and interannual climate change signals when considering these domains. More integrative studies of all the Andes including modeling studies could be used for a more robust definition of Andean regions with similar variability and climate main characteristics, in order to provide information relevant for studies and other reports (e.g., IPCC and/or national assessments) useful for decision and policy making.
CP-resolution modeling studies are very few in the Andes (see part I) and generally performed for a particular region instead of the entire Andes. In 2021–2023, two international initiatives performed kilometer-scale climate experiments over South America, one from UK Met Office (down to 40° South; Halladay et al., 2022; Halladay et al., 2023) and another one (full continent) from the NCAR ‘South American Affinity Group’, (SAAG; Dominguez et al., 2024). These two experiments have been a milestone for the climate sciences in the region. Halladay et al. (2023) present results of three 10-year CP simulations (4.5 km grid spacing) for a domain that spans tropical and subtropical South America. One of the simulations is forced by reanalysis data (hindcast), while the other two simulations are forced by data from the UK Met Office GCM (present-day and future conditions). Although these CP simulations contain the Andes, Halladay et al. (2023) focus their analysis over other parts of South America. The NCAR project includes two 20-year CP simulations (4 km grid spacing) for a domain containing all of South America. One of the simulations is forced by reanalysis (ERA5) while the other simulation uses a Pseudo Global Warming setup (PGW; Schär et al., 1996; Rasmussen and Houze, 2016). Additionally, under the umbrella of CORDEX, the ongoing Flagship Pilot Study (FPS) for Southeastern South America, has provided a set of six coordinated convection-permitting RCM simulations covering the subtropical part of South America (from 15°S to 36°S), which may also be used for improving our understanding of the impact of the subtropical Andes on the regional climate.
This type of long-term high-resolution simulations could be very useful for providing robust statistics of processes associated with the hydroclimate of the Andes. Furthermore, model outputs from these modeling efforts can constitute high-resolution datasets (both in time and space) of hydrometeorological variables that can be of use for process-oriented studies, impact studies, etc. However, the results from these databases will have to be used with caution, for various reasons. For example, bias correction methods would probably have to be implemented before using model output as input for other (e.g., hydrological) models and/or as tools for decision making. In addition, uncertainty in the quantification of changes associated with global warming, as estimated from these projects, is difficult to assess because of the small number of simulations. This provides a great dependency of the climate change signals on the internal model variability and biases, as well as on the specific future scenarios used. Therefore, performing long-term simulations at such resolution with a larger diversity of models and scenarios at the Andes scale would be needed in order to reduce uncertainties linked to internal model biases or dependencies on particular scenarios.
4.1.2 Short-term and/or high-resolution experiments
Short-term simulations (e.g., for a few days) can be performed at very high resolution (e.g., grid spacing ≾ 1 km, Trachte et al., 2010; Henao et al., 2020), allowing the study of small-scale (∼ a few kilometers) and relatively fast (a few hours) processes within the Andes, e.g., localized convergence, extreme precipitation events, air pollution over Andean cities, etc. On the other hand, high resolution CP simulations with a somewhat larger grid spacing (e.g., ∼4 km) can be performed for ensembles with different configurations or different initial/boundary conditions, allowing the understanding of physical processes (e.g., experiments varying land over conditions), the estimation of model uncertainty (e.g., from multi-physics experiments), or estimating the range of atmospheric variability within the model (e.g., resembling natural variability). For example, high resolution simulations with different surface static data would facilitate the characterization of land-atmosphere interactions associated with very localized surface properties (e.g., small scale details in the topography, land cover, land use, etc.), along with the potential modifications of these interactions under regional or local changes (e.g., deforestation, urbanization, climate change). In particular, it is not known how the future change of the EDW over the Andes (see Section 2.2) could impact the local valley-wind processes and in turn the local diurnal cycle of precipitation.
Most of the studies reviewed in the previous sections and in part I are examples of what we mean by “short-term and/or high-resolution experiments”. These studies provide understanding of many processes within the Andes at the basic (fundamental) level. In this sense, the formulation of basic and application-driven questions for large scale efforts as for example, the continental scale CP projects by NCAR (Dominguez et al., 2024) and the MetOffice (Halladay et al., 2023), can greatly benefit from previous smaller-scale but more detailed (e.g., process-based) studies using kilometer-scale modeling. Conclusions from the smaller-scale studies may not directly apply to results from larger-scale efforts, because of potential feedbacks between larger and smaller scales. If this is the case, maybe the contrast between small- and large-scale efforts could still provide an idea of the relative role of local-scale processes and features.
4.2 Scientific challenges for future modeling activities
Important challenges that have not been tackled in the modeling community of the region yet have to do with increasing model complexity. Several of the processes affecting the main climatic features along the Andes are based on atmospheric-only models. For example, given the enormous impact that the SST variability, mainly in the Pacific Ocean, exerts on the regional circulation patterns in the proximity of the Andes, the need of coupled atmosphere-ocean regional models arise as one possible way to move forward. Of course, other components of the climate system may also play a significant role in shaping the main features of the regional and local climate in the Andes (e.g., improved land-surface schemes, coupling with urban models and with hydrological models, among others).
4.2.1 Earth systems platforms vs. atmosphere only models
The Andes mountains require the use of the most accurate and actualized morphological data, as well as, models that are capable to simulate the multiple processes and interactions that occur over the area. The Andes is one of the richest regions in the world in biodiversity with the presence of a large variety of climates and ecosystems. Ecosystem-climate numerical experiments must integrate rich processes in order to properly represent the area (Tovar et al., 2022). Andean water cycle involves a large diversity of features: local rain, snow, glaciers, wetlands, high altitude lakes, endorheic basins, and processes such as monsoon, glacier melting, tropical water recycle, atmospheric rivers, orographic precipitation, large water transport, groundwater flooding, and deep convection. In addition, ecosystem-climate numerical experiments need to consider other processes that influence the climate over the region such as transport of aerosols, wildfires and sea-air interactions (e.g., associated with upwellings).
Atmosphere Only Models (AOM) primarily represent the dynamics of the atmosphere at a high degree of detail (e.g., WRF). Other components of the climate system tend to not be as well represented as the atmosphere and they are only provided in a level of detail that is enough to satisfy the response of the atmosphere (e.g., land schemes in WRF atmospheric model). This allows a faster time of integration and less complexity when performing numerical experiments. However, in recent years, some of these models have been increasing the representation of the other components of the climate system, since performance of the model is sensitive to the dynamics of the other components (e.g., WRF can be used with the Community Land Model, and the most recent version of NoahMP land model integrates more processes over each iteration).
On the contrary, the Earth System Modeling platforms attempt to provide a depiction of the entire climate system as accurately as possible. These kinds of modeling platforms tend to be based on the dynamical coupling of dedicated models for each one of the components of the climate system. For example, RegIPSL11, couples WRF (atmosphere), ORCHIDEE (land), and NEMO (ocean). Previous regional modeling studies have coupled global or regional atmospheric and oceanic models in order to better represent the Humboldt current system and the coastal Peru-Chile upwelling system (e.g., Sepulchre et al., 2009; Oerder et al., 2016). Oerder et al. (2016) showed in particular a good representation of the coastal wind-SST coupling, that could in turn influence valley-wind systems in the western Andes valleys (e.g., Rosales et al., 2022). The increase of processes and features represented in the models should contribute to different purposes: (1) better depiction of the dynamics and response of the entire climate system, (2) better representation of all the elements present over a given area, (3) increase on suitability for impact and policy making of the climate data being produced since specific variables particular of each component are inherently being processed. The large diversity of features along the Andes mountain range, provokes a cascade of interactions and coupled responders of mechanisms and dynamics which are usually not present in current modeling platforms. This makes it necessary to enlarge the processes being represented in the modeling tools.
Due to the necessity of creating a coordinated modeling experiment in the Andes, a CORDEX FPS is currently in preparation under the umbrella of ANDEX. The main goal of this FPS would be to construct a tool of hydroclimatic simulation adapted to the specificity of the Andes in order to respond to the necessities of the Andean hydro-climate scientific community.
