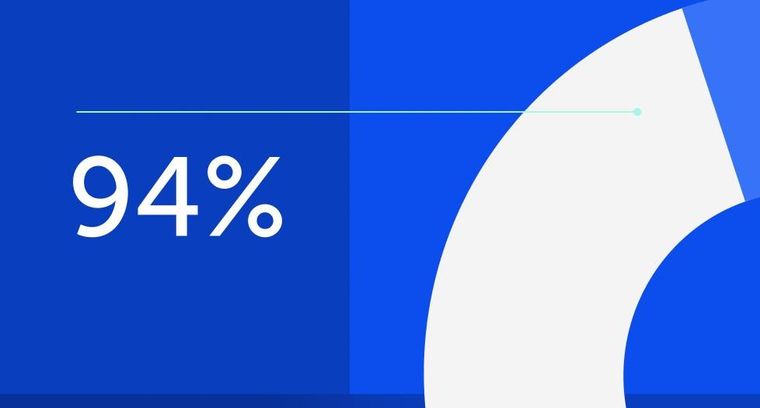
94% of researchers rate our articles as excellent or good
Learn more about the work of our research integrity team to safeguard the quality of each article we publish.
Find out more
ORIGINAL RESEARCH article
Front. Earth Sci., 10 July 2024
Sec. Georeservoirs
Volume 12 - 2024 | https://doi.org/10.3389/feart.2024.1416824
In order to solve the problems of conventional water injection development difficulties and low recovery factor in low-permeability reservoirs, the method of high-pressure air drive is adopted to achieve the purpose of reservoir energy enhancement and efficiency improvement. This paper conducted an experimental study on the mechanism of low-temperature oxidation (LTO) for crude oil in the process of high-pressure air flooding, elaborated the relationship between the LTO properties of crude oil and the temperature, pressure, and water saturation of the reservoir, and analyzed the differences in LTO oxygen consumption and oil components under different reaction conditions. In addition, combined with the air flooding physical simulation experiment, the dynamic evolution law of recovery rate in the air flooding process was revealed. Findings from this inquiry indicate that an escalation in the oxidation temperature significantly amplifies the oxygen incorporation reaction within the crude oil matrix. This augmentation in oxidative conditions leads to an uptick in oxygen consumption, which subsequently precipitates a reduction in the lighter fractions of the oxidized oil while enriching its heavier components. Elevated pressures were found to enhance the propensity for the amalgamation of unstable hydrocarbons with oxygen, fostering comprehensive and heterogeneous oxidation reactions. Notably, an excessive presence of water was observed to detrimentally affect the thermal efficacy of crude oil oxidation processes. In the context of low-permeability reservoirs, air injection techniques have emerged as superior in effectuating oil displacement, although an increase in injection pressures has been associated with the phenomenon of gas channeling. Interestingly, adopting a sequential strategy of initiating water flooding before air flooding facilitated the conveyance of high-pressure air via established flushing channels, although it appeared to attenuate the intensity of crude oil oxidation, culminating in an oil recovery efficiency peaking at 51%.
In recent years, along with the progress in exploration and utilization , the proportion of domestic newly proved reservoirs classified as low-permeability, extra-low-permeability, and tight oil reservoirs has significantly increased. Consequently, the challenges of using undeveloped reservoirs have also grown constantly, leading to higher technical demands for their development (Mokhtari et al., 2022; Gao et al., 2023; Liu et al., 2023; Shi et al., 2023; Zhang et al., 2023). Extra-low permeability and tight reservoirs can be hydraulically fractured to induce fracture expansion and modify the reservoir, thereby increasing flow pathways and improving seepage capacity (Cao et al., 2024a; Cao et al., 2024b; Jia et al., 2024). The conventional reservoir adopted the water injection mining method for low-permeability reservoirs; however, widespread problems such as “injecting not, extracting not” have emerged. Enhancing recovery effectiveness in low-permeability reservoirs has thus become an emergency issue. Research demonstrated that gas injection could notably increase the effectiveness of low-permeability reservoir exploitation because gas molecules were compressible, buckling, and expanding with greater elastic energy, thus supplementing the energy of the formation and enhancing recovery (Wang et al., 2021; Matkivskyi, 2022; Zeng et al., 2022; Liao et al., 2023). Air offered advantages such as a lower financial burden and stabilized components compared to other gas media. Hence, air injection exploitation held major significance for the conversion of low-permeability reservoir reserves into yield and profitability (Nevmerzhitskiy, 2022; Zhang and Hao, 2022; Gu et al., 2023).