Data assimilation is crucial in atmospheric modeling in weather and climate for improving model accuracy by integrating observational data into the simulations (e.g., Hacker et al., 2018). In the case of the Andes, ground-based in-situ and radiosounding observations are very sparse, which hinders the use of data assimilation techniques. Satellite data is available, but mostly with spatial and temporal resolutions that have limitations for capturing many of the details over the Andes (e.g., Condom et al., 2020). To the best of our knowledge, there are no published research studies of atmospheric modeling including data assimilation focused on the Andes. Other studies focusing in other components or other regions have shown evidences of error reductions, for example, in hydrological modeling in the Amazon (Wongchuig et al., 2024), and in atmospheric modeling in Southeastern South America (Casaretto et al., 2023; Maldonado et al., 2020; Dillon et al., 2021; Corrales et al., 2023) and in other mountainous regions in the world (e.g., Carrió et al., 2019; Hacker et al., 2018; Doglioni et al., 2024). Therefore, including data assimilation in future atmospheric modeling studies in the Andes is among the priority challenges that could help refine initial conditions, reduce uncertainties, and improve the overall performance of models in capturing the complex interactions within the Andean hydroclimate system.
4.2.2 Interactions between the atmosphere and other climate components
Simulating correctly the spatiotemporal variability of precipitation in the tropical and subtropical Andes is a challenging task for an atmospheric model as it is a region of interplay between tropical convection processes and orographic circulations. It is paramount that these kinds of studies can be reproduced in other areas of the mountain range, and for larger periods in order to be able to truly analyze the climatic patterns in the area.
Future atmospheric simulations will benefit from increased (kilometer and sub-kilometer) higher resolution and more sophisticated model parameterizations for representing various processes, which will allow better characterizations and prediction of the atmospheric phenomena that shapes weather and climate (e.g., Palmer and Stevens, 2019). In addition, a high resolution (and more realistic) representation of the heterogeneity in land cover in the Andes is expected to improve the simulation of surface fluxes and near surface variables. Because of all these effects, kilometer-scale resolution is expected to be associated with a more realistic simulation of land-atmosphere interactions and the atmospheric branch of the hydrological cycle. This point is particularly relevant for the Andes considering the large rainforest surrounding this region (e.g., Amazon, Orinoco), playing crucial role in regional water cycle (e.g., Ruiz-Vásquez et al., 2020; Sierra et al., 2023; Wongchuig et al., 2021). In addition, moisture recycling through evapotranspiration is an important hydroclimate driver that could interact in some regions of the Andes with low level jets sourcing humidity (Bedoya-Soto and Poveda, 2024).
In turn, the better representation of some hydrological components could improve the local atmospheric processes. For example, in the region of the Altiplano, including the Titicaca lake (the largest highest navigable and freshwater lake in the world above 3000 m a.s.l.), some studies suggest that local surface atmospheric circulation processes (e.g., lake breezes) and associated convection development, controlling the local diurnal cycle of precipitation, are directly connected to the presence of the lake (e.g., Junquas et al., 2018; Zamuriano-Carbajal, 2019; Sierra et al., 2021). The highest places, where glaciers are situated, could also beneficiate of a better representation of the land cover, through improving local atmospheric mechanisms like turbulent and radiative fluxes (e.g., Wagnon et al., 2003; Sicart et al., 2014) and local katabatic winds (e.g., Ayala et al., 2015), particularly in a context of accelerated glacier retreat due to climate change (e.g., Vuille et al., 2008; Rabatel et al., 2013). Therefore, more studies are needed to understand the surface-atmosphere interaction processes and their relation with convection processes in the Andes, as well as their future evolution in the context of climate change (e.g., high-resolution dynamical downscaling, sensitivity experiments of the surface temperature variability over the local circulation like lake-breeze winds, katabatic winds, interactions with slope and valley winds, etc.).
There are still plenty of processes that have not been studied through numerical modeling which have an impact in the local and regional scales: high altitude wetland/floodplains, flash floods, landslides and erosion, biodiversity and impact of the climate change, deforestation, food production, wildfires and impact and adaptation studies. With an increase in model resolution, land-surface schemes would have to be more sophisticated, including a more detailed representation of some processes, partly due to a more detailed specification of land cover types. For example, some land-cover features, like lakes and small urban locations, require a detailed configuration within atmospheric models, both in terms of their geophysical parameters (e.g., albedo) and their effects on surface fluxes (e.g., via the use of lake or urban-canopy models). In addition, parts of the higher/colder terrain of the Andes can require a more detailed representation of surface fluxes (e.g., the partitioning between rainfall and snowfall) associated with glaciers, ice fields, wetlands, etc. In other parts of the world, it has been shown for example, the positive impact when including the representation of the water table on the climate of the great plains in North America (Barlage et al., 2015) and South America (e.g., Martinez et al., 2016), or the wetlands of Pantanal when representing the discharge of the Paraná river (Schrapffer et al., 2020).
On the other hand, PBL schemes will require more testing and development, especially in sub-kilometer simulations, which constitutes a gray zone (or “terra incognita”) for these schemes (e.g., Honnert et al., 2020; LeMone et al., 2019). Currently, most PBL schemes in atmospheric models are column models, with some assumptions related to flat terrain which might lead to limitations over mountainous terrain (Serafin et al., 2018), as in the case of the Andes. In addition, in sub-kilometer simulations the effects of both vertical and horizontal eddy fluxes can be equally important, and a 3D turbulence scheme would be needed (e.g., Olson et al., 2019). Complex terrain can also impact radiative fluxes, because of shadow effects from nearby topographic features and/or effects from upward longwave radiation over nearby slopes (Serafin et al., 2018). Some numerical models have some kind of representation of these effects (e.g., Skamarock et al., 2019).
It is worth mentioning that very few studies exist using a coupled ocean-atmosphere model to explore how the SST variability impacts the Andes climate. Recently, Cerón et al. (2021) identified a particular dipole-like SST structure producing below average precipitation along the Colombian Pacific region. ENSO events can also impact the surface component through anomalies in river discharge, soil moisture and NDVI in the Andes (e.g., Poveda et al., 2010; 2011). Consequently, a complete regional earth system modeling would be required to reproduce the complete interactions and feedbacks between all sub-systems occurring during these events. In addition, a better understanding of the different influences of projected Atlantic/Pacific SST over the atmospheric processes in the Andes would be needed in the context of climate change in order to better identify future hydroclimate trajectories.
4.3 Societal impacts and potential benefits of models for decision-making
Scientific research by Andean universities and institutes is highly constrained by a general lack of funding, especially from the Andean countries with for example, limited amount of tenure track and postdoctoral positions, scholarships for graduate students, equipment (including computational resources) and other resources and tools needed for research in water, weather and climate. In addition, the lack of funding is also reflected in the scarcity of observations of crucial variables over the Andes, affecting not only monitoring operations, but also numerical modeling activities (e.g., validation, data assimilation, etc.).
Currently, there are different ongoing projects able to greatly boost research about numerical modeling of the weather and climate of the Andes. The GEWEX Regional Hydroclimate Project for the Andes (ANDEX, https://www.gewex.org/project/andex/) will assist to create the necessary connections within the community in order to coordinate efforts and be capable to tackle the already recognized challenges and the ones to come in the future. In addition, the South American Affinity Group (SAAG, https://ral.ucar.edu/projects/south-america-affinity-group-saag), hosted by NCAR and gathering more than 100 researchers and scholars, including many from Andean institutions allows not only access to CP continental scale simulations (performed by NCAR), but also provides scientists from different regions (including the U.S. and South America) to share and discuss about scientific questions and methods relevant to the hydroclimate of South America (Dominguez et al., 2024), including the Andes.
Stakeholders, policy making and the society at large over the Andes can benefit from improving numerical modeling of weather and climate over the region. Essential Climate Variables (ECVs) like precipitation, near surface temperature, humidity and winds, among others, are associated with natural hazards and resources. Thus, ECVs estimates from numerical models can contribute an important source of climate information and services, impacting the safety, livelihoods and economic prosperity of nations, and the protection of ecosystems and natural resources. Forecasts (e.g., at the synoptic or seasonal scale) with atmospheric models can contribute to Early Warning Systems (EWS) in multiple scales, informing communities about hazards like heavy precipitation events or droughts. This is in line with the “Sendai Framework for Disaster Risk Reduction” (UNDRR, 2015) and the “Early Warnings for All” initiative from the United Nations (WMO, 2022). Furthermore, climate projections from dynamical numerical models are an instrumental tool in estimating the potential changes in ECVs associated with anthropogenic climate change (IPCC, 2021). In this sense, the study and improvement of climate projections is in line with the Climate Action goal of the 2030 Agenda for Sustainable Development (UN, 2015). As described in part I of this review (Martinez et al., in revision), the raw values of ECVs from dynamical models for the Andes are associated with biases and uncertainties of different types. Therefore, different types of bias correction, interpolation and other post processing techniques must be used (e.g., Mourre et al., 2016; Heredia et al., 2018) in order to provide ECVs estimates suitable for instances, like hydrological, hydraulic, ecological and other impact models. This task requires a better integration of climate scientists with researchers from other disciplines in the Andes, seeking for interdisciplinary fruitful collaborations.