Aiming at the oxidation mechanism of reservoir air injection exploitation and recovery enhancement, both domestic and foreign experts and scholars have carried out widespread research. Bastow et al. (2003) investigated the effect of air on alkyl phenols in crude oil, where prolonged exposure to air resulted in crude oil oxidation and affected the distribution of alkyl phenols. Gundogar and Kok (2014) considered that the heat release during the oxidation process was greater when the content of asphaltenes in crude oil was higher. Atmospheric oxygen facilitated the conversion of the light hydrocarbon components of crude oil to higher molecular hydrocarbons in the presence of a catalyst (Nosova et al., 2018). Cheng et al. (2023) modified the oxidation numerical model by incorporating the geological characteristics of low-permeability reservoirs. CMG STARS was adopted to investigate the impacts of formation permeability, temperature, gas injection rate, and bottom-hole pressure on air flooding. Verşan Kök et al. (2017) and Kok et al. (2020) examined oxidation reaction regulations in both light and heavy oils using a thermogravimetric analyzer and differential scanning calorimeter; they believed that the oxidation phase was divided into two steps, namely low-temperature oxidation (LTO) and high-temperature oxidation (HTO) reactions, with LTO being a multistep sequential reaction. Amine Ifticene et al. (2022) investigated the combustion and transformation behavior of asphaltenes, caseinates, and oil shale in an airy atmosphere, as well as the kinetic mechanisms. Ren et al. (2002) explored the oxygen consumption potential, reaction rate, and reaction pathway of the LTO reaction, which depended on the nature of oil and oxygen concentration at low oil saturation and low oxygen partial pressure. Liao et al. (2020) revealed the features of the crude oil oxidation reaction in the whole temperature domain of air injection from 30°C to 600°C through simulation experiments, classified the crude oil oxidation reaction with air injection into four temperature intervals, and summarized the oxidation reaction mechanism among various temperature intervals. Yuan et al. (2021); Yuan et al. (2022) presented the discrepancies and linkages among the various crude oil composition oxidation processes developed by air injection, addressed the capability of distinct categories of catalysts to catalyze intensified crude oil combustion, and elaborated on the dilemmas and strategies associated with the challenges involved in air injection (Ariskina et al., 2020). The mechanism of high-pressure air drive development was complicated; the majority of current studies are based on the crude oil oxidation thermodynamic model and the related mechanism research is not perfect. At the same time, most of the scholars tend to focus on theoretical research that is not combined with actual field applications; they rely on physical simulations that are less in line with the actual mining conditions, thereby limiting their ability to guide field production and development.
Furthermore, this study examined the effect of reservoir temperature, pressure, and water saturation on the LTO properties through an oil oxidation test. Considering the actual reservoir environment, long-core physical simulation laboratory experiments were applied to evaluate the characteristics of air flooding and subsequent water flooding after air flooding for oil recovery at various injection pressures. High-pressure air injection development is of great significance for both reserve conversion and the development of low-permeability reservoirs. The results of the research provide the basic theoretical evidence for the economic and efficient development of low-permeability reservoirs by injecting high-pressure air and provide technical support for the development of low-permeability oilfield mines.
The crude oil was obtained as degassed oil from the Xinjiang oilfield, following the treatment procedure stipulated in the industry standard SY/T 6316–1997 “Methods for analyzing fluid properties in heavy oil reservoirs: determination of crude oil viscosity.” The samples were then dewatered and de-admixed. The oil viscosity was 57 mPa s after treatment, and the experimental core was an artificial low-permeability sandstone core, with core data parameters listed in Table 1 for various testing groups. The experimental gas used was high-pressure compressed air (purity 99.99%) containing 20.974% oxygen.
In accordance with various ions in the oilfield water inspection report, the formation water was prepared in the laboratory. Its total mineralization was 12,396.02 mg/L, pH value was 8.02, and density was 1.0178 g/cm3. Based on Surin’s classification, this formation water belonged to the sodium bicarbonate water type. The details of various ions and water-soluble salt levels of this formation water are provided in Table 2.
The experimental apparatus comprised a high-temperature and high-pressure reactor, a DGM-Ⅲ multi-function core replacement apparatus (Chengdu Core Science and Technology Co., Ltd.), an Agilent 6890 Gas Chromatograph (GC), an Agilent 7890B GC, an FB-45/7 oil-free air compressor, a heating device, and an ES-100A constant-speed constant-pressure pump.
The laboratory equipment consists of a reactor, air compressor, pressure and temperature detection device, gas chromatograph, and liquid chromatograph. The reactor capacity was 200 mL, with temperature resistance at 400°C and pressure resistance at 70 MPa. We investigated the influence of various temperatures, pressures, and water saturations on the oxidation properties at low temperatures of crude oil. The experimental procedure involved the following: first, the device tightness was inspected, the crude oil was incorporated into the reactor, and the reactor was filled with air up to the reservoir pressure of 24 MPa. Subsequently, the reactor was inserted into a heating device, with the experimental temperatures (60°C, 80°C, 100°C, and 120°C) set to perform LTO experiments. The oxidation period was 7 days, during which the gas and liquid phases of the oxidation reaction were characterized by chromatographic analysis. In agreement with the previous oxidation experiments, the reservoir temperature was set at 80°C, the experimental pressure was altered (16 MPa, 24 MPa, 32 MPa, and 40 MPa), and the experiments were repeated. Finally, the reservoir pressure and temperature were kept constant, the crude oil water saturation was varied (0, 20%, 40%, 60%, and 80%), and the experiment was repeated, keeping the oil–water volume sum at 40 mL.
Long cores were used to carry out air flooding experiments aimed at the target blocks to investigate oil recovery during air flooding, followed by water flooding at varying displaced pressures. The laboratory procedure is shown in Figure 1. The long cores were evacuated and saturated with formation water. Then, the formation water was driven off with crude oil up to the saturated oil phase, thus calculating the bound water saturation and saturated oil content. The above steps were repeated to gain six groups of laboratory long cores, whose physical parameters are listed in Table 1. Maintaining the experimental temperature at 80°C and adopting air displacement with constant injection pressure (16, 24, and 32 MPa), respectively, the pressures, injection volumes, and oil and gas production of the injection end and exit section of cores #1, #2, and #3 were measured. Cores #4, #5, and #6 were initially water-flooded, followed by air flooding until the economic limit was reached, using the same injection pressure gradients (16 MPa, 24 MPa, and 32 MPa) as before.
Figure 2 shows the details of the variation in oxygen content and oxygen consumption following oxidized oil at various pressures. In contrast, the oxygen consumed in crude oil oxidation occurred more rapidly with increasing oxidizing pressure, while the oxygen content of the output gas was lowered. Owing to increasing oxidation pressure, the air solubility of crude oil increased, and unstable hydrocarbons within crude oil contacted more oxygen inside the air to undergo the LTO reaction (Zhao et al., 2022; 2023). Following oxidation time addition, the depth of the oxidation reaction was intensified further, thus more oxygen was depleted.
Figure 2. Variation in oxygen content and oxygen consumption of crude oil upon oxidation at various pressures. (A) Oxygen content and (B) oxygen consumption.
Fractional transformation in crude oil upon oxidation at various pressures for 7 days and the percentage content of different carbon number intervals are presented in Figure 3. Owing to the fact that the hydrocarbon composition in crude oil underwent an LTO reaction upon exposure to oxygen in the air, a variety of oxygen-containing derivatives were formed. However, hydrocarbons with varied carbon numbers in crude oil possessed distinct oxidation activeness, and therefore, C5–C35 was partitioned into four intervals. Following oxidation at 40 MPa, compared to crude oil, the C5–C14 amount decreased by 46.23%, the C15–C20 amount increased by 15.77%, C21–C28 amount increased by 41.14%, and >C28 amount increased by 101.01%. The oxygen addition reaction in crude oil was intensified with higher oxidation pressure. The activated hydrogen was substituted with oxygen atoms, generating oxygen-containing derivatives that would further experience the aromatization, condensation, and polycondensation processes to form macromolecules with higher condensation degrees.
The LTO period of crude oil mainly involved two main categories of reactions (Zhao et al., 2019; Pu et al., 2020): oxy-addition reaction to form hydroperoxides and isomerization and decomposition with hydroperoxides to produce peroxides such as ketones, alcohols and aldehydes, CO, CO2, and H2O. The variations in the oxygen content and oxygen consumption of the crude oil oxidized at varied temperatures are illustrated in Figure 4.
Figure 4. Variation in oxygen content and oxygen consumption of crude oil upon oxidation at various temperatures. (A) Oxygen content and (B) oxygen consumption.
It was observed that the volume of oxygen consumption of crude oil during oxidation increased at elevated oxidation temperatures. The oxygen consumption during oxidation was only 1.724% at 60°C, indicating that the oxy-addition reaction was weak. The oxygen content of residual gas upon crude oil oxidation for 7 days was 17.664%, with oxygen consumption of 3.31% as the oxidation temperature reached 120°C. Practically, along with the warming of the oxidation temperature and higher thermal energy offered by external sources, the Brownian motion of oxygen molecules within the gas accelerated, which easily collided and integrated with the reactive components of crude oil susceptible to reaction, thus facilitating the process of the oxygen addition reaction and intensifying the oxidation degree. The crude oil oxidized at low temperatures would produce peroxides, with the oxygen consumption speed growing; hence, the corresponding oxygen consumption would be substantially enlarged.
As evidenced in Figure 5, the lighter components with lower carbon numbers exhibited a decreasing trend with increasing oxidation temperatures in comparison with crude oil, while the medium and heavy components with higher carbon numbers tended to increase. The component carbon numbers C5–C35 were categorized into four regions to better reflect the temperature effect on oxidized properties within the oil samples. In contrast to the original oil compositions, C5–C14 of oil samples oxidized at 60°C was reduced by 4.25%. The C5–C14 content of the oxidized oil samples decreased by 42.52% upon the 120°C oxidation temperature, while the C21∼C28 and >C28 contents increased by 36.53% and 68.36%, respectively, at this point. With increasing visible oxidation temperature, the oxidative addition reaction of the crude oil intensified; a majority of light components (C5–C14) converted into heavy components (C21 ∼ C28 and > C28), while the molecular interactions among the resin and asphaltene in heavy components formed a spatial mesh structure, which explained the increase in the viscosity and density of oxidized oil (Jin et al., 2022; Akande et al., 2023; Zhai et al., 2023).