Numerical modeling of the atmospheric processes that shape the hydroclimate of the Andes is very challenging, but significant progress has been made. Future modeling efforts will include the improvement of dynamical models (e.g., see Section 4.2), in addition to the coupling with recent advances in Artificial Intelligence, including Machine Learning methods (e.g., Haupt et al., 2021). With enough observation-based data dynamical models could even become obsolete at some point (e.g., Schultz et al., 2021). In the case of the Andes, there are still many challenges, including the lack of observations (Condom et al., 2020) and data assimilation studies (Hacker et al., 2018), as well as the urgent need for understanding the mechanisms associated with weather systems (e.g., Arias et al., 2021a), local climate (Espinoza et al., 2020; Poveda et al., 2020) and their potential changes under global warming (Pabón-Caicedo et al., 2020). This warrants the scientific research on both numerical modeling (e.g., Palmer and Stevens, 2019) and basic climate science (Emanuel, 2020), which is expected to keep contributing to a better recognition and protection of our environment and communities in the Andes.
Author contributions
CJ: Conceptualization, Investigation, Project administration, Writing–original draft, Writing–review and editing. JM: Conceptualization, Investigation, Project administration, Writing–original draft, Writing–review and editing. DB: Writing–original draft, Writing–review and editing. MV: Writing–original draft, Writing–review and editing. LF: Writing–original draft, Writing–review and editing. KT: Writing–original draft, Writing–review and editing. LC: Writing–original draft, Writing–review and editing. PA: Writing–original draft, Writing–review and editing. JB: Writing–original draft, Writing–review and editing. TC: Writing–original draft, Writing–review and editing. KG: Writing–original draft, Writing–review and editing. JP-C: Writing–original draft, Writing–review and editing. GP: Writing–original draft, Writing–review and editing. SS: Writing–original draft, Writing–review and editing. AS: Writing–original draft, Writing–review and editing. JE: Project administration, Writing–original draft, Writing–review and editing.
Funding
The author(s) declare that financial support was received for the research, authorship, and/or publication of this article. This research has been supported by the CECC project (Water Cycle and Climate Change, IRD/AFD) and the HighRes-AmSur project from the regional program CLIMAT-AmSud (22-CLIMAT-05). JM and PA are supported by MINCIENCIAS through program No. 80740-49-2020 and by ClimatAmSud through MINCIENCIAS grant 80740-238-2021. KG acknowledges support from ANID (Concurso de Fortalecimiento al Desarrollo Científico de Centros Regionales 2020-R20F0008-CEAZA and Fondecyt regular 1241321). DB and JB acknowledge support from ANID-FONDAP-1523A0002. DB acknowledges support from COPAS COASTAL ANID FB210021.
Acknowledgments
Comments and suggestions provided by two reviewers were very helpful in improving this paper. This work was developed within the GEWEX Regional Hydroclimate Project for the Andes (ANDEX, https://www.gewex.org/project/andex/). The authors also thank Yamina Silva (IGP, Peru) and Luis Blacutt (UMSA, Bolivia) for their participation in discussions. The authors dedicate this study to the memory of Eugenia Kalnay, whose work was fundamental to the international Atmospheric Science community and to the memory of Kyoko Ikeda, whose work greatly contributed to atmospheric modeling in South America.
Conflict of interest
The authors declare that the research was conducted in the absence of any commercial or financial relationships that could be construed as a potential conflict of interest.
The author(s) declared that they were an editorial board member of Frontiers, at the time of submission. This had no impact on the peer review process and the final decision.
Publisher’s note
All claims expressed in this article are solely those of the authors and do not necessarily represent those of their affiliated organizations, or those of the publisher, the editors and the reviewers. Any product that may be evaluated in this article, or claim that may be made by its manufacturer, is not guaranteed or endorsed by the publisher.
Supplementary material
The Supplementary Material for this article can be found online at: https://www.frontiersin.org/articles/10.3389/feart.2024.1427837/full#supplementary-material
Footnotes
1IPSL Regional Earth System model, https://gitlab.in2p3.fr/ipsl/lmd/intro/regipsl/regipsl/-/wikis/home
References
Almazroui, M., Ashfaq, M., Islam, M. N., Rashid, I. U., Kamil, S., Abid, M. A., et al. (2021). Assessment of CMIP6 performance and projected temperature and precipitation changes over South America. Earth Syst. Environ. 5, 155–183. doi:10.1007/s41748-021-00233-6
Al-Yaari, A., Condom, T., Junquas, C., Rabatel, A., Ramseyer, V., Sicart, J.-E., et al. (2023). Climate variability and glacier evolution at selected sites across the world: past trends and future projections. Earth's Future 11, e2023EF003618. doi:10.1029/2023EF003618
Arias, P. A., Garreaud, R., Poveda, G., Espinoza, J. C., Molina-Carpio, J., Masiokas, M., et al. (2021a). Hydroclimate of the andes part II: hydroclimate variability and sub-continental patterns. Front. Earth Sci. 8, 505467. doi:10.3389/feart.2020.505467
Arias, P. A., Ortega, G., Villegas, L. D., and Martínez, J. A. (2021b). Colombian climatology in CMIP5/CMIP6 models: persistent biases and improvements. Rev. Fac. Ing. Univ. Antioquia 100, 75–96. doi:10.17533/udea.redin.20210525
Ayala, A., Pellicciotti, F., and Shea, J. M. (2015). Modeling 2 m air temperatures over mountain glaciers: exploring the influence of katabatic cooling and external warming. J. Geophys. Res. Atmos. 120, 3139–3157. doi:10.1002/2015JD023137
Baladima, F., Thomas, J. L., Voisin, D., Dumont, M., Junquas, C., Kumar, R., et al. (2022). Modeling an extreme dust deposition event to the French alpine seasonal snowpack in April 2018: meteorological context and predictions of dust deposition. J. Geophys. Res. Atmos. 127, e2021JD035745. doi:10.1029/2021JD035745
Bambach, N. E., Rhoades, A. M., Hatchett, B. J., Jones, A. D., Ullrich, P. A., and Zarzycki, C. M. (2022). Projecting climate change in SouthSouth America using variable-resolution community earth system model: an application to Chile. Int. J. Climatol. 42, 2514–2542. doi:10.1002/joc.7379
Barlage, M., Tewari, M., Chen, F., Miguez-Macho, G., Yang, Z. L., and Niu, G. Y. (2015). The effect of groundwater interaction in North American regional climate simulations with WRF/Noah-MP. Clim. Change 129, 485–498. doi:10.1007/s10584-014-1308-8
Bedoya-Soto, J. M., and Poveda, G. (2024). Moisture recycling in the Colombian andes. Water Resour. Res. 60, e2022WR033601. doi:10.1029/2022WR033601
Berbery, E. H., and Barros, V. R. (2002). The hydrologic cycle of the La Plata basin in South America. J. Hydrometeorol. 3 (6), 630–645. doi:10.1175/1525-7541(2002)003<0630:thcotl>2.0.co;2
Bieniek, P. A., Bhatt, U. S., Walsh, J. E., Rupp, T. S., Zhang, J., Krieger, J. R., et al. (2016). Dynamical downscaling of ERA-Interim temperature and precipitation for Alaska. J. Appl. Meteor Climatol. 55, 635–654. doi:10.1175/JAMC-D-15-0153.1
Blázquez, J., and Solman, A. S. (2020). Multiscale precipitation variability and extremes over South America: analysis of future changes from a set of CORDEX regional climate model simulations. Clim. Dyn. 55, 2089–2106. doi:10.1007/s00382-020-05370-8