Discrepancies in the water saturation of various reservoirs might contribute to alterations in the oxidation properties of crude oil. As illustrated in Figure 6, the oxygen content of the output gas following the oxidation of crude oil increased as water saturation increased, reducing oxygen demand in turn. The oxygen content increased from 18.09% to 20.466% after 7 days of oxidation, with a corresponding reduction in oxygen consumed by 2.38% upon water saturation increasing up to 80% from 0. The volume of oxygen consumed by crude oil during the oxidation reaction decreased in response to higher water saturation, accompanied by a corresponding decrease in oil saturation. Verşan Kök et al. (2021) and Kok et al. (2022) concluded that the oxidation reaction with crude oil was generally characterized by three phases: LTO phase (<350°C), FD phase (350°C–400°C), and HTO phase (>400°C). Distillation and evaporation of light components within the crude oil, as well as oxygen atom addition reactions, normally occur during the LTO stage. The oxygenation reactions involved the generation of several hydrocarbon derivatives, including alcohols, aldehydes, ketones, acids, and peroxides, among others. Extremely little CO2 was produced over the period; therefore, the gas chromatographic analysis did not reveal any noticeable alteration in CO2.
Figure 6. Variation in oxygen content and oxygen consumption of crude oil upon oxidation at various water saturation values. (A) Oxygen content and (B) oxygen consumption.
The percentage content of light components increased after the oil oxidation reaction, as illustrated in Figure 7; meanwhile, the medium and heavy composition percentage contents declined. The components C5–C35 were partitioned into four regions depending on the oxidation activity. The oxidized oil samples with 80% water saturation exhibited an increase of 46.92% in C5–C14 compared with 0 water saturation, a decrease of 12.96% in C15–C20, a decrease of 22.92% in C21–C28, and a decrease of 34.41% in >C28. This explains why higher water saturation is unfavorable to crude oil oxidation. Contrary to the temperature effect on oxidation characteristics in crude oil, crude oil oxidation would become weaker along with the increase in water saturation, attributed to the fact that the water-containing situation would affect the thermal efficiency generated by crude oil oxidation, which is detrimental to heat diffusion, while heat loss was serious.
The variation in oil recovery with various gas injection pressures is depicted in Figure 8. The ultimate oil recovery of the long core increased from 37.1% to 40.2% as gas injection pressure increased from 16 to 32 MPa. Owing to greater gas mobility in the pores and throats, as well as viscosity discrepancies in gas and crude oil, sufficient initial contact of air with crude oil in the form of small discontinuous bubbles occurs, dissolving more air into crude oil and enabling crude oil expansion and elastic energy. The unstable hydrocarbons of crude oil interact with the oxygen of air in the non-homogeneous LTO reaction (Li et al., 2017; Li et al., 2020; Chen et al., 2018; 2019; Zhao et al., 2024); thus, heat generated by the oxidation reaction could lower crude oil viscosity. On one hand, a portion of the produced CO2 could dissolve into the oil, resulting in reduced viscosity. On the other hand, the flue gas, formed together with CO, N2, and volatilized light hydrocarbon components, contributes to further displacing crude oil. Gas pressurization plays a dominant role in boosting the formation energy and elasticity energy of oil dissolved in air. The post-experimental permeability of different experimental groups of cores is shown in Table 3. The permeability of experimental groups of cores was unchanged during air flooding; the process of air flooding would change crude oil properties in contact with air, and a variety of mechanisms would drive the crude oil out of the core pore space.
With further expansion of air injection, the gas breakthrough accelerated to form gas channeling. Oil recovery grew slower following gas scrambling, at which time it was mostly the high-speed carrying function of high-pressure air that allowed the residual oil in the gas flow channel to be extracted. As illustrated in Figure 9, various injection pressures correspond to different gas channeling points. The higher the injection pressure, the earlier the gas flushing occurred, which would lead to a worse development effect. Meanwhile, the gas–oil ratios of the experimental tests were similar when the gas channeling occurred. With increased gas injection pressure, crude oil on the pore wall was stripped off, enlarging the space for gas and oil flow and reducing resistance, which increased the likelihood of gas channeling occurring sooner, corresponding to smaller injection PV. The gas–oil ratio increased rapidly after gas channeling. After the occurrence of gas channeling, it mainly depended on the high-speed carrying effect of high-pressure air to drive out the residual oil in the gas flow channel, and the growth of the recovery factor was slow, which affected the development effect of the reservoir. Therefore, the gas injection parameters could be optimized in the practical production of the oilfield to improve the reservoir development effect.