Blázquez, J., and Solman, S. (2023). Temperature and precipitation biases in CORDEX RCM simulations over South America: possible origin and impacts on the regional climate change signal. Clim. Dyn. 61, 2907–2920. doi:10.1007/s00382-023-06727-5
Boisier, J. P., Alvarez-Garretón, C., Cordero, R. R., Damiani, A., Gallardo, L., Garreaud, R. D., et al. (2018). Anthropogenic drying in central-southern Chile evidenced by long-term observations and climate model simulations. Elem. Sci. Anth 6, 74. doi:10.1525/elementa.328
Bozkurt, D., Rojas, M., Boisier, J. B., Rondanelli, R., Garreaud, R., and Gallardo, L. (2019). Dynamical downscaling over the complex terrain of southwest South America: present climate conditions and added value analysis. Clim. Dyn. 53, 6745–6767. doi:10.1007/s00382-019-04959-y
Bozkurt, D., Rojas, M., Boisier, J. P., and Valdivieso, J. (2018). Projected hydroclimate changes over Andean basins in central Chile from downscaled CMIP5 models under the low and high emission scenarios. Clim. Change 150, 131–147. doi:10.1007/s10584-018-2246-7
Bozkurt, D., Turuncoglu, U., Sen, O. L., and Dalfes, H. N. (2012). Downscaled simulations of the ECHAM5, CCSM3 and HadCM3 global models for the Eastern Mediterranean-Black Sea region: evaluation of the reference period. Clim. Dyn. 39 (1–2), 207–225. doi:10.1007/s00382-011-1187-x
Cai, W., McPhaden, M. J., Grimm, A. M., Rodrigues, R. R., Taschetto, A. S., Garreaud, R. D., et al. (2020). Climate impacts of the el niño–southern oscillation on South America. Nat. Rev. Earth Environ. 1, 215–231. doi:10.1038/s43017-020-0040-3
Cano, L. M., Carmona, M. A., Martínez, J. A., and Arias, P. A. (2022). Estimación y pronóstico de radiación solar en el valle de Aburrá – Colombia. Rev. la Acad. Colomb. Ciencias Exactas, Físicas Nat. 46 (179), 529–549. doi:10.18257/raccefyn.1576
Carrió, D. S., Homar, V., and Wheatley, D. M. (2019). Potential of an EnKF storm-scale data assimilation system over sparse observation regions with complex orography. Atmos. Res. 216, 186–206. doi:10.1016/j.atmosres.2018.10.004
Casaretto, G., Dillon, M. E., Skabar, Y. G., Ruiz, J. J., and Sacco, M. (2023). Ensemble Forecast Sensitivity to Observations Impact (EFSOI) applied to a regional data assimilation system over south-eastern South America. Atmos. Res. 295, 106996. doi:10.1016/j.atmosres.2023.106996
Cerón, W. L., Kayano, M. T., Andreoli, R. V., Canchala, T., Carvajal-Escobar, Y., and Alfonso-Morales, W. (2021). Rainfall variability in southwestern Colombia: changes in ENSO-related features. Pure Appl. Geophys. 178 (3), 1087–1103. doi:10.1007/s00024-021-02673-7
Chimborazo, O., Minder, J. R., and Vuille, M. (2022). Observations and simulated mechanisms of elevation-dependent warming over the tropical andes. J. Clim. 35 (3), 1021–1044. doi:10.1175/jcli-d-21-0379.1
Chimborazo, O., and Vuille, M. (2021). Present-day climate and projected future temperature and precipitation changes in Ecuador. Theor. Appl. Climatol. 143, 1581–1597. doi:10.1007/s00704-020-03483-y
Comin, A. N., Schumacher, V., Justino, F., and Fernrández, A. (2018). Impact of different microphysical parameterizations on extreme snowfall events in the Southern Andes. Weather Clim. Extrem. 21, 65–75. doi:10.1016/j.wace.2018.07.001
Condom, T., Martínez, R., Pabón, J. D., Costa, F., Pineda, L., Nieto, J. J., et al. (2020). Climatological and hydrological observations for the South American Andes: in situ stations, satellite, and reanalysis data sets. Front. Earth Sci. 8, 92. doi:10.3389/feart.2020.00092
Corrales, P. B., Galligani, V., Ruiz, J., Sapucci, L., Dillon, M. E., Skabar, Y. G., et al. (2023). Hourly assimilation of different sources of observations including satellite radiances in a mesoscale convective system case during RELAMPAGO campaign. Atmos. Res. 281, 106456. doi:10.1016/j.atmosres.2022.106456
Díaz, L. B., Saurral, R. I., and Vera, C. S. (2020). Assessment of South America summer rainfall climatology and trends in a set of global climate models large ensembles. Int. J. Climatol. 41, 1–19. doi:10.1002/joc.6643
Dillon, M. E., Maldonado, P., Corrales, P., Skabar, Y. G., Ruiz, J., Sacco, M., et al. (2021). A rapid refresh ensemble based data assimilation and forecast system for the RELAMPAGO field campaign. Atmos. Res. 264, 105858. doi:10.1016/j.atmosres.2021.105858
Di Luca, A., Elia, R., and Laprise, R. (2013). Potential for small scale added value of RCMs downscaled climate change signal. Clim. Dyn. 40 (3–4), 601–618. doi:10.1007/s00382-012-1415-z
Doglioni, G., Serafin, S., Weissmann, M., Ferrari, G., and Zardi, D. (2024). The assimilation of surface observations in mountainous terrain in the WRFDA system. EGU General Assem., EGU24–15473. doi:10.5194/egusphere-egu24-15473
Dominguez, F., Rasmussen, R., Liu, C., Ikeda, K., Prein, A., Varble, A., et al. (2024). Advancing South American water and climate science through multidecadal convection-permitting modeling. Bull. Am. Meteorological Soc. 105 (1), E32–E44. doi:10.1175/bams-d-22-0226.1
Dudhia, J. (1989). Numerical study of convection observed during the winter monsoon experiment using a mesoscale two-dimensional model. J. Atmos. Sci. 46 (20), 3077–3107. doi:10.1175/1520-0469(1989)046<3077:nsocod>2.0.co;2
Eghdami, M., and Barros, A. P. (2019). Extreme orographic rainfall in the eastern andes tied to cold air intrusions. Front. Environ. Sci. 7. doi:10.3389/fenvs.2019.00101
Emanuel, K. (2020). The relevance of theory for contemporary research in atmospheres, oceans, and climate. AGU Adv. 1 (2), e2019AV000129. doi:10.1029/2019av000129
Espinoza, J. C., Garreaud, R., Poveda, G., Arias, P. A., Molina-Carpio, J., Masiokas, M., et al. (2020). Hydroclimate of the Andes Part I: main climatic features. Front. Earth Sci. 8, 64. doi:10.3389/feart.2020.00064
Eva, H. D., Belward, A. S., De Miranda, E. E., Di Bella, C. M., Gond, V., Huber, O., et al. (2004). A land cover map of South America. Glob. Chang. Biol. 10, 731–744. doi:10.1111/j.1529-8817.2003.00774.x
Falco, M., Carril, A. F., Li, L. Z. X., Cabrelli, C., and Menéndez, C. G. (2020). The potential added value of Regional Climate Models in South America using a multiresolution approach. Clim. Dyn. 54, 1553–1569. doi:10.1007/s00382-019-05073-9
Farr, T. G., Rosen, P. A., Caro, E., Crippen, R., Duren, R., Hensley, S., et al. (2007). The shuttle radar topography mission. Rev. Geophys 45 (2), RG2004. doi:10.1029/2005rg000183
Fernández, A., Schumacher, V., Ciocca, I., Rifo, A., Muñoz, A. A., and Justino, F. (2021). Validation of a 9-km WRF dynamical downscaling of temperature and precipitation for the period 1980–2005 over Central South Chile. Theor. Appl. Climatol. 143, 361–378. doi:10.1007/s00704-020-03416-9
Flores Rojas, J. L., Moya-Alvarez, A. S., Kumar, S., Martínez-Castro, D., Villalobos-Puma, E., and Silva-Vidal, Y. (2019). Analysis of possible triggering mechanisms of severe thunderstorms in the tropical central Andes of Peru, Mantaro Valley. Atmosphere 10 (6), 301. doi:10.3390/atmos10060301
Giorgi, F. (2019). Thirty years of regional climate modeling: where are we and where are we going next? J. Geophys. Res. Atmos. 124 (11), 5696–5723. doi:10.1029/2018jd030094
Giorgi, F., Jones, C., and Asrar, G. R. (2009). Addressing climate information needs at the regional level: the CORDEX framework. World Meteorol. Organ. (WMO) Bull. 58 (3), 175.