In the case of low-permeability reservoirs, the characteristics of small pore throat scale, poor permeability performance, non-Darcy seepage effect of liquids, and serious microscopic non-homogeneity contribute to the difficulty of water injection to establish an effective replacement pressure system, as well as the still large amount of residual oil requiring appropriate successor extraction technology after water flooding. As shown in Figure 10, the injection PV counts sequentially enlarge in accordance with injection pressure decline, while water flooding reaches the economic limit, and the water flooding oil recovery could achieve 41.2% at most. Since more water entered into the tiny pores to replace crude oil during high injection pressure, in addition to the “finger-in and bypass phenomenon” that occurred in advance, the water content increased rapidly upon water sighting at the extraction side, corresponding to the smaller number of injected PV. During subsequent air flooding, the water flow is greater, and the dissolution and expansion effect is stronger; thus, the air injection could continue to drive off the residual oil in the core. Yet, residual oil following water flooding was dispersed among the tiny pores, while crude oil suffered from insufficient contact oxidation time during the replacement process, resulting in poor oxidizing action of the air upon crude oil. As a result, once the airflow channel formed in the large pore throat, high-speed airflow was transported directly along the channel, unable to adequately replace residual oil in the tiny pores, causing a rapid increase in the gas production rate and a limited increase in oil expulsion efficiency, thereby reducing the oil expulsion efficiency of air flooding to less than 10%.
Figure 10. Variation in oil recovery water flooding subsequent air flooding from cores #4, #5, and #6 at various injection pressures.
Distinct from the general gas displacement process, the oxygen in the air during air injection would undergo the LTO reaction with crude oil, resulting in a series of property changes such as crude oil viscosity, density, and composition, thereby affecting the final oil recovery factor. In accordance with the theory of the oxidation reaction, crude oil mainly reacted with oxidative addition at temperatures lower than 150°C. Oxygen atoms were attached to the molecular chains of liquid hydrocarbons through free radical reactions, generating oxidized derivatives such as peroxides, ketones, alcohols, aldehydes, and acids, causing a tendency to increase the viscosity of the oil samples. Furthermore, the more pronounced the oxidative addition reaction, the greater the increase in viscosity of the oil sample. Therefore, by analyzing the viscosity change of the produced oil samples, we can reflect the LTO process of the oil samples to some extent. Figure 11 depicts the variation in produced oil viscosity during the air drive process. Upon increasing the amount of injected air, more air would be dissolved in the crude oil. There are increased opportunities for the LTO reaction of crude oil when the contact time between air and crude oil becomes longer. As observed in the figure, the viscosity increase of the produced oil was not clear with increased air injection, indicating that the LTO process of oil during subsequent air flooding was quite weak.
Figure 12 illustrates the results for the comparison of produced oil components with varying injection pressures. As shown in the figure, the variation in produced oil in C5–C14, C15–C20, C21–C28, and >C28 fractions under air flooding at different injection pressures was relatively minor. Particularly, the produced oil fraction content at low pressure (16 MPa) varied by less than 2% for each hydrocarbon component. In contrast, C5–C14 of the produced oil samples under high pressure (36 MPa) showed a slight decline compared with the initial oil samples, while C15–C20 appeared to increase a bit. It was indicated that the crude oil under high-pressure air flooding caused mild oxidation, resulting in a change in crude oil hydrocarbons toward an increased carbon number. Nevertheless, given the weak changes in the produced oil viscosity, it suggested that the LTO process of crude oil during the subsequent air injection is rather feeble.
In the process of crude oil injection air oxidation, not only would the oil phase undergo a series of alterations due to oxidation, such as composition, viscosity, density, and other physical parameters, but the gas phase would also be oxidized, resulting in a downward trend of oxygen concentration. Distillation extraction and decarboxylation reactions could generate a specific concentration of hydrocarbons, CO2, CO, and other gases in the gas phase. Table 4 shows the composition of the produced gas at various injection pressures. Since crude oil was composed of more light hydrocarbons, during the high-temperature air flooding process, air dissolved in the crude oil distills and extracts some of the light hydrocarbons from the oil phase into the gas phase. As a result, the produced gas contains a few components of C1–C5 hydrocarbons (<0.1%). In addition, there was no CO2 and CO generation in the subsequent air drive process, and the residual oxygen amount was above 20.5%. All the above indicated that the oil LTO process in the process of air flooding was so feeble that it could not perform the oil displacement mechanism of flue gas flooding formed by air injection and oxidation for crude oil. In addition, we should pay attention to monitoring the variation in the oxygen content of the produced gas in the field application of oilfield air flooding, to avoid the oxygen not being effectively consumed and mixed with natural gas in the wellbore or surface pipeline, which may lead to safety accidents.
Table 4. Composition of produced gas at various injection pressures during water flooding subsequent to air flooding.
This research focused on the characteristics of crude oil LTO during high-pressure air flooding in low-permeability reservoirs and the evolution mechanism of the development effect of air flooding. Using static oxidation and physical simulation experiments, the following conclusions were obtained:
(1) Elevated oxidation temperatures exacerbated the LTO reactions of crude oil, leading to increased formation of oxygenated derivatives undergoing aromatization, condensation, and polycondensation reactions, resulting in more highly condensed macromolecules. The content of heavy fractions (C21–C28 and >C28) increased. Oxygen consumption during the LTO process increased under high-pressure conditions. High water saturation was unfavorable to the thermal effect and heat diffusion generated by crude oil LTO.