Gomez-Rios, S., Zuluaga, M. D., and Hoyos, C. D. (2023). Orographic controls over convection in an inter-andean valley in northern South America. Mon. Weather Rev. 151 (1), 145–162. doi:10.1175/mwr-d-21-0231.1
González-Rojí, S. J., Messmer, M., Raible, C. C., and Stocker, T. F. (2022). Sensitivity of precipitation in the highlands and lowlands of Peru to physics parameterization options in WRFV3.8.1. Geosci. Model Dev. 15, 2859–2879. doi:10.5194/gmd-15-2859-2022
Grell, G. A., and Freitas, S. R. (2013). A scale and aerosol aware stochastic convective parameterization for weather and air quality modeling. Atmos. Chem. Phys. Discuss. 13 (9), 23845–23893. doi:10.5194/acp-14-5233-2014
Gutierrez, R. A., Junquas, C., Armijos, E., Sörensson, A. A., and Espinoza, J.-C. (2024). Performance of regional climate model precipitation simulations over the terrain-complex Andes-Amazon transition region. J. Geophys. Res. Atmos. 129, e2023JD038618. doi:10.1029/2023JD038618
Gutowski, W. J., Ullrich, P. A., Hall, A., Leung, L. R., O’Brien, T. A., Patricola, C. M., et al. (2020). The ongoing need for high-resolution regional climate models: process understanding and stakeholder information. Bull. Am. Meteorological Soc. 101 (5), E664–E683. doi:10.1175/bams-d-19-0113.1
Gutowski Jr, W. J., Giorgi, F., Timbal, B., Frigon, A., Jacob, D., Kang, H. S., et al. (2016). WCRP coordinated regional downscaling experiment (CORDEX): a diagnostic MIP for CMIP6. Geosci. Model Dev. 9 (11), 4087–4095. doi:10.5194/gmd-9-4087-2016
González-Rojí, S. J., Messmer, M., Raible, C. C., and Stocker, T. F. (2022). Sensitivity of precipitation in the highlands and lowlands of Peru to physics parameterization options in WRFV3. 8.1. Geosci. Model Dev. 15 (7), 2859–2879.
Hacker, J., Draper, C., and Madaus, L. (2018). Challenges and opportunities for data assimilation in mountainous environments. Atmosphere 9 (4), 127. doi:10.3390/atmos9040127
Halladay, K., Kahana, R., Berthou, S., and Kendon, E. (2022). Convection-permitting climate simulations for South America: a land-surface perspective (with insights from Africa and Europe). Available at: https://www.cima.fcen.uba.ar/cpcmw2022/Posters/P4-2_KateHalladay.pdf.
Halladay, K., Kahana, R., Johnson, B., Still, C., Fosser, G., and Alves, L. (2023). Convection-permitting climate simulations for SouthSouth America with the Met Office unified model. Clim. Dyn. doi:10.1007/s00382-023-06853-0
Haupt, S. E., Chapman, W., Adams, S. V., Kirkwood, C., Hosking, J. S., Robinson, N. H., et al. (2021). Towards implementing artificial intelligence post-processing in weather and climate: proposed actions from the Oxford 2019 workshop. Philosophical Trans. R. Soc. A 379 (2194), 20200091. doi:10.1098/rsta.2020.0091
Hausfather, Z., Marvel, K., Schmidt, G. A., Nielsen-Gammon, J. W., and Zelinka, M. (2022). Climate simulations: recognize the ‘hot model’ problem. Nature 605, 26–29. doi:10.1038/d41586-022-01192-2
Henao, J. J., Mejía, J. F., Rendón, A. M., and Salazar, J. F. (2020). Sub-kilometer dispersion simulation of a CO tracer for an inter-Andean urban valley. Atmos. Pollut. Res. 11 (5), 928–945. doi:10.1016/j.apr.2020.02.005
Heredia, M. B., Junquas, C., Prieur, C., and Condom, T. (2018). New statistical methods for precipitation bias correction applied to WRF model simulations in the Antisana region, Ecuador. J. Hydrometeorol. 19 (12), 2021–2040. doi:10.1175/jhm-d-18-0032.1
Hodnebrog, Ø., Steensen, B. M., Marelle, L., Alterskjaer, K., Dalsøren, S. B., and Myhre, G. (2021). Understanding model diversity in future precipitation projections for South America. Clim. Dyn. 58, 1329–1347. doi:10.1007/s00382-021-05964-w
Hong, S.-Y., Noh, Y., and Dudhia, J. (2006). A new vertical diffusion package with an explicit treatment of entrainment processes. Mon. Wea. Rev. 134, 2318–2341. doi:10.1175/mwr3199.1
Honnert, R., Efstathiou, G., Beare, R., Ito, J., Lock, A., Neggers, R., et al. (2020). The atmospheric boundarylayer and the “gray zone” ofturbulence: a critical review. J. Geophys. Research:Atmospheres 125, e2019JD030317. doi:10.1029/2019jd030317
Insel, N., Poulsen, C. J., and Ehlers, T. A. (2010). Influence of the Andes Mountains on South American moisture transport, convection, and precipitation. Clim. Dyn. 35 (7), 1477–1492. doi:10.1007/s00382-009-0637-1
IPCC (2021). “Summary for policymakers,” in Climate change 2021: the physical science basis. Contribution of working group I to the sixth assessment report of the intergovernmental panel on climate change. Editors V. Masson-Delmotte, P. Zhai, A. Pirani, S. L. Connors, C. Péan, S. Bergeret al. (Cambridge, United Kingdom and New York, NY, USA: Cambridge University Press), 3–32. doi:10.1017/9781009157896.001
Janjic, Z. I. (1994). The step-mountain eta coordinate model: further developments of the convection, viscous sublayer, and turbulence closure schemes. Mon. Weather Rev. 122 (5), 927–945. doi:10.1175/1520-0493(1994)122<0927:tsmecm>2.0.co;2
Jiménez Sánchez, G., Markowski, P. M., Jewtoukoff, V., Young, G. S., and Stensrud, D. J. (2019). The Orinoco low level jet: an investigation of its characteristics and evolution using the WRF model. J. Geophys. Res. Atmos. 124 (20), 10696–10711. doi:10.1029/2019jd030934
Jiménez Sánchez, G., Markowski, P. M., Young, G. S., and Stensrud, D. J. (2020). The Orinoco low-level jet: an investigation of its mechanisms of formation using the WRF model. J. Geophys. Res. Atmos. 125 (13), e2020JD032810. doi:10.1029/2020jd032810
Junquas, C., Heredia, M. B., Condom, T., Ruiz-Hernández, J. C., Campozano, L., Dudhia, J., et al. (2022). Regional climate modeling of the diurnal cycle of precipitation and associated atmospheric circulation patterns over an Andean glacier region (Antisana, Ecuador). Clim. Dyn. 58, 3075–3104. doi:10.1007/s00382-021-06079-y
Junquas, C., Li, L., Vera, C. S., Le Treut, H., and Takahashi, K. (2016). Influence of SouthSouth America orography on summertime precipitation in Southeastern South America. Clim. Dyn. 46 (11), 3941–3963. doi:10.1007/s00382-015-2814-8
Junquas, C., Takahashi, K., Condom, T., Espinoza, J. C., Chávez, S., Sicart, J. E., et al. (2018). Understanding the influence of orography on the precipitation diurnal cycle and the associated atmospheric processes in the central Andes. Clim. Dyn. 50 (11), 3995–4017. doi:10.1007/s00382-017-3858-8
Kendon, E. J., Prein, A. F., Senior, C. A., and Stirling, A. (2021). Challenges and outlook for convection-permitting climate modelling. Philosophical Trans. R. Soc. A 379 (2195), 20190547. doi:10.1098/rsta.2019.0547
LeMone, M. A., Angevine, W. M., Bretherton, C. S., Chen, F., Dudhia, J., Fedorovich, E., et al. (2019). 100 years of progress in boundary-layer meteorology. A century of progress in atmospheric and related sciences: celebrating the American meteorological society centennial. Meteorol. Monogr. Amer Meteorol. Soc. 59 (9.1–9.85), 9.1–9.85. doi:10.1175/amsmonographs-d-18-0013.1
Lenaerts, J. T., Van Den Broeke, M. R., van Wessem, J. M., van de Berg, W. J., van Meijgaard, E., van Ulft, L. H., et al. (2014). Extreme precipitation and climate gradients in Patagonia revealed by high-resolution regional atmospheric climate modeling. J. Clim. 27 (12), 4607–4621. doi:10.1175/jcli-d-13-00579.1
Lucas Picher, P., Argüeso, D., Brisson, E., Tramblay, Y., Berg, P., Lemonsu, A., et al. (2021). Convection-permitting modeling with regional climate models: latest developments and next steps. Wiley Interdiscip. Rev. Clim. Change 12 (6), e731. doi:10.1002/wcc.731
Lucas-Picher, P., Laprise, R., and Winger, K. (2017). Evidence of added value in North American regional climate model hindcast simulations using ever-increasing horizontal resolutions. Clim. Dyn. 48 (7-8), 2611–2633. doi:10.1007/s00382-016-3227-z
Maldonado, P., Ruiz, J., and Saulo, y C. (2020). Parameter sensitivity of the WRF-LETKF system for assimilation of radar observations: imperfect-model observing system simulation experiments. Wea. Forecast. 35 (4), 1345–1362. doi:10.1175/WAF-D-19-0161.1
Mamani, R., and Hendrick, P. (2021). WRF model parameterization around the highland Titicaca Lake. Earth Space Sci. 8 (12), e2021EA001649. doi:10.1029/2021ea001649
Martinez, J. A., Arias, P. A., Castro, C., Chang, H. I., and Ochoa Moya, C. A. (2019). Sea surface temperature related response of precipitation in northern South America according to a WRF multi-decadal simulation. Int. J. Climatol. 39 (4), 2136–2155. doi:10.1002/joc.5940
Martinez, J. A., Arias, P. A., Junquas, C., Espinoza, J. C., Condom, T., Dominguez, F., et al. (2022). The Orinoco low-level jet and the cross equatorial moisture transport over tropical South America: lessons from seasonal WRF simulations. J. Geophys. Res. Atmos. 127, e2021JD035603. doi:10.1029/2021jd035603
Martinez, J. A., Dominguez, F., and Miguez-Macho, G. (2016). Impacts of a groundwater scheme on hydroclimatological conditions over southern South America. J. Hydrometeorol. 17 (11), 2959–2978.
Martínez-Castro, D., Kumar, S., Flores Rojas, J. L., Moya-Álvarez, A., Valdivia-Prado, J. M., Villalobos-Puma, E., et al. (2019). The impact of microphysics parameterization in the simulation of two convective rainfall events over the central Andes of Peru using WRF-ARW. Atmosphere 10 (8), 442. doi:10.3390/atmos10080442
Mass, C. F., Ovens, D., Westrick, K., and Colle, B. A. (2002). Does increasing horizontal resolution produce more skillful forecasts? The results of two years of real-time numerical weather prediction over the pacific northwest. Bull. Am. Meteorological Soc. 83 (3), 407–430. doi:10.1175/1520-0477(2002)083<0407:dihrpm>2.3.co;2
Maussion, F., Scherer, D., Finkelnburg, R., Richters, J., Yang, W., and Yao, T. (2011). WRF simulation of a precipitation event over the Tibetan Plateau, China—an assessment using remote sensing and ground observations. Hydrol. Earth Syst. Sci. 15, 1795–1817. doi:10.5194/hess-15-1795-2011
Mourre, L., Condom, T., Junquas, C., Lebel, T., E Sicart, J., Figueroa, R., et al. (2016). Spatio-temporal assessment of WRF, TRMM and in situ precipitation data in a tropical mountain environment (Cordillera Blanca, Peru). Hydrology Earth Syst. Sci. 20 (1), 125–141. doi:10.5194/hess-20-125-2016
Moya-Álvarez, A. S., Estevan, R., Kumar, S., Rojas, J. L. F., Ticse, J. J., Martínez-Castro, D., et al. (2020). Influence of PBL parameterization schemes in WRF_ARW model on short-range precipitation's forecasts in the complex orography of Peruvian Central Andes. Atmos. Res. 233, 104708. doi:10.1016/j.atmosres.2019.104708
Moya-Álvarez, A. S., Gálvez, J., Holguín, A., Estevan, R., Kumar, S., Villalobos, E., et al. (2018b). Extreme rainfall forecast with the WRF-ARW model in the Central Andes of Peru. Atmosphere 9 (9), 362. doi:10.3390/atmos9090362
Moya-Álvarez, A. S., Martínez-Castro, D., Flores, J. L., and Silva, Y. (2018a). Sensitivity study on the influence of parameterization schemes in WRF_ARW model on short-and medium-range precipitation forecasts in the Central Andes of Peru. Adv. Meteorology 2018, 1–16. doi:10.1155/2018/1381092
Ochoa, A., Campozano, L., Sanchez, E., Gualan, R., and Samaniego, E. (2016). Evaluation of downscaled estimates of monthly temperature and precipitation for a Southern Ecuador case study. Int. J. Climatol. 36 (3), 1244–1255. doi:10.1002/joc.4418
Oerder, V., Colas, F., Echevin, V., Masson, S., Hourdin, C., Jullien, S., et al. (2016). Mesoscale SST–wind stress coupling in the Peru–Chile current system: which mechanisms drive its seasonal variability? Clim. Dyn. 47, 2309–2330. doi:10.1007/s00382-015-2965-7
Olmo, M. E., Espinoza, J.-C., Bettolli, M. L., Sierra, J. P., Junquas, C., Arias, P. A., et al. (2022). Circulation patterns and associated rainfall over south tropical South America: GCMs evaluation during the dry-to-wet transition season. J. Geophys. Res. Atmos. 127, e2022JD036468. doi:10.1029/2022JD036468
Olson, J. B., Kenyon, J. S., Djalalova, I., Bianco, L., Turner, D. D., Pichugina, Y., et al. (2019). Improving wind energy forecasting through numerical weather prediction model development. Bull. Am. Meteorological Soc. 100 (11), 2201–2220. doi:10.1175/bams-d-18-0040.1
Onol, B. (2012). Effects of coastal topography on climate: high-resolution simulation with a regional climate model. Clim. Res. 52, 159–174. doi:10.3354/cr01077
Ortega, G., Arias, P. A., Villegas, J. C., Marquet, P. A., and Nobre, P. (2021). Present day and future climate over central and South America according to CMIP5/CMIP6 models. Int. J. Climatol. 41 (15), 6713–6735. doi:10.1002/joc.7221
Pabón-Caicedo, J. D., Arias, P. A., Carril, A. F., Espinoza, J. C., Borrel, L. F., Goubanova, K., et al. (2020). Observed and projected hydroclimate changes in the Andes. Front. Earth Sci. 8, 61. doi:10.3389/feart.2020.00061
Palazzi, E., Mortarini, L., Terzago, S., and Von Hardenberg, J. (2019). Elevation-dependent warming in global climate model simulations at high spatial resolution. Clim. Dyn. 52 (5-6), 2685–2702. doi:10.1007/s00382-018-4287-z
Palmer, T., and Stevens, B. (2019). The scientific challenge of understanding and estimating climate change. Proc. Natl. Acad. Sci. 116 (49), 24390–24395. doi:10.1073/pnas.1906691116
Pepin, N. C., Arnone, E., Gobiet, A., Haslinger, K., Kotlarski, S., Notarnicola, C., et al. (2022). Climate changes and their elevational patterns in the mountains of the world. Rev. Geophys. 60, e2020RG000730. doi:10.1029/2020RG000730
Pielke, R. A. (2013). “Sr,” in Mesoscale meteorological modeling, 98. Academic Press, Univ. of Michigan.