(2) By increasing the air injection pressure to 26 MPa, the air flooding recovery factor of the long core reached up to 41.2%. The recovery factor can be boosted to the economic limit by switching to air flooding after water flooding. The final recovery factor reached 50.92% due to the weak effect of LTO and the influence of gas flushing.
(3) Injection pressure was identified as one of the influencing factors in gas flushing, requiring optimization of the gas injection parameters to avoid premature gas flushing. In actual development, it was necessary to block the gas flow channels to improve the wave efficiency. At the same time, considering the safety of the field, oxygen-reduced air flooding development could be selected to achieve economic efficiency and safety goals.
The original contributions presented in the study are included in the article/Supplementary Material; further inquiries can be directed to the corresponding author.
XC: conceptualization, writing–original draft, and writing–review and editing. ZB: investigation, software, and writing–review and editing. ZL: methodology, project administration, and writing–review and editing. YG: data curation, methodology, and writing–review and editing. YZ: supervision, validation, and writing–review and editing. XL: formal analysis, methodology, project administration, and writing–review and editing.
The author(s) declare that no financial support was received for the research, authorship, and/or publication of this article.
Authors XC, ZB, ZL, YG, and YZ were employed by the Exploration and Development Research Institute of Xinjiang Oilfield Company.
The remaining author declares that the research was conducted in the absence of any commercial or financial relationships that could be construed as a potential conflict of interest.
All claims expressed in this article are solely those of the authors and do not necessarily represent those of their affiliated organizations, or those of the publisher, the editors, and the reviewers. Any product that may be evaluated in this article, or claim that may be made by its manufacturer, is not guaranteed or endorsed by the publisher.
Akande, I., Bridgwater, T., van Koningsbruggen, P. J., and Yuan, Q. (2023). A viscosity study of charcoal-based nanofluids towards enhanced oil recovery. J. Mol. Liq. 387, 122615. doi:10.1016/j.molliq.2023.122615
Amine Ifticene, M., Yuan, C., Al-Muntaser, A. A., Onishchenko, Y. V., Emelianov, D. A., and Varfolomeev, M. A. (2022). Behavior and kinetics of the conversion/combustion of oil shale and its components under air condition. Fuel 324, 124597. doi:10.1016/j.fuel.2022.124597
Ariskina, K. A., Yuan, C., Abaas, M., Emelianov, D. A., Rodionov, N., and Varfolomeev, M. A. (2020). Catalytic effect of clay rocks as natural catalysts on the combustion of heavy oil. Appl. Clay Sci. 193, 105662. doi:10.1016/j.clay.2020.105662
Bastow, T. P., van Aarssen, B. G. K., Herman, R., Alexander, R., and Kagi, R. I. (2003). The effect of oxidation on the distribution of alkylphenols in crude oils. Org. Geochem. 34, 1103–1111. doi:10.1016/S0146-6380(03)00067-6
Cao, Z., Wang, P., Li, Z., and Du, F. (2024a). Migration mechanism of grouting slurry and permeability reduction in mining fractured rock mass. Sci. Rep. 14, 3446. doi:10.1038/s41598-024-51557-y
Cao, Z., Yang, X., Li, Z., Huang, C., Du, F., Wang, W., et al. (2024b). Fracture propagation and pore pressure evolution characteristics induced by hydraulic and pneumatic fracturing of coal. Sci. Rep. 14, 9992. doi:10.1038/s41598-024-60873-2
Chen, Y., Pu, W., Li, Y., Liu, X., Jin, F., Hui, J., et al. (2018). Novel insight into the viscosity-temperature characteristic by the comparison of tahe ordinary- and ultra- heavy oils. Energy fuels. 32, 12308–12318. doi:10.1021/acs.energyfuels.8b03091
Chen, Y., Pu, W., Liu, X., Li, Y., Gong, X., Hui, J., et al. (2019). Specific kinetic triplet estimation of Tahe heavy oil oxidation reaction based on non-isothermal kinetic results. Fuel 242, 545–552. doi:10.1016/j.fuel.2019.01.097
Cheng, Z., Wang, T., Bai, Z., Wang, L., Yuan, C., Zhao, Z., et al. (2023). A numerical simulation investigation on low permeability reservoirs air flooding: oxidation reaction models and factors. Geoenergy Sci. Eng. 223, 211506. doi:10.1016/j.geoen.2023.211506
Gao, M.-W., Zhang, M.-S., Du, H.-Y., Zhao, M.-W., Dai, C.-L., You, Q., et al. (2023). A novel triple responsive smart fluid for tight oil fracturing-oil expulsion integration. Pet. Sci. 20, 982–992. doi:10.1016/j.petsci.2023.01.008
Gu, Z., Lu, T., Li, Z., Li, B., Du, L., and Zhang, C. (2023). Analysis on the mechanism and characteristics of nanofluid imbibition in low permeability sandstone core pore surface: application in reservoir development engineering. Colloids Surf. Physicochem. Eng. Asp. 659, 130774. doi:10.1016/j.colsurfa.2022.130774
Gundogar, A. S., and Kok, M. V. (2014). Thermal characterization, combustion and kinetics of different origin crude oils. Fuel 123, 59–65. doi:10.1016/j.fuel.2014.01.058
Jia, Y., Cao, Z., Li, Z., Du, F., Huang, C., Lin, H., et al. (2024). Nonlinear evolution characteristics and seepage mechanical model of fluids in broken rock mass based on the bifurcation theory. Sci. Rep. 14, 10982. doi:10.1038/s41598-024-61968-6
Jin, F., Jiang, T., Yuan, C., Varfolomeev, M. A., Wan, F., Zheng, Y., et al. (2022). An improved viscosity prediction model of extra heavy oil for high temperature and high pressure. Fuel 319, 123852. doi:10.1016/j.fuel.2022.123852
Kok, M. V., Varfolomeev, M. A., and Nurgaliev, D. K. (2020). Low-temperature oxidation reactions of crude oils using TGA–DSC techniques. J. Therm. Anal. Calorim. 141, 775–781. doi:10.1007/s10973-019-09066-y
Kok, M. V., Varfolomeev, M. A., Nurgaliev, D. K., and Kandasamy, J. (2022). Application of TGA-MS technique for oil shale characterization and kinetics. J. Therm. Anal. Calorim. 147, 10767–10774. doi:10.1007/s10973-022-11210-0
Li, Y.-B., Chen, Y., Pu, W.-F., Gao, H., and Bai, B. (2017). Experimental investigation into the oxidative characteristics of Tahe heavy crude oil. Fuel 209, 194–202. doi:10.1016/j.fuel.2017.07.029
Li, Y.-B., Luo, C., Lin, X., Li, K., Xiao, Z.-R., Wang, Z.-Q., et al. (2020). Characteristics and properties of coke formed by low-temperature oxidation and thermal pyrolysis during in situ combustion. Ind. Eng. Chem. Res. 59, 2171–2180. doi:10.1021/acs.iecr.9b05635
Liao, G., Wang, H., Wang, Z., Tang, J., Wang, B., Pan, J., et al. (2020). Oil oxidation in the whole temperature regions during oil reservoir air injection and development methods. Pet. Explor. Dev. 47, 357–364. doi:10.1016/S1876-3804(20)60052-0
Liao, H., Pan, W., He, Y., Fang, X., and Zhang, Y. (2023). Study on the mechanism of CO2 injection to improve tight sandstone gas recovery. Energy Rep. 9, 645–656. doi:10.1016/j.egyr.2022.11.210
Liu, G., Jiang, F., Ge, L., Zhang, Q., Chen, X., Fan, Z., et al. (2023). Investigation of salinity and ion effects on low salinity water flooding efficiency in a tight sandstone reservoir. Energy Rep. 9, 2732–2744. doi:10.1016/j.egyr.2023.01.098
Matkivskyi, S. (2022). Increasing hydrocarbon recovery of Hadiach field by means of CO2 injection as a part of the decarbonization process of the energy sector in Ukraine. Min. Min. Depos. 16, 114–120. doi:10.33271/mining16.01.114
Mokhtari, R., Anabaraonye, B. U., Afrough, A., Mohammadkhani, S., and Feilberg, K. L. (2022). Experimental investigation of low salinity water-flooding in tight chalk oil reservoirs. J. Pet. Sci. Eng. 208, 109282. doi:10.1016/j.petrol.2021.109282
Nevmerzhitskiy, Y. (2022). Development of production decline curves for non-Darcy oil flow in low-permeability reservoirs. J. Pet. Sci. Eng. 218, 111039. doi:10.1016/j.petrol.2022.111039
Nosova, A., Petrov, S., Safiulina, A., Kayukova, G. P., and Bashkirceva, N. (2018). The transformation of high-viscosity oil of carbonate rock in the presence of CO[AcAc]3 catalyst in a vapor-air medium. Pet. Sci. Technol. 36, 1001–1006. doi:10.1080/10916466.2018.1458121
Pu, W., Zhao, S., Hu, L., Varfolomeev, M. A., Yuan, C., Wang, L., et al. (2020). Thermal effect caused by low temperature oxidation of heavy crude oil and its in-situ combustion behavior. J. Pet. Sci. Eng. 184, 106521. doi:10.1016/j.petrol.2019.106521
Ren, S. R., Greaves, M., and Rathbone, R. R. (2002). Air injection LTO process: an IOR technique for light-oil reservoirs. SPE J. 7, 90–99. doi:10.2118/57005-PA
Shi, J., Cheng, L., Cao, R., Fang, J., Yang, C., Liu, G., et al. (2023). Analysis and quantitative evaluation of temperature influence mechanism of multi-cycle water huff-n-puff in ultra-low permeability reservoirs. Energy 263, 125600. doi:10.1016/j.energy.2022.125600
Verşan Kök, M., Varfolomeev, M. A., and Nurgaliev, D. K. (2017). Thermal characterization of crude oils in the presence of limestone matrix by TGA-DTG-FTIR. J. Pet. Sci. Eng. 154, 495–501. doi:10.1016/j.petrol.2017.02.001