Posada-Marín, J. A., Rendón, A. M., Salazar, J. F., Mejía, J. F., and Villegas, J. C. (2019). WRF downscaling improves ERA-Interim representation of precipitation around a tropical Andean valley during El Niño: implications for GCM-scale simulation of precipitation over complex terrain. Clim. Dyn. 52 (5), 3609–3629. doi:10.1007/s00382-018-4403-0
Poveda, G., Álvarez, D. M., and Rueda, Ó. A. (2011). Hydro-climatic variability over the Andes of Colombia associated with ENSO: a review of climatic processes and their impact on one of the Earth’s most important biodiversity hotspots. Clim. Dyn. 36 (11), 2233–2249. doi:10.1007/s00382-010-0931-y
Poveda, G., Espinoza, J. C., Zuluaga, M. D., Solman, S. A., Garreaud, R., and van Oevelen, P. J. (2020). High impact weather events in the Andes. Front. Earth Sci. 8, 162. doi:10.3389/feart.2020.00162
Poveda, G., Graham, N. E., Epstein, P. R., and Rojas, W. (2010). Climate and ENSO variability associated with vector-borne diseases in Colombia. El Nino South. Oscillation, 183–204. doi:10.1017/cbo9780511573125.007
Prein, A. F., Langhans, W., Fosser, G., Ferrone, A., Ban, N., Goergen, K., et al. (2015). A review on regional convection-permitting climate modeling: demonstrations, prospects, and challenges. Rev. Geophys. 53 (2), 323–361. doi:10.1002/2014rg000475
Rabatel, A., Francou, B., Soruco, A., Gomez, J., Cáceres, B., Ceballos, J. L., et al. (2013). Current state of glaciers in the tropical Andes: a multi-century perspective on glacier evolution and climate change. Cryosphere 7, 81–102. doi:10.5194/tc-7-81-2013
Rasmussen, K. L., and Houze Jr, R. A. (2016). Convective initiation near the Andes in subtropical South America. Mon. Weather Rev. 144 (6), 2351–2374. doi:10.1175/mwr-d-15-0058.1
Rauscher, S. A., Coppola, E., Piani, C., and Giorgi, F. (2010). Resolution effects on regional climate model simulations of seasonal precipitation over Europe. Clim. Dyn. 35, 685–711. doi:10.1007/s00382-009-0607-7
Riahi, K., Van Vuuren, D. P., Kriegler, E., Edmonds, J., O’Neill, B. C., Fuji- mori, S., et al. (2017). The shared socioeconomic pathways and their energy, land use, and greenhouse gas emissions implica-tions: an overview. Glob. Environ. Changw 42, 153–168. doi:10.1016/j.gloenvcha.2016.05.009
Rivera, J., and Arnould, G. (2020). Evaluation of the ability of CMIP6 models to simulate precipitation over Southwestern South America: climatic features and long-term trends (1901–2014). Atmos. Res. 241, 104953. doi:10.1016/j.atmosres.2020.104953
Rojas, M. (2006). Multiply nested regional climate simulation for southern South America: sensitivity to model resolution. Mon. Weather Rev. 134, 2208–2223. doi:10.1175/MWR3167.1
Rosales, A. G., Junquas, C., da Rocha, R. P., Condom, T., and Espinoza, J. C. (2022). Valley–Mountain circulation associated with the diurnal cycle of precipitation in the tropical andes (Santa river basin, Peru). Atmosphere 13, 344. doi:10.3390/atmos13020344
Ruiz-Vásquez, M., Arias, P. A., Martínez, J. A., and Espinoza, J. C. (2020). Effects of Amazon basin deforestation on regional atmospheric circulation and water vapor transport towards tropical South America. Clim. Dyn. 54, 4169–4189. doi:10.1007/s00382-020-05223-4
Saavedra, M., Junquas, C., Espinoza, J. C., and Silva, Y. (2020). Impacts of topography and land use changes on the air surface temperature and precipitation over the central Peruvian Andes. Atmos. Res. 234, 104711. doi:10.1016/j.atmosres.2019.104711
Salazar, A., Baldi, G., Hirota, M., Syktus, J., and McAlpine, C. (2015). Land use and land cover change impacts on the regional climate of non-Amazonian South America: a review. Glob. Planet. Change 128, 103–119. doi:10.1016/j.gloplacha.2015.02.009
Salazar, A., Katzfey, J., Thatcher, M., Syktus, J., Wong, K., and McAlpine, C. (2016). Deforestation changes land–atmosphere interactions across South American biomes. Glob. Planet. Change 139, 97–108. doi:10.1016/j.gloplacha.2016.01.004
Salazar, A., Thatcher, M., Goubanova, K., Bernal, P., Gutiérrez, J., and Squeo, F. (2024). CMIP6 precipitation and temperature projections for Chile. Clim. Dyn. 62, 2475–2498. doi:10.1007/s00382-023-07034-9
Sauter, T. (2020). Revisiting extreme precipitation amounts over southern South America and implications for the Patagonian Icefields. Hydrol. Earth Syst. Sci. 24, 2003–2016. doi:10.5194/hess-24-2003-2020
Schär, C., Frei, C., Lüthi, D., and Davies, H. C. (1996). Surrogate climate-change scenarios for regional climate models. Geophys. Res. Lett. 23 (6), 669–672. doi:10.1029/96GL00265
Schrapffer, A., Sörensson, A., Polcher, J., and Fita, L. (2020). Benefits of representing floodplains in a land surface model: pantanal simulated with ORCHIDEE CMIP6 version. Clim. Dyn. 55, 1303–1323. doi:10.1007/s00382-020-05324-0
Schultz, M. G., Betancourt, C., Gong, B., Kleinert, F., Langguth, M., Leufen, L. H., et al. (2021). Can deep learning beat numerical weather prediction? Philosophical Trans. R. Soc. A 379 (2194), 20200097. doi:10.1098/rsta.2020.0097
Schumacher, V., Alfonso, F., Flavio, J., and Alcimoni, C. (2020). WRF high resolution dynamical downscaling of precipitation for the central andes of Chile and Argentina. Front. Earth Sci. 8. doi:10.3389/feart.2020.00328
Schwartz, C. S., Kain, J. S., Weiss, S. J., Xue, M., Bright, D. R., Kong, F., et al. (2009). Next-day convection-allowing WRF model guidance: a second look at 2-km versus 4-km grid spacing. Mon. Weather Rev. 137 (10), 3351–3372.
Segura, H., Junquas, C., Espinoza, J. C., Vuille, M., Jauregui, Y. R., Rabatel, A., et al. (2019). New insights into the rainfall variability in the tropical Andes on seasonal and interannual time scales. Clim. Dyn. 53, 405–426. doi:10.1007/s00382-018-4590-8
Sepulchre, P., Sloan, L. C., Snyder, M., and Fiechter, J. (2009). Impacts of Andean uplift on the Humboldt Current system: a climate model sensitivity study. Paleoceanography 24, PA4215. doi:10.1029/2008PA001668
Serafin, S., Adler, B., Cuxart, J., De Wekker, S., Gohm, A., Grisogono, B., et al. (2018). Exchange processes in the atmospheric boundary layer over mountainous terrain. Atmosphere 9 (3), 102. doi:10.3390/atmos9030102
Sicart, J. E., Litt, M., Helgason, W., Tahar, V. B., and Chaperon, T. (2014). A study of the atmospheric surface layer and roughness lengths on the high altitude tropical Zongo glacier, Bolivia. J. Geophys. Res. Atmos. 119 (7), 3793–3808. doi:10.1002/2013jd020615
Sierra, J. P., Espinoza, J. C., Junquas, C., Wongchuig, S., Polcher, J., Moron, V., et al. (2023). Impacts of land-surface heterogeneities and Amazonian deforestation on the wet season onset in southern Amazon. Clim. Dyn. 61, 4867–4898. doi:10.1007/s00382-023-06835-2
Sierra, J. P., Junquas, C., Espinoza, J. C., Segura, H., Condom, T., Andrade, M., et al. (2021). Deforestation impacts on Amazon-Andes hydroclimatic connectivity. Clim. Dyn. 58, 2609–2636. doi:10.1007/s00382-021-06025-y
Skamarock, W. C., Klemp, J. B., Dudhia, J., Gill, D. O., Barker, D. M., Duda, M. G., et al. (2008). A description of the advanced research wrf version 3. NCAR Tech. Note NCAR/TN-475+STR 1.
Skamarock, W. C., Klemp, J. B., Dudhia, J., Gill, D. O., Liu, Z., Berner, J., et al. (2019). A description of the advanced research WRF model version 4 (Vol. 145). National Center for Atmospheric Research.
Soares, W., and Marengo, J. (2008). Assessments of moisture fluxes east of the Andes in South America in a global warming scenario. Int. J. Climatol. 29, 1395–1414. doi:10.1002/joc.1800
Solman, S. A. (2013). Regional climate modeling over South America: a review. Adv. Meteorol. 2013 (1), 504357.