Verşan Kök, M., Varfolomeev, M. A., and Nurgaliev, D. K. (2021). Effect of inlet pressure on crude oil combustion -laboratory approach-. J. Pet. Sci. Eng. 198, 108174. doi:10.1016/j.petrol.2020.108174
Wang, Z.-H., Sun, B.-W., Guo, P., Wang, S.-S., Liu, H., Liu, Y., et al. (2021). Investigation of flue gas water-alternating gas (flue gas–WAG) injection for enhanced oil recovery and multicomponent flue gas storage in the post-waterflooding reservoir. Pet. Sci. 18, 870–882. doi:10.1007/s12182-021-00548-z
Yuan, C., Emelianov, D. A., Varfolomeev, M. A., Rodionov, N. O., Suwaid, M. A., and Vakhitov, I. R. (2021). Mechanistic and kinetic insight into catalytic oxidation process of heavy oil in in-situ combustion process using copper (Ⅱ) stearate as oil soluble catalyst. Fuel 284, 118981. doi:10.1016/j.fuel.2020.118981
Yuan, C., Pu, W., Ifticene, M. A., Zhao, S., and Varfolomeev, M. A. (2022). Crude oil oxidation in an air injection based enhanced oil recovery process: chemical reaction mechanism and catalysis. Energy fuels. 36, 5209–5227. doi:10.1021/acs.energyfuels.2c01146
Zeng, D., Dong, B., Zhang, S., Yi, Y., Huang, Z., Tian, G., et al. (2022). Annular corrosion risk analysis of gas injection in CO2 flooding and development of oil-based annulus protection fluid. J. Pet. Sci. Eng. 208, 109526. doi:10.1016/j.petrol.2021.109526
Zhai, M., Zhou, K., Sun, Z., Xiong, Z., Du, Q., Zhang, Y., et al. (2023). Rheological characterization and shear viscosity prediction of heavy oil-in-water emulsions. J. Mol. Liq. 381, 121782. doi:10.1016/j.molliq.2023.121782
Zhang, R., and Hao, C. (2022). Research on the development of hydraulic flushing caverning technology and equipment for gas extraction in soft and low permeability tectonic coal seams in China. ACS Omega 7, 21615–21623. doi:10.1021/acsomega.2c01465
Zhang, T., Ming, T., Yuan, L., Zhu, G., Zhang, C., Liu, Y., et al. (2023). Experimental study on stress-dependent multiphase flow in ultra-low permeability sandstone during CO2 flooding based on LF-NMR. Energy 278, 127874. doi:10.1016/j.energy.2023.127874
Zhao, S., Gao, H., Pu, W., Varfolomeev, M. A., and Yuan, C. (2022). Isothermal oxidation behavior of heavy crude oil and its low-temperature oxidized oils: implications for in-situ upgrading of heavy oil. Fuel 313, 122704. doi:10.1016/j.fuel.2021.122704
Zhao, S., Pu, W., Chen, Q., Yuan, C., and Varfolomeev, M. A. (2024). Propagation of combustion front within fractured shale and its influence on shale structure and crude oil properties: an experimental study. SPE J. 29, 2389–2398. doi:10.2118/219456-PA
Zhao, S., Pu, W., Gao, H., Wang, R., Varfolomeev, M. A., Jiang, Q., et al. (2023). Isothermal low-temperature oxidation kinetics of heavy crude oil and its oxidized oils. Fuel 344, 128146. doi:10.1016/j.fuel.2023.128146
Keywords: low-permeability reservoir, high-pressure air, low-temperature oxidation, component, oil recovery
Citation: Chen X, Ba Z, Lu Z, Gao Y, Zhou Y and Li X (2024) Evaluation of low-temperature oxidation analysis and the development effect of high-pressure air injection in low-permeability reservoirs. Front. Earth Sci. 12:1416824. doi: 10.3389/feart.2024.1416824
Received: 19 April 2024; Accepted: 13 June 2024;
Published: 10 July 2024.
Edited by:
Vasyl Lozynskyi, Dnipro University of Technology, UkraineReviewed by:
Kanay Rysbekov, Satbayev University, KazakhstanCopyright © 2024 Chen, Ba, Lu, Gao, Zhou and Li. This is an open-access article distributed under the terms of the Creative Commons Attribution License (CC BY). The use, distribution or reproduction in other forums is permitted, provided the original author(s) and the copyright owner(s) are credited and that the original publication in this journal is cited, in accordance with accepted academic practice. No use, distribution or reproduction is permitted which does not comply with these terms.
*Correspondence: Xinyu Chen, cGFwZXJfMjAyMkAxNjMuY29t
Disclaimer: All claims expressed in this article are solely those of the authors and do not necessarily represent those of their affiliated organizations, or those of the publisher, the editors and the reviewers. Any product that may be evaluated in this article or claim that may be made by its manufacturer is not guaranteed or endorsed by the publisher.
Research integrity at Frontiers
Learn more about the work of our research integrity team to safeguard the quality of each article we publish.