Solman, S. A., and Blázquez, J. (2019). Multiscale precipitation variability over South America: analysis of the added value of CORDEX RCM simulations. Clim. Dyn. 53, 1547–1565. doi:10.1007/s00382-019-04689-1
Staal, A., Tuinenburg, O. A., Bosmans, J. H. C., Holmgren, M., van Nes, E. H., Scheffer, M., et al. (2018). Forest-rainfall cascades buffer against drought across the Amazon. Nat. Clim. Chang. 8, 539–543. doi:10.1038/s41558-018-0177-y
Steinhoff, D. F., Monaghan, A. J., and Clark, M. P. (2015). Projected impact of twenty-first century ENSO changes on rainfall over Central America and northwest South America from CMIP5 AOGCMs. Clim. Dyn. 44 (5), 1329–1349. doi:10.1007/s00382-014-2196-3
Sun, X., and Barros, A. P. (2015a). Impact of Amazonian evapotranspiration on moisture transport and convection along the eastern flanks of the tropical Andes. Quart. J. R. Meteorol. Soc. 141, 3325–3343. doi:10.1002/qj.2615
Sun, X., and Barros, A. P. (2015b). Isolating the role of surface evapotranspiration on moist convection along the eastern flanks of the tropical andes using a quasi-idealized approach. J. Atmos. Sci. 72, 243–261. doi:10.1175/JAS-D-14-0048.1
Thaler, V., Loikith, P. C., Mechoso, C. R., and Pampuch, L. A. (2021). A multivariate assessment of climate change projections over South America using the fifth phase of the Coupled Model Intercomparison Project. Int. J. Climatol. 41, 4265–4282. doi:10.1002/joc.7072
Tokarska, K. B., Stolpe, M. B., Sippel, S., Fischer, E. M., Smith, C. J., Lehner, F., et al. (2020). Past warming trend constrains future warming in CMIP6 models. Sci. Adv. 6 (12), eaaz9549. doi:10.1126/sciadv.aaz9549
Toledo, O., Palazzi, E., Cely Toro, I. M., and Mortarini, L. (2021). Comparison of elevation-dependent warming and its drivers in the tropical and subtropical Andes. Clim. Dyn. 58, 3057–3074. doi:10.1007/s00382-021-06081-4
Torma, C., Giorgi, F., and Coppola, E. (2015). Added value of regional climate modeling over areas characterized by complex terrain: precipitation over the Alps. J. Geophys. Res. Atmos. 120 (9), 3957–3972. doi:10.1002/2014JD022781
Torres-Alavez, J. A., Das, S., Corrales-Suastegui, A., Coppola, E., Giorgi, F., Raffaele, F., et al. (2021). Future projections in the climatology of global low-level jets from CORDEX-CORE simulations. Clim. Dyn. 57, 1551–1569. doi:10.1007/s00382-021-05671-6
Tovar, C., Carril, A. F., Gutiérrez, A. G., Ahrends, A., Fita, L., Zaninelli, P., et al. (2022). Understanding climate change impacts on biome and plant distributions in the Andes: challenges and opportunities. J. Biogeogr. 00, 1420–1442. doi:10.1111/jbi.14389
Trachte, K., Rollenbeck, R., and Bendix, J. (2010). Nocturnal convective cloud formation under clear sky conditions at the eastern Andes of south Ecuador. J. Geophys. Res. Atmos. 115 (D24). doi:10.1029/2010jd014146
Trachte, K., Seidel, J., Figueroa, R., Otto, M., and Bendix, J. (2018). Cross-Scale precipitation variability in a semiarid catchment area on the western slopes of the central Andes. J. Appl. Meteorol. Climatol. 57 (3), 675–694. doi:10.1175/jamc-d-17-0207.1
UN (2015). Transforming our world: the 2030 Agenda for sustainable development. Available at: https://sdgs.un.org/publications/transforming-our-world-2030-agenda-sustainable-development-17981.
UNDRR (2015). Sendai framework for disaster Risk reduction 2015-2030, united nations Office for disaster Risk reduction. Available at: https://www.undrr.org/publication/sendai-framework-disaster-risk-reduction-2015-2030.
Urrego-Ortiz, J., Martínez, J. A., Arias, P. A., and Jaramillo-Duque, Á. (2019). Assessment and day-ahead forecasting of hourly solar radiation in medellín, Colombia. Energies 12 (22), 4402. doi:10.3390/en12224402
van Vuuren, D. P., Edmonds, J., Kainuma, M., Riahi, K., Thomson, A., Hibbard, K., et al. (2011). The representative concentration pathways: an overview. Clim. Change 109 (1), 5–31. doi:10.1007/s10584-011-0148-z
Vera, C., Baez, J., Douglas, M., Emmanuel, C. B., Marengo, J., Meitin, J., et al. (2006). The South American low-level jet experiment. Bull. Am. Meteorological Soc. 87 (1), 63–78. doi:10.1175/bams-87-1-63
Villarroel, C., Carrasco, J., Casassa, G., and Falvey, M. (2013). Modeling near-surface air temperature and precipitation using WRF with 5-km resolution in the Northern Patagonia Icefield: a pilot simulation. Int. J. Geosci. 4 (8), 1193–1199.
Vuille, M., Francou, B., Wagnon, P., Juen, I., Kaser, G., Mark, B. G., et al. (2008). Climate change and tropical Andean glaciers: past, present and future. Earth Sci. Rev. 89, 79–96. doi:10.1016/j.earscirev.2008.04.002
Wagnon, P., Sicart, J. E., Berthier, E., and Chazarin, J. P.(2003). Wintertime high-altitude surface energy balance of a Bolivian glacier, Illimani, 6340 m above sea level. J. Geophy. Res. Atmos. 108(D6).
WMO (2022). Early warnings for all the UN global early warning initiative for the implementation of climate adaptation executive action plan 2023-2027. Available at: https://library.wmo.int/records/item/58209-early-warnings-for-all.
Wongchuig, S., Espinoza, J. C., Condom, T., Segura, H., Ronchail, J., Arias, P. A., et al. (2021). A regional view of the linkages between hydro-climatic changes and deforestation in the Southern Amazon. Int. J. Climatol. 42, 3757–3775. doi:10.1002/joc.7443
Wongchuig, S., Paiva, R., Siqueira, V., Papa, F., Fleischmann, A., Biancamaria, S., et al. (2024). Multi satellite data assimilation for large scale hydrological hydrodynamic prediction: proof of concept in the Amazon basin. Water Resour. Res. 60 (8), e2024WR037155. doi:10.1029/2024wr037155
Yepes, J., Mejía, J. F., Mapes, B., and Poveda, G. (2020). Gravity waves and other mechanisms modulating the diurnal precipitation over one of the rainiest spots on Earth: observations and Simulations in 2016. Mon. Weather Rev. 148 (9), 3933–3950. doi:10.1175/mwr-d-19-0405.1
Keywords: Andes, atmospheric modeling, climate projections, kilometer-scale modeling, hydroclimate
Citation: Junquas C, Martinez JA, Bozkurt D, Viale M, Fita L, Trachte K, Campozano L, Arias PA, Boisier JP, Condom T, Goubanova K, Pabón-Caicedo JD, Poveda G, Solman SA, Sörensson AA and Espinoza JC (2024) Recent progress in atmospheric modeling over the Andes – part II: projected changes and modeling challenges. Front. Earth Sci. 12:1427837. doi: 10.3389/feart.2024.1427837
Received: 04 May 2024; Accepted: 05 September 2024;
Published: 11 November 2024.
Edited by:
Bian He, Chinese Academy of Sciences (CAS), ChinaReviewed by:
Eduardo Zorita, Helmholtz Centre for Materials and Coastal Research (HZG), GermanyVanderlei Vargas Jr., Cooperative Institute for Research in the Atmosphere (CIRA), United States
Copyright © 2024 Junquas, Martinez, Bozkurt, Viale, Fita, Trachte, Campozano, Arias, Boisier, Condom, Goubanova, Pabón-Caicedo, Poveda, Solman, Sörensson and Espinoza. This is an open-access article distributed under the terms of the Creative Commons Attribution License (CC BY). The use, distribution or reproduction in other forums is permitted, provided the original author(s) and the copyright owner(s) are credited and that the original publication in this journal is cited, in accordance with accepted academic practice. No use, distribution or reproduction is permitted which does not comply with these terms.
*Correspondence: C. Junquas, Y2xlbWVudGluZS5qdW5xdWFzQGlyZC5mcg==
†These authors have contributed equally to this work and share first authorship